- 1Department for Ecology and Ecosystem Modeling, University of Potsdam, Potsdam, Germany
- 2Berlin-Brandenburg Institute of Advanced Biodiversity Research (BBIB), Berlin, Germany
In recent years, increasing concerns have been raised about the environmental risk of microplastics in freshwater ecosystems. Small microplastics enter the water either directly or accumulate through disintegration of larger plastic particles. These particles might then be ingested by filter-feeding zooplankton, such as rotifers. Particles released into the water may also interact with the biota through the formation of aggregates, which might alter the uptake by zooplankton. In this study, we tested for size-specific aggregation of polystyrene microspheres and their ingestion by a common freshwater rotifer Brachionus calyciflorus. The ingestion of three sizes of polystyrene microspheres (MS) 1-, 3-, and 6-μm was investigated. Each MS size was tested in combination with three different treatments: MS as the sole food intake, MS in association with food algae and MS aggregated with biogenic matter. After 72 h incubation in pre-filtered natural river water, the majority of the 1-μm spheres occurred as aggregates. The larger the particles, the higher the relative number of single particles and the larger the aggregates. All particles were ingested by the rotifer following a Type-II functional response. The presence of algae did not influence the ingestion of the MS for all three sizes. The biogenic aggregation of microspheres led to a significant size-dependent alteration in their ingestion. Rotifers ingested more microspheres (MS) when exposed to aggregated 1- and 3-μm MS as compared to single spheres, whereas fewer aggregated 6-μm spheres were ingested. This indicates that the small particles when aggregated were in an effective size range for Brachionus, while the aggregated larger spheres became too large to be efficiently ingested. These observations provide the first evidence of a size- and aggregation-dependent feeding interaction between microplastics and rotifers. Microplastics when aggregated with biogenic particles in a natural environment can rapidly change their size-dependent availability. The aggregation properties of microplastics should be taken into account when performing experiments mimicking the natural environment.
Introduction
Plastics have become a universal material due to their numerous properties. The mass production of plastics started in the 1950s at just one million tons per year. Nowadays, production has reached 335 million tons worldwide (Meng et al., 2020). Over 8 million tons of mostly single-use plastics enter the ocean each year (Jambeck et al., 2015), despite increasing recycling efforts and public awareness around the world. According to recent estimates, between 1.15 and 2.41 million tons of plastic are transported from rivers to the sea (Réu et al., 2019). In the past, research on the impact of plastic has focused on marine environments, with comparatively fewer studies conducted in freshwater habitats. Plastics are entering all ecosystems in all sizes, and large pieces disintegrate into smaller particles due to physical or chemical degradation. The resulting small particles below 5 mm are called secondary microplastics (Hartmann et al., 2019). In addition to these secondary microplastics, primary microplastics in the form of beads and pellets are manufactured to be used in personal care products and in industrial cleaning. Nanoplastics (size range from 1 to 1,000 nm) are frequently used in the cosmetics industry, though their use is decreasing (Strungaru et al., 2019). Particles from tire wear and shedding of microfibers from synthetic clothing also pollute aquatic ecosystems (Barboza et al., 2018). Unlike large plastic debris, microplastics are invisible to the naked eye and cannot be removed from the environment for recycling. The estimated number of microplastics smaller than 100 μm is still underestimated also in the marine environment (Lindeque et al., 2020).
Microplastic particles, particularly spheres, can be ingested by numerous zooplankton species (Kögel et al., 2020; Zheng et al., 2020). For example, rotifers, copepods, and cladocerans have the ability to ingest polystyrene microbeads ranging from 1.7 to 30.6 μm (Scherer et al., 2018). Typically, in these studies, microspheres are provided as defined and standardized food particles. Therefore, care is taken to avoid any clumps or aggregates and spheres are sonicated before use to assure that only singular particles are present. However, the plastic's surface serves as a physical substrate for microorganisms such as bacteria, fungi, algae and heterotrophic protists, which altogether form a complex biofilm. When the particles are in the size range of nanoparticles, they become enveloped within a biofilm (Martel et al., 2014; Ikuma et al., 2015; Summers et al., 2018). This conglomerate of plastics and biota is called “plastisphere” (Zettler et al., 2013; Kirstein et al., 2019; Amaral-Zettler et al., 2020). Moreover, extracellular polymeric substances (EPS), particularly transparent exopolymers particles (TEP), produced by microorganisms play a significant role in the formation of nano- and microplastic agglomerates (Cunha et al., 2019). These aggregated particles might be ingested by invertebrates having low feeding selectivity, such as filter-feeders (Scherer et al., 2017). The ingested plastic aggregates might then affect the fitness of the consumers (Vroom et al., 2017). Herbivorous rotifers are filter-feeding metazoans and their feeding behavior and low selectivity allows them to ingest small particles such as microplastics. In the freshwater food web, rotifers play a pivotal role in the energy transfer from primary producers to secondary consumers and potentially also transfer pollutants to higher trophic levels through ingestion and accumulation (Snell and Janssen, 1995). Several studies show that brachionid rotifers can ingest polystyrene microbeads with negative effects on their reproduction and growth rate (Juchelka and Snell, 1994; Baer et al., 2008; Jeong et al., 2016, 2018). Thus, these properties make rotifers very suitable animals for studying microplastics ingestion.
The aim of the present study was to investigate the ingestion of microplastics by the cosmopolitan freshwater planktonic rotifer Brachionus calyciflorus. We examined the ingestion of three sizes of polystyrene microspheres as single food particles, in association with a similar-sized food alga and as biogenic aggregated microspheres through bacteria and exopolymers particles. Moreover, we characterized the size of the aggregated microplastics and the number of singles MS.
We tested the following hypotheses: (i) the ingestion of microspheres is size-dependent and influenced by the presence of algal food, and (ii) the biogenic aggregation of microspheres influences their ingestion.
Materials and Methods
Polystyrene Microsphere
As microplastic particles, we used polystyrene microspheres (MS) of three different diameters: 1.03, 3.06, and 5.73 μm; for convenience, we refer to them as 1, 3 and 6 MS (Polysciences, Inc. Fluoresbrite® YG Polystyrene Microspheres, USA) (see Table 1). A stock solution was prepared with deionized MilliQ water under sterile conditions to minimize bacterial growth. To keep the MS as singular particles, each stock solution was sonicated for 30 min and was mixed using a vortexer.
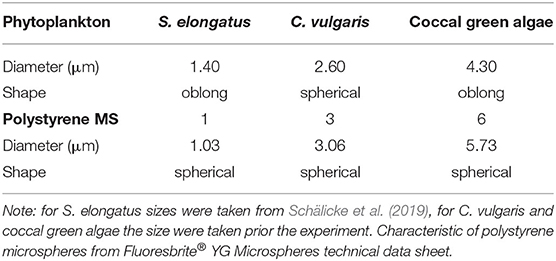
Table 1. Characteristics of the phytoplankton: Synechococcus elongatus, Chlorella vulgaris, and coccal green algae.
Organism
Stock cultures of all experimental organisms, algae and rotifers, were kept in glass flasks in a modified Woods Hole WC-medium (Guillard and Lorenzen, 1972) with regular substitution of the medium to sustain the continuous growth phase. Cultures were kept at 20°C in a light-dark cycle of 14:10 h and a light intensity of 35-μM photons s−1 m−2. We used the herbivorous monogononta rotifer Brachionus calyciflorus s.s. Pallas 1776 [strain IGB (Paraskevopoulou et al., 2018)] as a generalist consumer. Stock cultures were maintained with the food alga Monoraphidium minutum (SAG 243-1, culture collection of algae, Göttingen, Germany). During the three days prior to the experiment, the rotifers were fed daily with 1 × 106 cells ml−1 to assure constant food saturation conditions (Fussmann et al., 2005). For the treatments with additional food algae, we used the phytoplankton species Synechococcus elongatus (SAG 89.79), Chlorella vulgaris (SAG 211-11b), and an unidentified coccal green alga grown under the same conditions as M. minutum.
Aggregation Experiment
To study the effect of a natural bacteria community on potential aggregation of the MS, we incubated the polystyrene MS in a pre-filtered water sample. In May, 2019 we took a natural water sample (1l) from the river Havel in the urban area of Potsdam (Germany). The sample was pre-filtered with a 30-μm mesh and afterwards filtered through a glass fiber filter (Whatman®, GF/C), retaining most microplankton and allowing bacteria to pass through. In order to avoid any differences among the MS sizes the experiment with the natural water was conducted contemporarily and on the same day. After incubating the MS in 3 ml for 72 h in a rocking shaker, the degree of aggregation was quantified in two steps. The concentration used for the experiment are shown in Table 2 and they were the same used for the ingestion experiment. We first quantified the number of single particles (not aggregated) in a subsample for each concentration using a haemocytometer (Paul Marienfeld GmbH & Co., Germany) and a microscope (Zeiss Axioskop 2, Germany). Because we could not unambiguously determine the number of particles within one aggregate due to the formation of clumps, we filtered 1 ml subsample from each concentrations through 0.2-μm polycarbonate filters and stained them with DAPI (4′,6′-diamidino-2-phenylindole) and alcian blue. DAPI specifically binds to double-stranded DNA and polyphosphate (Zafiriou and Farrington, 1980), and with an aqueous solution of 0.02% alcian blue we could stain the polysaccharides contained in the transparent exopolymer particles (TEP) forming the aggregates. The stained polysaccharides were inspected microscopically on a glass slide, covered with immersion oil and a cover slip (Passow, 2002). From each sample, 30 pictures were randomly taken and the area of each aggregate was quantified using the open-source software ImageJ (Schneider et al., 2012). We quantified the aggregation for each particle size and concentration separately. We tested for differences in the frequency distribution (log2 scaled) among concentrations within each particle size using a test of homogeneity. We found no differences among the concentration in the 3 and 6 μm spheres (χ2 = 9.7 and 5.2, respectively; p > 0.6), but some differences within the 1 μm spheres with smaller aggregates at the lowest concentration (χ2 = 19.9, p = 0.035). We then pooled the data for each particle size and compared the median sizes among the different sizes. These were highly significantly different from each other (Kruskall-Wallis-test, p = 0.005).
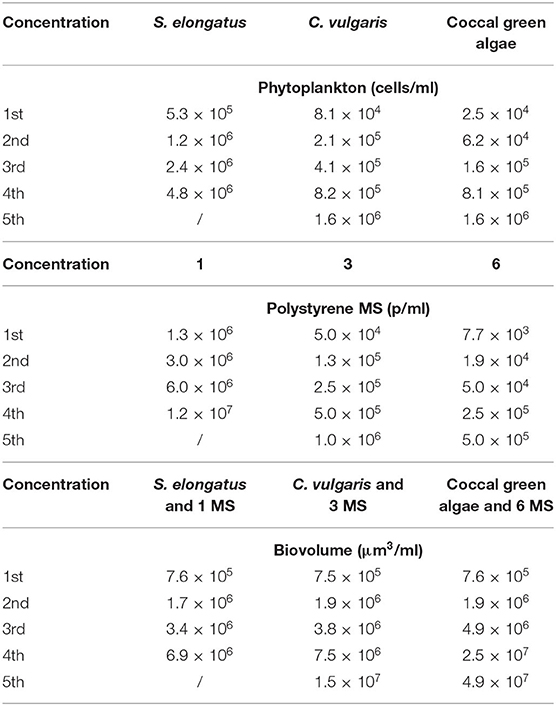
Table 2. Concentration and (bio) volume of phytoplankton (Synechococcus elongatus, Chlorella vulgaris, and coccal green algae) and polystyrene MS (1, 3, and 6 μm) used in the aggregation and ingestion experiments.
Ingestion Experiment
We measured the ingestion rate in three treatments: microspheres as the sole food source (MS), microspheres in association with algae (MS + algae) and microspheres incubated with pre-filtered, natural water in the presence of bacteria (MS aggregated) (Table 2). For all three treatments we used several particle concentrations (Table 2), and for each particle concentration we had three replicates. From these data, the functional response was calculated; see below. Seventy-five randomly chosen adult rotifers from the prepared cultures were transferred into 3-ml particle suspension on a UVA-transparent polystyrene 12-well microtiter plate (Greiner Bio-One). After 2 min of exposure in a rocking shaker, the rotifers were washed with the medium, narcotized with carbonated water and preserved in Lugol's solution. The exposure time of 2 min takes the short gut passage time of <10 min and the practicability of the quantification of the ingested MS into account. At high concentrations, the particles form clumps in the animals' gut and cannot be quantified. The maximum particle concentration was chosen in order to cover the full range of the functional response until saturation (Mohr and Adrian, 2000; Fussmann et al., 2005; Seifert et al., 2014).
The number of ingested MS per individual rotifer was quantified using an epifluorescent microscope (Zeiss Axioskop 2, Germany). Between 30 and 36 rotifers per sample were transferred to a microscope slide and carefully squeezed under a coverslip until the MS were compressed into a single layer (Baer et al., 2008). The MS found in the gut and in the trophi of each rotifer were counted either directly or, in some cases, pictures of the gut were taken and the MS number was quantified with ImageJ. All experiments were run in triplicate. To study the effect of algae as an accompanying food source, algae of similar size were added for each size of MS. We used an additive design so that the addition of algae doubled the total volume of MS particles per treatment (Table 2). Prior to the addition, the cell number from stock cultures was quantified with a haemocytomer (Paul Marienfeld GmbH & Co., Germany) and the addition was adjusted to equalize the volume of the respective MS.
Statistical Analysis
The resource-dependent consumption of food items by a consumer can be described as functional response curves. The type I functional response has a linear relationship between ingestion and resource concentration until a saturation is reached and ingestion remains constant with increasing resource concentration. The type II functional response exhibits a saturation function where ingestion approaches asymptotically its maximum. The type III functional response has a sigmoidal shape with very low ingestion at low resource concentration. To fit and compare the consumer functional response, we followed the procedure of Pritchard et al. (2017). Type II and III can be characterized by:
N0 is the initial resource concentration; T is the experimental time; a is the instantaneous resource attack rate of the consumer; h represents the time spent subjugating, ingesting and digesting the resource and q is the scaling exponent. Type II and III functional responses differ in their value for q: When q = 0 a type II functional response prevails, when q > 0 the sigmoidal type III prevails. The number of MS ingested during the experimental period of 2 min was expressed as MS ingested per hour.
For fitting the functional response, we used the r-package FRAIR v0.5.100 (Pritchard et al., 2017). Three steps were involved: (i) model selection, (ii) model fitting, and (iii) comparison of fit and coefficients. The model selection step was used to distinguish between Type-II (saturation function) and Type-III (sigmoidal) functional response. When the evidence for a Type-II response was positive, we fitted a linear functional response Type I and a functional response Type II and compared the models using the Akaike information criterion (AIC). After providing starting estimates and fixed values of the parameters, the model was optimized using maximum likelihood estimation (MLE) with the bbml package and the function ml2 (Bolker, 2008). The last step included comparisons of the fitted coefficients through two approaches: the delta or difference method of Juliano (2001), and non-parametric bootstrapping of the raw data. We compared the fitted coefficients for each MS size separately. The comparison of the fitted coefficients with the delta or difference methods of Juliano (2001) yielded the difference between two fitted coefficients. The functional responses were plotted with empirical approximations of 95% confidence intervals (CI) based on the bootstrapped model fits for the number of MS ingested per rotifer. The lack of overlap between the CIs of the model parameters was considered equivalent to a null hypothesis test (Pritchard et al., 2017). All statistical analyses were carried out using R 3.4.3 (R Core Team, 2017).
Results
Aggregation Experiment
The incubation for 72 h with the natural bacteria community led to significant formations of aggregates that were size-specific. The percentage of single particles was the lowest in the smallest size: 1-μm MS 16 ± 1% (mean ± SE), and increased with the biggest sizes: 42 ± 3% (mean ± SE) for the 3-μm MS and 67 ± 8% (mean ± SE) for the 6-μm (Figure 1A). The vast majority of the 1-μm MS occurred in aggregates, whereas the majority of the 6-μm MS were single particles. The area of the aggregates also showed a size-specific pattern. The smaller the MS, the smaller the area of the aggregates: for the 1-μm, the area was 64 ± 16 μm2 (mean ± SE), for the 3-μm 133 ± 18 μm2 (mean ± SE), and 245 ± 33 μm2 (mean ± SE) for the 6-μm spheres (Figure 1B). Staining with DAPI revealed the presence of bacteria in the size range of 0.5–2.0 μm (Figures 2A,B) and the alcian blue revealed that transparent exopolymer particles were involved in the formation of the aggregate (Figures 2C–E).
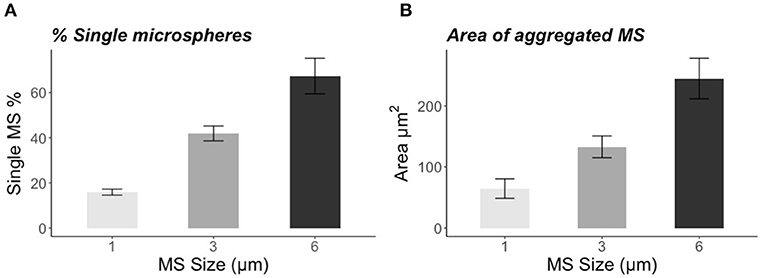
Figure 1. (A) Percentage of single microspheres (1, 3, and 6 μm) found in the sample after 72 h of incubation with natural water and bacteria. (B) Area (μm2) of aggregated microspheres (1, 3, and 6 μm) measured with ImageJ. The error bars represent SE.
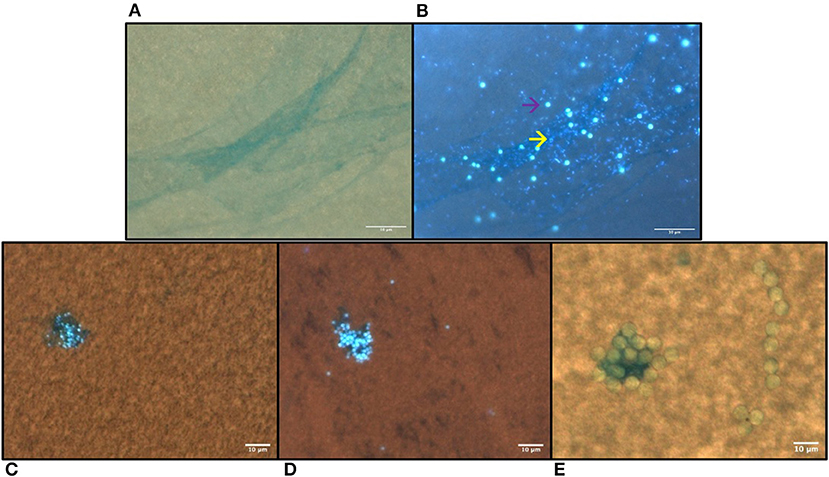
Figure 2. Microplastic aggregation formed after 72 h of incubation in prefiltered natural freshwater stained with alcian blue and DAPI. (A) Transparent exopolymer particles (TEP) stained with alcian blue and DAPI in 1 μm MS sample visualized under bright light and (B) under UV-light, the yellow arrow indicates the bacteria and the purple arrow indicates the MS; (C) 1 μm, (D) 3 μm MS aggregation visualized with merged UV-light and bright light (E) 6 μm. The microspheres are visible also under the UV light (B–D). The scale bar is 10 μm.
Functional Response
Ingestion Rate
Brachionus calyciflorus fed on all three tested sizes of polystyrene MS (Figures 3A,B), in all combinations: microplastics alone, microplastics in association with food algae and microplastics incubated with bacteria in natural water. For each size and treatment, the number of MS ingested by the rotifers increased with the increasing concentration of MS in suspension, reaching a plateau. The comparison with the different types of functional responses, based on the AIC (Table 3), revealed a Type-II functional response (Figures 4A–C). Subsequently, all functional response curves were fit by the Type-II functional response and the handling time and attack rate were calculated (Table 4).
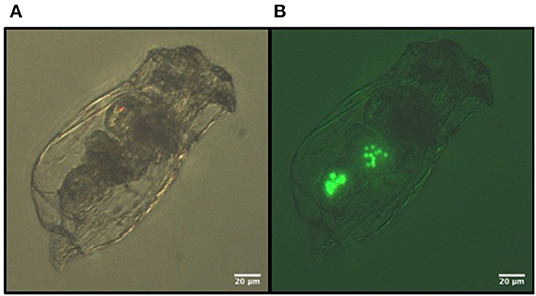
Figure 3. Images of the 3 μm fluorescent polystyrene MS ingested by B. calyciflorus: (A) bright light, (B) fluorescent light.
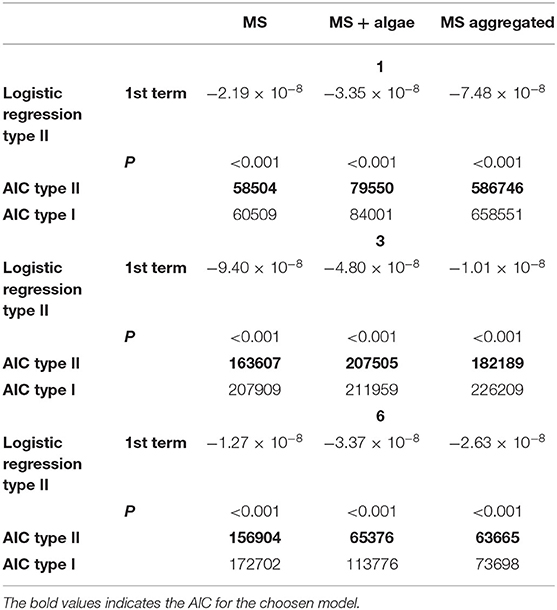
Table 3. The results of logistic regressions for the selection of type II are shown, together with the Akaike information criterion (AIC) values for fitted type II (see Equation 1 in the text) and type I functional response models for three sizes of MS (1, 3, and 6) and the treatments (MS, MS + algae, and MS aggregated).
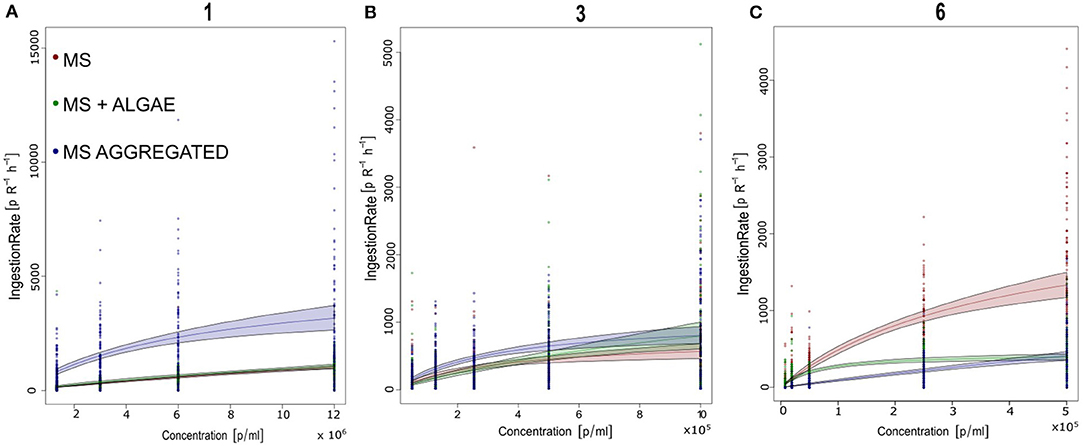
Figure 4. Number of polystyrene microspheres ingested by Brachionus calyciflorus (IGB) within 1h at offered microsphere densities of 103-107 particles / ml. Data from all 3 replicates are shown. The curves represent the best-fitting functional response model (Rogers Type II functional response). Parameter estimated for each treatment and size are given in Tables 3, 4. (A) 1 μm; (B) 3 μm; and (C) 6 μm.
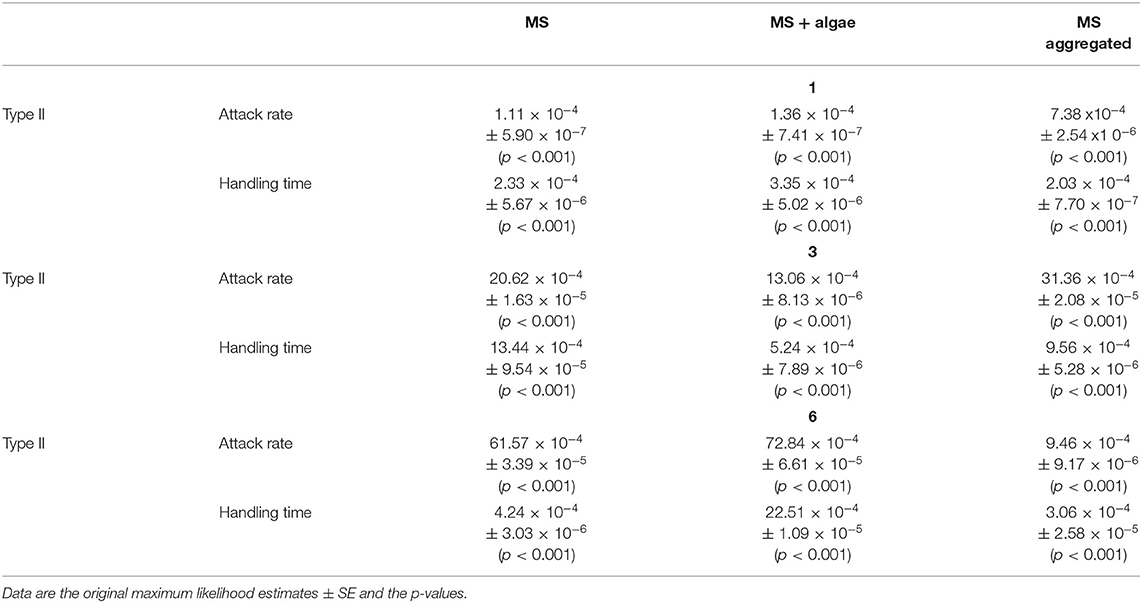
Table 4. Parameter estimates from type II (see Equation 1 in the text) functional responses for three sizes of MS (1, 3, and 6) and the treatments (MS, MS + algae, MS aggregated).
With regard to the 1- and 3-μm MS, B. calyciflorus showed the highest ingestion of MS when these were aggregated with biogenic particles though this was less pronounced for the 3-μm MS (Table 5). We found lower ingestion when the MS were provided as the sole food source and in association with algae. The opposite pattern was found for the 6-μm MS, where the ingestion was higher when they were provided as the sole food source and lower when aggregated with biogenic particles (Table 5). The maximum number of ingested 1-μm MS occurred at the highest concentration of MS aggregated with biogenic particles, with 3155 MS h−1. The 3-μm MS were ingested at a rate of 895 MS h−1 and the maximum ingestion occurred at the highest concentration of MS aggregated with biogenic particles. The maximum ingestion of 1483 MS h−1 occurred with the 6-μm MS at the highest concentration as single particles (Table 5).
Attack Rate and Handling Time
The differences in the ingestion rates are reflected in the attack rate and the handling time: For the 1-μm MS, the attack rate of the aggregated particles increased by a factor of 6.65 compared to the singular particles (Table 4). This effect leveled off for the 3-μm MS with a factor of 1.57 and reversed for the 6-μm MS where the attack rate was 9 times lower for the aggregated MS (Table 4). The handling time differed much less between these two treatments.
Discussion
The scope of this study was to investigate the ingestion of microplastics in the rotifer B. calyciflorus mediated by biogenic aggregation and in the presence of food algae. The three sizes of polystyrene MS (1, 3, and 6 μm) were ingested in all treatments. Our study has shown that the ingestion of the three sizes as the sole food, in association with algae or aggregated with biogenic particles followed the Holling's type II model and the ingestion can be influenced by the aggregation of MS.
Biogenic Aggregation of Microspheres
We found aggregations of the polystyrene microspheres (MS) in each concentration, after an incubation of 72 h in prefiltered natural freshwater. Staining these aggregates with DAPI and alcian blue revealed that they contained a community of bacteria and transparent exopolymer particles (TEP). The exopolymers are contained in the TEP encapsulated and trapped the microplastic particles to form an amorphous matrix. The presence and persistence of microplastics has been shown to enhance the agglomeration of particulate matter and micro-algal cells (Kettner et al., 2019). Several studies have shown that bacteria and glycoproteins contribute to the formation of plastic aggregates. This confirms the high aggregation potential of microplastics to rapidly coagulate with biogenic particles, forming pronounced aggregates within a few days (Michels et al., 2018; Summers et al., 2018). Here, we provide further evidence on how fast the formation of aggregates can take place when the pristine plastic MS were exposed to prefiltered natural freshwater. The percentage of aggregated MS after 72 h of incubation with prefiltered natural water was size-specific: The smaller the particles, the higher the number of aggregated particles. In detail, the percentage of single particles was below 20% for the 1-μm spheres and the aggregates were smaller than those from larger spheres. This finding indicates that the small particles were easily trapped by the TEPs, although larger aggregates are not formed. On the contrary, a higher percentage of single MS 3 (>40%) and 6 μm (>60%) was found, but the aggregated MS were larger. This indicates that the larger particles were less efficiently captured by TEPs, but once they were caught, the aggregates grow larger. We did not find differences in the aggregation pattern within the different sphere sizes at different concentrations (except for a slightly higher share of small aggregates in the 1 μm MS at the lowest concentration); however, it should be taken into account that the absolute numbers of the tested particles differed among sizes because of size-specific differences in functional response curves (see Table 1).
The process of aggregation might change over the season due to different numbers and composition of bacteria, temperature and water chemistry, however, we believe that the process itself and the resulting pattern does not change much. In general, the aggregation of detritus and living organisms (bacteria, algae, fungi, and microzooplankton) is a common phenomenon in lakes and oceans, known as lake or marine snow (Grossart and Simon, 1993; Silver, 2015) and a substantial incorporation of plastics into these aggregates seems very likely. Another process that alters the properties of MP in the environment is due to aging and the association with colloids which modifies the particles' surface (Alimi et al., 2018). Thus, the environmental conditions together with the specific properties of the particles affects their aggregation behavior in aquatic environments (Wang et al., 2021).
It is still a matter of debate whether the microbial community associated with plastic is specific to that kind of substrate or to an unspecific community from the surrounding water (Amaral-Zettler et al., 2020). Either way, aggregate formation alters the properties of the plastic, leading to higher sedimentation, or altered ingestion by consumers (Besseling et al., 2017; Alimi et al., 2018; Summers et al., 2018).
Ingestion of Microplastics Particles as the Sole Food Source
The ingestion of the MS (1, 3, and 6 μm), even if considered below the optimal size of feeding efficiency (Rothhaupt, 1990a), showed a Type-II functional response model. Previous studies demonstrated that the highest feeding efficiency for B. calyciflorus and closely related Brachionus species is in the range of a 3.5- to ~10-μm equivalent spherical diameter (ESD) (Vadstein, 1993; Baer et al., 2008) or even higher (Pagano, 2008). We found a Type-II functional response for the 1-μm MS, considered in the similar size range of (large) bacteria or small algae. In general, very small particles were ingested with lower efficiency, but the presence of aggregated small particles can increase the ingestion efficiency. As in Rothhaupt (1990b), the larger MS (6 μm) are preferably ingested in terms of biovolume than the smaller MS (1 and 3 μm), as the attack rate is higher. Comparing the ingestion rate of 3-μm MS as a sole food source from our study with the ingestion of the similar-sized food alga Monoraphidium minutum, we found a similar maximum ingestion rate as in Fussmann et al. (2005), using the same algal and rotifer strains but applying the radioisotope method. The highest ingestion based on biovolume was found for the 6-μm MS. The volume of one 6-μm MS is eight times larger than one 3 μm in size, and 216 times larger than a 1-μm MS. Thus, the total microplastics uptake of 1-μm MS was lower than for the larger-sized MS. However, toxicity does not necessarily correlate with the total amount of ingested plastic. Mueller et al. (2020), found for freshwater nematodes that the toxicity increased with the surface area-to-volume ratio of the applied microspheres. The absolute concentration of microplastics in this study were quite high. However, for this size fraction of 1–6 μm, no reliable data on the distribution and abundance in the field are available and it is suggested that with increasing fragmentation of larger particles the concentration of such small particles strongly increases. Most field studies about microplastics are limited by the sampling methodology and the respective detection limit of the devices that were used (Besseling et al., 2019). A commonly used lower limit of mesh size lies between 300 and 800 μm. Applying smaller mesh sizes would retain a broader fraction of MPs (Wiggin and Holland, 2019) but would be more difficult to handle. A huge amount of microplastics enters the environment via the discharge from waste water treatment plants. Whereas particles larger than 10 μm are relatively efficiently removed, smaller particles likely enter the environment in higher rates (Chen et al., 2018). Once these particles are released to the environment, they might accumulate through sedimentation as aggregates in regions with low flow velocity, for example in reservoirs. Resuspension of plastics from the sediment might then makes it available again for the biota (Besseling et al., 2019).
Ingestion of MS Together With Food Algae
Rotifers are often regarded as unselective filter-feeders; however, some degree of selectivity has been found (Starkweather, 1980). In experiments with flavored polystyrene spheres (Demott, 1986), it was found that B. calyciflorus fed preferentially on 12-μm spheres, but did not discriminate against those with adsorbed algal flavors (Snell, 1998). Similarly, large daphnids exhibit no taste discrimination for small beads and smaller daphnids show some degree of taste and acute size discrimination. Contrarily, calanoid copepods can evaluate the resource quality in small and large particles and discriminate accordingly (Scherer et al., 2017). Thus, non-discriminating filter feeders are expected to take up more MP than raptorial feeders which might lead to reduced food intake and population growth and supports selective feeders (Setälä et al., 2016). In our experiment, we added the algae to the tested MS concentrations, leading to an increase in the total number of available particles. Thus, with algae added to low microplastics concentrations when the functional response curve is nearly linear, the uptake of the spheres is not necessarily reduced. Only at high particle concentrations, is the uptake of plastic particles expected to decrease due to algal additions.
Ingestion of MS Aggregated With Biogenic Particles
The biogenic formation of aggregates within 72 h specifically altered the ingestion of smaller and larger microplastics particles.
It is known from previous studies that the diet of B. calyciflorus includes not only algae but also bacteria to some extent. Bacteria may be utilized as food (Raatz et al., 2018) and, moreover, it seems likely that Brachionus can also ingest larger detrital particles and bacterial aggregates, deriving nutritional benefit from those cells as well (Starkweather et al., 1979).
The difference between the parameters' estimation when the MS are associated with biogenic particles shows us that the ingestion of MS is influenced by their size. Despite the size selectivity of the rotifers B. calyciflorus, the presence of aggregated MS increases the feeding efficiency of particles considered below the optimal size, such as 1 and 3 μm, by making them more available when aggregated; ingestion of otherwise edible MS (6 μm) can be prevented through aggregation. These results are reflected in the differences in the calculated attack rates between the experiments with and without aggregation. When the MS were ingested, it was not possible to recognize whether they were ingested as singular or aggregated particles. Nevertheless, the reduction in the attack rate of the 6-μm MS when aggregates were present indicates that the large particles were inedible and interfered with the ingestion of the well-edible singular MS. The high attack rate for the aggregated 1-μm MS indicates that the aggregates were of a well-edible size. However, technically, the effective number of particles was lower and the attack on one aggregate represents the attack on all MS within this aggregate. Zhao et al. (2018) found that the aggregation of small MP (0.5–1 μm) and nanoplastics (30 and 100 nm) facilitated the uptake from mussels. Besides the increase in particle size through aggregation, changes in surface topography and density were found. Once incorporated into aggregates, several transformations can occur, including an increase in the effective particle size and change in surface topography and density as a result of the physical and biological processes in the aggregate microcosm (Zhao et al., 2018).
Once a food item was captured, the calculated handling time varied only little among these two treatments. Thus, the similar handling times of singular MS and aggregated MS indicates that the handling time increases proportionately to the number of MS within the aggregate. This means that the “effective” handling time of an aggregate with 10 MS can be 10 times longer than the handling time of one singular MS in order to end up at the same calculated handling time. Overall, the ingestion and the effect on life history and survival can differ depending on whether microplastics are provided in a pristine state or aged and/or in natural water.
We did not test for toxicity; however, the toxicity of polystyrene nanoplastics to the rotifer Brachionus plicatilis was lower when the particles were provided in natural sea water compared to reconstituted sea water (Manfra et al., 2017). A potential reason for that was the interplay between surface charge, aggregation and salt. In a study on marine copepods, the aging of plastics promoted their uptake by marine zooplankton (Vroom et al., 2017). Even the type of plastic on which a natural biofilm developed influenced the food quality of the biofilm for a freshwater snail (Vosshage et al., 2018). These results underline the importance of experiments under near-natural conditions to better estimate the effect of microplastics on the biota and to complement standardized toxicological tests.
Conclusion
Our results demonstrate that the aggregation of MS accelerates the ingestion of smaller MS particles and prevents the ingestion of the largest ones in the freshwater rotifer B. calyciflorus. The aggregation potential of microplastics has to be considered in order to recreate the environmental interaction between microplastics and aquatic organisms. The aggregation processes, together with degradation processes, are the cause of physical and chemical alteration of pristine microplastics. These two processes might alter the response of the aquatic organism to microplastics in laboratory and natural environments. In particular, non-selective filter feeders such as crustaceans and rotifers that feed mainly size-specific (Burns, 1968; Geller, 1981; Bern, 1990; Brendelberger, 1991; Baer et al., 2008; Scherer et al., 2017) are affected by aggregation processes. Consequently, the variation in the MS size range might lead to an increased interaction between the smallest particles and aquatic consumers. To test for the response of aquatic organisms to microplastics, the increased or decreased ingestion of microplastics is fundamental to take into account. In order to fill this gap, further studies are needed on the direct and indirect effects of aggregated microplastics on the life cycles of aquatic consumers.
Data Availability Statement
The raw data supporting the conclusions of this article will be made available by the authors, without undue reservation.
Author Contributions
CD, JP, and GW designed the experiment. CD conducted the experiment and performed the analysis of the data. CD and GW wrote the manuscript. All authors discussed the results and provided extensive comments on the manuscript concerning the analysis, interpretation, and writing. All authors approved the final version and have accepted accountability for all aspects of the work.
Funding
This research was supported by the BMBF project MikroPlaTaS (02WPL1448C).
Conflict of Interest
The authors declare that the research was conducted in the absence of any commercial or financial relationships that could be construed as a potential conflict of interest.
Acknowledgments
We thank S. Saumweber and C. Schirmer for providing us with samples and technical support and Dr. P. Colangeli and Dr. T. Klauschies for their valuable advice on the experimental design. We also thank the reviewers for their comments that improved the manuscript.
Supplementary Material
The Supplementary Material for this article can be found online at: https://www.frontiersin.org/articles/10.3389/fenvs.2020.574274/full#supplementary-material
References
Alimi, O. S., Farner Budarz, J., Hernandez, L. M., and Tufenkji, N. (2018). Microplastics and nanoplastics in aquatic environments: aggregation, deposition, and enhanced contaminant transport. Environ. Sci. Technol. 52, 1704–1724. doi: 10.1021/acs.est.7b05559
Amaral-Zettler, L. A., Zettler, E. R., and Mincer, T. J. (2020). Ecology of the plastisphere. Nat. Rev. Microbiol. 18, 139–151. doi: 10.1038/s41579-019-0308-0
Baer, A., Langdon, C., Mills, S., Schulz, C., and Hamre, K. (2008). Particle size preference, gut filling and evacuation rates of the rotifer Brachionus “Cayman” using polystyrene latex beads. Aquaculture 282, 75–82. doi: 10.1016/j.aquaculture.2008.06.020
Barboza, L. G. A., Dick Vethaak, A., Lavorante, B. R. B. O., Lundebye, A. K., and Guilhermino, L. (2018). Marine microplastic debris: an emerging issue for food security, food safety and human health. Mar. Pollut. Bull. 133, 336–348. doi: 10.1016/j.marpolbul.2018.05.047
Bern, L. (1990). Size-related discrimination of nutritive and inert particles by freshwater zooplankton. J. Plankton Res. 12, 1059–1067. doi: 10.1093/plankt/12.5.1059
Besseling, E., Quik, J. T. K., Sun, M., and Koelmans, A. A. (2017). Fate of nano- and microplastic in freshwater systems: a modeling study. Environ. Pollut. 220, 540–548. doi: 10.1016/j.envpol.2016.10.001
Besseling, E., Redondo-Hasselerharm, P., Foekema, E. M., and Koelmans, A. A. (2019). Quantifying ecological risks of aquatic micro- and nanoplastic. Crit. Rev. Environ. Sci. Technol. 49, 32–80. doi: 10.1080/10643389.2018.1531688
Brendelberger, H. (1991). Filter mesh size of cladocerans predicts retention efficiency for bacteria. Limnol. Oceanogr. 36, 884–894. doi: 10.4319/lo.1991.36.5.0884
Burns, W. (1968). Particle size and sedimentation in the feeding behavior of two species of Daphnia. Limnol. Oceanogr. 14, 392–402. doi: 10.4319/lo.1969.14.3.0392
Chen, J. P., Li, J., Liu, H., and Chen, J. P. (2018). Microplastics in freshwater systems: a review on occurrence, environmental effects, and methods for microplastics detection. Water Res. 137, 362–374. doi: 10.1016/j.watres.2017.12.056
Cunha, C., Faria, M., Nogueira, N., Ferreira, A., and Cordeiro, N. (2019). Marine vs freshwater microalgae exopolymers as biosolutions to microplastics pollution. Environ. Pollut. 249, 372–380. doi: 10.1016/j.envpol.2019.03.046
Demott, W. R. (1986). The role of taste in food selection by freshwater zooplankton. Oecologia 69, 334–340. doi: 10.1007/BF00377053
Fussmann, G. F., Weithoff, G., and Yoshida, T. (2005). A direct, experimental test of resource vs. consumer dependence. Ecology 86, 2924–2930. doi: 10.1890/04-1107
Geller, W. (1981). The filtration apparatus of cladocera: filter mesh-sizes and their implications on food selectivity. Oecologia 49, 316–321. doi: 10.1007/BF00347591
Grossart, H.-P., and Simon, M. (1993). Limnetic macroscopic organic aggregates (lake snow): occurrence, characteristics, and microbial dynamics in Lake Constance. Limnol. Oceanogr. 38, 532–546. doi: 10.4319/lo.1993.38.3.0532
Guillard, R. R. L., and Lorenzen, C. J. (1972). Yellow-green algae with chlorophyllide c. J. Phycol. 8, 10–14. doi: 10.1111/j.1529-8817.1972.tb03995.x
Hartmann, N. B., Hu, T., Thompson, R. C., Hassello, M, Verschoor, A., Daugaard, A. E., et al. (2019). Are we speaking the same language? Recommendation for a definition and categorization framework for plastic debris. Environ. Sci. Technol. 53, 1039–1047. doi: 10.1021/acs.est.9b02238
Ikuma, K., Decho, A. W., and Lau, B. L. T. (2015). When nanoparticles meet biofilms- interactions guiding the environmental fate and accumulation of nanoparticles. Front. Microbiol. 6:591. doi: 10.3389/fmicb.2015.00591
Jambeck, J. R., Geyer, R., Wilcox, C., Siegler, T. R., Perryman, M., Andrady, A., et al. (2015). Plastic waste inputs from land into the ocean. Science 347, 768LP−771. doi: 10.1126/science.1260352
Jeong, C. B., Kang, H. M., Lee, Y. H., Kim, M. S., Lee, J. S., Seo, J. S., et al. (2018). Nanoplastic ingestion enhances toxicity of persistent organic pollutants (pops) in the monogonont rotifer Brachionus koreanus via multixenobiotic resistance (MXR) disruption. Environ. Sci. Technol. 52, 11411–11418. doi: 10.1021/acs.est.8b03211
Jeong, C. B., Won, E. J., Kang, H. M., Lee, M. C., Hwang, D. S., Hwang, U. K., et al. (2016). Microplastic size-dependent toxicity, oxidative stress induction, and p-JNK and p-p38 Activation in the monogonont rotifer (Brachionus koreanus). Environ. Sci. Technol. 50, 8849–8857. doi: 10.1021/acs.est.6b01441
Juchelka, C. M., and Snell, T. W. (1994). Rapid toxicity assessment using rotifer ingestion rate. Arch. Environ. Contam. Toxicol. 26, 549–554. doi: 10.1007/BF00214160
Juliano, S. A. (2001). “Nonlinear curve fitting,” in Design and Analysis of Ecological Experiments, eds S. M. Scheiner and J. Gurevitch (Oxford: Oxford University Press), 178–196.
Kettner, M. T., Oberbeckmann, S., Labrenz, M., and Grossart, H. P. (2019). The eukaryotic life on microplastics in brackish ecosystems. Front. Microbiol. 10:538. doi: 10.3389/fmicb.2019.00538
Kirstein, I. V., Wichels, A., Gullans, E., Krohne, G., and Gerdts, G. (2019). The plastisphere – Uncovering tightly attached plastic “specific” microorganisms. PLoS ONE 14:e0215859. doi: 10.1371/journal.pone.0215859
Kögel, T., Bjorøy, Ø., Toto, B., Bienfait, A. M., and Sanden, M. (2020). Micro- and nanoplastic toxicity on aquatic life: determining factors. Sci. Total Environ. 709:136050. doi: 10.1016/j.scitotenv.2019.136050
Lindeque, P. K., Cole, M., Coppock, R. L., Lewis, C. N., Miller, R. Z., Watts, A. J. R., et al. (2020). Are we underestimating microplastic abundance in the marine environment? A comparison of microplastic capture with nets of different mesh-size. Environ. Pollut. 265:114721. doi: 10.1016/j.envpol.2020.114721
Manfra, L., Rotini, A., Bergami, E., Grassi, G., Faleri, C., and Corsi, I. (2017). Comparative ecotoxicity of polystyrene nanoparticles in natural seawater and reconstituted seawater using the rotifer Brachionus plicatilis. Ecotoxicol. Environ. Saf. 145, 557–563. doi: 10.1016/j.ecoenv.2017.07.068
Martel, J., Peng, H.-H., Young, D., Wu, C.-Y., and Young, J. D. (2014). Of nanobacteria, nanoparticles, biofilms and their role in health and disease: facts, fancy and future. Nanomedicine 9, 483–499. doi: 10.2217/nnm.13.221
Meng, Y., Kelly, F. J., and Wright, S. L. (2020). Advances and challenges of microplastic pollution in freshwater ecosystems: a UK perspective. Environ. Pollut. 256:113445. doi: 10.1016/j.envpol.2019.113445
Michels, J., Stippkugel, A., Lenz, M., Wirtz, K., and Engel, A. (2018). Rapid aggregation of biofilm-covered microplastics with marine biogenic particles Proc. R. Soc. B. 285:20181203. doi: 10.1098/rspb.2018.1203
Mohr, S., and Adrian, R. (2000). Functional responses of the rotifers Brachionus calyciflorus and Brachionus rubens feeding on armored and unarmored ciliates. Limnol. Oceanogr. 45, 1175–1179. doi: 10.4319/lo.2000.45.5.1175
Mueller, M., Fueser, H., Trac, L. N., Mayer, P., Traunspurger, W., and Ho, S. (2020). Surface-related toxicity of polystyrene beads to nematodes and the role of food availability. Environ. Sci. Technol. 54, 1790–1798. doi: 10.1021/acs.est.9b06583
Pagano, M. (2008). Feeding of tropical cladocerans (Moina micrura, Diaphanosoma excisum) and rotifer (Brachionus calyciflorus) on natural phytoplankton : effect of phytoplankton size – structure. J. Plankton Res. 30, 401–14. doi: 10.1093/plankt/fbn014
Paraskevopoulou, S., Tiedemann, R., and Weithoff, G. (2018). Differential response to heat stress among evolutionary lineages of an aquatic invertebrate species complex. Biol. Lett. 14:20180498. doi: 10.1098/rsbl.2018.0498
Passow, U. (2002). Transparent exopolymer particles (TEP) in aquatic environments. Prog. Oceanogr. 55, 287–333. doi: 10.1016/S0079-6611(02)00138-6
Pritchard, D. W., Paterson, R. A., Bovy, H. C., and Barrios-O'Neill, D. (2017). frair: an R package for fitting and comparing consumer functional responses. Methods Ecol. Evol. 8, 1528–1534. doi: 10.1111/2041-210X.12784
R Core Team (2017). R: A Language and Environment for Statistical Computing. Vienna: R Foundation for Statistical Computing. Available online at: https://www.r-project.org/
Raatz, M., Schälicke, S., Sieber, M., Wacker, A., and Gaedke, U. (2018). One man's trash is another man's treasure—the effect of bacteria on phytoplankton–zooplankton interactions in chemostat systems. Limnol. Oceanogr. Methods 16, 629–639. doi: 10.1002/lom3.10269
Réu, P., Svedberg, G., Hässler, L., Möller, B., Svahn, H. A., and Gantelius, J. (2019). A 61% lighter cell culture dish to reduce plastic waste. PLoS ONE 14:e0216251. doi: 10.1371/journal.pone.0216251
Rothhaupt, K. O. (1990a). Differences in particle size-dependent feeding efficiencies of closely related rotifer species. Limnol. Oceanogr. 35, 16–23. doi: 10.4319/lo.1990.35.1.0016
Rothhaupt, K. O. (1990b). Population growth rates of two closely related rotifer species: effects of food quantity, particle size, and nutritional quality. Freshw. Biol. 23, 561–570. doi: 10.1111/j.1365-2427.1990.tb00295.x
Schälicke, S., Teubner, J., Martin-creuzburg, D., and Wacker, A. (2019). Fitness response variation within and among consumer species can be co-mediated by food quantity and biochemical quality. Sci. Rep. 9:16126. doi: 10.1038/s41598-019-52538-2
Scherer, C., Brennholt, N., Reifferscheid, G., and Wagner, M. (2017). Feeding type and development drive the ingestion of microplastics by freshwater invertebrates. Sci. Rep. 7:17006. doi: 10.1038/s41598-017-17191-7
Scherer, C., Weber, A., Lambert, S., and Wagner, M. (2018). “Interactions of microplastics with freshwater biota,” in Freshwater Microplastics. The Handbook of Environmental Chemistry, eds M. Wagner and S. Lambert, Vol. 58 (Cham: Springer), 153–180. doi: 10.1007/978-3-319-61615-5_8
Schneider, C. A., Rasband, W. S., and Eliceiri, K. W. (2012). NIH image to imagej: 25 years of image analysis. Nat. Methods 9, 671–675. doi: 10.1038/nmeth.2089
Seifert, L. I., de Castro, F., Marquart, A., Gaedke, U., Weithoff, G., and Vos, M. (2014). Heated relations: temperature-mediated shifts in consumption across trophic levels. PLoS ONE 9:e95046. doi: 10.1371/journal.pone.0095046
Setälä, O., Norkko, J., and Lehtiniemi, M. (2016). Feeding type affects microplastic ingestion in a coastal invertebrate community. Mar. Pollut. Bull. 102, 95–101. doi: 10.1016/j.marpolbul.2015.11.053
Silver, M. (2015). Marine snow: a brief historical sketch. Limnol. Oceanogr. Bull. 24, 5–10. doi: 10.1002/lob.10005
Snell, T. W. (1998). “Review paper: chemical ecology of rotifers,” in Rotifera VIII: A Comparative Approach. Developments in Hydrobiology, Vol. 134, eds E. Wurdak, R. Wallace, and H. Segers (Dordrecht: Springer), 267–276. doi: 10.1007/978-94-011-4782-8_34
Snell, T. W., and Janssen, C. R. (1995). Rotifers in ecotoxicology : a review. Hydrobiologia 313, 231–247. doi: 10.1007/978-94-009-1583-1_32
Starkweather, P. L. (1980). Aspects of the feeding behavior and trophic ecology of suspension-feeding rotifers. Hydrobiologia 73, 63–72. doi: 10.1007/BF00019427
Starkweather, P. L., Gilbert, J. J., and Frost, T. M. (1979). Bacterial feeding by the rotifer Brachionus calyciflorus clearance and ingestion rates, behavior and population dynamics. Oecologia 30, 26–30. doi: 10.1007/BF00346392
Strungaru, S. A., Jijie, R., Nicoara, M., Plavan, G., and Faggio, C. (2019). Micro- (nano) plastics in freshwater ecosystems: abundance, toxicological impact and quantification methodology. Trends Anal. Chem. 110, 116–128. doi: 10.1016/j.trac.2018.10.025
Summers, S., Henry, T., and Gutierrez, T. (2018). Agglomeration of nano- and microplastic particles in seawater by autochthonous and de novo-produced sources of exopolymeric substances. Mar. Pollut. Bull. 130, 258–267. doi: 10.1016/j.marpolbul.2018.03.039
Vadstein, O. (1993). Particle size dependent feeding by the rotifer Brachionus plicatilis. Hydrobiologia 255, 261–267. doi: 10.1007/978-94-011-1606-0_34
Vosshage, A. T. L., Neu, T. R., and Gabel, F. (2018). Plastic alters biofilm quality as food resource of the freshwater gastropod Radix balthica. Environ. Sci. Technol. 52, 11387–11393. doi: 10.1021/acs.est.8b02470
Vroom, R. J. E., Koelmans, A. A., Besseling, E., and Halsband, C. (2017). Aging of microplastics promotes their ingestion by marine zooplankton. Environ. Pollut. 231, 987–996. doi: 10.1016/j.envpol.2017.08.088
Wang, X., Bolan, N., Tsang, D. C. W., Sarkar, B., Bradney, L., and Li, Y. (2021). A review of microplastics aggregation in aquatic environment: influence factors, analytical methods, and environmental implications. J. Hazard. Mater. 402:123496. doi: 10.1016/j.jhazmat.2020.123496
Wiggin, K. J., and Holland, E. B. (2019). Validation and application of cost and time effective methods for the detection of 3–500 μm sized microplastics in the urban marine and estuarine environments surrounding Long Beach, California. Mar. Pollut. Bull. 143, 152–162. doi: 10.1016/j.marpolbul.2019.03.060
Zafiriou, O. C., and Farrington, J. W. (1980). The use of DAPI for identifying aquatic microfloral. Limnol. Oceanogr. 25, 943–948. doi: 10.4319/lo.1980.25.5.0943
Zettler, E. R., Mincer, T. J., and Amaral-Zettler, L. A. (2013). Life in the “plastisphere”: Microbial communities on plastic marine debris. Environ. Sci. Technol. 47, 7137–7146. doi: 10.1021/es401288x
Zhao, S., Ward, J. E., Danley, M., and Mincer, T. J. (2018). Field-based evidence for microplastic in marine aggregates and mussels: implications for trophic transfer. Environ. Sci. Technol. 52, 11038–11048. doi: 10.1021/acs.est.8b03467
Keywords: microplastics ingestion, Brachionus calyciflorus, aggregation, microplastics, polystyrene, functional response
Citation: Drago C, Pawlak J and Weithoff G (2020) Biogenic Aggregation of Small Microplastics Alters Their Ingestion by a Common Freshwater Micro-Invertebrate. Front. Environ. Sci. 8:574274. doi: 10.3389/fenvs.2020.574274
Received: 19 June 2020; Accepted: 30 November 2020;
Published: 21 December 2020.
Edited by:
Andrew Turner, University of Plymouth, United KingdomReviewed by:
Ceri Lewis, University of Exeter, United KingdomUlrike Obertegger, Fondazione Edmund Mach, Italy
Copyright © 2020 Drago, Pawlak and Weithoff. This is an open-access article distributed under the terms of the Creative Commons Attribution License (CC BY). The use, distribution or reproduction in other forums is permitted, provided the original author(s) and the copyright owner(s) are credited and that the original publication in this journal is cited, in accordance with accepted academic practice. No use, distribution or reproduction is permitted which does not comply with these terms.
*Correspondence: Claudia Drago, ZHJhZ28mI3gwMDA0MDt1bmktcG90c2RhbS5kZQ==