- 1Surface Waters – Research and Management, Swiss Federal Institute of Aquatic Science and Technology (Eawag), Kastanienbaum, Switzerland
- 2Energy Systems, SINTEF Energy Research, Trondheim, Norway
- 3Laboratory of Hydraulics, Hydrology and Glaciology (VAW), ETH Zurich, Zurich, Switzerland
As the demand for hydroelectricity progresses worldwide, small hydropower operators are increasingly examining the feasibility of using existing infrastructure (e.g., settling basins) in run-of-the-river schemes for intermittent power production. Such flexible production causes short-term discharge fluctuations (hydropeaking) in downstream reaches with potential adverse effects for the sensitive fauna and flora in alpine streams. In an experimental field study on a previously unregulated section of the upper Rhone River (Switzerland), we measured density and composition of macroinvertebrate drift in two habitats (riffle, pool) following a 15-minute hydropeaking wave. The experimental hydropeaking was replicated five times over 14 days with decreasing recovery times between peaks (8, 3, 2 days, and 24 h), and drift measurements were compared with kick samples for the benthic community. Results from the kick sampling showed that benthic macroinvertebrate abundance and composition did not significantly change between the experimental peaks. There were habitat specific reactions in macroinvertebrate drift to hydropeaking, with the pool experiencing more pronounced drift abundances than the riffle. Overall, drift abundance was not significantly correlated with recovery time, but results indicate taxa-specific differences. This research advocates for the importance of completing more in-situ field experiments in order to better understand the ecological impact of flexible power production in small hydropower plants.
Introduction
Recent growth in energy demand has accelerated human reliance on hydropower, stimulating an increase in construction of new hydropower plants around the globe (Zarfl et al., 2015; Bejarano et al., 2018; Bejarano et al., 2019). Hydropower is now the leading form of market-based renewable energy (Jager et al., 2015), and demand is expected to escalate for the next 30 years (Zarfl et al., 2015; Mihalicz et al., 2019). There is, however, limited growth potential for new hydropower operations in many parts of the world, and the negative ecological impacts of large hydropower plants are well known (Poff et al., 2007; Ellis and Jones, 2013; Miller and Judson, 2014). In light of this, much attention is being focused on the endorsement of small hydropower plants (SHPs; Anderson et al., 2015).
SHPs are usually defined as facilities with installed capacity of <10 MW. Because of their size, there is a perception that SHPs have fewer adverse ecological impacts than large hydropower plants (Kibler and Tullos, 2013; Anderson et al., 2015) and some governments, such as those in the EU, United Kingdom, and in Switzerland, are currently subsidizing SHP construction (Anderson et al., 2015; Lange et al., 2018). In reality, the ecological footprint of SHPs per megawatt of power produced may actually be significantly higher than that of the large hydropower plants (Ziv et al., 2012; Kibler and Tullos, 2013). Research towards understanding the ecological impact is gaining momentum as there is a growing number of operational and prospective SHPs (Anderson et al., 2015).
SHPs are typically operated as run-of-the-river schemes allowing for continuous production of power. Increasingly, SHP operators are evaluating opportunities to use existing infrastructure (e.g., settling basins) for sub-daily storage of limited volumes of water to meet fluctuating market demands. As a consequence of varying power production with demand, downstream rivers experience drastic daily and sub-daily fluctuations in streamflow and stage, a phenomenon widely known as hydropeaking (e.g., Carolli et al., 2015; Tonolla et al., 2017). Because of the heterogeneous nature of hydropeaking and (small) hydropower plant design and operation, considerable variability exists in peak frequency, magnitude, duration, and potential to optimize operations with respect to the natural environment.
In recent decades, research focused on characterizing and quantifying the complex spatial and temporal impacts hydropeaking has on aquatic habitats, organisms, and ecosystem processes. A large variety of effects has been observed such as stranding of fish (Bell et al., 2008; Tuhtan et al., 2012; Auer et al., 2017), obstruction to fish migration patterns (Jones and Petreman, 2015), changes in food sources (Lauters et al., 1996; Lagarrigue et al., 2002), degradation of habitat quality (García et al., 2011), impairment of flood-intolerant river bank vegetation (Bejarano et al., 2020) and macrophytes (De Jalon et al., 1994), sharp fluctuations in river temperature (Zolezzi et al., 2011; Vanzo et al., 2016), and modifications of natural rates of sediment transport (e.g., Anselmetti et al., 2007; Petticrew et al., 2007). Hydropeaking has also been shown to impact a river ecosystem’s resilience towards disturbance, such as droughts or floods; for instance, regular catastrophic flooding induced by hydropeaking can deplete benthic macroinvertebrate communities thereby reducing resource pools for recolonization (Gibbins et al., 2010; Feeley et al., 2012), natural biological recovery and thus regional biodiversity (Feeley et al., 2012).
Benthic macroinvertebrates (re)colonize via drift during their aquatic larval stages (Robinson et al., 2004a; Gibbins et al., 2010; Bruno et al., 2016). Using an experimental channel, Mochizuki et al. (2006) found that the duration of stable flow conditions before each peak was a significant determinant of drifting macroinvertebrate abundance. A longer recovery time between peak events resulted in higher drift abundance of macroinvertebrates. Similarly, in a recent flume study, Bruno et al. (2016) showed that over five consecutive days with daily hydropeaks of 5 h duration, macroinvertebrate drift strongly declined after an initial catastrophic drift at the first hydropeak, as the time and the proportion of incoming drift likely affected recolonization in between peaks. Along these lines, researchers often assume that a recolonization period of at least 20–60 days is sufficient to provide a full recovery for drift experiments (see Mochizuki et al., 2006; Bruno et al., 2016). However, a clear understanding of the lower limit of the necessary duration of recovery, let alone the factors that control this duration, is still lacking. As a 20–60 days estimate of peak-free time is incompatible with societal energy demands, it is critical to know how peaking frequency changes drift patterns. This relationship might provide information on the resilience of the benthic community in natural systems undergoing new anthropogenic changes. Community resilience can vary on multiple spatial and temporal scales and is known to depend on the integrity of the biological community (Doretto et al., 2018), in particular the ecological condition of the upstream reach (Bruno et al., 2016).
Despite recent scientific interest in this area, our knowledge of the complex ecological impacts of hydropeaking and of efficient mitigation strategies is still limited (Miller and Judson, 2014; Tonolla et al., 2017). For the numerous SHPs, often proposed for complex and sensitive alpine ecosystems, frequent short-duration hydropeaking events will become more common, particularly during the colder months when energy demand is high and discharge in alpine streams is generally low. Many flow regulation studies rely either on large hydropower plants (see Robinson et al., 2003; Miller and Judson, 2014), simulate artificial high flows that last at least 50 min (see Mochizuki et al., 2006), take place in experimental flumes and channels (Poff and Ward, 1991; Bruno et al., 2010; Bruno et al., 2016; Doretto et al., 2018; Schülting et al., 2019), or are carried out in summer or early fall (Mihalicz et al., 2019). Though these studies are significant, none of these study design variables are consistent with the newly flexible operations of a SHP in natural alpine environments. Furthermore, Kjaerstad et al. (2018) found that, in the same regulated river, drift density significantly differed between shallow and deep habitats.
How hydropeaking alters the abundance and composition of macroinvertebrates in downstream river ecosystems, including benthic communities, should be measured and evaluated at all relevant timescales (Miller and Judson, 2014) in order to balance societal energy needs with the ecological integrity of freshwater ecosystems. Our research attempts to shed light on the influence of hydropeaking frequency, i.e., on the recovery time, on macroinvertebrate communities downstream from a SHP, under consideration of habitat-specific responses.
Our field study was conducted in late fall on the upper Rhone River in the Swiss Alps with a newly operational SHP performing hydropeaking for the first time on this river section. The study is part of SmallFlex, an interdisciplinary research project which evaluates options to increase the production flexibility of this SHP using existing infrastructure. Experimental hydropeaks of short duration were produced using the settling basin and the forebay tank as storage volume. The recovery time between the hydropeaks was progressively shortened from eight days after the first peak to one day before the last peak. The benthic and the drifting macronivertebrate community were sampled in the floodplain downstream of the hydropower plant release, and at an upstream location in the residual flow reach. Sampling was performed in two different habitat types, riffle and pool, because the taxonomic structure of macroinvertebrates is known to differ between riffle and pool habitats in headwater streams (Herbst et al., 2018), and therefore the community could potentially react differently to the hydropeaks.
With this experimental setup, we tested the following three hypotheses. 1) The hydropeaking experiment causes a reduction in abundance of hydropeaking sensitive taxa and a shift in community composition of benthic and drifting macro-invertebrates during base flow as compared to the upstream site unaffected by hydropeaking. 2) In response to the progressively decreasing recovery times between experimental hydropeaks, the abundance of sensitive taxa decreases, resulting in a shift in the composition of the drifting macroinvertebrate community during the hydropeaking wave when we compare samples from different experiments. 3) The response of both the abundance and the community composition in the impacted reach to the hydropeaking depends on the hydro-morphological characteristics of the site, and is thus different between the pool and the riffle habitats.
Materials and Methods
Study Area
The Gletsch–Oberwald SHP was completed in 2018 and is located at 46°32′37.39″N, 8°21′42.73″E on the upper Rhone River, Valais, Switzerland (Figure 1). The Rhone River flows from the Rhone Glacier at an altitude of 2,208 m a.s.l. through a near-natural floodplain of national importance and into the area of Gletsch, where the water is captured by the SHP at 1,750 m a.s.l. The water is returned to the river at 1,450 m a.s.l below a steep residual flow reach of 2.7 km length into the upper part of another floodplain of national importance upstream of the town of Oberwald. The hydrological regime of the Rhone River is glacier-fed, with average monthly discharge ranging between ∼0.25 m3/s from January to March and ∼8.6 m3/s in July and August (average discharges for the years 1956–2017 at the gauging station 2,268 of the Swiss Federal Office for the Environment in Gletsch; Supplementary Figure S1). The residual flow reach is fed with a general minimum discharge of 0.2 m3/s and a higher minimum discharge of 0.75 m3/s in September. In the summer months, the flow often exceeds the capacity of the SHP of 5.7 m3/s.
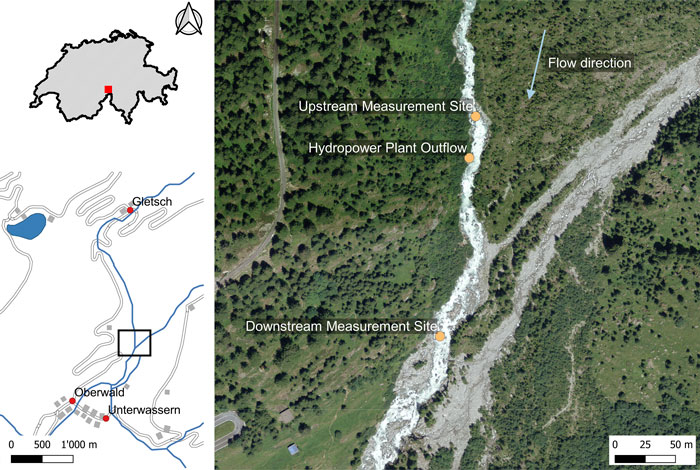
FIGURE 1. Location of the Gletsch–Oberwald SHP and the two measurement sites for the field experiment. Incoming tributaries between measurement sites are intermittent (map generated using data from GeoVITe, 2019); data source: Federal Office of Topography.
The Gletsch–Oberwald SHP is operated as run-of-the-river, but part of its infrastructure could potentially be used as storage volume to provide flexible power production. In particular, tests were made within the SmallFlex project to store up to 2,460 m3 of water in the settling basin and the forebay tank. This accumulated volume allows for short peaks of 60 min duration at most. However, future operations with peak durations of 15 and 30 min were considered more likely.
Experimental Design and Sampling Sites
The experimental field study was scheduled over a two-week period in November 2018, during near representative late autumnal conditions. Five experimental hydropeaks of equal magnitude (2.6 m3/s) and duration (15 min) were performed on five different days (Nov. 8, 16, 19, 21, 22), allowing for recovery times between peaks of 8, 3, 2 and 1 day (Table 1). All peaks were performed at 13:00h, with minor delays for peak 4 (4 min delay) and peak 5 (2 min delay). Prior to each peak (09:00 h), all study parameters (benthic macroinvertebrates, drift, abiotic variables) were surveyed to represent undisturbed baseflow conditions for comparison.

TABLE 1. Summary table of basic site information including date of each experiment, average water temperature [HOBO Loggers (°C)], notes on weather, daily air temperature (°C) minima and maxima, and the duration of recovery time before each additional experiment (days).
There were two measurement sites (see Figure 1) with an elevation difference of about 75 m: 1) an upstream site 30 m upstream from the SHP outflow which was not affected by the experimental hydropeaking, and 2) a downstream site at 600 m distance in a floodplain which experienced experimental hydropeaking. Upstream and downstream measurement sites were intended to resemble each other as much as possible. It is worth clarifying that the upstream site is located in the residual flow reach, as sampling beyond the residual flow reach, i.e. upstream of the water capture, was impossible for logistical reasons (road closed for winter). Moreover, the upstream site is located in a steep and narrow channel within a small canyon and is characterized by step-pools with few adjacent riffles. The downstream site, located in a small floodplain, instead offers a series of separate riffles and pools. Two benthic mancroinvertebrate collection methods were employed on the riffle and pool habitats of the two measurement sites: 1) drift-net sampling, and 2) kick-net sampling.
Macroinvertebrate Drift Sampling
For the drift-net sampling, standard drift nets (25 × 40 cm) with a mesh size of 500 µm were used to collect drifting invertebrates. A mesh size of 500 µm was chosen to compensate for the greater probability during the fall months of clogging by leaf litter and organic matter, and the nets were frequently checked for clogging. All drift measurements were made with three nets per habitat. The three nets were placed directly next to each other in a transect across the channel at the same locations on each day, so that temporal and spatial comparisons could be accurately made. At both the upstream and downstream site two physical habitats were sampled: riffle and pool. The physical habitats were selected based on expert knowledge, and were expected to be persistent during the set of experiments.
The upstream channel width was 7.86 m with riffle and pool habitats immediately adjacent, providing convenient drift sampling. The downstream riffle was located in a 2.63 m wide side channel, which flows into and out of the main river channel. The downstream pool was located in a separate 3.45 m wide side channel, which also flows into and out of the main channel. They were approximately 10 m walking distance apart with the flow of water being distinct for each habitat. Therefore, any sampling influence on each another was eliminated.
The net frames were left in place during each experiment, where new nets were mounted to the frames for each individual sample. Before each experimental peak, baseflow drift was measured for a 60 min period both upstream and downstream and one sample was taken per net (sample 0; Figure 2). During and after each experimental peak, five different samples were taken from each drift net (samples 1–5): sample 1 (first stage of the rising limb; 5 min); sample 2 (second stage of the rising limb; 5 min); sample 3 (peak; 5 min); sample 4 (peak transition to falling limb; 20 min); sample 5 (falling limb to post-peak; 40 min). Peak sampling times (samples 1–3) were shortened to 5 min per sample to achieve a higher sampling resolution for the 15 min peak period and to ensure that identification of drift dynamics during the peak would be possible (Figure 2).
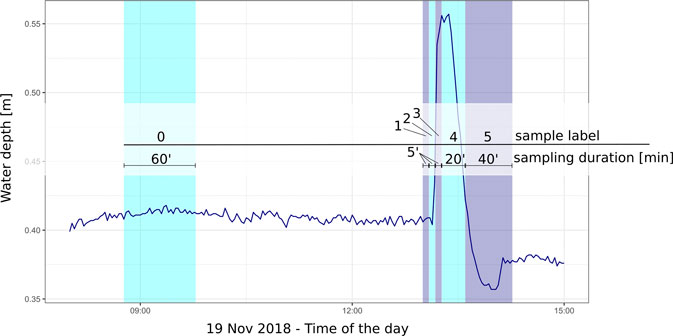
FIGURE 2. Timing of the sampling (experimental peak 3 shown as example); the blue line represents the water depth recorded in the main channel at the downstream site during the experiment. Vertical coloured bands identify the six drift samplings performed. Once the 15 min peak ended, the hydropower plant would then refill the storage volume, resulting in a water level lower than baseflow conditions for approximately 30 min.
Macroinvertebrate Kick Sampling
Benthos kick samples were collected using a 500 µm mesh D-frame net in an area of between 30–43 m2 at the downstream sites and 17 m2 at the upstream site. At each site, five replicates at 30 s intervals were taken at each habitat and before every experiment. In order to avoid interference with the drift sampling, kick samples were taken downstream of drift sampling locations. Owing to the limited habitat options at the upstream site, we were unable to differentiate between riffle and pool in the kick samples. Consequently, no habitat differentiation was recorded for the upstream kick samples, and habitat-specific analysis were only performed for the effects of hydropeaking in the downstream location.
Sample Treatment and Identification of Taxa
After collection, the drift and kick samples were immediately conserved with 70% ethanol and transferred to the laboratory. The samples were sorted in the lab with 0.25 mm sieve and white buckets. They were sorted and then identified using a ZEISS Stemi 508 Greenough Stereo Microscope. Swiss specific keys were used to identify Ephemeroptera (Studemann et al., 1992) and Plecoptera (Lubini et al., 2012). The Waringer and Graf (2011) key was used to identify Trichoptera, and the Mauch (2017) key to identify Diptera. All other taxa were identified using Tachet et al. (2000) and Dobson et al. (2012). As most of the invertebrates were in the early or pupa stages of their life cycle, all were identified at family level only and in some cases order (eg, Tubellaria, Collembola, and Hydracarina).
Physical and Chemical Parameter Sampling
To calculate the filtered water volume during the drift samplings and to characterize the physical and chemical conditions of the experiments, several parameters were recorded. Flow velocity was measured at three locations in front of each net (left, middle, and right), at sixty percent depth (as seen in Irvine, 1985; Imbert and Perry, 2000) using a MiniAir2 velocity meter (Schiltknecht AG, Gossau, Switzerland) at least once during each peak sample (samples 1–3) and at least four times during longer sampling periods of pre and post-peak (samples 0, 4, and 5). Average velocity values were computed by averaging all measurements during a particular stage (e.g., averaging three locations in front of each net × 3 nets × 5 repetitions = 45 samples during experiment 1, baseflow). Velocity maxima were computed as maximal values of net-averages (e.g., three net-averages × 5 repetitions = 15 samples during experiment 1, baseflow). The observed flow velocities and corresponding water depths, measured in front of the nets with a rigid meter, allowed for an accurate calculation of the filtered volume v [m3] of water during each sampling. In particular, a standard trapezoidal rule was applied to spatially and temporally integrate the point measurements over the net cross-sectional area and sampling interval. Turbined discharge data was provided by Forces Motrices Valaisannes (FMV).
The water temperature (°C) and the total pressure (kPa) were recorded with three HOBO U20L-04 temperature loggers throughout November with a time set interval of every 2 min, using local time. The loggers were placed at three different locations: 1) at the upstream measurement site, 2) just below the hydropower plant outflow, and 3) at the downstream measurement site. Additionally, a fourth HOBO logger was installed at the downstream measurement site to measure the air temperature and atmospheric pressure (see Figure 1).
The water quality/chemistry parameters of temperature, specific conductivity, dissolved oxygen, pH, and turbidity, were taken at the upstream and downstream sites before each peak using a WTW MultiLine® 3630 IDS portable meter, and the Hach 2100Q Turbidity meter, respectively. Water quality parameters were also taken at the downstream site during peak and immediately after each peak event.
We sampled the substrate of each habitat prior to each experiment, paying attention not to perturb the biological sampling area (i.e. the area upstream the nets). The substrate was sampled following the Wolman approach (Wolman, 1954) with a Wildco Gravelometer, and analysed in terms of grain size distribution.
Data Analysis
The data analysis was composed by two parts: first the raw collected data were consolidated in a unique dataset. Second, a set of statistical analysis tests was applied to the consolidated dataset. The dataset includes 209 drift samples and 75 kick samples. One post-peak drift sample had to be excluded from the study due to faulty sample preservation. Staphylinidae and Collembola were identified as semi-aquatic and collectively comprised of <0.5% of the total number of animals, therefore, they were excluded from the analysis. Rare taxa and Crambidae were taken out of the analysis as well, as collectively they accounted for <0.005% of the animals. Terrestrial taxa (e.g., winged individuals) were also excluded from the analysis as they made up less than 0.02% of the total animals and were out of the scope of the research. Diamesinae accounted for 0.007% of the total animals, and as they are considered a subfamily of Chironomidae, they were tallied in with Chironomidae. The drift of macroinvertebrate is discussed in terms of abundance and density. The abundance is expressed as number of individuals [ind], while the density ρ [ind/m3] is obtained by dividing the abundance with the filtered volume of water [m3] during each sampling interval. This conversion to density metric is needed to compare samples having different durations and hydrodynamic conditions. If not differently specified, the data analysis is conducted in terms of drift density.
The assemblages were analysed using PRIMER Version 7 with the PERMANOVA+ add-on (Anderson et al., 2008; Clarke and Gorley, 2015, 2018). We applied a non-metric multidimensional scaling (NMDS) to a resemblance matrix calculated with the Bray–Curtis similarity index applied to the data set. Prior to analysis, the observed densities ρ were log-transformed to log10 (ρ +1) so that the NMDS could depict patterns in species composition and relative drift density.
We performed a permutational multivariate analysis of variance (PERMANOVA) with a similarity percentage (SIMPER) analysis to check for significant differences in the drifting communities and to identify the taxa that most contributed to dissimilarity. For the PERMANOVA, we considered the following factors: “experimental peak” (1–5, continuous variable) to identify the different experiments (i.e. dates); and “sample stage” (0–5) to identify the stage of each sample during each experiment. The factor “net” was considered as nested into the “sample stage” factor. We ran a pairwise comparison within the factors to check for significant differences among experiments and among samples stages. The analysis was run splitting the dataset in two habitats (riffle and pool). The SIMPER calculates the Bray–Curtis Dissimilarity index between two groups and contributions of each taxon to the average dissimilarity between groups. The kick samples have been divided by habitat (riffle and pool, where possible) and were standardized by sampling area. We used the same factor “experimental peak” as applied for the drift analysis. The analysis was performed using PRIMER (Anderson et al., 2008; Clarke and Gorley, 2015, 2018) and R (R Core Team, 2020) with the package “vegan” (Oksanen et al., 2019) and “lmerTest” (Kuznetsova et al., 2017).
Results
Physical and Chemical Characteristics
Flow velocity at the downstream riffle and pool during baseflow and peak conditions is detailed in Table 2. In the riffle, the average peak flow velocity was between −0.02 and +0.16 m s−1 different than baseflow, with peak flow maxima exceeding baseflow maxima by 0.10–0.31 m s−1. In the pool, average velocities increased between 0.00 and 0.14 ms−1 and maxima increased between 0.01 and 0.23 m s−1 from baseflow to peak flow. During the peak, the water level increased on average by 5 cm in the riffle and 2 cm in the pool. Higher average water level increases of 13 cm were observed in the main channel (e.g., Figure 2).

TABLE 2. Downstream (DS) velocities (m s−1) and depths (cm) recorded for both the downstream riffle and the pool, including average and maximum flow velocity and during baseflow (sample 0) and peak flow (sample 3).
Water temperature fluctuated at daily and sub-daily timescales. No clear relationship between water temperatures and drift abundance or density emerged. For each experimental peak, the water temperature dropped by 1°C or less as compared to the baseflow temperature. This thermopeak was evident at the hydropower plant outflow and at the downstream measurement site.
Baseflow downstream turbidity varied between 4.6 and 6.2 NTU. During peak flow the NTU would rise to between 13.4 and 27.8 NTU, and post-peak it would quickly drop back to between 4.1 and 12.0 NTU, though not always to baseflow turbidity values. Prior to each experimental peak, turbidity had returned to the same small range of NTU values. There was no significant correlation between variations in turbidity and drift abundance, density, and/or composition. The upstream turbidity was stable between 2.1 and 4.4 NTU throughout all experiments, and no clear trend in turbidity upstream or downstream was evident, with values oscillating between 6.2 and 8.3 NTU before, during, and after each peak. Similarly, no direct relationship between pH and drift abundance, density and/or composition was noted. Downstream and upstream specific conductivity had sub-daily fluctuations between 101 and 121 μS/cm with no correlation to drift abundance, density and/or composition. Downstream and upstream dissolved oxygen both ranged between 11.5 and 12.7 mg/L throughout all experiments. The downstream dissolved oxygen measurements showed no distinct change when the peak started, and again no correlation was detected between dissolved oxygen and drift abundance, density, and/or composition.
The grain size distributions from the two habitats are shown in Supplementary Figure S2. Riffle habitat (panel A) shows a persistent state throughout all the experiments, with grain size distribution median values ranging between 60 and 80 mm. Pool habitat (panel B) has an initial (i.e., experimental peak 1) grain size distribution with median diameter of 10 mm. For the following peaks (2–5) the grain size distributions show larger median values (40–50 mm) and distribution shapes almost overlapping. A clear coarsening of the substrate was observed after the first experimental peak, whilst there are no further clear trends (i.e. coarsening or refining) during the following peaks.
Macroinvertebrate Abundance and Density
A total of 192,889 individuals were included in the analysis, of which 127,506 individuals in the drift samples and 65,638 individuals in the kick samples. A breakdown of abundance and density for each peak is presented in Table 3. Across all experimental peaks, the downstream pool contained higher drift abundance (85,464 individuals) than the downstream riffle (40,130). The upstream pool also exhibited more drifting individuals (1,063) than the upstream riffle (849). Experimental peak 2 resulted in the highest number of drifting macroinvertebrates in both the downstream riffle (9,654 individuals) and the pool (19,846 individuals).
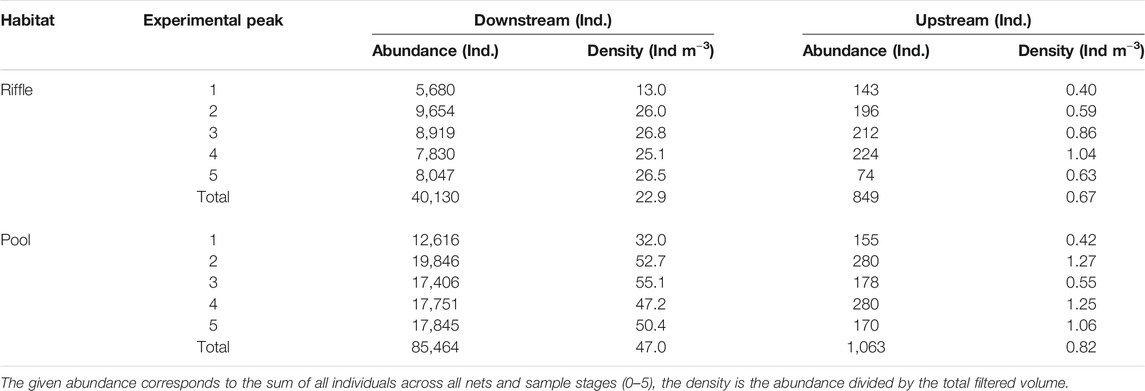
TABLE 3. Abundance and drift density observed at the five experimental peaks in the two habitats at the downstream and upstream measurements site.
An overview of drift density and concurrent flow discharge for net 2 (as a representative example) for all peaks can be found in Figure 3. Relatively low density values are apparent for experimental peak 1 in the riffle habitat, as well as after the peak for the pool habitat (Figure 3). During the 8-days recovery time between peak 1 and peak 2 a considerable rebound in total drift density for the riffle for all three stages occurred (Figure 3).
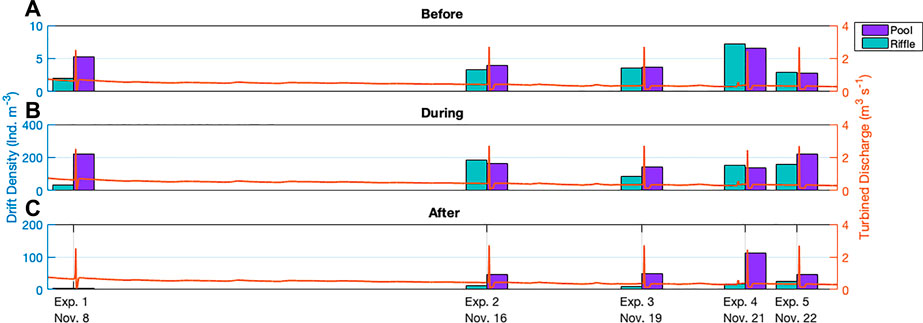
FIGURE 3. Drift density measured at the central net (net 2) for the downstream riffle and pool habitats (A) before (sample 0), (B) during (samples 1–3), and (C) after (samples 4–5) hydropeaking, as example. Samples were combined to better show trends in the individual habitats and account for slight variations in peak timing. The left y-axis shows drift density (ind. m−3) and varies for each stage of the peak. The right y-axis shows the turbined discharge (m3s−1). The x-axis indicates the timing of the experimental peaks.
Before and during the peaks, a decrease in drift density in the pool habitat typically followed a reduction in recovery time. A small exception to this trend transpired during experiment 4 when an unscheduled small release from the hydropower plant caused a brief increase in discharge prior to the major peak (Figure 3). As well, during experiment 5, there is an anomalous increase in pool drift density during the peak that is not evident before or after the peak.
Composition of Macroinvertebrate Community
Altogether, 29 macroinvertebrate taxa were considered in the analysis. This includes a group of unidentified Plecopterans and a group of unidentified Dipterans which could not be identified further than order due to life stage and/or poor sample quality. Of these, 28 taxa were collected in the drift samples with Turbellaria not present, and 26 different taxa were present in the kick samples with Dixidae and Thaumaleidae not present. Overall, Limnephilidae (Drusus ssp.) was the most common taxon, comprising 76.8% of the total individuals. The second most common taxon was Baetidae (5.7%), followed closely by Chironomidae (4.3%). Almost all insect larvae were very early instars.
The taxa contributing the highest abundance in the drift samples were Limnephilidae (Drusus ssp.), Baetidae and Chironomidae (Figure 4). Taxa with the smallest presence in drift samples were Dipterians, such as Stratiomyidae, Blephariceridae, Dixidae, Simuliidae, Psychodidae, Ceratopogonidae, Pediciidae, Tipulidae, Thaumaleidae, Limoniidae, Oligochaeta and Lectura sp. Only three taxa showed considerable propensity to drift, in that they contributed a notable increase in fractional contribution to drift samples compared to kick samples. These taxa and their respective contributions to kick and drift samples are Limnephilidae (54% in kick and 88% in drift samples), Hydracarina (0.15 and 0.5%), and Thaumaleidae (0.0 and 0.1%). Only Limnephilidae had relatively large abundance in both drift and kick samples (>50%). The taxa with the smallest relative difference in fractional contribution to kick and drift samples were those with consistently limited presence, contributing less than 0.05% of the total individuals in both sample sets (Simuliidae, Stratiomyidae, Psychodidae, Blephariceridae, Oligochaeta, Dimesinae).
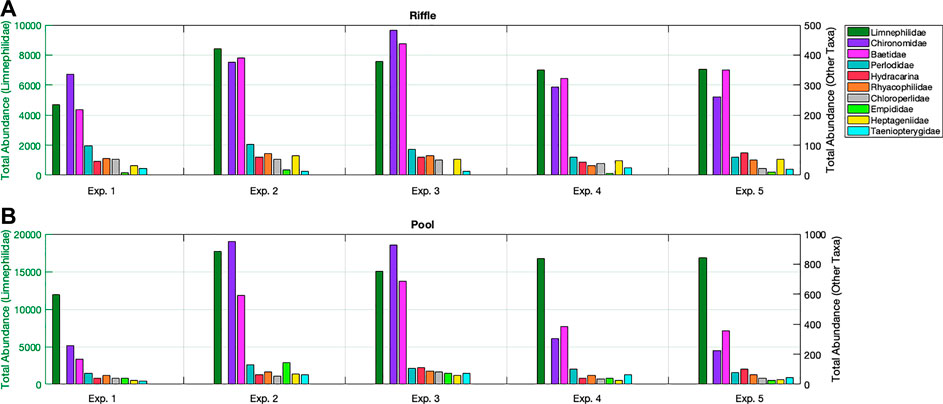
FIGURE 4. Downstream riffle (A) vs. pool (B): total abundance of the ten most common taxa, sum of all samples and nets for each experimental peak. The left y-axis is total abundance of Limnephilidae (Drusus ssp.) and the right y-axis total abundance for all other taxa. The x-axis refers to the experimental peak.
No significant differences were observed between the upstream and downstream site in the base drift macroinvertebrate composition. The p-values for the factor upstream/downstream were 0.81 for the pool and 0.09 for the riffle habitat. In order to assess whether the abundances of specific taxa were changing with decreasing recovery time during the study, the abundances of drifting individuals summed over the three nets for each habitat over all stages of an experiment (i.e., samples 0–5) are presented in Figure 4 for the 10 most common taxa.
In the riffle habitat, Taeniopterygidae, Empididae, Rhyacophilidae, and Hydracarina exhibited no discernible pattern, oscillating between increases and decreases throughout the entire set of experimental peaks (Table 4, Figure 4). Limnephilidae (Drusus ssp.) had the lowest abundance on experimental peak 1. Beyond that date no distinct relationship between Limnepilidae (Drusus ssp.) and recovery time (Table 4; Figure 4) was apparent. The abundance of the remaining taxa adhered to a single peak pattern with lowest values on experimental peak 1 or when recovery time was shortest on experimental peak 5. For example, Perlodidae and Chloroperlidae had their lowest abundance on experimental peak 1 and the highest after 8 days of recovery on experimental peak 2. Both taxa consistently decreased in abundance as the recovery time decreased over the next three experimental peaks (Table 4; Figure 4). Chironomidae, Heptageniidae, and Baetidae exhibited a similar peak in drifting individuals, with low values for experimental peak 1, an inflection point of high values for experimental peak 3, and a decreasing trend to the lowest values for experimental peaks 4 and 5 (Table 4; Figure 4).
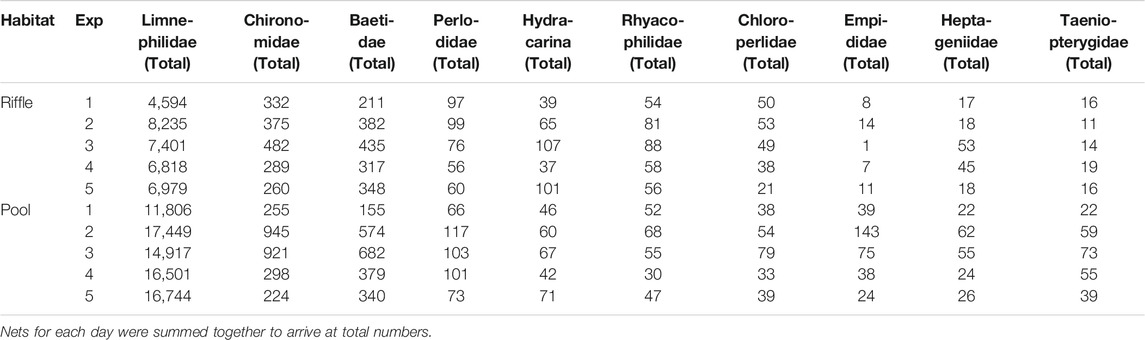
TABLE 4. Abundance of ten most common drifting macroinvertebrate taxa (in descending order) separated by riffle and pool as well as by experiment in the downstream habitats.
In the pool habitat, Limnephilidae (Drusus ssp.) have their lowest abundance on experimental peak 1, after which, any slight fluctuations in Limnephilidae (Drusus ssp.) abundance do not correlate with the varying recovery times (Table 4; Figure 4). Additionally, Chloroperlidae and Taeniopterygidae, and Hydracarina exhibited no discernible pattern in the pool, oscillating between increases and decreases throughout all experimental peaks (Table 4). The remaining taxa again followed a single peak pattern with Chironomidae, Empididae, Heptageniidae, and Perlodidae peaking on experimental peak 2, and Baetidae and Rhyacophilidae peaking on experimental peak 3. These six taxa all decreased in abundance with decreasing recovery time after their initial peak values.
The results of the NMDS showed the characteristic drift patterns during the individual stages of a hydropeaking event (Figure 5). Baseflow drift communities (i.e., sample 0) grouped together for each habitat. In the first stage of the rising limb, the community and abundance changed with the increase in velocity and were less clustered in the NMDS ordination (sample 1). In the second stage of the rising limb, the community shifted towards a more peak-like composition (samples 2–3). The peak stage displayed a distinct clustered appearance indicating similar communities and abundance between experimental peaks, as did the transition to the falling limb (samples 3–4). Post-peak, the community started to shift back towards baseflow drift, but remained slightly altered in composition and abundance compared to base drift (sample 5).
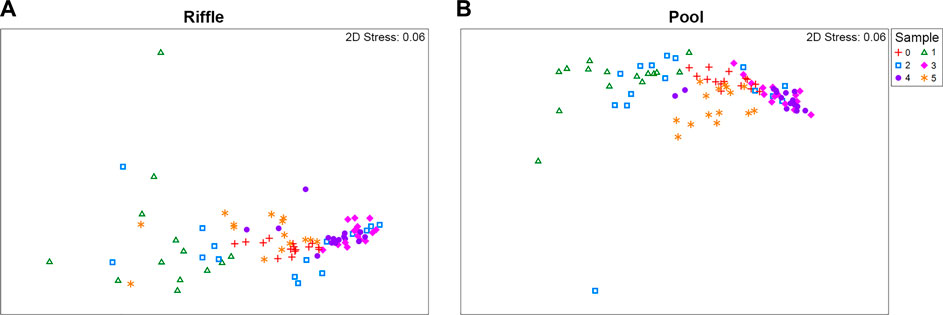
FIGURE 5. Non-metric multidimensional scaling (NMDS) of macroinvertebrate densities in downstream drift samples based on the Bray Curtis Similarity Index for the habitats riffle (A) and pool (B). Crosses: sample 0—baseflow, triangles: sample 1—first stage of the rising limb, squares: sample 2—second stage of the rising limb, diamonds: sample 3—peak, circles: sample 4—peak transition to falling limb, stars: sample 5—post-peak. These figures include all experiments and each individual net.
The drift samples during the hydropeaking wave grouped closer together than did the samples taken during baseflow drift, rising limb, and falling limb (Figure 5). This indicates that the composition and abundance were most similar during each experiment rather than before or after. This similarity is likely related to slight differences in timing of the peak, whereas one sample was always occurring entirely during the peak.
Statistical Tests
Drift Samples: Riffle
PERMANOVA revealed statistically significant effects of the factors “experimental peak” (p = 0.028) and “sample stage” (p = 0.001) on drift composition (Table 5). The factor “experimental peak” accounted for a small part of the variance (R2 = 0.051), while the factor “sample stage” accounted for the largest part of the variance (R2 = 0.405). The PERMANOVA test was not significant for the factor “net” (nested into “sample stage”) which is why we have not indicated differences among nets in the results thus far. Pairwise comparisons highlighted significant differences in composition for the factor “experimental peak” between peaks 1 vs. 5, and 2 vs. 5 (p = 0.032 and p = 0.038, respectively). For the factor “sample stage”, differences are significant for sample 0 vs. 2, 0 vs. 3, 2 vs. 4, 2 vs. 5, 3 vs. 4, and 3 vs. 5. The PERMANOVA test suggests that the drifting community before and after the peak wave is similar, but different during the peak wave.
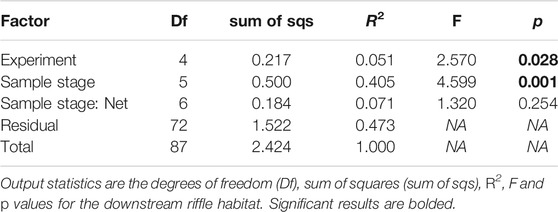
TABLE 5. Statistics showing the results of factors: “experiment”, “sample stage”, and “net” nested in “sample stage”.
The SIMPER analysis shows that the taxa accounting for 90% of the differences for the factor “sample stage” are Drusinae, Baetidae, Chironomidae, Rhyacophilidae, Nemouridae, and Perlodidae. The taxa are similar before and during the experiment, thus suggesting that the drifting community before and during the experiment changes in abundance, but not in community composition.
Drift Samples: Pool
PERMANOVA revealed a highly significant (p < 0.001) relationship between drift composition and the factor “sample stage” and a weaker relationship with the factor “experimental peak” (p = 0.027) (Table 6). The factor “experimental peak” accounts only for a very small fraction of the variance (R2 = 0.007), while the factor “sample stage” accounts for almost half of the variance (R2 = 0.497). The PERMANOVA test was not significant for the factor “net” (nested into “sample stage”) signifying no consistent influence of net location on drift measurements. The pairwise comparison was significant only for experimental peak 3 vs. 5 (p = 0.018). Within the “sample stage” factor, PERMANOVA revealed that the baseflow drifting community (samples 0, 4 and 5) was significantly different from the community during the peak (1, 2, and 3) and that every non-peak sample was significantly different from every peak sample. For each experimental peak, there was always a significant pairwise difference except for samples 1 vs. 2, 1 vs. 3, and 2 vs. 3. That is, the drifting communities during the peak were not significantly different from each other.
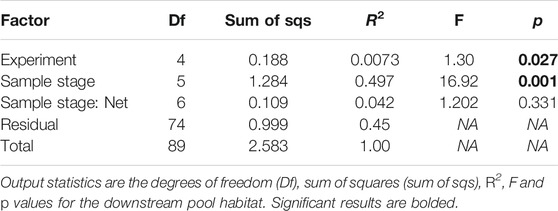
TABLE 6. Statistics showing the results of factors: “experiment”, “sample stage”, and “net” nested in “sample stage”.
The SIMPER analysis of the community demonstrates that five families (Drusinae, Baetidae, Chironomidae, Rhyacophilidae, and Perlodidae) account for 91% of the differences within the factor “experimental peak”. Among these families, the consistently most abundant taxa, Drusinae, accounts for 70% of the differences. These same families are the most relevant for the factor “sample stage”. It is important to note that the taxa are similar before and during the experiment, thereby suggesting that the drifting community before and during the experiment changes primarily in drift densities, but not in community composition.
Kick Samples
Using the PERMANOVA analysis with the factor “experimental peak”, we did not detect a significant change in the benthos community in the downstream riffle or pool (p = 0.071 and p = 0.083, respectively). Therefore, any change that was observed in the drift community is unlikely to be a result of changes in the kick community. However, our model accounted only for a small fraction of the variance (R2 = 0.11 for riffle and R2 = 0.12 for pool). Reductions in benthic densities were not present. Using the PERMANOVA analysis and the factor “experiment” for the upstream site, the analysis again showed no significant differences among benthos samples, demonstrating that the macroinvertebrate kick community changes are not significant. Visible decreases in kick abundance from experimental peak 1 to last peak 5 (Figure 6) exist, but the coarse data likely prevented a statistically significant p-value.
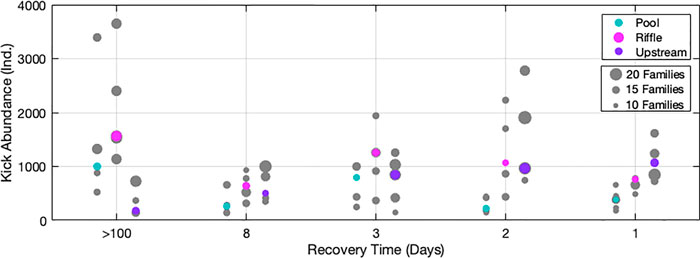
FIGURE 6. Habitat-specific kick sample abundances. The x-axis shows recovery time with five replicates per experimental peak from the upstream, and two downstream habitats (riffle and pool); y-axis shows total abundance. The dots are proportional to the number of families in the sample. The dots also include taxa Turbellaria, Collembola, and Hydracarina though they were only identified to order level. The colored dots indicate the median samples for each habitat.
Discussion
Macroinvertebrates During Baseflow Conditions
The observed data did not confirm our hypothesis that the abundance and composition of benthic and drifting macroinvertebrates would be significantly different in the affected river section compared to the unaffected upstream site. There was no significant difference during baseflow conditions between the upstream and the downstream locations for both the kick and the drift sampling. This was further supported by the NMDS analysis, which indicated that the drifting community composition quickly returned to baseflow conditions after each peak.
The NMDS analysis showed consistent patterns in the composition and drift density of macroinvertebrate drift across all experimental peaks (Figure 5). The results showed five distinct stages of invertebrate drift: baseflow drift, rising limb, peak, falling limb, and post-peak. During our experimental peaks, the largest drift densites were predominantly found in sample 3 – peak stage, at the start of the highest flow. Drift density changes occurred quickly during the peak when also the composition differed from baseflow drift samples. In post-peak, the community composition and drift density was only marginally different from baseflow drift, and was closer to baseflow drift community composition and density than during peak.
These findings are consistent with previous flume, channel flow and field studies. For example, Mochizuki et al. (2006) conducted an experiment using artificial high flows in an experimental channel and found distinct rising limb, peak, and falling limb patterns in their drift samples. Bruno et al. (2016) described four distinct stages of macroinvertebrate drift in their hydropeaking simulations: baseflow drift (pre-hydropeaking drift), initial maximal peak drift, lower drift for the remainder of the peak (becoming closer to baseflow drift), and altered baseflow drift (post-hydropeaking). Similarly, in a field study, Bruno et al. (2010) observed significant differences between the drifting macroinvertebrate community composition before and during a single hydropeaking event at varying distances from the hydropower plant discharge.
Effects of Recovery Time on Macroinvertebrate Abundance and Composition
In summary, our observations showed no statistically significant effects of reducing the recovery time in the range from eight days down to one day on macroinvertebrate abundance and the overall community composition during the two weeks of our field study. However, at the level of individual taxa, the data indicated decreasing abundances of certain taxa in the course of the experiment. We therefore suggest that long-term research be conducted on rivers that move from natural or near-natural to affected by hydropower production, to fully assess the impacts on benthic communities of peak frequency from flexible small hydropower peaking. This assessment is based on the results of our study as discussed in the following paragraphs.
Effect of Recovery Time on Benthic Abundance and Density
Observed drift and kick communities had very similar assemblages, and we did not detect significant change in the abundance and composition of the benthos community of either the upstream or downstream riffle or pool. Therefore, it is unlikely that any observable change in the drift community abundance and/or density resulted from changes in the benthic community. Stability in the benthos community is not unusual. Miller and Judson (2014) assessed the impact of double-peak release patterns on macroinvertebrate drift, benthic assemblages, and fish consumption and observed no reductions in benthic densities; Imbert and Perry (2000) compared abrupt flow to stepwise flow increase experiments and did not detect changes in benthic densities in either experiment type (see also Scullion and Sinton, 1983; Irvine and Henriques, 1984). One possible explanation for the negligible effect of recovery time on the benthos community is that the abundance of invertebrates drifting may have constituted only a small proportion of the total benthos present (Irvine and Henriques, 1984). It is also possible that there was enough continuous recolonization and the hydropeaking waves did not have any consequence on the benthos community. We did note a slight decrease in the downstream benthic densities across all experimental peaks. This decrease was not statistically significant though, and could have been the result of either fewer drifting macroinvertebrates or organisms burrowing into the substrate to avoid scour, making them less easy to capture (Irvine, 1985) through kick sampling.
Despite 15 min peaks leading to a 15–69% increase in maximum velocity in the riffle, and a 2–114% increase in maximum velocity in the pool (Table 2), results from this study suggest that disturbances caused by these peaks were not large enough or long enough to permanently alter the benthic invertebrate communities. Miller and Judson (2014) suggest that stability in the benthic densities could be the result of non-bed mobilizing flows, while Imbert and Perry (2000) suggest that minor drift losses from the benthos during short time intervals may eventually reduce populations if these reductions accrue over consecutive generations (Hershey et al., 1993) or if emigration outweighs immigration (Imbert and Perry, 2000).
Effect of Recovery Time on Drift Abundance and Density
Previous experiments such as those completed in experimental channels and flumes have reported a high abundance of drifting macroinvertebrates on the first day of peak experiments, likely due to several weeks or months without anthropogenic modification of flow (see Irvine and Henriques, 1984; Irvine, 1985; Bruno et al., 2016). Our results contradict those findings. In fact, on our first experimental peak (Nov. 8), we observed the lowest total abundance of drifting macroinvertebrates, in both the riffle and the pool (for all samples added together), and the highest abundance, in both the upstream and downstream habitats, after only eight days of recovery (experimental peak 2). We hypothesize that a precipitation event that lasted for the three days prior to the experimental peak 1 caused naturally high flows and a reduction in total taxa present. At the Swiss Federal Office gauging station 2,419 “Rhone–Reckingen” (located 11 km downstream from the study site), the three days immediately prior to the first experimental peak had an average discharge of 9.24 m3s−1 (max 10.9 m3s−1), whereas the average streamflow for the experiment days was 4.59 m3 s−1 (max 7.92 m3 s−1). Studies completed by Lauters et al. (1996) and Kjaerstad et al. (2018) support this hypothesis as they observed low impacts of hydropeaking on benthic macroinvertebrate densities at high natural flows. In comparison with the flume and channel flow studies, the natural environment of this study offered fewer variables within our control, and the magnitude of individual samples prohibited repeated sampling for each recovery time. Our analysis did not detect any significant differences between experiments with various recovering time. The study has been conducted in an area where a recolonization from upstream un-impacted river reaches is possible. Moreover, the area was previously undisturbed and not impacted by hydropeaking, thus being able to recover quickly from alterations (i.e., lesser precariousness, higher resilience, Walker et al., 2004). Different patterns could result from similar experiments in heavily modified reaches.
Taxa Specific Responses
The composition of drift during our peak was similar to that reported elsewhere (see Bruno et al., 2010), with juvenile instars of Ephemeroptera, Plecoptera, Tricoptera, and Diptera as dominant taxa. Most of our taxa were early larval instars which have been shown by Schülting et al. (2016) to influence drift abundance. More specifically, our peak drift samples had a high abundance of high flow sensitive species: Baetidae (Ephemeroptera), Rhyacophilidae (Tricoptera), Limnephilidae (Tricoptera), and Chironomidae (Diptera) (Kjaerstad et al., 2018). Both Chironomidae and Baetidae are organic matter associated taxa (Bruno et al., 2016) and large amounts of organic matter drift were present in our samples (see also “Influence of Organic Matter Availability” section). Jakob et al. (2003), Bruno et al. (2010), and references therein reported Tricoptera as a high flow sensitive species as well. Taxa that have lower tendency to drift and that are proportionately lower in numbers in the drift samples in our study such as substrate burrowing organisms (i.e. Oligochaeta in our case), seemed to be relatively unaffected by the peak. This observation was also reported by Kjaerstad et al. (2018). Diamesinae were uncommon in our drift samples. The flow may not have been elevated enough to dislodge them from the course substrate (see Bruno et al., 2010).
The data showed a consistent decrease of the abundance of some taxa in the drift sample with decreasing recovery time. In the riffle, this was the case for Perlodidae and Chloroperlidae from experiment 2 to experiment 5 and for Chironomidae, Heptageniidae, and Baetidae from experiment 3 to experiment 5. In the pool, the abundances of Chironomidae, Perlodidae, Empididae, Heptageniidae, consistently decreased with less recovery time (experiment 2 to experiment 5) and Baetidae and Rhyacophilidae decreased consistently from experiment 3 to experiment 5. Similarly, the results of the study completed by Bruno et al. (2016) showed a depleting effect of five flume hydropeaking repetitions (over five consecutive days) on Chironomidae drift rate. Unlike our results, Baetidae were observed to sustain a similar drift rate during hydropeaking throughout their flume experiments (Bruno et al., 2016).
Habitat Comparison: Downstream Riffle Versus Pool
Most research on hydropeaking impacts is conducted in flumes or in experimental channels with simulated riffles and/or runs, or it is performed on a regulated river with riffles and runs (for example, Poff and Ward, 1991; Mochizuki et al., 2006). Our study aimed to fill a gap in understanding of riffle and pool habitat responses to hydropeaking in a natural river setting. In our study, the pool had a much larger total peak drifting density (123 ind. m−3) than the riffle (55 ind. m−3, Table 3; Figure 3), although the kick samples showed higher abundance in the riffle compared to the pool (Figure 6). We are presuming that greater numbers of high flow tolerant species were likely residing in the riffle and this presumption is supported by Kjaerstad et al. (2018) who also found that total densities significantly differed between shallow and deep areas for upstream and downstream locations. As well, we found more organic matter in the pool than in the riffle (pers. obs.) which can lead to a consequential impact on organic matter associated taxa.
Another possible explanation might be that the change in the physical properties of the benthos during the hydropeaking was larger in the pool than in the riffle. The grain size distribution analysis confirmed that the substrate composition of the riffle and pool habitats differed: as expected, the pool habitat had a smaller median grain size distribution diameter (40–50 mm) than the riffle habitat (60–80 mm). From a visual qualitative analysis, the investigated habitats did not exhibit changes during the experiment, and this was reflected by the persistent observed grain size distribution. One measurable change related to the pool grain size distribution after the first experimental peak might be accounted for by surmising that a fine gravel/sandy layer covering the river bottom in the pool habitat was loosened and transported downstream during experimental peak 1.
Bruno et al. (2010) found that while sediment mobilization plays a role in initiating macroinvertebrate drift, sediment agitation (non-scouring) is also a significant drift initiator (see also Poff and Ward, 1991; Imbert and Perry, 2000; Miller and Judson, 2014). In fact, Bruno et al. (2010) measured considerable drift during a non-scouring peak: a peak which had a 600% increase in streamflow (discharge) from 1 to 7 m3 s−1. Our experiments showed comparable relative increases in turbined discharge from pre-peak to peak flow (from ∼0.35 to 2.6 m3 s−1) and, as there was little to no change in grain size distribution and no notable geomorphological changes during our experiments, it is safe to classify these peaks as non-scouring, supporting our assumption that the drift during our experimental peaks was caused by agitation rather than sediment mobilization.
Limitations From Experimental Design and Environmental Conditions
Net Positions
The variation in net position did not show any significant differences in drift densities or composition (Tables 5 and 6). The similarity across net transects of our habitats was likely related to river shape and channel morphology. Fuller et al. (2011) assessed flood-type disturbances on four rivers for both periphyton and macroinvertebrate communities and found that high and low shear stress areas feature different types of macroinvertebrates (i.e., filter-feeders, gatherers, scrapers, collector–gatherers etc.). We hypothesize that because the river channels in our study were so consistently narrow, all our samples were taken from similar sheer stress areas. However, since shear stress was not measured in our experiment, we would require field tests and empirical data to assess that suggestion.
Influence of Short Duration Hydropeaking
Bruno et al. (2016) hypothesized that most macroinvertebrate drift loss happens in the first 5–10 min of a peak. Therefore, maintaining a high discharge over long stretches of time would be unlikely to increase the catastrophic drift effect on the macroinvertebrate community. However, an elevated discharge could delay the deposition of new organic matter and the recolonizing of animals after the disturbance. Since we did not record a decline in benthos from upstream to downstream communities, it is possible that the magnitude or duration of the peaks were not high enough to remove the majority of benthos from the study sites, and/or that recovery times were sufficient to allow for the rapid recolonization of drifting upstream macroinvertebrates downstream.
Influence of Temperature and Water Quality
In their review, Poff and Zimmerman (2010) outlined the importance of incorporating environmental factors – for example, water temperature – when assessing ecological responses to altered flow regimes. A study completed by Schülting et al. (2016) assessing the effects of combined hydropeaking and cold thermopeaking on macroinvertebrate drift in experimental flumes, found that water temperature can have a mitigating impact on drift during thermopeaking (see also Carolli et al., 2012; Bruno et al., 2013). In light of this, it is worth noting that we did not find any relationship between macroinvertebrate drift or benthos and water temperature. Our findings are more in line with those of Miller and Judson (2014) who found that temperature can be a poor predictor of seasonal drift, although temperature variations during our experiment were comparably small.
Other physical parameters relevant to macroinvertebrate drift assemblages (changes in grain size distribution, dissolved oxygen, pH, or specific conductivity, Poff and Ward, 1991) were also measured. Variations in these parameters were small and did not significantly impact drift patterns during our experiments. For example, our calculations indicated that dissolved oxygen remained saturated at all times, corresponding to the findings of Poff and Ward (1991). Specific conductivity and pH remained in baseflow ranges during all measurements. However, our model explained only a part of the samples variability (PERMANOVA R2 = 0.527 for riffle and R2 = 0.5463 for pool).
Influence of Organic Matter Availability
Along with the considerable macroinvertebrate drift densities during peaks, we also had large amounts of drifting organic matter. As mentioned previously, the coupling of these two hydropeaking consequences has been shown in other studies. For example, Mochizuki et al. (2006) found that hydropeaking in experimental channels increases organic matter export along with macroinvertebrate drift (see also Irvine and Henriques, 1984; Irvine, 1985; Bruno et al., 2016). In the same study, Mochizuki et al. (2006) found that within 10 days of baseflow, particulate matter deposits and algal production and/or organic matter accumulated in the experimental channel. However, due to the complex nature of our study site and allowing that our experiment took place in late fall when organic matter is plentiful, we did not see a depletion of organic matter (pers. obs.) over the course of our samples. This abundance of organic matter may account for the negligible differences in the drifting macroinvertebrate recovery times; with enough readily available organic matter from the riparian and surrounding landscape, as well as from upstream to downstream drifting, those macroinvertebrates lost when the organic matter was flushed during the experimental peaks were readily recolonized. Additional experiments at the peak of winter months or in late winter would better determine the significance of this environmental influence on drift recovery.
Upstream Influences
As an outcome of the recent construction of this hydropower plant, the natural morphology of the reach between intake and outlet (our upstream site) has a newly impaired hydrology. As of late summer 2018, the upstream study site was continuing to adapt. Our results show that the upstream community and downstream macroinvertebrate community assemblages were the same during the experiment, indicating that upstream has the potential to recolonize the downstream via drift. Bruno et al. (2016) determined that 18 h was a potentially sufficient time to replace the individuals removed during hydropeaking events in an experimental flume. However, in their experiment, 18 h was in reality not enough time for incoming drift to reach full colonization potential. This shortfall was likely due to the reduced availability of coarse and fine particulate organic matter in the flume (Bruno et al., 2016). As indicated previously, there was considerable organic matter in all our drift samples further highlighting discrepancies when comparing flume-generated results to natural environments. Robinson et al. (2004b) completed a study on three experimental floods of varying discharge (10, 25 and 10 m3 s−1) lasting 7–8 h in an alpine river and demonstrated that benthic communities reach or even exceed pre-flood densities after 20–35 days.
In our natural alpine river, with a near-natural upstream reach that includes a floodplain of national importance and amply available organic matter, we recorded relatively short recovery times. Our measurements indicate times for sufficient recovery that fall between the estimates of Bruno et al. (2016) and Robinson et al. (2004b). Depending on the magnitude of flow perturbations, Smith and Brown (2006) suggested that drifting organisms can effectively recolonize benthic habitats in natural flow conditions. Additionally, a vertically connected hyporheic zone can provide refugia and help recolonize depleted benthic habitats (Townsend and Hildrew, 1994; Bruno et al., 2010). More long-term research is warranted into recolonization from upstream habitats on the recovery time of the downstream receiving environments, as the availability of organic matter changes throughout the year.
Conclusions
To our knowledge, this is the first real world study of a novel SHP with the objective to measure and compare the response and recovery of drifting and benthic invertebrates to short hydropeaking events. It is also the first to assess the recovery of the drifting benthic macroinvertebrates in two separate habitats during late fall/early winter months. Our investigation tested how the kick and drift macroinvertebrate communities change in abundance, density, and composition between experimental peaks and between habitats. We found that the drift community and the kick community had similar assemblages, and we did not detect a statistically significant change in the benthos or in the drift community in either the upstream or downstream riffle or pool between experimental peaks. An ecologically intact upstream reach, as well as an abundance of organic matter, resulted in no significant changes in the drift community for recovery times as low as 24 h. Recovery depends on multiple factors including spatial and temporal scales, as well as the natural integrity of the biological community (Doretto et al., 2018), in particular its upstream ecological condition (Bruno et al., 2016). It is worth noting that considerable differences between riffle and pool habitats highlight the complexity of impacts on natural streams, and the hazards of direct application of results from channel or flume studies. Furthermore, our results cannot exclude potential long-term effects of flexible small-scale hydropower production at this site. These would have to be assessed with a long-term monitoring in case flexible production will be effectively implemented.
The research conducted at the novel Gletsch–Oberwald SHP may benefit water policy decision-makers by offering dependable data on the ecological impact of hydropeaking operations. It may also encourage hydropower operators to investigate practices that can effectively balance minimizing ecological damage with meeting societal energy demands. This research calls for further in-situ field studies as the variables influencing river ecosystem integrity, the impact on and recovery times of local benthic communities, are quite complex.
Data Availability Statement
The datasets used for this study are publicly available on the ERIC–open repository of Eawag at the following URL: https://doi.org/10.25678/0002e4.
Author Contributions
CA, CW, and MS contributed to the conceptualization of the experiment. CA designed and implemented the field study and laboratory analysis. MC, DV, and CA contributed to the data analysis and visualization. All authors contributed to the interpretation of the results and writing the manuscript.
Funding
This research is part of the SmallFlex project, which is financially supported by the Swiss Federal Office of Energy (SFOE, Grant SI/501636-01) and Forces Motrices Valaisannes (FMV).
Conflict of Interest
The authors declare that this study received funding from Forces Motrices Valaisannes (FMV). The funder had the following involvement in the study: They defined the technical boundary conditions for producing the hydropeaks (amount of water stored, peak discharge), they were involved in defining the time span allocated for the experiment, and they produced the hydropeaks for the study. Besides that, they were not involved in the study design, collection, analysis, interpretation of data, the writing of this article or the decision to submit it for publication.
Acknowledgments
All authors would like to thank Nathalie Friese for help in the field campaign and laboratory analysis. Nikolas Aksamit provided insightful comments, suggestions, and discussions. We thank Benjamin Misteli and Alexander Freude-von Känel for laboratory assistance. Cécile Münch-Alligné and Steve Crettenand provided helpful and necessary logistical support. We would also like to thank Kate Mathers for her technical suggestions and field support, and Christopher Robinson for his technical suggestions and for lending us field equipment. Thanks also to Nico Bätz, Gabriele Consoli, Simone Jola, Esther Leitgeb, and Lucie Sprecher for their field support during the field campaign.
Supplementary Material
The Supplementary Material for this article can be found online at: https://www.frontiersin.org/articles/10.3389/fenvs.2020.602374/full#supplementary-material.
References
Anderson, D., Moggridge, H., Warren, P., and Shucksmith, J. (2015). The impacts of ‘run-of-river’ hydropower on the physical and ecological condition of rivers. Water Environ. J. 29, 268–276. doi:10.1111/wej.12101
Anderson, M., Gorley, R. N., and Clarke, K. R. (2008). PERMANOVA+ for PRIMER: Guide to Software and Statistical Methods. Plymouth, UK: PRIMER-E Ltd., 218.
Anselmetti, F. S., Bühler, R., Finger, D., Girardclos, S., Lancini, A., Rellstab, C., et al. (2007). Effects of Alpine hydropower dams on particle transport and lacustrine sedimentation. Aquat. Sci. 69, 179–198. doi:10.1007/s00027-007-0875-4
Auer, S., Zeiringer, B., Führer, S., Tonolla, D., and Schmutz, S. (2017). Effects of river bank heterogeneity and time of day on drift and stranding of juvenile European grayling (Thymallus thymallus L.) caused by hydropeaking. Sci. Total Environ. 575, 1515–1521. doi:10.1016/j.scitotenv.2016.10.029 |
Bejarano, M. D., Jansson, R., and Nilsson, C. (2018). The effects of hydropeaking on riverine plants: a review. Biol. Rev. Camb. Phil. Soc. 93, 658–673. doi:10.1111/brv.12362
Bejarano, M. D., Sordo-Ward, Á., Alonso, C., Jansson, R., and Nilsson, C. (2020). Hydropeaking affects germination and establishment of riverbank vegetation. Ecol. Appl. 30, 1–16. doi:10.1002/eap.2076
Bejarano, M. D., Sordo-Ward, A., Gabriel-Martin, I., and Garrote, L. (2019). Tradeoff between economic and environmental costs and benefits of hydropower production at run-of-river-diversion schemes under different environmental flows scenarios. J. Hydrol. 572, 790–804. doi:10.1016/j.jhydrol.2019.03.048
Bell, E., Kramer, S., Zajanc, D., and Aspittle, J. (2008). Salmonid fry stranding mortality associated with daily water level fluctuations in Trail Bridge Reservoir, Oregon. N. Am. J. Fish. Manag. 28, 1515–1528. doi:10.1577/m07-026.1
Bruno, M. C., Cashman, M. J., Maiolini, B., Biffi, S., and Zolezzi, G. (2016). Responses of benthic invertebrates to repeated hydropeaking in semi-natural flume simulations. Ecohydrology 9, 68–82. doi:10.1002/eco.1611
Bruno, M. C., Maiolini, B., and Carolli, M. (2010). Short time-scale impacts of hydropeaking on benthic invertebrates in an Alpine stream (Trentino, Italy). Limnologica 40, 281–290. doi:10.1016/j.limno.2009.11.012
Bruno, M. C., Siviglia, A., Carolli, M., and Maiolini, B. (2013). Multiple drift responses of benthic invertebrates to interacting hydropeaking and thermopeaking waves. Ecohydrology 6, 511–522. doi:10.1002/eco.1275
Carolli, M., Bruno, M. C., Siviglia, A., and Maoilini, B. (2012). Responses of benthic invertebrates to abrupt changes of temperature in flume simulations. River Res. Applic. 28, 678–691. doi:10.1002/rra.1520
Carolli, M., Vanzo, D., Siviglia, A., Zolezzi, G., Bruno, M. C., and Alfredsen, K. (2015). A simple procedure for the assessment of hydropeaking flow alterations applied to several European streams. Aquat. Sci. 77, 639–653. doi:10.1007/s00027-015-0408-5
Clarke, K. R., and Gorley, R. N. (2018). Getting started with PRIMER v7. Plymouth, UK: PRIMER-E Ltd., 20.
Clarke, K. R., and Gorley, R. N. (2015). User Manual/Tutorial: Primer v7. Plymouth, UK: PRIMER-E Ltd., 300
De Jalon, D. G., Sanchez, P., and Camargo, J. A. (1994). Downstream effects of a new hydropower impoundment on macrophyte, macroinvertebrate and fish communities. Regul. Rivers Res. Manag. 9, 253–261. doi:10.1002/rrr.3450090406
Dobson, M., Pawley, S., Fletcher, M., and Powell, A. (2012). Guide to freshwater invertebrates. Ambleside, UK: Freshwater Biological Association scientific publication No. 68.
Doretto, A., Piano, E., Falasco, E., Fenoglio, S., Bruno, M. C., and Bona, F. (2018). Investigating the role of refuges and drift on the resilience of macroinvertebrate communities to drying conditions: an experiment in artificial streams. River Res. Appl. 34, 777–785. doi:10.1002/rra.3294
Ellis, L. E., and Jones, N. E. (2013). Longitudinal trends in regulated rivers: a review and synthesis within the context of the serial discontinuity concept. Environ. Rev. 21, 136–148. doi:10.1139/er-2012-0064
Feeley, H. B., Davis, S., Bruen, M., Blacklocke, S., and Kelly-Quinn, M. (2012). The impact of a catastrophic storm event on benthic macroinvertebrate communities in upland headwater streams and potential implications for ecological diversity and assessment of ecological status. J. Limnol. 71, 32. doi:10.4081/jlimnol.2012.e32
Fuller, R. L., Doyle, S., Levy, L., Owens, J., Shope, E., Vo, L., et al. (2011). Impact of regulated releases on periphyton and macroinvertebrate communities: the dynamic relationship between hydrology and geomorphology in frequently flooded rivers. River Res. Appl. 27, 630–645. doi:10.1002/rra.1385
García, A., Jorde, K., Habit, E., Caamaño, D., and Parra, O. (2011). Downstream environmental effects of dam operations: changes in habitat quality for native fish species. River Res. Appl. 27, 312–327. doi:10.1002/rra.1358
GeoVITe. (2019). User-friendly geodata service. Oderzo, Italy: GeoVITeAvailable at: https://geovite.ethz.ch. (Accessed July 21, 2020).
Gibbins, C., Batalla, R. J., and Vericat, D. (2010). Invertebrate drift and benthic exhaustion during disturbance: response of mayflies (Ephemeroptera) to increasing shear stress and river-bed instability. River Res. Appl. 26, 499–511. doi:10.1002/rra.1282
Herbst, D. B., Cooper, S. D., Medhurst, R. B., Wiseman, S. W., and Hunsaker, C. T. (2018). A comparison of the taxonomic and trait structure of macroinvertebrate communities between the riffles and pools of montane headwater streams. Hydrobiologia 820, 115–133. doi:10.1007/s10750-018-3646-4
Hershey, A. E., Pastor, J., Peterson, B. J., and Kling, G. W. (1993). Stable isotopes resolve the drift paradox for baetis mayflies in an Arctic river. Ecology 74, 2315–2325. doi:10.2307/1939584
Imbert, J. B., and Perry, J. A. (2000). Drift and benthic invertebrate responses to stepwise and abrupt increases in non-scouring flow. Hydrobiologia 436, 191–208. doi:10.1023/A:1026582218786
Irvine, J. R. (1985). Effects of successive flow perturbations on stream invertebrates. Can. J. Fish. Aquat. Sci. 42, 1922–1927. doi:10.1139/f85-238
Irvine, J. R., and Henriques, P. R. (1984). A preliminary investigation on effects of fluctuating flows on invertebrates of the Hawea River, a large regulated river in New Zealand. N. Z. J. Mar. Freshw. Res. 18, 283–290. doi:10.1080/00288330.1984.9516050
Jager, H. I., Efroymson, R. A., Opperman, J. J., and Kelly, M. R. (2015). Spatial design principles for sustainable hydropower development in river basins. Renew. Sustain. Energy Rev. 45, 808–816. doi:10.1016/j.rser.2015.01.067
Jakob, C., Robinson, C. T., and Uehlinger, U. (2003). Longitudinal effects of experimental floods on stream benthos downstream from a large dam. Aquat. Sci. 65, 223–231. doi:10.1007/s00027-003-0662-9
Jones, N. E., and Petreman, I. C. (2015). Environmental influences on fish migration in a hydropeaking river. River Res. Appl. 31, 1109–1118. doi:10.1002/rra.2810
Kibler, K. M., and Tullos, D. D. (2013). Cumulative biophysical impact of small and large hydropower development in Nu River, China. Water Resour. Res. 49, 3104–3118. doi:10.1002/wrcr.20243
Kjaerstad, G., Arnekleiv, J. V., Speed, J. D. M., and Herland, A. K. (2018). Effects of hydropeaking on benthic invertebrate community composition in two central Norwegian rivers. River Res. Appl. 34, 218–231. doi:10.1002/rra.3241
Kuznetsova, A., Brockhoff, P. B., and Christensen, R. H. B. (2017). lmerTest package: tests in linear mixed effects models. J. Stat. Softw. 82 (13), 1–26. doi:10.18637/jss.v082.i13
Lagarrigue, T., Céréghino, R., Lim, P., Reyes-Marchant, P., Chappaz, R., Lavandier, P., et al. (2002). Diel and seasonal variations in brown trout (Salmo trutta) feeding patterns and relationship with invertebrate drift under natural and hydropeaking conditions in a mountain stream. Aquat. Living Resour. 15, 129–137. doi:10.1016/S0990-7440(02)01152-X
Lange, K., Meier, P., Trautwein, C., Schmid, M., Robinson, C. T., Weber, C., et al. (2018). Basin-scale effects of small hydropower on biodiversity dynamics. Front. Ecol. Environ. 16, 397–404. doi:10.1002/fee.1823
Lauters, F., Lavandier, P., Lim, P., Sabaton, C., and Belaud, A. (1996). Influence of hydropeaking on invertebrates and their relationship with fish feeding habits in a Pyrenean River. Regul. Rivers Res. Manag. 12, 563–573. doi:10.1002/(SICI)1099-1646(199611)12:6<563::AID-RRR380>3.0.CO;2-M
Lubini, V., Knispel, S., and Vinçon, G. (2012). Die Steinfliegen der Schweiz. Bestim- mung und Verbreitung/Les plécoptères de la Suisse: identification et distribution. Fauna Helvetica 27. Neuchâtel: CSCF & SEG.
Mauch, E. (2017). Aquatische Diptera-Larven in Mittel-, Nordwest- und Nordeuropa. Übersicht über die Formen und ihre Identifikation. Lauterbornia. 83, Dinkelscherben, Germany: Erik Mauch Verlag
Mihalicz, J. E., Jardine, T. D., Baulch, H. M., and Phillips, I. D. (2019). Seasonal effects of a hydropeaking dam on a downstream benthic macroinvertebrate community. River Res. Appl., 35, 714–724. doi:10.1002/rra.3434
Miller, S. W., and Judson, S. (2014). Responses of macroinvertebrate drift, benthic assemblages, and trout foraging to hydropeaking. Can. J. Fish. Aquat. Sci. 71, 675–687. doi:10.1139/cjfas-2013-0562
Mochizuki, S., Kayaba, Y., and Tanida, K. (2006). Drift patterns of particulate matter and organisms during artificial high flows in a large experimental channel. Limnology 7, 93–102. doi:10.1007/s10201-006-0166-0
Oksanen, J., Guillaume Blanchet, F., Friendly, M., Kindt, R., Legendre, P., McGlinn, D., et al. (2019). vegan: Community Ecology Package. R package version 2.5-6 https://CRAN.R-project.org/package=vegan
Petticrew, E. L., Krein, A., and Walling, D. E. (2007). Evaluating fine sediment mobilization and storage in a gravel-bed river using controlled reservoir releases. Hydrol. Process. 21, 198–210. doi:10.1002/hyp.6183
Poff, N. L., Olden, J. D., Merritt, D. M., and Pepin, D. M. (2007). Homogenization of regional river dynamics by dams and global biodiversity implications. Proc. Natl. Acad. Sci. U.S.A. 104, 5732–5737. doi:10.1073/pnas.0609812104 |
Poff, N. L., and Ward, V. (1991). Drift responses of benthic invertebrates to experimental streamflow variation in a hydrologically stable stream. Can. J. Fish. Aquat. Sci. 48, 1926–1936. doi:10.1139/f91-229
Poff, N. L., and Zimmerman, J. K. H. (2010). Ecological responses to altered flow regimes: a literature review to inform the science and management of environmental flows. Freshw. Biol. 55, 194–205. doi:10.1111/j.1365-2427.2009.02272.x
R Core Team. (2020). R: a language and environment for statistical computing. Vienna, Austia: R foundation for statistical computingAvailable at: https://www.R-project.org.
Robinson, C. T., Tockner, K., and Burgherr, P. (2004a). Drift benthos relationships in the seasonal colonization dynamics of alpine streams. Arch. Hydrobiol. 160, 447–470. doi:10.1127/0003-9136/2004/0160-0447
Robinson, C. T., Uehlinger, U., and Monaghan, M. T. (2003). Effects of a multi-year experimental flood regime on macroinvertebrates downstream of a reservoir. Aquat. Sci. 65, 210–222. doi:10.1007/s00027-003-0663-8
Robinson, C. T., Uehlinger, U., and Monaghan, M. T. (2004b). Stream ecosystem response to multiple experimental floods from a reservoir. River Res. Appl. 20, 359–377. doi:10.1002/rra.743
Schülting, L., Feld, C. K., and Graf, W. (2016). Effects of hydro- and thermopeaking on benthic macroinvertebrate drift. Sci. Total Environ. 573, 1472–1480. doi:10.1016/j.scitotenv.2016.08.022 |
Schülting, L., Feld, C. K., Zeiringer, B., Huđek, H., and Graf, W. (2019). Macroinvertebrate drift response to hydropeaking: an experimental approach to assess the effect of varying ramping velocities. Ecohydrology 12, e2032. doi:10.1002/eco.2032
Scullion, J., and Sinton, A. (1983). Effects of artificial freshets on substratum composition, benthic invertebrate fauna and invertebrate drift in two impounded rivers in mid-Wales. Hydrobiologia 107, 261–269. doi:10.1007/BF00036696
Smith, F., and Brown, A. V. (2006). Effects of flow on meiofauna colonization in artificial streams and reference sites within the Illinois River, Arkansas. Hydrobiologia 571, 169–180. doi:10.1007/s10750-006-0237-6
Studemann, D., Landolt, P., Sartori, M., Hefti, D., and Tomka, I. (1992). Ephemeroptera. Insecta Helvetica, Fauna, Geneva, Switzerland: SEG
Tachet, H., Richoux, P., Bournaud, M., and Usseglio-Polatera, P. (2000). Invertébrés d'eau douce, systématique, biologie, écologie. Paris, France: CNRS éditions
Tonolla, D., Bruder, A., and Schweizer, S. (2017). Evaluation of mitigation measures to reduce hydropeaking impacts on river ecosystems - a case study from the Swiss Alps. Sci. Total Environ. 574, 594–604. doi:10.1016/j.scitotenv.2016.09.101 |
Townsend, C. R., and Hildrew, A. G. (1994). Species traits in relation to a habitat templet for river systems. Freshw. Biol. 31, 265–275. doi:10.1111/j.1365-2427.1994.tb01740.x
Tuhtan, J. A., Noack, M., and Wieprecht, S. (2012). Estimating stranding risk due to hydropeaking for juvenile European grayling considering river morphology. KSCE J. Civ. Eng. 16, 197–206. doi:10.1007/s12205-012-0002-5
Vanzo, D., Siviglia, A., Carolli, M., and Zolezzi, G. (2016). Characterization of sub-daily thermal regime in alpine rivers: quantification of alterations induced by hydropeaking. Hydrol. Process. 30, 1052–1070. doi:10.1002/hyp.10682
Walker, B., Holling, C. S., Carpenter, S. R., and Kinzig, A. (2004). Resilience, adaptability and transformability in social-ecological systems. Ecol. Soc. 9 (2), 5. doi:10.5751/ES-00650-090205
Waringer, J., and Graf, W. (2011). Atlas der Mitteleuropäischen Köcherfliegenlarven/Atlas of central European Trichoptera larvae. Dinkelscherben, Germany: Erik Mauch Verlag.
Wolman, M. G. (1954). A method of sampling coarse river-bed material. Trans. Am. Geophys. Union 35 (6), 951–956. doi:10.1029/TR035i006p00951
Zarfl, C., Lumsdon, A. E., Berlekamp, J., Tydecks, L., and Tockner, K. (2015). A global boom in hydropower dam construction. Aquat. Sci. 77, 161–170. doi:10.1007/s00027-014-0377-0
Ziv, G., Baran, E., Nam, S., Rodríguez-Iturbe, I., and Levin, S. A. (2012). Trading-off fish biodiversity, food security, and hydropower in the Mekong River Basin. Proc. Natl. Acad. Sci. U.S.A. 109, 5609–5614. doi:10.1073/pnas.1201423109 |
Keywords: field experiment, macroinvertebrate drift, flexible power production, physical habitat, pool, riffle
Citation: Aksamit CK, Carolli M, Vanzo D, Weber C and Schmid M (2021) Macroinvertebrate Recovery to Varying Hydropeaking Frequency: A Small Hydropower Plant Experiment. Front. Environ. Sci. 8:602374. doi: 10.3389/fenvs.2020.602374
Received: 03 September 2020; Accepted: 24 December 2020;
Published: 29 January 2021.
Edited by:
Tor Haakon Bakken, Norwegian University of Science and Technology, NorwayReviewed by:
Raphael Ligeiro, Federal University of Pará, BrazilAgustina Cortelezzi, Consejo Nacional de Investigaciones Científicas y Técnicas (CONICET), Argentina
Copyright © 2021 Aksamit, Carolli, Vanzo, Weber and Schmid. This is an open-access article distributed under the terms of the Creative Commons Attribution License (CC BY). The use, distribution or reproduction in other forums is permitted, provided the original author(s) and the copyright owner(s) are credited and that the original publication in this journal is cited, in accordance with accepted academic practice. No use, distribution or reproduction is permitted which does not comply with these terms.
*Correspondence: Martin Schmid, bWFydGluLnNjaG1pZEBlYXdhZy5jaA==