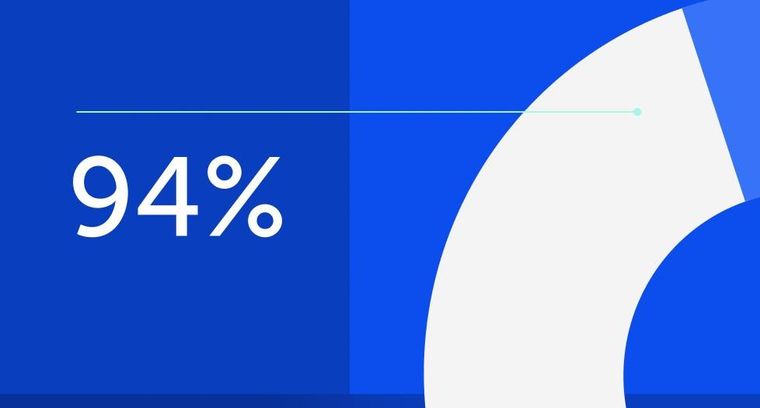
94% of researchers rate our articles as excellent or good
Learn more about the work of our research integrity team to safeguard the quality of each article we publish.
Find out more
ORIGINAL RESEARCH article
Front. Environ. Sci., 08 October 2020
Sec. Conservation and Restoration Ecology
Volume 8 - 2020 | https://doi.org/10.3389/fenvs.2020.581476
This article is part of the Research TopicCarbon Sink Accounting, Certification and TradeView all 4 articles
Montane ecosystems occur throughout the world, and harbor many endemic species. They also provide key ecological services, including the catchment of water resources and the storage of organic carbon. These ecosystems are vulnerable to global climate change and increasing human pressures, including forestry and their conversion to arable land. In the extensive and biodiverse Mexican montane regions, ongoing deforestation and conversion to arable lands has led to diminished ecosystem health and services. Here, we undertook a comprehensive evaluation of carbon stocks within Mexican montane habitats in the Flora and Fauna Conservation Area of Nevado de Toluca. This aimed to integrate these habitats into Mexican and global census of forest carbon, the first step needed to convert on carbon credit markets to incentivize conservation of this region by local communities. Our study evaluated both, living biomass and belowground soil organic carbon in sites within forests, alpine grasslands and converted arable land. We addressed the following questions: (1) What are the organic carbon stocks, including the soil component, of our studied montane habitats? (2) What are the avoided CO2 emissions from maintaining natural forests and preventing conversion to arable land? And (3) Within our study area, are organic carbon stocks in the soil correlated to carbon stocks in aboveground living biomass? We found whole ecosystem organic carbon stocks ranged from 68 Mg OC ha–1 in unburnt alpine grasslands to 668 Mg OC ha–1 in Abies religiosa forests. By avoiding conversion of the A. religiosa forests to arable lands, we show that emissions of 1,122 to 1,671 Mg CO2 ha–1 are avoided. Notably, the belowground soil organic carbon stock comprised ≥ 40% of the total ecosystem organic carbon stock. We recommend soil organic carbon stocks should be included within Mexican and global forestry carbon stock inventories, and should be considered within voluntary carbon-credit markets used to incentivize the conservation of Mexican montane habitats.
The highland slopes of mountains are covered by montane ecosystems, including forests and alpine grasslands. Montane habitats cover more than 20% of the Earth’s surface, and can form isolated ecosystems that harbor a density of endemic species. In addition to biodiversity, these regions provide important ecological services. This notably includes the catchment, storage and provision of water, as montane habitats can store snow and ice that is released as water run-off within basins and rivers during warm periods (Keith et al., 2009; Ariza et al., 2013; Schermer et al., 2016).
Montane forests typically reside in bands below the alpine zone and are characterized by temperate to cold climates, due to the high elevations that mountains reach. Due to this restricted distribution, montane habitats are vulnerable to rising temperatures from global climate change which can reduce their resilience and functions (e.g., Nogués-Bravo et al., 2007; Koörner and Ohsawa, 2005). Montane ecosystems themselves store organic carbon (OC) within soils and vegetation that also provides soil stability (Ward et al., 2014).
Mexican montane ecosystems are globally recognized as priority ecosystems for conservation due to their high rate of endemism and species diversity (Olson and Dinerstein, 2002; Valencia, 2004). These montane ecosystems are characterized by broadleaf, conifer and cloud forests that occur from ∼1500 m above sea level (masl) and by alpine grasslands which occur > 3700 masl (Rzedowski, 1975; Challenger and Soberón, 2008; Mastretta-Yanes et al., 2015). Both, rural communities and large urban cities occupy surrounding lowland areas, and depend on the services provided by montane ecosystems, such as freshwater supply and soil fertility (e.g., Works and Hadley, 2004; Galicia and García-Romero, 2007; Santini et al., 2019a).
In Mexico, most montane ecosystems (over 50%) belong to the local communities (or ejidos) that have the right to manage the natural resources in these areas (Ley Agraria, 1992; Madrid et al., 2009). Protected areas in Mexico are also monitored by governmental bodies, including the national commission of natural protected areas and the national forestry commission (CONANP and CONAFOR, respectively by their Spanish acronym) that work together with local communities to implement conservation and sustainable use of protected areas.
Despite their value, montane ecosystems are under increasing human pressures. In Mexico, the loss of montane forests was 43,072 ha year–1 during the 1990s. Between 2002 and 2007, montane forests lost 38,712 ha year–1, and more recently (2007–2011) montane forests are being deforested at 11,670 ha year–1 (Comisión Nacional Forestal, 2018). However, in addition to deforestation, undocumented logging is ongoing and the conversion of montane forests to arable land is expected to increase alongside a rapidly growing population (Mendoza-Ponce et al., 2019; Santini et al., 2019a).
Ongoing deforestation causes the loss of ecosystem services, biodiversity, and contributes up to 17% of the global carbon dioxide (CO2) emissions (van der Werf et al., 2009; Agrawal et al., 2011; Global Forest Resources Assessment, 2020). Therefore, conservation is essential to maintain montane ecosystem health, ensure water and forestry resources, and contribute to achieving Mexico’s targets to reduce the country’s CO2 emissions by 25% by 2030 (IPBES, 2018; Santini et al., 2019a).
Organic carbon is stored within the living vegetation, litter and soils (Pearson et al., 2007; Global Forest Resources Assessment, 2020). Current Mexican land inventories only consider OC stored within the living aboveground biomass, and do not consider the presence of the soil OC due to a scarcity of data (Comisión Nacional Forestal, 2018). However, soil OC may represent a significant fraction of the total OC stocks of Mexican montane habitats and, if disturbed, can substantially contribute to CO2 emissions into the atmosphere (e.g., Mendoza-Ponce et al., 2019; Santini et al., 2019a).
Soil OC derives from vegetation inputs, such as the litterfall, woody debris and rhizodeposition, and therefore we may expect a relationship between high live biomass and high soil OC inputs (e.g., Chen et al., 2013; Berhongaray et al., 2019). For example, a study in a chronosequence of the conifer Cunninghamia lanceolata observed that natural old forests with high levels of living biomass store higher soil OC than plantations with lower biomass levels (Chen et al., 2013). However, soil OC stocks are also influenced by additional factors such as decomposition rates that vary with soil temperatures, the molecular characteristics of the OC, the disturbance regime, pH and water content, which influences the oxygen availability in the soil. Due to this range of variables, high living biomass does not always correlate with high soil OC stocks across ecosystems (e.g., De Deyn et al., 2008; Parker et al., 2015; Crowther et al., 2016).
Here, we provide a comprehensive quantification of OC stocks in Mexican montane ecosystems, including alpine grasslands, montane conifer forests and an arable land, within the Flora and Fauna Conservation Area Nevado de Toluca, Mexico. Nevado de Toluca is a volcano which was established as a National Park in 1935 to conserve over 51,000 ha of endemic and diverse vegetation (Figures 1A,B). However, the Nevado de Toluca was reclassified as a Flora and Fauna Conservation Area in 2013. This is still a protected area, but more permissive to human settlements, and activities such as grazing, agriculture and forest logging. Some of the original habitats at the Nevado de Toluca have been subject to major human impacts. Between 1935 and 1965, 16% of natural conifer forests (∼8,300 ha) were cleared for conversion to arable land, pastures and human settlements (Toscana-Aparicio and Granados-Ramírez, 2015). Forest clearing has decreased more recently to 1.3% year–1, between 2000 and 2009 (Regil García et al., 2014). However, forest degradation due to logging and extraction of timber, fuels and pulp continues at unmonitored rates (Mastretta-Yanes et al., 2014).
Figure 1. (A) Habitats of study at the Flora and Fauna Conservation Area Nevado de Toluca, State of Mexico, Mexico. Our habitats were an unburnt and a burnt grassland at > 4000 masl, a natural P. hartwegii forest at 3960 masl, a P. montezumae plantation at 3284 masl, two managed A. religiosa and P. pseudostrobus forests at ∼ 2990 masl, three A. religiosa conservation forests at ∼3250 masl and an arable land at 3268 masl. (B) Conifer and broadleaf forests in Mexico occupy 16.5% of the territory. Alpine grasslands are highly vulnerable ecosystems and only cover 0.008% of the Mexican territory (Challenger and Soberón, 2008).
Whilst the benefits of montane habitats are appreciated, the carbon stocks within these regions have not been fully quantified. Here, we assessed the carbon stocks and estimated avoided emissions from forest conservation (non-conversion to arable lands). This aimed to integrate these habitats into global and national census of forest carbon, the first step needed to convert on carbon credit markets to incentivize conservation of this region by local communities. To achieve this aim, we addressed the following questions: (1) What are the organic carbon stocks, including the soil component, of our studied montane habitats? (2) What are the avoided CO2 emissions from maintaining natural forests and avoiding conversion to arable land? And (3) Are soil organic carbon stocks in our study area linked to organic carbon stocks in the living biomass? This study of carbon stocks can be employed by local communities (ejidos), Mexican government agencies (including CONANP and CONAFOR) and non-profit organizations to estimate carbon inventories, that can be further used to inform conservation and management strategies of montane ecosystems.
The Nevado de Toluca is classified as a Flora and Fauna Conservation Area, located in the State of Mexico (between 18°52′ and 19°23′ N and 99°33′ and 99°52′ W). The Flora and Fauna Conservation Area Nevado de Toluca is a volcano that covers 53,591 ha across an elevation gradient that extends from 2990 to 4680 masl and is part of the Trans-Mexican Volcanic Belt (Mastretta-Yanes et al., 2015). Conifer forests (Pinus spp. and Abies religiosa) and small patches of broadleaf species (Quercus sp. and Alnus jorullensis) occur between 2990 and 3200 masl, whilst alpine grasslands occur above 3700 masl (Rzedowski, 1975; Challenger and Soberón, 2008) (Figure 1A). Two important basins occur within the Nevado de Toluca: The Lerma Santiago Basin and the Balsas Basin which provide water resources to the Nevado de Toluca region (with 10,255 inhabitants) the city of Toluca (located ∼ 23 km east of the volcano with 873,536 inhabitants) and to Mexico City (located ∼ 95 km east from the volcano with 8,918,653 inhabitants; National Institute for Statistics and Geography, 2015).
The climate at Nevado de Toluca is temperate, with a mean minimum temperature of −2.9°C and a mean maximum temperature of 10.5°C. Mean annual precipitation is 1216 mm (Station 00015062; National Meteorological Service, 2019). The rainy season occurs from July to September. Approximately 90% of the soils at Nevado de Toluca are Andosols which typically exhibit low bulk densities, high organic matter, low pH with the organic matter stabilized through formation of aluminum-humus complexes (Peña-Ramírez et al., 2009). Other soil types such as Phaeozems, Regosols, and Cambisols also occur in small proportions (Vilchis, 2006; National Institute for Statistics and Geography, 2015).
We stratified our study area according to the dominant habitat types, aspect, and based on access granted by local communities (ejidos). In total, we studied four natural forests, one dominated by P. hartwegii and three by A. religiosa; two A. religiosa and P. pseudostrobus managed old forests and a 30-year-old plantation of P. montezumae. We also studied a natural unburnt alpine grassland of Festuca tolucensis, a-burnt alpine grassland of F. tolucensis which is subject to controlled fire every 3 years and an arable land (Figure 1A and Table 1). At each of our habitats, we assessed whole ecosystem OC stocks, including the soil component. Within forests we assessed both aboveground and belowground biomass and their associated OC stock, and OC from the litter, understory vegetation, downed wood and soil. For our alpine grassland’s habitats, we assessed aboveground and belowground biomass and their associated OC stock and soil OC stock. For the arable habitat, we assessed soil OC stock. Within all our studied habitats, we also assessed soil nitrogen density and soil pH. Detailed explanation of the sampling and analyses are described below.
Within each of the forests, we established six plots. Plot size was 35 m × 35 m, where DBH of trees was > 50 cm. Plot size was 25 m × 25 m, where DBH of trees varied between 14 and 50 cm. Forests plots were 50 m apart (Pearson et al., 2007). For each of the alpine grasslands’ habitats, we established six plots of 5 m × 30 m and within each plot we established six subplots of 0.25 m × 0.25 m (Santini et al., 2019b). Plots for alpine grasslands were 30 m apart. For our arable habitat, we established a plot of 300 m × 300 m and we sampled a total of nine points every 100 m (Muñoz-Rojas et al., 2016).
To examine aboveground OC and belowground OC of forests, we assessed DBH of all trees > 5 cm within our established plots. Aboveground biomass of trees was estimated using allometric equations (Table 2). The OC from aboveground biomass was estimated by using a conversion factor of 0.48 (the proportion of carbon in aboveground biomass; Mendoza-Ponce and Galicia, 2010) multiplied by the dry biomass (kg m–2) and converted to Mg OC ha–1. We estimated belowground biomass of trees by using the equation of Cairns et al. (1997) and by multiplying the dry biomass (kg m–2) by 0.37 as determined by Mendoza-Ponce and Galicia (2010). We then converted kg m–2 to Mg OC ha–1.
Table 2. Allometric equations for the calculation of aboveground and belowground biomass of the studied species.
We measured standing dead trees following the same criteria used for live trees. Standing dead trees were divided in three categories: (1) dead trees without leaves, (2) dead trees without secondary branches and (3) dead trees without primary and secondary branches. Biomass for dead trees without leaves was calculated as the total dry biomass minus the biomass of leaves, equivalent to 3% of the total biomass (Pearson et al., 2007). For dead trees without secondary branches, biomass was calculated as the total dry biomass minus 15% of the total biomass (Návar, 2009). Finally, the biomass of dead Pinus spp. trees without primary and secondary branches was calculated as the equivalent of 62% of the total biomass (Návar, 2009) and the biomass of dead A. religiosa trees without primary and secondary branches was calculated as the equivalent of 80% of the total biomass (Avedaño-Hernández et al., 2009). The OC from standing dead trees was estimated by using a conversion factor of 0.48 multiplied by the dry biomass (kg m–2) and converted to Mg OC ha–1 (Mendoza-Ponce and Galicia, 2010).
To examine aboveground biomass of alpine grasslands we harvested aboveground biomass within four to six subplots established at each plot. The biomass was oven-dried at 60°C for 72 h and weighed. The OC stocks of total aboveground biomass were determined by using a conversion factor of 0.40 (the assessed proportion of carbon in aboveground biomass of alpine grasslands) multiplied by the dry biomass (kg m–2) and converted to Mg OC ha–1. To determine belowground biomass of natural unburnt alpine grasslands, we collected a total of 15 cores of 30 cm depth (i.e., the observed root-depth) within the established plots. Cores were collected with a stainless-steel gauge auger of 3.2 cm diameter (Dormer Soil Samplers, Australia). Belowground biomass from our burnt alpine grassland was estimated with an allometric equation developed from our data of aboveground and belowground biomass of natural unburnt alpine grasslands (Supplementary Figure S1 and Table 2).
Within forests, we assessed OC from litter by collecting all forest floor material within 0.25 m × 0.25 m subplots within each of the six plots. Additionally, within our A. religiosa (site 1) and P. montezumae habitats, we assessed OC from the understory vegetation by collecting all understory vegetation material within 0.25 m × 0.25 m subplots at each of the six plots. Both, litter and understory vegetation material were dried at 60°C for 7 days and weighed. A fraction of our samples was processed with a TissueLyser II (Qiagen, Venlo, Netherlands) and was analyzed for OC content with a continuous-flow gas-ratio mass spectrometer (Finnigan Delta PlusXL, Waltham, MA, United States) coupled to an elemental analyzer (Costech Analytical Tech, Inc., Valencia, CA, United States) at the Environmental Isotope Laboratory, University of Arizona, United States. Dry weight (kg m–2) was multiplied by a conversion factor of 0.42 (i.e., the OC content for our samples) and converted to Mg OC ha–1.
Within each plot of our studied forests, we established two transects of 50 m. We assessed the diameter of fragments of downed wood > 10 cm in diameter intersecting the transect (Pearson et al., 2007). Downed wood was classified into three groups: sound, intermediate or rotten. We assessed specific gravity of 30 pieces of sound, intermediate and rotten downed wood per forest as the oven-dry mass of the wood sample divided by the mass of water displaced by its fresh volume (Chave et al., 2006). Using the specific gravity for each group of wood, biomass was calculated and converted to OC by using a conversion factor of 0.5 (Pearson et al., 2007).
We determined soil OC stocks and soil nitrogen at bedrock depths for our alpine grasslands (0–50 cm) and forests (0–100 cm). The subsoil of our arable habitat was highly compacted and we were only able to sample at depths of 0–50 cm. Soil OC stocks and nitrogen content were assessed by collecting one core at each of the plots by using a stainless-steel gauge auger of 3.2 cm diameter (Dormer Soil Samplers, Australia). Each core was divided into depth intervals of 0–15, 15–30, 30–50 cm and, when applicable, 50–100 cm. To determine soil bulk density, a known volume of soil was dried at 60°C for 3 days to a constant mass and weighed. Soil bulk density was calculated as dry mass divided by volume. Samples were homogenized with a TissueLyser II (Qiagen, Venlo, Netherlands). OC content and nitrogen were assessed with a continuous-flow gas-ratio mass spectrometer (Finnigan Delta PlusXL, Waltham, MA, United States) coupled to an elemental analyzer (Costech Analytical Tech, Inc., Valencia, CA, United States) at the Environmental Isotope Laboratory, University of Arizona, United States.
We determined soil OC stocks as per Eq. 1:
Then we converted g OC cm–2 to Mg OC ha–1 as per Eq. 2:
Finally, we added up the amount of OC from each core layer to the total sampling depth (that is 50 or 100 cm). We also determined soil OC density and soil nitrogen density as per Eqs 3 and 4:
We assessed soil pH by using a soil: water ratio 1:2 with a pH meter HI 2210 (Hanna Instruments, RI, United States).
Conversion from forestry to arable land within the Nevado de Toluca Conservation Area is likely to occur in the next few years if conservation incentives are not placed. Thus, we estimated OC losses (see Eq. 5) from conversion of 1 ha of forest to arable land by considering that 100% of the biomass OC is burnt and thus emitted as CO2. We also assumed that a conservative value of 40% of soil OC loss occurs within the top 0–50 cm after conversion (Guo and Gifford, 2002).
We used a mean pricing value of carbon of US $ 3 per Mg CO2 (World Bank and Ecofys, 2018) to estimate potential monetary incentives for conservation of forests that can be provided to the local communities (ejidos) that granted permission to study their lands.
We used a one-way analysis of variance (ANOVA) to test for differences in whole ecosystem OC stocks. We also assessed differences in OC stocks from aboveground biomass, downed wood, litter, belowground biomass, and soil at two depths (i.e., 0–50 and 0–100 cm). We used habitat as fixed factor and plot as a random factor nested within habitat. We used a Tukey post hoc test to compare mean OC stocks among habitats. A two-way ANOVA was used to test for differences between habitat and depth (0–15, 15–30, 30–50, 50–100 cm) for soil pH, bulk density and soil OC density and nitrogen density. In this two-way ANOVA, habitat and depth were fixed factors, and when applicable, plot was a random factor nested within habitat.
Understory vegetation was only dominant at our A. religiosa (site 1) and P. montezumae forests. Thus, we used a t-test to determine if there were significant differences between these habitats in the OC of understory vegetation.
A linear regression analysis was used to determine the relationship between belowground biomass and aboveground biomass for unburnt alpine grasslands. We also used linear regression analyses to determine the relationship between OC from aboveground biomass and soil OC and between OC from belowground biomass and soil OC. Parametric test assumptions of normality and homogeneity of variances were tested using D’Agostino–Pearson, Shapiro–Wilk, and Bartlett’s tests. We used Prism version 8.1.2 for Mac (GraphPad Software, La Jolla, CA, United States) for our statistical analyses.
We found values of OC from aboveground biomass to be significantly different among habitats (F(8,43) = 10.27, p < 0.0001). The OC from aboveground biomass was lowest in our burnt and unburnt grassland habitats, with values of 2.50 ± 0.74 and 8.33 ± 1.80 Mg OC ha–1, respectively (Figure 2a and Table 3). OC from aboveground biomass was highest in the three conserved A. religiosa habitats with values of 133 ± 25 to 215 ± 42 Mg OC ha–1 (Figure 2a and Table 3). Values of OC from belowground biomass were also significantly different among habitats (F(8,43) = 10.73, p < 0.0001). OC from belowground biomass was lowest in our grassland habitats, which was 4.10 ± 0.90 Mg OC ha–1 for the burnt grassland habitat and 5.34 ± 0.90 Mg OC ha–1 for the unburnt grassland habitat, and was highest in the three A. religiosa forests with values of 24.14 ± 1.80 to 38.20 ± 6.75 Mg OC ha–1 (Figure 2b and Table 3).
Figure 2. Organic carbon (OC) stocks at our 10 studied habitats from the Nevado de Toluca, Mexico. (a) OC from aboveground biomass, understory vegetation, downed wood and litter when present and (b) OC from belowground biomass, and soil OC at different depths. (c) Examples of our studied habitats, alpine grasslands, P. montezumae, A. religiosa and P. pseudostrobus (site 2), A. religiosa (site 3) and arable land habitats.
Table 3. Organic carbon stocks (in Mg OC ha–1) at our studied montane habitats within the Nevado de Toluca Flora and Fauna Conservation Area, State of Mexico, Mexico.
We found dominant understory vegetation at our A. religiosa (site 1) and P. montezumae habitats. The OC from the understory vegetation was similar between these habitats, that is 1.88 ± 0.75 Mg OC ha–1 for A. religiosa (site 1) and 2.81 ± 0.73 Mg OC ha–1 for P. montezumae (p = 0.52; Figure 2a and Table 3). For litter, we found no significant differences among habitats with values ranging from 5.24 ± 1.1 Mg OC ha–1 at the P. hartwegii habitat to 10.50 ± 1.37 Mg OC ha–1 at the A. religiosa (site 2) habitat (F(6,34) = 1.13; p = 0.36; Figure 2a and Table 3). In addition, we found significant differences for downed wood among habitats (F(6,34) = 2.4; p = 0.04). Our A. religiosa habitats (sites 2 and 3), had values of 67.8 ± 20.7 and 109.7 ± 60.1Mg OC ha–1, respectively, which were significantly highest than those for other forests (Figure 2a and Table 3).
Significant differences in soil properties, including soil OC stocks were observed between habitats. When considering the whole soil profile, that is, at bedrock depths for our alpine grasslands and forests and at 0–50 cm depth for our arable land, soil OC stock was highest in the A. religiosa forests with mean values ranging from 236 to 397 Mg OC ha–1, intermediate values were found for the P. montezumae and the A. religiosa and P. pseudostrobus forests with mean values of 183 to 232 Mg OC ha–1. Lowest values of soil OC were found for grasslands, P. hartwegii and the arable land with mean values ranging from 54 to 183 Mg OC ha–1 (F(9,53) = 15.92; p < 0.0001; Table 3). Significant differences were also found when comparing the soil profile at depths 0–50 cm across habitats (F(9,53) = 17.9; p < 0.0001, Table 3).
Bulk density and pH increased with soil depth (p < 0.0001; Tables 4, 5). In contrast, OC and N density decreased with increasing soil depth (p < 0.0001; Tables 4, 5). Among habitats pH was lowest in the arable habitat (i.e., 5.18 ± 0.08) and highest in the A. religiosa and P. pseudostrobus (site 1) and the A. religiosa (site 2) habitats with values of 6.33 ± 0.22 and 6.40 ± 0.22, respectively (F(9,224) = 13.45; p < 0.0001, Table 4). Bulk density was different among habitats (F(9,224) = 24.54; p < 0.0001). Bulk density was lowest at the A. religiosa and P. pseudostrobus (site 2) and the A. religiosa (site 3) habitats with values of 0.44 ± 0.02 and 0.43 ± 0.02 g cm–3, respectively. Highest values of bulk density were 0.74 ± 0.02 g cm–3 in the unburnt alpine grassland habitat. OC density was highest in the A. religiosa (sites 2 and 3) forests with mean values of 37.7 ± 8.6 and 44.0 ± 4.8 mg cm–3, respectively and lowest values of 11.2 ± 1.4 and 12.5 ± 1.8 mg cm–3 in the unburnt grassland and P. hartwegii forest respectively (F(9,224) = 6.33; p < 0.0001; Table 4). Similar to OC density, nitrogen density was lowest in our unburnt grassland and P. hartwegii habitats with values of 0.8 ± 0.1 and 1.3 ± 0.1 mg cm–3 respectively and highest in the A. religiosa (sites 2 and 3) forests with values of 2.3 ± 0.5 and 2.8 ± 0.3 mg cm–3, respectively (F(9,224) = 7.30; p < 0.0001; Table 4).
Table 4. Physicochemical characteristics of soils at our studied habitats at Nevado de Toluca, State of Mexico, Mexico.
Table 5. Results of a two-way ANOVA statistical tests for bulk density (g cm–3), pH, soil organic carbon (OC) density (mg cm–3), and soil nitrogen (N) density (mg cm–3).
The ecosystem OC stocks were significantly different among habitats (F(9,53) = 10.47, p < 0.0001). We found that the A. religiosa (sites 2 and 3) forests exhibited the highest whole ecosystem OC stocks with values of 633 ± 102 and 668 ± 163 Mg OC ha–1 respectively, intermediate values of whole ecosystem OC stocks were found in the P. montezumae, A. religiosa, and P. pseudostrobus and A. religiosa (site 1) forests with mean values ranging from 304 to 466 Mg OC ha–1 (Figure 2 and Table 6). We found lowest whole ecosystem OC stocks in our grassland habitats, the arable land and the P. hartwegii forest with values ranging from 68 to 227 Mg OC ha–1 (Figure 2 and Table 6).
Table 6. Whole organic carbon stocks (Mg OC ha–1) at our studied montane habitats within the Nevado de Toluca Flora and Fauna Conservation Area, State of Mexico, Mexico.
We found a significant linear relationship between soil OC and aboveground biomass OC (R2 = 0.60, p = 0.02, Figure 3A) and between soil OC and belowground biomass OC (R2 = 0.62, p = 0.01, Figure 3B).
Figure 3. (A) Relationship between soil OC (Mg OC ha–1) and aboveground OC (Mg OC ha–1) at nine habitats within the Nevado de Toluca Conservation Area. The regression line was y = 1.2 x + 71.5, R2 = 0.6, p = 0.02. (B) Relationship between soil OC (Mg OC ha–1) and belowground OC (Mg OC ha–1) at nine habitats within the Nevado de Toluca Conservation Area. The regression line was y = 8.5 x + 36.6, R2 = 0.62, p = 0.01.
We estimated the avoided emissions from conversion of forests to arable land (Figure 4) based on assumptions that 100% of biomass and 40% of soil OC from 0 to 50 cm is emitted as CO2. Our estimated values of avoided CO2 emissions range from 616 Mg CO2 ha–1 for the P. hartwegii natural forest to 1,671 Mg CO2 ha–1 for the A. religiosa (site 3) forest. Conservation of every ha of forests can provide monetary incentives ranging from US$1,848 to US$5,014 if we consider a mean carbon price of US$ 3 (Figure 4).
Figure 4. Estimates of avoided CO2 emissions from conservation of forests and the value for forest conservation in $US assuming every Mg CO2 is worth US$ 3.
We assessed whole ecosystem OC stocks at 10 dominant montane habitats at the Flora and Fauna Conservation Area, Nevado de Toluca, Mexico. Whole ecosystem OC stocks ranged from 68 Mg OC ha–1 in alpine grasslands to 668 Mg OC ha–1 in an A. religiosa forest. We found that the soil OC to bedrock depth comprises up to 63% of the whole OC stock in forests and up to 93% in alpine grasslands. We also found that avoiding conversion of 1 ha of A. religiosa forests to arable lands can avoid emissions of 1,122 to 1,671 Mg CO2 ha–1, these values are equivalent to avoiding emissions of 478,000 to 712,000 L of consumed gasoline (United States Environmental Protection Agency, 2020). Thus, conservation of natural forests may be promoted as a strategy to mitigate CO2 emissions and meet the targets of Mexico toward the Paris Agreement on Climate Change by 2030. Implementation can be established through voluntary carbon payments to local communities that are responsible for safeguarding these forests, and should be accompanied by sustainable -forestry and -arable practices.
The OC stocks from aboveground biomass of our studied forests are similar to those reported by Mendoza-Ponce and Galicia (2010) for Pinus spp., i.e., 36–146 Mg OC ha–1 and for A. religiosa, i.e., 178 Mg OC ha–1. Mendoza-Ponce and Galicia (2010) found that old pine forests of ∼103 years store more carbon than younger reforested pine forests of 12–28 years old within the biomass component. Because old forests exhibit higher OC stocks than younger forests, and because sequestration of OC, can take decades to centuries, management strategies that favor conservation of old forests such as our A. religiosa habitats are critical to mitigate CO2 emissions (Juying et al., 2009; Global Forest Resources Assessment, 2020). In addition to forest age, other functional-traits such as wood density, tree height and diameter at breast height have been shown to impact biomass levels and forest productivity (De Deyn et al., 2008). These differences may also explain variations in OC stocks between A. religiosa and Pinus spp.
The occurrence of highly biodiverse taxa and variability of functional-traits within Mexican mountain regions is the result of long evolutionary processes, and often explains their resilience to environmental changes (Mastretta-Yanes et al., 2015; Cruz-Nicolás et al., 2019). Therefore, conservation strategies must ensure the maintenance of species diversity, for example by using native species during reforestation campaigns, as well as ensuring connectivity among habitats to allow the species and gene-flow movement across elevational gradients (Wehenkel et al., 2017).
The OC stocks within the first meter of soil in our A. religiosa forests are up to 65% higher than the mean value (i.e., 153 Mg OC ha–1) for other conserved A. religiosa sites within the State of Mexico as reported by Pérez-Ramírez et al. (2013). However, the definition of conserved forests within the Pérez-Ramírez et al. (2013) study includes forests that have not been altered in 25 years, while our A. religiosa sites are old forests of more than 75 years old (Mendoza-Ponce and Galicia, 2010). Thus, the high values of soil OC stocks in our study may be associated to long-term accumulation of organic vegetation and woody debris within the soils at these sites (Chen et al., 2013). OC stocks of soils for our Pinus spp. habitats were similar to those reported for Pinus spp. at 0.3–1 m depth, that is, 93.1 to 172.6 Mg OC ha–1 (de Jong et al., 1999; Acosta-Mireles et al., 2008; Ordoñez et al., 2008).
Alpine grasslands are highly vulnerable ecosystems that only occur at high elevations across seven volcanoes, and cover only 0.008% of the Mexican landscape. However, despite having low carbon stocks compared to forests, alpine grasslands are a habitat for endemic (such as Castilleja tolucensis) and iconic species (including the red lynx, Lynx rufus, and the volcano rabbit, Romerolagus diazi). Despite its importance, these grasslands have been threatened by afforestation programs, and by visitors’ extraction of native flora (Maza-Villalobos et al., 2019). Governmental agencies such as the CONAFOR and CONANP must develop urgent programs to inform local communities, tourists and frequent visitors, such as hikers, of the functions and vulnerability of Mexican alpine grasslands.
Aboveground biomass OC from our alpine grasslands is within the ranges reported for alpine grasslands in Mexico dominated by Calamagrostis spp. and Festuca spp., i.e., 2.73–8.57 Mg OC ha–1 (Mendoza-Ponce and Galicia, 2010). To our knowledge no previous studies have reported OC stocks of soils for Mexican alpine grasslands. Yet, our results are similar to those from the Tibetan Plateau alpine grasslands at 0.5 m depth, i.e., 54 Mg OC ha–1 (Yang et al., 2008) and lower than soil OC stocks for Andean alpine grasslands comprising Calamagrostis longearistata and Ageratina ciszoenzis at 0.3 m depth, i.e., 137–285 Mg OC ha–1 (Oliveras et al., 2014). High values of soil OC stocks in the Andean alpine grasslands may reflect the high-water content of those soils that results in low rates of OC decomposition (Oliveras et al., 2014).
The current Comisión Nacional Forestal (2018) does not consider the soil OC stock component of ecosystems due to a lack of data. However, we found that the soil OC stock component for our studied montane habitats comprises from 40% (at the P. hartwegii habitat) to 93% (at the burnt alpine grasslands) of the total ecosystem OC stock. This considerably expands the carbon stock within these ecosystems. As such, incorporating the soil OC stock within inventories of Mexican ecosystems by the CONAFOR will further motivate strategies to reduce emissions from deforestation and forest degradation.
Our results also suggest that organic carbon from living biomass may also reflect biomass inputs, which determine soil OC stocks across our studied habitats (Chen et al., 2013). be These results are consistent with Li et al. (2010), who found that aboveground biomass, mean tree-height and stand-density explained 76.7% of the variation in soil OC across temperate forests in Japan. In addition, Tashi et al. (2016), found that high basal area and leaf litter inputs increased stocks of soil OC in subtropical, broadleaf, and temperate forests. We did not assess living biomass from our arable habitat, as it is highly variable across the annual crop cycle between planting and harvesting. However, assessing this biomass variability across the arable cycle is a valuable topic for further research.
A major cause of montane deforestation is their conversion to arable lands. This conversion reduces carbon inputs and/or increases OC losses compared to the original vegetation. This results in reductions of ∼40% of the soil OC stock over time (Guo and Gifford, 2002). Our arable habitat has been subject to crop rotation of potato (i.e., Solanum tuberosum) and broad-bean (i.e., Vicia faba L.) every 3 to 5 years and while sampling we observed crop residues such as potatoes. We suggest that these crop residues are incorporated within the soils having a positive effect in the OC stock. Both, crop rotation and the incorporation of crop residues within arable soils have been proposed as strategies to increase soil OC stocks (e.g., Smith et al., 1998; West and Post, 2002). For example, Nunes Carvalho et al. (2014) found soil OC stocks increased from 66 to 73 Mg OC ha–1, by increasing primary production after 9 years of crop-pasture rotation.
Despite relative high levels of soil OC in the top 0–50 cm, the arable land also exhibited highly compacted subsoils, which are likely linked to the use of heavy machinery (NSS field observation). Soil compaction results in soils with high bulk density, which has negative impacts on soil functions due to reduced water infiltration, reduced oxygen levels and microbial activity that typically impedes root growth (Weil and Brady, 2016). This soil compaction in our studied arable habitat may reduce long-term productivity unless soil management practices are improved.
We observed similar soil OC stocks in the two grassland habitats and the P. hartwegii habitat, despite the P. hartwegii forest exhibiting aboveground biomass of over 12 times that of the grasslands. All three habitats occur at relatively high elevations (i.e., >3900 masl). Our data and observations indicate that lower soil OC stocks at higher elevations at our studied habitats may be associated with erosion due to strong winds and snow melt-processes at high elevations (e.g., Duniway et al., 2019). These habitats are subject to stronger winds (i.e., 25 km h–1) than our lower elevation habitats (i.e., 11 km h–1; National Meteorological Service, 2019). Winds > 70 km h–1 have been recorded at high elevation areas at the Nevado de Toluca (National Meteorological Service, 2019). Additionally, bare patches and less dense vegetation (i.e., grasslands versus forest) can result in increased erosion rates at these habitats (e.g., Juying et al., 2009).
Our study shows that avoiding the conversion of 1 ha of conserved old forests of A. religiosa to arable lands could avoid the emissions 1,122 to 1,671 Mg CO2 ha–1. Considering a mean value of carbon of US$ 3 per Mg CO2 (World Bank and Ecofys, 2018), the conservation of 1 ha of A. religiosa forest can be worth between US$ 3,365 to US$ 5,014. The OC stocks reported in this study can contribute to national and international carbon stock inventories and enable the inclusion of Nevado de Toluca lands within voluntary carbon credit markets for CO2 mitigation. The redemption of these carbon stock on carbon credit markets can provide financial incentives for the conservation of the forests by the local communities.
A range of local communities in Mexico have successfully established conservation and sustainable forestry programs. This includes joint efforts with the CONANP, the CONAFOR and non-profit organizations such as the Mexican civil council for sustainable silviculture (CCMSS by its Spanish acronym) and the Forest Stewardship Council (FSC). Additionally, local communities such as Ejido Santiago Tlacotepec have joined the voluntary carbon market for forest conservation through the Mexican Carbon Platform (MEXICO2). This study provides further motivation for these local communities and organizations to measure carbon stocks within their montane regions, and apply to these carbon markets that incentivize long-term conservation of montane ecosystems.
Organic carbon stocks from our studied montane habitats range from 68 Mg OC ha–1 in alpine grasslands to 668 Mg OC ha–1 in conserved A. religiosa forests. Land conversion from natural forests such as A. religiosa to arable land can result in significant CO2 emissions which could be avoided with conservation. The measurement of organic carbon stocks from this research can incentivize local communities, government and non-profit organizations to include montane ecosystems within voluntary carbon-markets programs to improve habitat conservation and to reach the targets of the Paris Agreements to mitigate the CO2 emissions of Mexico by 2030.
We recommend Mexican government agencies improve the awareness of local communities on the importance of forest conservation to the sustainable, long-term provision of ecosystem services, including water, clean air, and recreational spaces. Forest conservation is also important for the provision of nutrients and water resources to neighboring arable lands, pastures or plantations. These conservation practices must include the protection of native species, and maintaining connectivity across habitats, including alpine grasslands, and should be accompanied by sustainable practices in neighboring arable lands.
The organic carbon stock in soils comprises from 40 to 93% of the total organic carbon stock in Mexican montane ecosystems. Therefore, carbon stock inventories should include soil carbon stocks to better estimate the CO2 emissions that result from the conversion of forests to arable lands. Further research may assess changes to carbon stock due to varying biomass in arable lands during the annual crop-cycle of planting and harvesting as well as the study of broadleaf montane forests.
All datasets generated for this study are included in the article/Supplementary Material.
Written informed consent was obtained from the individual(s) for the publication of any potentially identifiable images or data included in this article.
NS, AV-A, MA, CL, RN, NG-R, AM-Y, EG, and DP: study design. NS, AV-A, NG-R, and BO-R: field work. NS, MA, CL, RN, EG, and DP: writing – reviewing and editing. All authors contributed to the article and approved the submitted version.
This work was supported by the National Council of Science and Technology (CONACYT) through a Cátedra program to NS and Scientific Licence Number SGPA/DGGFS/712/1596/17; Bitácora 09/N1-0120/05/17.
The authors declare that the research was conducted in the absence of any commercial or financial relationships that could be construed as a potential conflict of interest.
We would like to particularly thank the communities of Ejido Santiago Tlacotepec, Ejido Rincón de Guadalupe, and Ejido San Bartolo at Nevado de Toluca as they provided support and permission to perform this study. We also thank the following people for technical support: Eusebio Roldán Félix from the Consejo Civil Mexicano para la Silvicultura Sostenible, Ana E. Escalante and Karen Carrasco from the Laboratorio Nacional de Ciencias de la Sostenibilidad at the National Autonomous University of Mexico (UNAM), Juan Pablo Jaramillo-Correa from the Instituto de Ecología UNAM for supervision of AV-A, and David Dettman and Xiaoyu Zhang from the Environmental Isotope Laboratory, University of Arizona, United States.
The Supplementary Material for this article can be found online at: https://www.frontiersin.org/articles/10.3389/fenvs.2020.581476/full#supplementary-material
Acosta-Mireles, M., Vargas-Hernández, J., Velásquez-Martínez, A., and Etchevers-Barra, J. D. (2002). Estimación de la biomasa aérea mediante el uso de relaciones alométricas en seis especies arbóreas en Oaxaca, México. Agrociencia 36, 725–736.
Acosta-Mireles, A., Carrillo-Anzures, F., and Lavariega, M. D. (2008). Determinación del carbono total en bosques mixtos de Pinus patula Schl Et Cham. Terra Latinoamericana 27, 105–114.
Aguirre-Calderón, O., and Jiménez-Pérez, J. (2011). Evaluación del contenido de carbono en bosques del sur de Nuevo León. Rev. Mex. Cien. Forest. 2, 73–84. doi: 10.29298/rmcf.v2i6.575
Agrawal, A., Nepstad, D., and Chhatre, A. (2011). Reducing emissions from deforestation and forest degradation. Ann. Rev. Environ. Resour. 36, 373–396.
Ariza, C., Maselli, D., and Kohler, T. (2013). Mountains: Our Life, Our Future. Progress and Perspectives On Sustainable Mountain Development from Rio 1992 to Rio 2012 and Beyond. Bern: Swiss Agency for Development and Cooperation (SDC).
Avedaño-Hernández, D. M., Acosta-Mireles, M., Carrillo-Anzures, F., and Etchevers-Barra, J. D. (2009). Estimación de biomasa y carbono en un bosque de Abies religiosa. Rev. Fitotec. Mex. 32, 233–238.
Ayala-López, R., De Jong Bergsma, B. H. J., and Ramírez-Maldonado, H. (2001). Ecuaciones para estimar biomasa en la Meseta Central de Chiapas. Rev. Chap. Ser. Cien. Forest. Ambi.. 7, 153–157.
Berhongaray, G., Cotrufo, F. M., Janssens, I. A., and Ceulemans, R. (2019). Below-ground carbon inputs contribute more than above-ground inputs to soil carbon accrual in a bioenergy poplar plantation. Plant Soil. 434, 363–378. doi: 10.1007/s11104-018-3850-z
Bonilla, E. (2009). Uso de Ecuaciones Alométricas Para Estimar Biomasa y Carbono en Pinus montezumae Lamb. Thesis, Universidad Autónoma Chapingo, Mexico, 70.
Cairns, M. A., Brown, S., Helmer, E. H., and Baumgardner, G. A. (1997). Root biomass allocation in the world’s upland forests. Oecologia 111, 1–11. doi: 10.1007/s004420050
Challenger, A., and Soberón, J. (2008). ‘Los ecosistemas Terrestres’ in Capital Natural de México Conocimiento Actual de la Biodiversidad, Vol 1. México: Comisión Nacional para el Conocimiento y Uso de la Biodiversidad, 87–108.
Chave, J., Muller-Landau, H. C., Baker, T. R., Easdale, T. A., Steege, H., and Webb, C. O. (2006). Regional and phylogenetic variation of wood density across 2456 neotropical tree species. Ecol. Appl.. 16, 2356–2367. doi: 10.1890/1051-0761(2006)016%5B2356:rapvow%5D2.0.co;2
Chen, G., Yang, Z., Gao, R., Xie, J., Guo, J., Huang, Z., and Yang, Y. (2013). Carbon storage in a chronosequence of Chinese fir plantations in southern China. For. Ecol. Manag. 300, 68–76. doi: 10.16/j.foreco.2012.07.046
Crowther, T. W., Todd-Brown, K. E. O., and Bradford, M. A. (2016). Quantifying global soil carbon losses in response to warming. Nature 540, 104–108. doi: 10.1038/nature20150
Cruz-Nicolás, J., Giles-Pérez, G., González-Linares, E., Múgica-Gallart, J., Lira-Noriega, A., Gernandt, D. S., et al. (2019). Contrasting evolutionary processes drive morphological and genetic differentiation in a subtropical fir (Abies, Pinaceae) species complex. Bot. J. Linn. Soc.. 192, 401–420. doi: 10.1093/botlinnean/boz077
De Deyn, G. B., Cornelissen, J. H. C., and Bardgett, R. D. (2008). Plant functional traits and soil carbon sequestration in contrasting biomes. Ecol. Lett. 11, 516–531. doi: 10.1111/j.1461-0248.2008.01164.x
de Jong, B. H., Cairns, M. A., Haggerty, P. K., Ramírez-Marcial, N., Ochoa-Gaona, S., Mendoza-Vega, J., et al. (1999). Land-use change and carbon flux between 1970s and 1990s in Central Highlands of Chiapas, Mexico. Environ. Manag. 23, 373–385. doi: 10.1007/s002679900193
Duniway, M. C., Pfennigwerth, A. A., Fick, S. E., Nauman, T. W., Belnap, J., and Barger, N. N. (2019). Wind erosion and dust from US drylands: a review of causes, consequences, and solutions in a changing world. Ecosphere 10:e02650. doi: 10.1002/ecs2.265
Galicia, L., and García-Romero, A. (2007). Land Use And Land Cover Change in Highland Temperate Forests in the Itzta-Popo National Park. Central Mexico. Mountain Research and Development.
Guo, L. B., and Gifford, R. M. (2002). Soil carbon stocks and land use change: a meta analysis. Glob. Change Biol. 8, 345–360. doi: 10.1046/j.1354-1013.2002.00486.x
IPBES (2018). The IPBES assessment report on land degradation and restoration. L. Montanarella, R. Scholes, and A. Brainich (Eds). Secretariat of the Intergovernmental Science-Policy Platform on Biodiversity and Ecosystem Services(Bonn: IPBES),744
Juying, J., Houyuan, Z., Yanfeng, J., and Ning, W. (2009). Research progress on the effects of soil erosion on vegetation. Acta Ecol. Sin. 29, 85–91. doi: 10.1016/j.chnaes.2009.05.001
Keith, H., Mackey, B. G., and Lindenmayer, D. B. (2009). Re-evaluation of forest biomass carbon stocks and lessons from the world’s most carbon-dense forests. Proc. Natl. Acad. Sci. U.S.A. 106, 11635–11640. doi: 10.1073/pnas.0901970106
Koörner, C., and Ohsawa, M. (2005). Mountain systems: Millennium Ecosystem Assessment, Current State and Trends Assessment. Island Press: Washington, 681–716.
La Sorte, F. A., and Jetz, W. (2010). Projected range contradiction of montane biodiversity under global warming. Proc. R. Soc. B. 277, 3401–3410. doi: 10.1098/rspb.2010.0612
Ley Agraria (1992). Diario Oficial de la Federación. México. Available online at: http://www.diputados.gob.mx/LeyesBiblio/ref/lagra/LAgra_orig_26feb92_ima.pdf
Li, P., Wang, Q., Endo, T., Zhao, X., and Kakubari, Y. (2010). Soil organic carbon stock is closely related to aboveground vegetation properties in cold-temperate mountainous forests. Geoderma 154, 407–415. doi: 10.1016/j.geoderma.2009.11.023
Madrid, L., Núñez, J. M., Quiroz, G., and Rodríguez, Y. (2009). La propiedad social forestal en México. Invest. Ambien 1, 179–196
Mastretta-Yanes, A., Cao, R., Nicasio-Arzeta, S., Quadri, P., Escalante-Espinosa, T., Arredondo, L., et al. (2014). Ser exitosa la estrategia del cambio de categor a para mantener la biodiversidad del Nevado de Toluca? Oikos 12, 7–17.
Mastretta-Yanes, A., Moreno-Letelier, A., Pi ero, D., Jorgensen, T. H., and Emerson, B. C. (2015). Biodiversity in the Mexican highlands and the interaction of geology, geography and climate within the Transmexican Volcanic Belt. J. Biogeogr. 42, 1586–1600. doi: 10.1111/jbi.12546
Maza-Villalobos, S., Cotler, H., Almeida-Leñero, L., Hoth, J., Steinmann, V., Mastretta, A., et al. (2019). Conservando el pastizal alpino mexicano; conocimientos, amenazas y esperanzas. Biodiv. Mexico. 142, 12–16.
Mendoza-Ponce, A., Corona-Núñez, R. O., Galicia, L., and Kraxner, F. (2019). Identifying hotspots of land use cover change under socioeconomic and climate change scenarios in Mexico. Ambio 48, 336–349. doi: 10.1007/s13280-018-1085-0
Mendoza-Ponce, A., and Galicia, L. (2010). Aboveground and belowground biomass and carbon pools in Highland temperate forests landscape in Central Mexico. Forestry 83, 497–506
Muñoz-Rojas, M., Abd-Elmabod, S. K., Zavala, L. M., De la Rosa, D., and Jordán, A. (2016). Climate change impacts on soil organic carbon stocks of Mediterranean agricultural areas: a case study in Northern Egypt. Agric. Ecosyst. Environ. 238, 142–152. doi: 10.1016/j.agee.2016.09.001
Comisión Nacional Forestal (2018). National Forestry and Soils Inventory ‘2009 – 2014’. México: Comisión Nacional Forestal.
National Institute for Statistics and Geography (2015). ‘Población’. México: Instituto Nacional de Estadística y Geografía. Available online at: https://www.inegi.org.mx/temas/estructura/
National Meteorological Service (2019). ‘Información Estadística Climatológica’. México: Servicio Meteorológico Nacional. Available online at: https://smn.conagua.gob.mx/es/climatologia/informacion-climatologica/informacion-estadistica-climatologica
Návar, J. (2009). Allometric equations for tree species and carbon stocks for forests of northwestern Mexico. Forest Ecol. Manag. 257, 427–434. doi: 10.1016/j.foreco.2008.09.028
Nogués-Bravo, D., Araújo, M. B., Errea, M. P., and Martínez-Riza, J. P. (2007). Exposure of global mountain systems to climate warming during the 21st Century. Glob. Environ. Change 17, 420–428. doi: 10.1016/j.gloenvcha.2006.11.007
Nunes Carvalho, J. L., Raucci, G. S., Frazao, L. A., Cerri, C. E. P., Bernous, M., and Cerri, C. C. (2014). Crop-pasture rotation: a strategy to reduce soil greenhouse gas emissions in the Brazilian Cerrado. Agric. Ecosyst. Environ. 183, 167–175. doi: 10.1016/j.agee.2013.11.014
Oliveras, I., Girardin, C., Doughty, C. E., Cahuana, N., Arenas, C. E., Oliver, V., et al. (2014). Andean grasslands are as productive as tropical cloud forests. Environ. Res. Lett. 9:115011. doi: 10.1088/1748-9326/9/11/115011
Olson, D. M., and Dinerstein, E. (2002). The global 200: priority ecoregions for global conservation. Ann. Missouri Bot. Garden. 89, 199–224. doi: 10.2307/3298564
Ordoñez, J. A. B., de Jong, B. H. J., García-Oliva, F., Aviña, F. L., Pérez, J. V., Guerrero, G., et al. (2008). Carbon content in vegetation, litter, and soil under 10 different land-use and land-cover classes in the Central Highlands of Michoacan, Mexico. Forest Ecol. Manag. 255, 2074–2084. doi: 10.1016/j.foreco.2007.12.024
Parker, T. C., Subke, J., and Wookey, P. A. (2015). Rapid carbon turnover beneath shrub and tree vegetation is associated with low soil carbon stocks at a subartic treeline. Glob. Change Biol. 21, 2070–2081. doi: 10.1111/gcb.12793
Pearson, T. R. H., Brown, S. L., and Birdsey, R. A. (2007). Measurement Guidelines For The Sequestration Of Forest Carbon. General Technical Report. Northern Research Station, Delaware, 42. doi: 10.2737/NRS-GTR-18
Peña-Ramírez, V. M., Vázquez-Selem, L., and Siebe, C. (2009). Soil organic carbon stocks and forest productivity in volcanic ash soils of different age (1835–30,500 years B.P.) in Mexico. Geoderma 149, 224–234. doi: 10.1016/j.geoderma.2008.11.038
Pérez-Ramírez, S., Ramírez, M. I., Jaramillo-López, P. F., and Bautista, F. (2013). Contenido de carbono orgánico en el suelo bajo diferentes condiciones forestales: reserva de la biosfera mariposa monarca, México. Rev. Chapingo Ser. Cienc. For. Ambient 19, 157–173. doi: 10.5154/r.rchscfa.2012.06.042
Regil García, H. H., Maass, S. F., Ordoñez Díaz, J. A. B., Nava Bernal, G. E., and Mallén Rivera, C. (2014). Deforestation and tree density reduction of the Nevado de Toluca National Park. Rev. Mexicana Ciencias Forest. 5, 42–63. doi: 10.29298/rmcf.v5i23.341
Rzedowski, J. (1975). An ecological and phytogeographical analysis of the grasslands of Mexico. Taxon 24, 67–80. doi: 10.2307/1219002
Santini, N. S., Adame, M. F., Nolan, R. H., Miquelajauregui, Y., Piñero, D., Mastretta-Yanes, A., et al. (2019a). Storage of organic carbon in the soils of Mexican temperate forests. For. Ecol. Manag. 446, 115–125. doi: 10.1016/j.foreco.2019.05.029
Santini, N. S., Lovelock, C. E., Hua, Q., Zawadzki, A., Mazumder, D., Mercer, T. R., et al. (2019b). Natural and regenerated saltmarshes exhibit similar soil and belowground organic carbon stocks, root production and soil respiration. Ecosystems 22, 1803–1822. doi: 10.1007/s10021-019-00373-x
Schermer, M., Darnhofer, I., Daugstad, K., Gabillet, M., Lavorel, S., and Steinbacher, M. (2016). Institutional impacts on the resilience of mountain grasslands: an analysis based on three European case studies. Land Use Pol. 52, 382–391. doi: 10.1016/j.landusepol.2015.12.009
Smith, P., Powlson, D. S., Glendining, M. J., and Smith, J. U. (1998). Preliminary estimates of the potential for carbon mitigation in European soils through no-till farming. Glob. Change Biol. 4, 679–685. doi: 10.1046/j.1365-2486.1998.00185.x
Tashi, S., Singh, B., Keitel, C., and Adams, M. (2016). Soil carbon and nitrogen stocks in forests along an altitudinal gradient in the eastern Himalayas and a meta-analysis of global data. Glob. Change Biol. 22, 2255–2268. doi: 10.1111/gcb.13234
Toscana-Aparicio, A., and Granados-Ramírez, R. (2015). Recategorización del parque nacional nevado de Toluca. Política y Cultura. 44, 79–105.
United States Environmental Protection Agency (2020). Greenhouse Gas Equivalencies Calculator. Available online at: https://www.epa.gov/energy/greenhouse-gas-equivalencies-calculator
Valencia, S. A. (2004). Diversidad del género Quercus (Fagaceae) en México. Boletín Soc. Bot. México 75, 33–53. doi: 10.17129/botsci.1692
van der Werf, G. R., Morton, D. C., DeFries, R. S., Olivier, J. G. J., Kasibhatla, P. S., Jackson, R. B., et al. (2009). CO2 emissions from forest loss. Nat. Geosci. 2, 737–738. doi: 10.1038/ngeo671
Vilchis, M. I. (2006). Cartografía morfoedáfica escala 1:20,000; 7 estudios de caso en el volcán Nevado de Toluca, México. Thesis de Licenciatura. Facultad de Geografía, Universidad Autónoma del Estado de México, Mexico.
Ward, A., Dargusch, P., Thomas, S., Liu, Y., and Fulton, E. A. (2014). A global estimate of carbon stores in the world’s mountain grasslands and shrublands, and the implications for climate policy. Glob. Environ. Change 28, 14–24. doi: 10.1016/j.gloenvcha.2014.05.008
Wehenkel, C., Mariscal-Lucero, S., Jaramillo-Correa, J. P., López-Sánchez, C. A., Vargas-Hernández, J. J., and Sáenz-Romero, C. (2017). “Genetic diversity and conservation of Mexican Forest Trees,” in Biodiversity and Conservation of Woody Plants, Vol. 17, eds M. R. Ahuja and M. S. Jain (Cham: Springer). doi: 10.1007/978-3-319-66426-2_2
West, T., and Post, W. M. (2002). Soil organic carbon sequestration rates by tillage and crop rotation: a global data analysis. Soil Sci. Soc. Am. J. 66, 1930–1946. doi: 10.2136/sssaj2002.1930
World Bank., and Ecofys. (2018). State and Trends of Carbon Pricing 2018. Washington, DC: World Bank.
Works, M. A., and Hadley, K. S. (2004). The cultural context of forest degradation in adjacent Purépechan communities, Michoacán, México. Geogr. J. 170, 22–38. doi: 10.1111/j.0016-7398.2004.05024.x
Keywords: carbon credits, forest conservation, forest management, conifer forest, alpine grasslands, arable lands
Citation: Santini NS, Villarruel-Arroyo A, Adame MF, Lovelock CE, Nolan RH, Gálvez-Reyes N, González EJ, Olivares-Resendiz B, Mastretta-Yanes A and Piñero D (2020) Organic Carbon Stocks of Mexican Montane Habitats: Variation Among Vegetation Types and Land-Use. Front. Environ. Sci. 8:581476. doi: 10.3389/fenvs.2020.581476
Received: 08 July 2020; Accepted: 14 September 2020;
Published: 08 October 2020.
Edited by:
Hans-Peter Schmidt, Ithaka Institute, SwitzerlandReviewed by:
Mohammad Imam Hasan Reza, Independent Researcher, Chittagong, BangladeshCopyright © 2020 Santini, Villarruel-Arroyo, Adame, Lovelock, Nolan, Gálvez-Reyes, González, Olivares-Resendiz, Mastretta-Yanes and Piñero. This is an open-access article distributed under the terms of the Creative Commons Attribution License (CC BY). The use, distribution or reproduction in other forums is permitted, provided the original author(s) and the copyright owner(s) are credited and that the original publication in this journal is cited, in accordance with accepted academic practice. No use, distribution or reproduction is permitted which does not comply with these terms.
*Correspondence: Nadia S. Santini, bmFkaWEuc2FudGluaUBpZWNvbG9naWEudW5hbS5teA==; bmFkaWFzaWx2YW5hc2FudGluaUBnbWFpbC5jb20=
Disclaimer: All claims expressed in this article are solely those of the authors and do not necessarily represent those of their affiliated organizations, or those of the publisher, the editors and the reviewers. Any product that may be evaluated in this article or claim that may be made by its manufacturer is not guaranteed or endorsed by the publisher.
Research integrity at Frontiers
Learn more about the work of our research integrity team to safeguard the quality of each article we publish.