- 1Department of Land, Air, and Water Resources, University of California, Davis, Davis, CA, United States
- 2U.S. Geological Survey, Sacramento, CA, United States
The ability of wetlands to accrete organic matter in response to rising sea level is a key to landscape resilience, especially in light of reduced sediment availability consequent to dam construction and channelization. This study examined the degradation of cattail (Typha spp.) and tule (Schoenoplectus acutus) litters in restored wetlands through the lens of lignin, a major structural biopolymer in vascular plants with degradation characteristics very sensitive to oxic versus anoxic conditions. A series of litterbags were deployed during the first 10 years after flooding of Deep (55 cm) and Shallow (25 cm) restored wetlands. As emergent marsh vegetation spread through the maturing wetlands, anoxic conditions were more prevalent and overall degradation rates of litter in litterbags were lower. In later experiments in the maturing wetlands, lignin was progressively enriched in litter as evidenced by carbon-normalized yields (Λ8) that increased in tule starting materials from 6.3 to 7.1 mg 100 mgOC–1 to as high as 9.9 mg 100 mgOC–1, and in cattail starting materials from 5.9 to 7.0 mg 100 mgOC–1 to as high as 10.9 mg 100 mgOC–1. However, in an experiment initiated soon after the restored wetlands were constructed, Λ8 in tule litter decreased from 6.8 to 3.6 mg 100 mgOC–1, highlighting the prevalence of initial oxic conditions. With the exception of the early oxic conditions for tule, there was an overall trend of decreasing lignin acid-to-aldehyde ratios with litter degradation, which runs counter to most studies in the literature. We hypothesize that this reflects the utilization of more oxygen-rich lignin components as electron acceptors in redox reactions. No consistent differences were observed in degradation patterns between the Shallow and Deep wetlands. There were distinct differences in lignin degradation in cattail (more resistant) versus tule (less resistant), which indicates that although anoxia may be the dominant control on organic matter accretion in wetlands, specific types of vegetation in restored or constructed wetlands affects organic matter preservation, and hence accretion. Thus, selective management of predominant species in wetlands may prove important for the ability of wetlands to maintain emergent vegetation during sea level rise and to preserve the overall stability of wetland soils.
Introduction
Rising sea levels have increased interest in the role of tidal wetlands for shoreline protection, adaptation, and climate change mitigation. Under what circumstances can wetlands accrete material at a rate that keeps up with sea level rise, or perhaps as important, catch up to current sea level? Past studies have led to debates about the relative importance of sediment supply to both accretion and longer-term stability, i.e., we know that wetlands can accrete through organic matter (OM) preservation with minimal sediment inputs (Miller et al., 2008), but we don’t know if those wetlands will offer similar coastal protection benefits compared to wetlands with more sediment. Across all factors is the question as to whether variable chemical compositions of different plant sources exert significant controls on OM preservation in wetland soils or whether OM accretion is almost entirely driven by anoxic conditions.
A fundamental paradigm for decades in soil OM (SOM) stabilization centered on the idea that aromatic-rich compound classes such as lignin are key toward building OM with an inherent resistance to microbial degradation. While this paradigm has been heavily disputed in unsaturated soils (Bahri et al., 2006; Heim and Schmidt, 2007), there is still strong reason to suspect that it plays a role in saturated wetland soils, as the primary enzymes used to degrade aromatic structures require the availability of oxygen. Despite the widespread use of molecular-level lignin measurements in environmental studies, this idea has not been well-tested in wetland accretion.
More broadly, much of what we know about the relationship between chemical structure and preservation in peats is derived from bulk techniques such as elemental analyses and 13C-NMR. Orem and Hatcher (1987) observed in sawgrass-derived peats an initial decrease in oxygen content of OM from 43.5% in surface horizons to 30.8% at approximately one meter, which can be readily explained by the preferential loss of oxygen-rich carbohydrates. This loss of carbohydrates is apparent in 13C-NMR spectra, which also show a greater abundance of phenolic, aromatic, and aliphatic carbon with increasing age. A likely source of this preserved carbon is vascular plant-derived phenolic lignin, aliphatic cutin, or suberin, which contains both aromatic and aliphatic components. Some have also attributed the aliphatic carbon to periphyton sources (Gaiser, 2009). However, very little molecular work has been done to confirm any of these sources in peats. Earlier molecular-level lignin analyses are largely confined to lignin-poor, cutin-poor sphagnum peats (Williams et al., 1998; Zaccone et al., 2008). Bourdon et al. (2000) observed general increases in acid:aldehyde ratios of lignin-derived phenols downcore in a cypress peaty marsh that they attributed to increased degradation. However, changes in acid:aldehyde ratios can also be due to simple solubilization (Hernes et al., 2007); hence, it is not clear whether degradation or solubilization is the dominant control on changing lignin compositions.
Lignin measurements have also been used in more recent studies as a means of deciphering OM source changes through time, with the relative proportions of woody versus nonwoody vegetation as the primary question (Zhang et al., 2019). In one study, there is evidence for a decrease in carbon-normalized lignin with age down core (Zhang et al., 2019), which runs counter to the idea that lignin and other aromatic compounds are preferentially preserved in anoxic settings. However, carbon-normalized yields are also sensitive to organic matter sources, so it is not entirely clear whether significant shifts are due to preferential degradation or changing biomes, as the decrease in carbon-normalized lignin yields were also accompanied by significant enrichment in δ 13C of 8–10‰ (Zhang et al., 2019). Studies of SOM stabilization in mineral soils provide information important to understanding preservation in peats. For example, Filley et al. (2008) demonstrated increased stores of unaggregated SOM in grassland soils when woody plant encroachment occurs, and changing lignin parameters captured the plant succession.
One means for directly testing the effects of degradation on lignin compositions from a single source is the use of litterbags. In one such study, leaves, grass, needles, and woods were introduced via litterbags into large tanks with continually flowing estuarine water and sampled for 4 years (Opsahl and Benner, 1995). Carbon-normalized lignin yields changed significantly in some, but not all sample types, but lignin diagnostic parameters did not change in a consistent manner until ∼75% of the original biomass was degraded after 1–2 years (Opsahl and Benner, 1995). While certainly relevant to lignin degradation in wetlands, a key difference is that these litterbag studies were conducted in oxygenated water.
In the study presented here, we utilized lignin analyses of cattail (Typha spp.) and tule (Schoenoplectus acutus) litterbags submerged in an experimental restored wetland in the Sacramento River/San Joaquin Delta in order to assess the relative importance of plant species (and associated lignin compositions) versus environmental conditions (i.e., anoxia) in the degradation/preservation of lignin. This open question is of fundamental importance toward future efforts in restoring wetlands for their multiple benefits to ecosystem services.
Materials and Methods
Site Description
Twitchell Island, the site of the constructed wetlands, resides in the northwestern portion of the Sacramento/San Joaquin River Delta (the Delta). As is typical of most islands in the Delta, it was surrounded by levees and drained for agriculture ∼150 years ago due to its rich, peaty soils. However, over time this has led to significant subsidence due to oxidation and erosion of the organic peats as well as compaction. The constructed wetlands (each 3 ha in surface area) were positioned in the central portion of Twitchell Island on a former agricultural field in an area that was ∼4.5 m below sea level. Water in the wetlands was contained by constructing berms from surface soil such that the West (Shallow) site could be maintained at 25 cm water depth and the East (Deep) site could be maintained at 55 cm water depth (Figure 1). Tule (Schoenoplectus acutus) were planted in five 10 × 25 m blocks, spaced every 2 m in the eastern half of both sites immediately before flooding, and cattails (Typha spp.) in surrounding ditches provided windborne seed (Miller et al., 2008). San Joaquin River water was pumped continuously into the south side of the wetlands with outflows on the north side governed by weirs, with flows such that mean water residence time was approximately 6 days in the West site and 13 days in the East site. In 2005, inflows were reduced to minimize outflow.
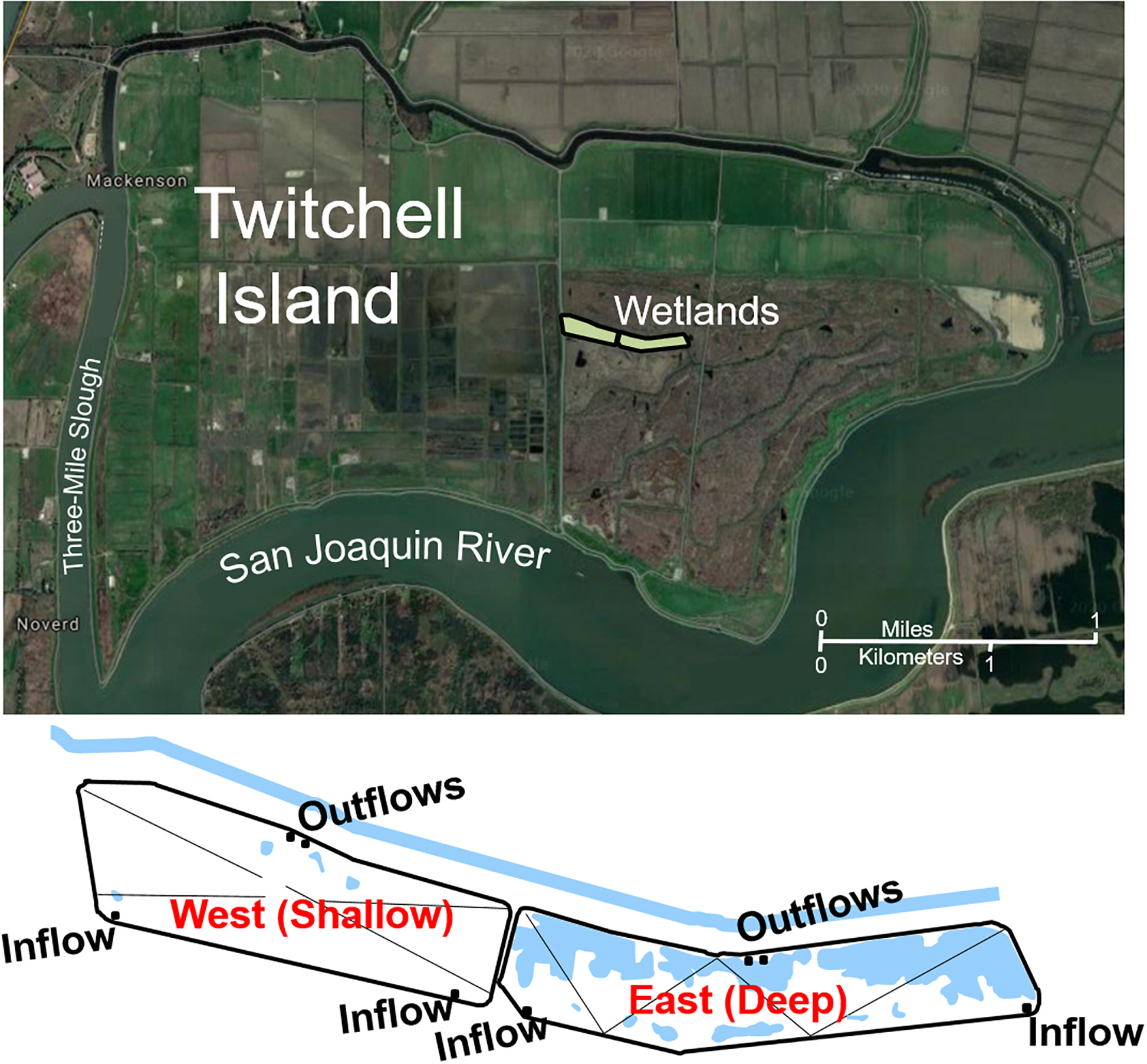
Figure 1. Location of reconstructed wetlands on Twitchell Island (each 3 ha) near the confluence of the Sacramento River and San Joaquin River in Central California, reproduced from Miller et al. (2008). The west wetland was maintained at 25 cm water depth and is referred to in the text as the Shallow wetland. The east wetland was maintained at 55 cm water depth and is referred to in the text as the Deep wetland.
Litterbag Experiments
Senescent, field-dried tule and cattail litters were deployed in mesh bags in the surface of both the Shallow and Deep wetlands (Miller and Fujii, 2010, 2011) in a series of 2-year studies initiated in Nov. 1997, May 2000, Feb. 2003, and Mar. 2005. Tule and cattail are both angiosperms that produce all structural types of lignin phenols. Each bag in the 1997 experiment contained ∼11 g of material, and all subsequent experiments utilized ∼15 g per bag. All litterbags were assigned unique identification numbers, and mass loss upon retrieval was calculated by subtracting the dried weight from the initial weight. The litterbags were all tethered to a pole to prevent them from drifting, and over the course of several weeks to a few months eventually became waterlogged and sank. An excess of litterbags were deployed to accommodate loss (e.g., ripped mesh or vegetation growing up through the bags). The location of most deployments was chosen to be in near proximity to established stands of emergent vegetation. The single exception was a Deep wetland deployment in the 2005 study that was specifically chosen to be in open water (i.e., no emergent vegetation but still an abundance of submerged and floating vegetation) nearer the water inlet as a comparison to other deployments. The Shallow wetland had no open water by 2005 to enable a similar comparison. Final sampling times varied among the deployments from 728 to 811 days (2+ years).
Although multiple litterbags were pulled at each sampling time/location/species, only a single sample was analyzed for lignin due to the time and expense involved in molecular level analyses. In all 2-year experiments, the T0 (initial) sample analyzed for lignin was a composite of five bags.
Lignin Analyses
Approximately 10 mg of dried, homogenized tule or cattail sample was weighed into monel reaction vessels (Prime Focus, Inc.) for lignin phenol analysis utilizing the alkaline cupric oxide (CuO) oxidation method of Hedges and Ertel (1982) with modifications as outlined in Spencer et al. (2010) and Hernes et al. (2013). Following oxidation, cinnamic acid was added as an internal standard; samples were acidified to pH = 1 with 12 N H2SO4, extracted three times with ethyl acetate, passed through Na2SO4 drying columns and dried under ultrapure nitrogen. Prior to GC-MS analysis, lignin phenols were redissolved in pyridine and trimethylsilyl-derivatized with equal parts sample and bis-trimethylsilyltrifluoromethylacetamide (BSTFA) for 10 min at 60°C. Lignin phenols were quantified on a GC-MS (Agilent 6890 gas chromatograph and 5973 mass selective detector and a DB-5ms capillary column; 30 m, 0.25 mm inner diameter, J&W Scientific) using a five-point calibration scheme (Hernes and Benner, 2002), and calibration curves were generated with quadratic fits. To account for changes in chromatography during a sample run, calibration curves were run before and after every 12 samples injected, and final values were calculated using a weighted average of values derived from each calibration curve based on proximity to each curve in the injection order. One Dabob Bay sediment standard (Spencer et al., 2010) and one blank were run for every 10 samples oxidized, and all samples were blank corrected (average correction was <0.1% of the sample values). Eight lignin phenols comprising three vanillyl phenols: vanillin (VAL), acetovanillone (VON), and vanillic acid (VAD); three syringyl phenols: syringaldehyde (SAL), acetosyringone (SON), and syringic acid (SAD); and two cinnamyl phenols: p-coumaric acid (CAD) and ferulic acid (FAD) were quantified in all samples. Typical lignin parameters include the following: (1) the sum of all eight phenol normalized to organic carbon, Λ8, in units of mg 100 mgOC–1, (2) ratios of the three syringyl phenols (S) to the three vanillyl phenols (V), S:V, (3) ratios of the two cinnamyl phenols (C) to V, C:V, and (4) ratios of vanillic acid to vanillin (an aldehyde), (Ad:Al)v.
Results and Discussion
Differential Degradation Across Time and Between Plant Types
The Twitchell Island Demonstration Project was initiated in 1997 to study wetland accretion by constructing a two 3 ha wetlands on Twitchell Island in the Sacramento/San Joaquin River delta (the Delta). Although it is unclear how long it takes for restored wetlands to reach maturity, it was expected that change would be quite rapid in the early years, and this is evident by a number of parameters. Coverage of emergent vegetation in the Shallow wetland (25 cm water depth) was 90% after 2 years, while the Deep wetland (55 cm water depth) had nearly 50% coverage (Miller and Fujii, 2010).
Dissolved oxygen concentrations within the wetlands are variable according to proximity to inlets, temperature, water depth, water flow (with residence time as a proxy) and the balance at any given point between primary production and respiration. Continuous oxygen concentrations were not measured; however, oxygenation and pH were reported to correlate (Miller and Fujii, 2010) (depletion of oxygen through respiration generates carbonic acid), and pH decreased sharply in the first 2 years before becoming more stable by summer of 1999, reflecting more extensive anoxia. This also parallels effluxes of CH4 (an indicator of anoxic redox reactions) and CO2 (an indicator of oxygen consumption through respiration), which showed significant increases in the 1998 and 1999 growing seasons before stabilizing in subsequent years (Miller, 2011). In the same gas efflux study, ∼70 surface water (approximately monthly) dissolved oxygen measurements within emergent vegetation averaged ∼8% saturation (Miller, 2011), with the inference that water at the soil/water interface would have been at or near 0%. Other investigators have reported dissolved oxygen at ∼6% of saturation at 10–15 cm water depth in these wetlands (Utz, 2016) and <1 mg L–1 at the peat-standing water interface in the interior of the wetland (He et al., 2015). Microenvironments within the litterbags likely achieved anoxia more rapidly than surrounding water, and the density of organic matter in the litterbags serves as a buffer to changing conditions.
Mass Loss Across the Litterbag Experiments
Trajectories of tule mass loss exhibited significant differences between litterbag studies started in 1997 compared to those started in 2000, 2003, and 2005 with the differences most pronounced at ∼1 year when <10% of the 1997 starting mass remained in the Deep wetland, but percent remaining in the 2003 and 2005 experiments was >50% (Figure 2A). Even in the Shallow wetland, the mass remaining at ∼1 year was more than twofold greater in the 2003 and 2005 experiments compared to 1997 (Figure 2B). In both the Deep and Shallow wetlands, there was some convergence at ∼2 years, with little additional loss in the 1997 experiments but continued decline in the others. Of special note was the 2005 deployment in open water, which led to significantly greater mass loss than its counterpart deployed near emergent vegetation (Figure 2A), thus highlighting the importance of anoxia in enhancing preservation.
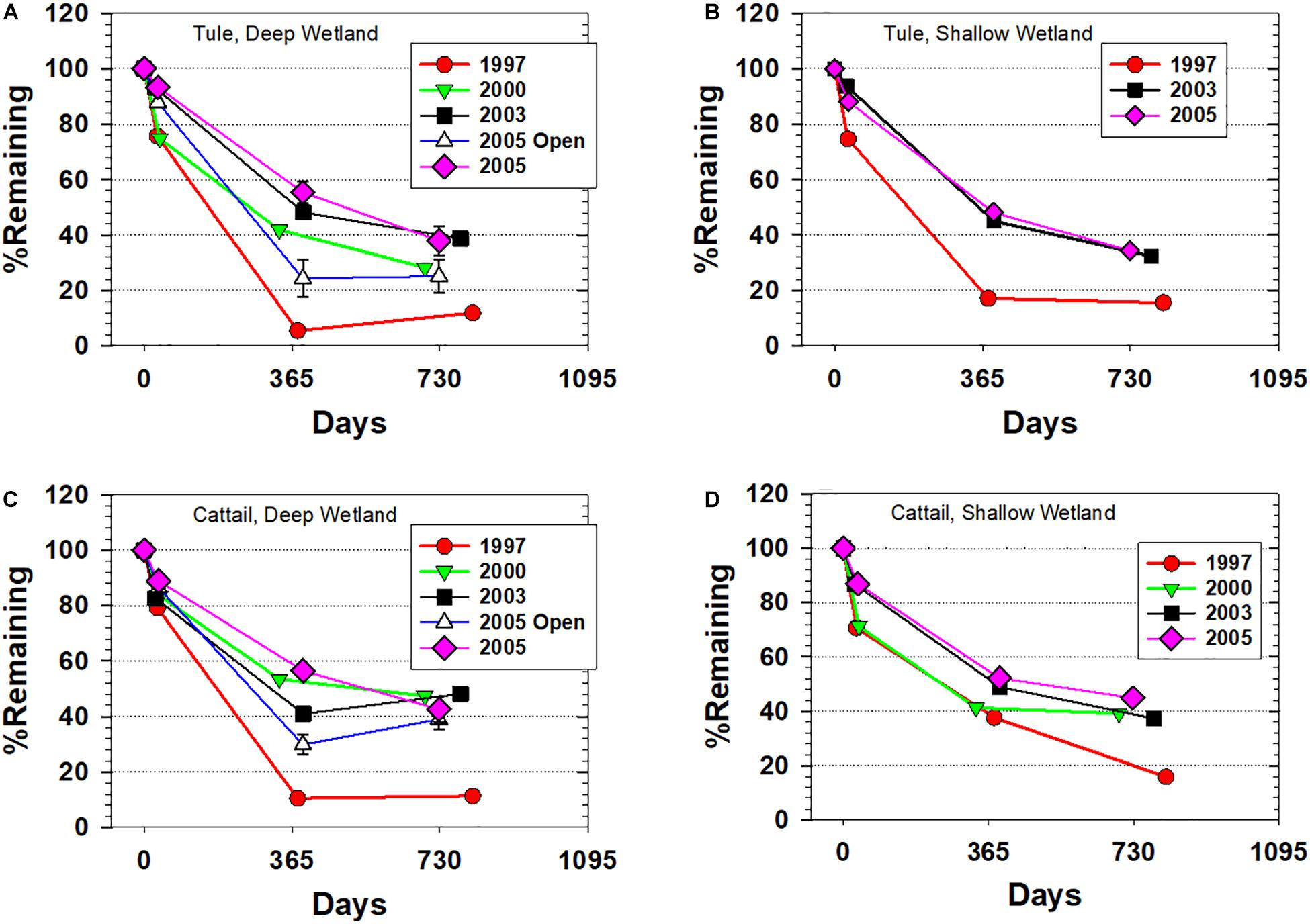
Figure 2. Ash-free percent remaining for all sampled litterbags versus time across the four deployments. (A) Tule litterbags from the Deep wetland, (B) Tule litterbags from the Shallow wetland, (C) Cattail litterbags from the Deep wetland, and (D) Cattail litterbags from the Shallow wetland. Error bars for select samples represent the difference between two litterbags that were averaged. “2005 Open” refers to a special deployment in open water near the water inlet.
Cattail mass loss trajectories largely mirrored those of the tule experiments: 1997 experiments in the Deep wetland demonstrated ∼90% mass loss at ∼1 year, the open water deployment in 2005 had half the percentage remaining at ∼1 year compared to the 2005 deployment near emergent vegetation, and some convergence was observed at ∼2 years (Figure 2C). The Shallow wetland, however, showed less degradation of the cattail at ∼1 year, before more closely matching tule values at ∼2 years (Figure 2D).
The changes in the mass loss trajectories from one deployment to the next demonstrates that at least out to 8 years, the wetlands were still evolving, likely with greater extent of anoxic/hypoxic conditions. The difference between the 1997 experiment and all subsequent experiments stands out as an example of how rapidly these wetlands evolved when flooding was initiated. In particular, the greater area of open water in 1997–1998 suggests that deployed litterbags were likely to have experienced extensive oxygenation. Therefore, the 1997 study could be expected to show greater extent of degradation both in bulk and molecular terms than litterbags started 6 years into the project, and indeed, these 1997 experiment samples showed the most rapid and most complete mass loss (Figure 2). The open water deployments in the Deep wetland in 2005 largely confirm the significance of oxygenation. The similarities of mass loss at ∼2 years for all 2003 and 2005 deployments among emergent vegetation, however, do demonstrate that the rate of change in the wetlands was declining.
A second notable mass loss observation is that there was very little difference between the Shallow and Deep wetlands, despite at least two factors that could impact oxic versus anoxic conditions: (1) deeper water is more prohibitive for oxygen diffusion from the atmosphere, and (2) greater water volume and increased water residence time in the Deep wetland also means that oxygen replenishment from the San Joaquin River source waters on a per volume basis is reduced and there is more time for oxygen removal. The absence of any significant differences in mass loss between the two wetlands indicates that litterbags were experiencing similar conditions, and the simplest explanation is that after 1997, they were both largely anoxic at the deployment sites.
Finally, mass loss comparison in the post-1997 experiments reveals slightly greater mass loss in tule (all <40% remaining after 2 years) compared to the cattail (most >40% remaining after 2 years). Difference in degradation rate between two plant species in and of itself is not a surprising finding, but it does point toward the potential for plant species composition to impact accretion rates of organic material in wetlands. An unknown for these two species is whether this difference would also be apparent in a study of the root biomass, which are known to have compositional distinctions from the shoots. Notably, all litterbags post-1997 demonstrated 25–40% remaining after 2 years, which shows strong potential for accretions. Indeed, 10-year accretion rates in these wetlands were measured at 3–6 cm year–1 in areas with emergent vegetation (Miller et al., 2008; Miller and Fujii, 2011). The dominant source of accreted material was organic matter, which is supported by these litterbag studies.
Implications of Molecular Changes in Lignin With Degradation
Linking mass loss data to specific mechanisms based on organic matter composition is critical toward developing predictive tools that can aid managers in making decisions about wetland restoration. In this experiment, decreasing degradation between the 1997 experiment and subsequent experiments appears to relate to developed anoxic conditions, which should then be evident in lignin carbon-normalized yields, Λ8. Lignin degradation proceeds most rapidly in oxygenated conditions, where non-specific enzymes (oxidases like laccases and heme-containing peroxidases) can utilize oxygen to break ring structures in the randomized lignin polymer (Janusz et al., 2017). Those enzymes become ineffective in an anoxic environment, in which case lignin should be more resistant to degradation than bulk carbon, as evidenced by increasing Λ8. In contrast, oxygenated conditions enables lignin degradation that more closely tracks carbon and mass loss, which is best exemplified by lignin turnover rates in surface soils that are essentially the same as for bulk carbon (Bahri et al., 2006; Heim and Schmidt, 2007).
Across the four sets of litterbag experiments analyzed for lignin in this study, Λ8 increased or remained relatively constant across all time points and conditions with three exceptions: (1) the tule litterbags that were deployed in 1997 decreased markedly from 6.21 to 3.97 mg 100 mgOC–1 in the Deep wetland and from 6.75 to 3.59 mg 100 mgOC–1 in the Shallow wetland, consistent with degradation under oxic conditions, (2) two of the three tule litterbag deployments in 2005 demonstrated an initial increase in Λ8, (6.62 to 9.85 mg 100 mgOC–1 in the Deep wetland open water experiment, 6.62 to 8.28 mg 100 mgOC–1 in the Shallow wetland) but then decreased over the last 1–2 years (down to 7.45 mg 100 mgOC–1 in the Deep wetland open water and 6.91 mg 100 mgOC–1 in the Shallow wetland), and (3) the cattail litterbag deployment in 2000 increased initially from 6.70 to 7.87 mg 100 mgOC–1 before decreasing to 6.32 mg 100 mgOC–1 (Figure 3). Using lignin as a marker for environmental conditions, we would interpret that these litterbags in which Λ8 decreased were experiencing oxic conditions over those periods.
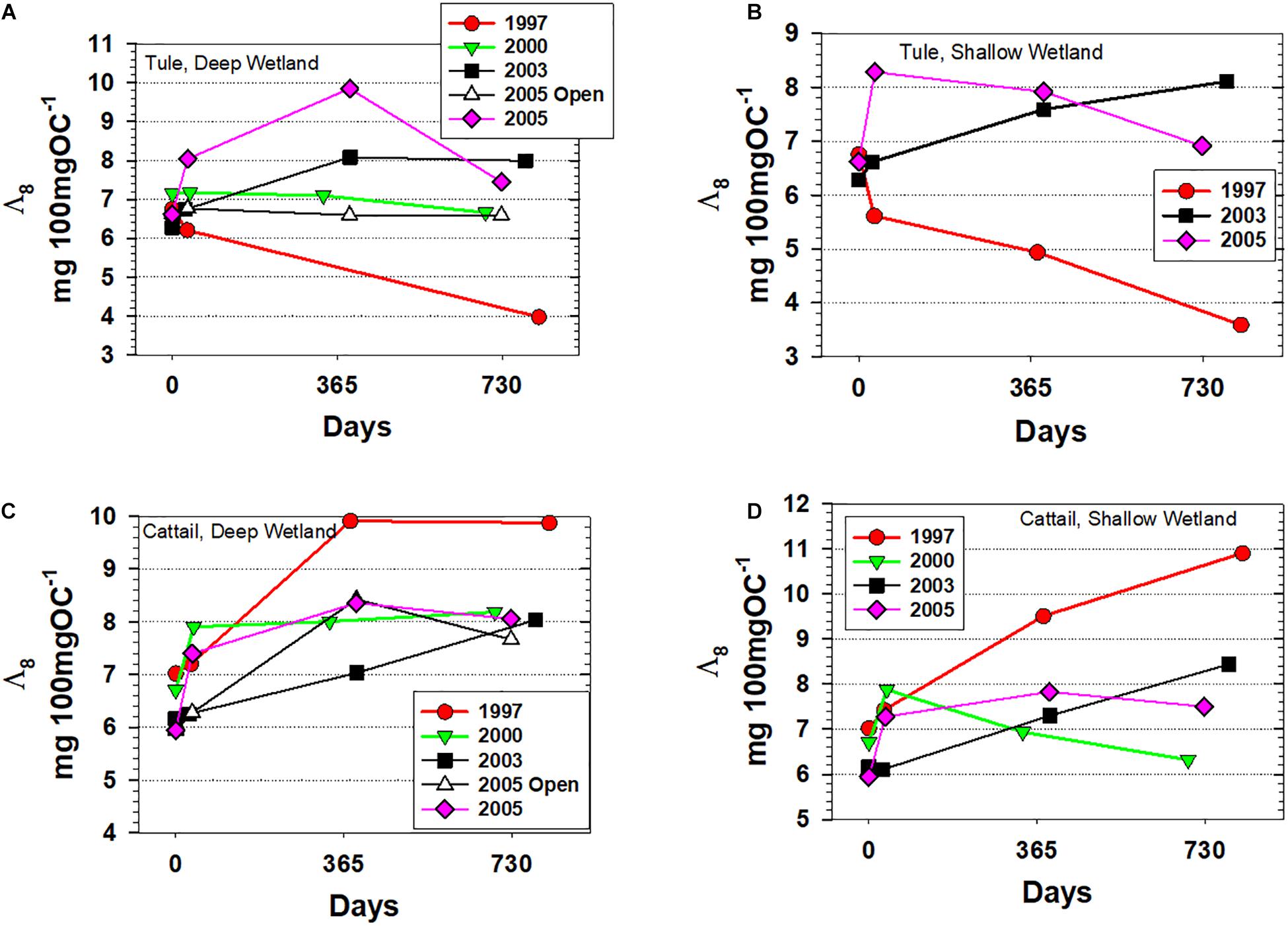
Figure 3. Carbon-normalized lignin yields for all experiments plotted versus days. (A) Tule litterbags from the Deep wetland, (B) Tule litterbags from the Shallow wetland, (C) Cattail litterbags from the Deep wetland, and (D) Cattail litterbags from the Shallow wetland.
However, if the 1997 tule litterbags experienced oxygenated conditions during the noted periods, then presumably the cattail litterbags deployed nearby might have also been under the same conditions and therefore would be expected to show the same extent of loss. And while the 2000 cattail experiment demonstrates that cattail Λ8 can also decrease under the right environmental conditions, the trends between cattail and tule in the 1997 experiment could not have been more disparate, with cattail Λ8 increasing from 7.02 to 9.87 and 10.90 mg 100 mgOC–1 in the Deep and Shallow wetlands, respectively (Figure 3). This result indicates that environmental conditions are not the only factor that dictates preservation potential in Twitchell Island wetlands, as species-specific composition and biopolymer conformation are important factors as well.
Another way to understand the mechanisms behind the loss rates of lignin-containing tissues during degradation under different conditions is to track the progression of lignin acid:aldehyde ratios. These ratios are essentially a measure of oxygenation, as the acid is structurally identical to the aldehyde with the exception of one oxygen that converts the aldehyde to an acid. In other words, under oxic degradation, it is typical for acid:aldehyde ratios to increase. Not surprisingly, the largest increase in acid:aldehyde for the vanillyl phenols, (Ad:Al)v, coincided with the largest decrease in Λ8, i.e., the tule experiments initiated in 1997 (Figure 4). The increase in (Ad:Al)v for tule doubled from 0.19 to 0.38 and nearly spanned the entire range (0.16 to 0.38) of (Ad:Al)v measured across all litterbag experiments, again a clear reflection of more oxidized conditions at the beginning of wetland restoration compared to later years.
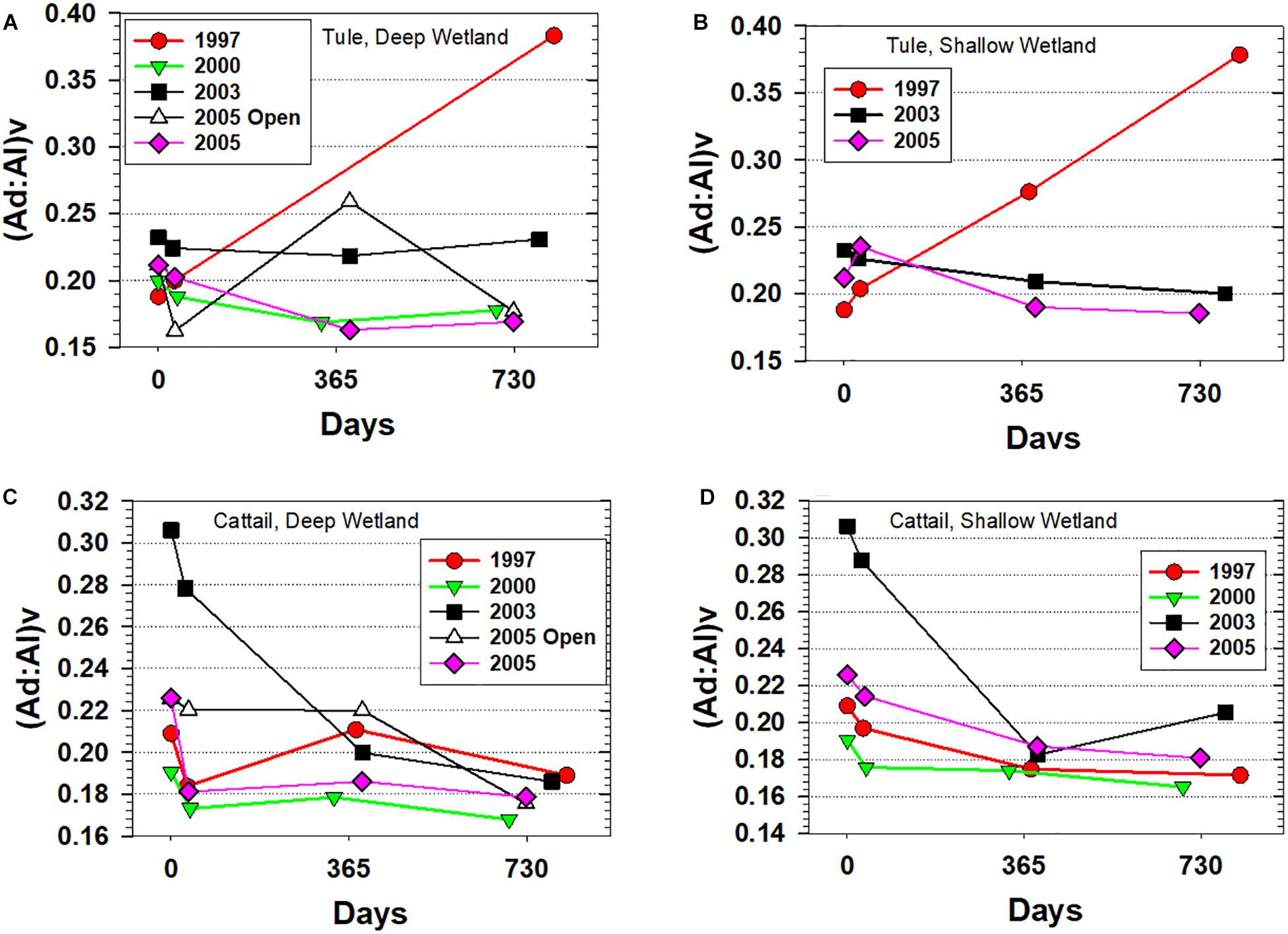
Figure 4. Vanillic acid to vanillin ratios, (Ad:Al)v, for all experiments plotted versus days. (A) Tule litterbags from the Deep wetland, (B) Tule litterbags from the Shallow wetland, (C) Cattail litterbags from the Deep wetland, and (D) Cattail litterbags from the Shallow wetland.
Despite similar mass loss in cattail compared to tule in the 1997 experiment, (Ad:Al)v for cattail was barely impacted, and in fact was slightly lower at the end of 2 years. In fact, cattail (Ad:Al)v in general trended in a direction opposite to that expected from degradation, in stark contrast to the singular trend in (Ad:Al)v for 1997 tule that is quite consistent with classical expectations for how lignin compositions change with degradation. The most notable decrease in cattail (Ad:Al)v occurred in the experiments initiated in 2003, with decreases from 0.31 to 0.18/0.19 (Figure 4)—a particularly interesting trend for multiple reasons. First, the elevated (Ad:Al)v of the starting material is distinctly greater than all other starting materials, and demonstrates a much larger range across all the experiments in fresh or senescent plant material for a single species than would typically be expected. Second, while increases in (Ad:Al)v as degradation progresses are well-documented in numerous studies of plant materials, woods, dissolved organic matter, and particulate organic matter (e.g., Hedges et al., 1988; Opsahl and Benner, 1995; Hernes and Benner, 2003), this is a rare instance in the published literature in which (Ad:Al)v is decreasing with degradation. In fact, the overall trend of decreasing (Ad:Al)v is evident in a number of the litterbag experiments (Figure 4), but simply most pronounced with the two 2003 cattail litterbag experiments.
Although lignin degradation studies in wetlands are comparatively rare, there are other relevant studies that record lignin degradation in intact plant materials in anoxic environments (Hedges et al., 1985; Hedges and Weliky, 1989; Opsahl and Benner, 1993, 1995). Comparisons of three types of wood (spruce, alder, and oak) in 2500 years marine sediments with their modern counterparts indicated >75% loss of biopolymer, increases in Λ8 across all woods (10.9 to 11.7 mg 100 mgOC–1 in spruce, 21.1 to 32.1 mg 100mgOC–1 in alder, and 19.0 to 28.9 mg 100 mgOC–1 in oak) that is consistent with anoxic degradation, and yet (Ad:Al)v was similar or increased in all degraded woods (0.13 to 0.20 in spruce, 0.13 to 0.14 in alder, and 0.11 to 0.21 in oak) (Hedges et al., 1985). The most direct comparison is a study in which conifer needles were analyzed for their lignin compositions downcore in a coastal sediment. Mixed fir/hemlock needles increased in Λ8 from 6.9 to 9.0 mg 100 mgOC–1 in the upper 0.5 m, while (Ad:Al)v decreased from 0.37 to 0.25 before increasing slightly at the bottom of the core to 0.29 (Hedges and Weliky, 1989). The authors in that study speculated that the decrease was due to a preferential loss of ester-bound vanillic acid that is readily cleaved. However, subsequent experiments involving a mix of species and tissues indicated that ester-bound lignin phenols were only a small portion of the total lignin and the ratios of ester-bound acids to aldehydes was not that different from bulk values (Opsahl and Benner, 1995). Yet another degradation study involving shoalgrass (Halodule wrightii) demonstrated that even when ester-bound lignin phenols are abundant, they are largely cleaved and lost from the litter material in 2 months (Opsahl and Benner, 1993), suggesting that any loss of ester-bound lignin phenols from pine needles would happen disproportionately at the top of the sediment core and not gradually. Therefore, alternate explanations for declining (Ad:Al)v with degradation in anoxic environments are needed.
The strong linear correlation between (Ad:Adl)v and (Ad:Al)s for both tule and cattail (Figure 5) indicates that whatever mechanism is responsible for declining (Ad:Al)v needs to account for both syringyl and vanillyl phenols. Fundamentally, all organic matter degradation involves redox reactions, and under anoxic conditions there are many different electron accepters to drive those reactions—for example the loss of carboxyl groups from organic acids, which would have the effect of reducing organic matter oxygen content and reducing acid:aldehyde ratios. However, within the lignin polyphenol structure, lignin monomers exist as phenylpropyl structures (aromatic ring with a three-carbon sidechain), and it is only with the CuO alkaline oxidation that those propyl sidechains are cleaved down to the structures that are commonly quantified and reported in lignin studies. In other words, within the lignin polymer, the acids and aldehydes from which acid:aldehyde is derived do not exist, only phenylpropyl precursors. Therefore, decreasing acid:aldehyde ratios in lignin are actually more complex. The propyl sidechains in the lignin polymeric structure are typically depicted as having hydroxyl (alcohol) and carbonyl (ketone/aldehyde) functional groups, and both have the capacity for reduction (i.e., loss of oxygen as water) and fall in between nitrate and sulfate on the environmental redox cascade (Stumm and Morgan, 1981). A mechanism such as this operating in wetland conditions would imply that the acid precursors are more efficiently reduced than the aldehyde precursors.
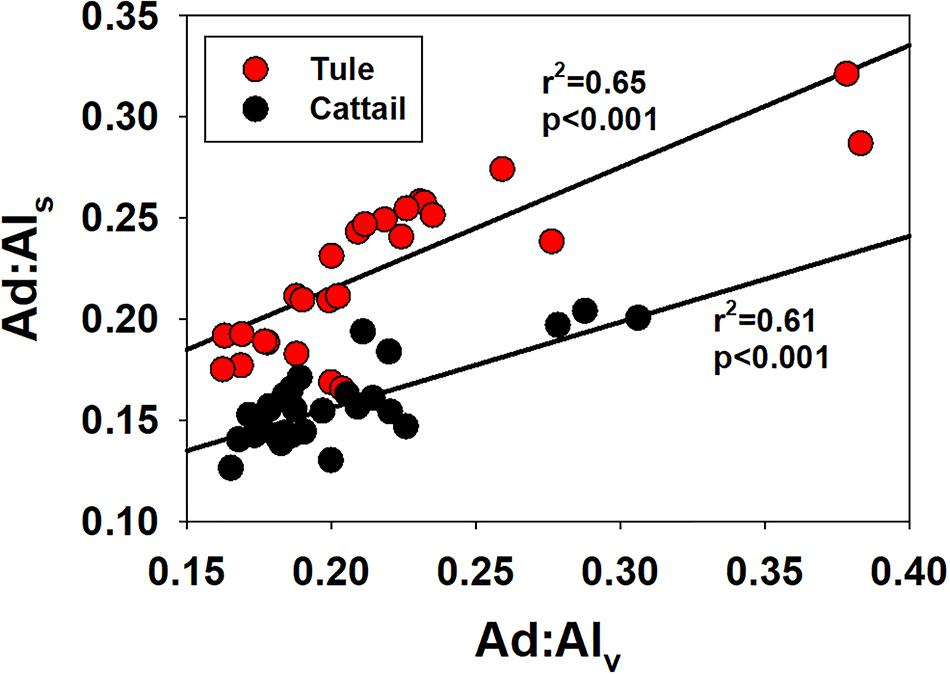
Figure 5. Syringic acid to syringaldehyde ratios, (Ad:Al)s versus vanillic acid to vanillin ratios, (Ad:Al)v, plotted separately for tule and cattail.
Beyond simply elucidating mechanisms for changing lignin composition in plant material, there are more important implications to consider in both the litter material that is preserved and the biomass that is lost. In oxygenated systems, lignin acid:aldehyde values from plant/litter material leachates are always significantly elevated over the parent materials (e.g., Pellerin et al., 2010; Hernes et al., 2017), a clear indication that lignin acid precursors are more soluble. Therefore, processes that generate more acid functionality in lignin will lead to greater and more rapid loss of plant materials. However, if generation of acid functionality is stopped—and even reversed—under anoxic conditions, then this could be one important mechanism to explain enhanced preservation that could lead to higher rates of accretion. Within different plant species, variable conformation of lignin polyphenols and associated polysaccharides could enhance or inhibit conversion of acid precursors, and this might be one way to explain differential degradation between cattail and tule.
Source Ramifications of Litterbag Studies
While lignin acid:aldehyde ratios are primarily used as indicators of degradation, syringyl to vanillyl (S:V) and cinnamyl to vanillyl (C:V) ratios carry source information. Classically, elevated S:V denotes significant angiosperm sources while low S:V signifies gymnosperm sources (Hedges and Mann, 1979). Elevated C:V indicates nonwoody tissues and frequently also differentiates between grasses and leaves, while low C:V indicates woody tissues (Hedges and Mann, 1979). However, these ratios are also subject to degradation effects (Opsahl and Benner, 1995) as well as leaching/solubilization and sorption effects (Hernes et al., 2007; Matiasek and Hernes, 2019); hence, diagenetic processing can blur the original source information.
While S:V demonstrated considerable variability in this study, ranging from 1.19 to 1.64 in tule and 1.36 to 3.58 in cattail (Figure 6; Supplementary Table 1), no degradation trend was evident that contributed to the variability. However, this highlights the diversity of values possible in the same or similar plant species with changing environmental conditions, either in plant growth/biosynthesis under different environmental stressors, or subsequent degradation. C:V demonstrated similar variability, with values ranging from 1.56 to 2.77 across all tule samples and 0.39 to 1.17 across all cattail samples (Figure 6; Supplementary Table 1). In contrast to S:V, however, there is a weak but significant trend of decreasing C:V with degradation time in each of the two species types (Figure 7). Decreasing C:V with degradation has been observed in many studies (Hedges and Weliky, 1989; Benner et al., 1991; Haddad et al., 1992; Opsahl and Benner, 1993, 1995), and initial release of ester-bound cinnamyl phenols does not account for the longer-term trend. Solubility alone also doesn’t explain the decline: whereas leachates from dicots are generally enriched in cinnamyl phenols over vanillyl phenols (i.e., leachates from dicots have higher C:V than the plant material), the trend seems to be the opposite for monocots such as the wetland plants in this study and grasses in general (Hernes et al., 2007, 2017; Pellerin et al., 2010). Clearly, cinnamyl phenols are more susceptible to degradation, either due to inherent structural properties or due to the bonding environment in which they are incorporated into lignin polyphenols; however, it is not apparent how relative cinnamyl proportions in one plant species compared to another translates to preservation potential.
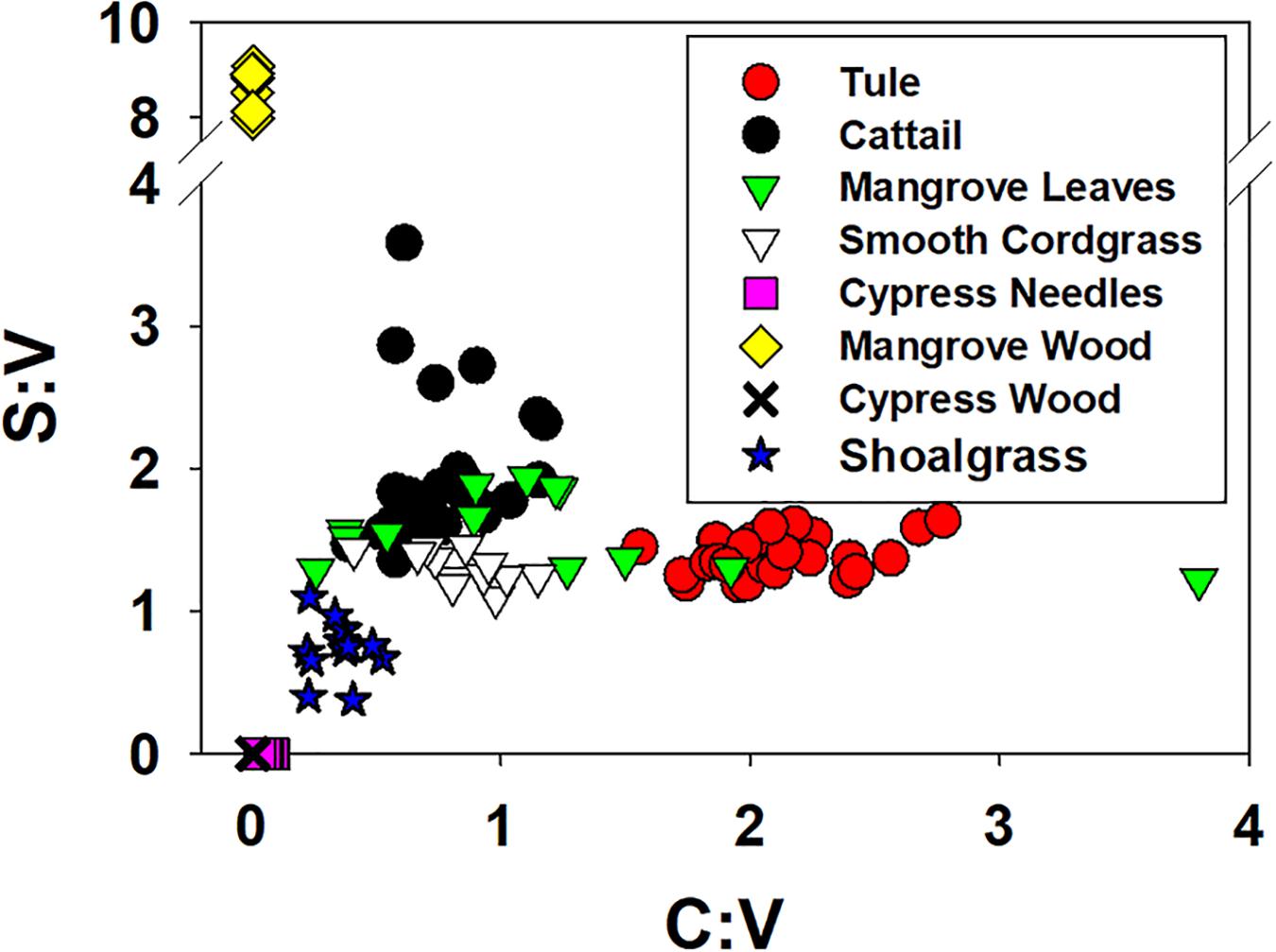
Figure 6. S:V ratios (sum of the syringyl phenols divided by the sum of the vanillyl phenols) versus C:V ratios for this study along with data from the litterbag studies of Opsahl and Benner (1995).
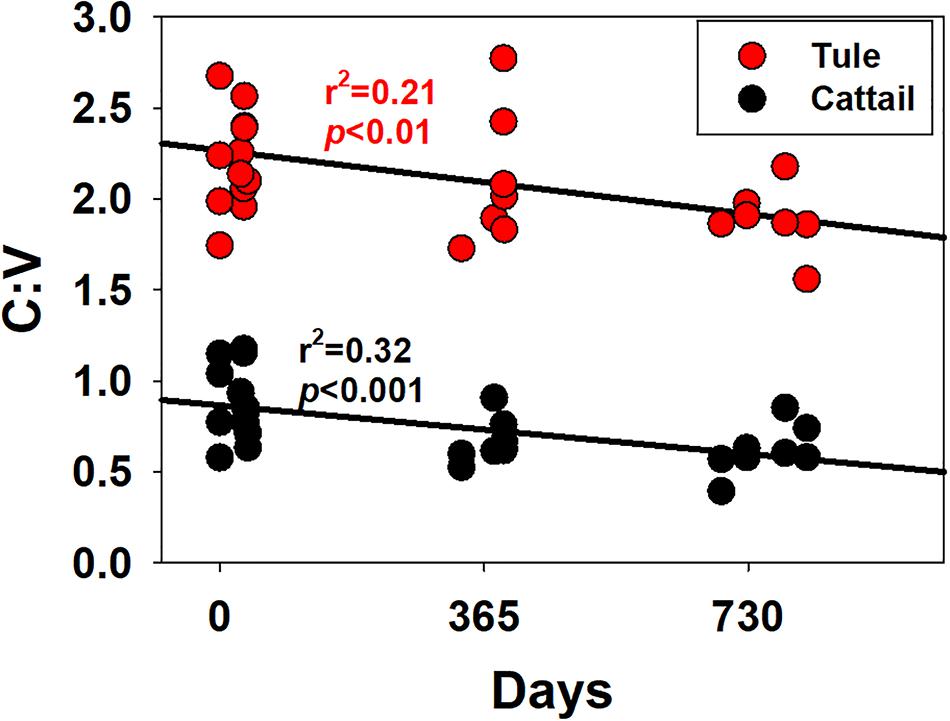
Figure 7. Ratios of the sum of the cinnamyl phenols, C, and the sum of the vanillyl phenols, V, versus time for all samples, plotted separately for tule and cattail.
In addition to questions about how composition relates to preservation, changing S:V and C:V has implications for the classical application of lignin data: source identification. Source interpretation most frequently stems from S:V versus C:V comparisons, but combining the data from this study with the litterbag and shoalgrass data from Opsahl and Benner (1993, 1995) demonstrates how challenging that could be when degradation and seasonal/annual variation in plant biosynthesis is involved (Figure 6). While cypress wood (no syringyl or cinnamyl phenols) and needles (no syringyl and only low levels of cinnamyl phenols) demonstrated little variability across 4 years of degradation, the range of values in the remaining plant types is considerable. The mangrove leaves from Opsahl and Benner (1995) spanned nearly the entire range of C:V in the eight sample types, and prior lignin analyses of tule (Pellerin et al., 2010) has yielded C:V even higher (4.8) than what was measured in this study. The dynamic range of S:V and C:V values for any single plant species has clear ramifications for attempting to use S:V and C:V endmembers for interpreting vegetation sources and biome shifts in sediment and peat cores. This is particularly true for sediment when adding in the combined effects of organic matter solubilization (Matiasek and Hernes, 2019) and sorption to mineral surfaces (Hernes et al., 2007) that alters lignin compositions even further. It is not an easy problem to resolve, as the face value implication seems to suggest that using lignin values from peat and sediment cores in any capacity for biome reconstruction is impossible. And yet there are many measurements used in environmental studies that exhibit a high degree of variability related to primary sources, but when integrated across time and space converge on useful averages, for example capturing lignin biome signatures in riverine deltas that integrate across the entire watershed (Kastner and Goni, 2003). The key is to trace peat and sedimentary values back upstream to primary sources to gain a better understanding for how peat and sedimentary values might differ from original sources.
Implications for Wetland Restoration
With an increasing focus on wetland restoration and all the ecosystem benefits and services provided, it is important to understand different factors that will lead to greater success in maximizing all of those benefits and services while incorporating stability to resist rising sea level and other environmental stressors. For coastal and estuarine wetlands, this is particularly important because of the large decline in sediment supply due to channelization and dam construction (e.g., Syvitski, 2003; Schoellhamer et al., 2013) that has been shown to lead to wetland loss (Day et al., 2000, 2011; Allison et al., 2012). For wetlands to survive the coming period of sea level rise and to help armor our coasts against storm surge, the organic matrix must be sufficiently robust to prevent mechanical loss.
The differential degradation observed between tule and cattail in this study demonstrates the key role that species composition and underlying chemical structures could play in determining the success of wetland restoration in meeting goals. This study indicates that some plant species are preserved more efficiently under typical anoxic conditions in wetlands; however, wetlands are complex systems, and restoration is generally motivated by multiple desirable services in addition to accretion/preservation. For example, zooplankton feeding experiments seem to indicate that tule could be highly beneficial for the lower foodweb in the Delta (Harfmann et al., 2019), and tule is much preferred over cattail for habitat and can survive deeper flooding in winter as well as higher water flows. Yet based on this study, tule might be less effective toward meeting goals for accretion and carbon sequestration than cattail. Prior studies have identified tule and phragmites as primary components of ancient delta peats (Atwater, 1980); however, the prevalence of invasive species likely changes what is possible in reconstruction, as initial vegetation analyses in these reconstructed ponds indicated much greater coverage from self-seeding cattail than from planted tule (Miller and Fujii, 2011). Another important variable to consider is that accretion is derived from both above and below-ground biomass and that this study did not include degradation of below-ground biomass. The latter has rarely been analyzed at the molecular level for lignin, but indications are that below-ground biomass is notably different in composition (e.g., more lignin and suberin) compared to its above-ground tissues (Filley et al., 2008). Nevertheless, increased utilization of biomarkers such as lignin that have unique degradation contrasts between oxic and anoxic environments could be critical in evaluating and predicting the success of future wetland restoration.
Data Availability Statement
All datasets presented in this study are included in the article/Supplementary Material.
Author Contributions
PH, RM, and BB made substantial contributions to the work conception and design. RM orchestrated all sampling. PH, RM, RD, and BB contributed to the acquisition, analysis, or interpretation of data for the work. PH, RM, and BB were responsible for drafting and critical revision of the manuscript for important intellectual content. PH, RM, RD, and BB provided approval for the publication of the content. PH, RM, RD, and BB agreed to be accountable for all aspects of the work in ensuring that questions related to the accuracy or integrity of any part of the work are appropriately investigated and resolved. All authors contributed to the article and approved the submitted version.
Funding
This work was funded by the California Department of Water Resources (RM), U.S. Geological Survey (BB), a Cooperative Ecosystem Studies Units grant through U.S. Geological Survey (PH), and the National Science Foundation grant OCE-1335622 (PH).
Conflict of Interest
The authors declare that the research was conducted in the absence of any commercial or financial relationships that could be construed as a potential conflict of interest.
Acknowledgments
We thank Lauren Hastings for her role in getting the project started. We thank all those who contributed to maintenance at the restored wetland and aided in all aspects of the field deployment, sampling, and sample processing. We thank the associate editor, the reviewers, and Stephen Opsahl for their constructive criticism. Any use of trade, firm, or product names is for descriptive purposes only and does not imply endorsement by the U.S. Government.
Supplementary Material
The Supplementary Material for this article can be found online at: https://www.frontiersin.org/articles/10.3389/fenvs.2020.564603/full#supplementary-material
Abbreviations
(Ad:Al)v, ratio of lignin vanillyl phenol acids to aldehydes; (Ad:Al)s, ratio of lignin syringyl phenol acids to aldehydes; S:V, ratio of syringyl to vanillyl lignin phenols; C:V, ratio of cinnamyl to vanillyl lignin phenols; DOC, dissolved organic carbon; POC, particulate organic carbon; POM, particulate organic matter; OM, organic matter; Σ8, sum of eight particulate lignin phenol concentrations; Λ8, lignin carbon normalized yields.
References
Allison, M. A., Demas, C. R., Ebersole, B. A., Kleiss, B. A., Little, C. D., Meselhe, E. A., et al. (2012). A water and sediment budget for the lower Mississippi-Atchafalaya River in flood years 2008-2010: implications for sediment discharge to the oceans and coastal restoration in Louisiana. J. Hydrol. 432, 84–97. doi: 10.1016/j.jhydrol.2012.02.020
Atwater, B. F. (1980). Attempts to Correlate The Late Quaternary Climatic Records Between San Francisco Bay, the Sacramento-San Joaquin Delta, and the Mokelumne. Ph.D dissertation, University of Delaware, Newark, DE.
Bahri, H., Dignac, M. F., Rumpel, C., Rasse, D. P., Chenu, C., and Mariotti, A. (2006). Lignin turnover kinetics in an agricultural soil is monomer specific. Soil Biol. Biochem. 38, 1977–1988. doi: 10.1016/j.soilbio.2006.01.003
Benner, R., Fogel, M. L., and Sprague, E. K. (1991). Diagenesis of belowground biomass of Spartina alterniflora in salt-marsh sediments. Limnol. Oceanogr. 36, 1358–1374. doi: 10.4319/lo.1991.36.7.1358
Bourdon, S., Laggoun-Defarge, F., Disnar, J., Maman, O., Guillet, B., Derenne, S., et al. (2000). Organic matter sources and early diagenetic degradation in a tropical peaty marsh (Tritrivakely, Madagascar). Implications for environmental reconstruction during the Sub-Atlantic. Org. Geochem. 31, 421–438. doi: 10.1016/S0146-6380(00)00010-3
Day, J., Shaffer, G., Britsch, L., Reed, D., Hawes, S., and Cahoon, D. (2000). Pattern and process of land loss in the Mississippi Delta: a spatial and temporal analysis of wetland habitat change. Estuaries 23, 425–438. doi: 10.2307/1353136
Day, J. W., Kemp, G. P., Reed, D. J., Cahoon, D. R., Boumans, R. M., Suhayda, J. M., et al. (2011). Vegetation death and rapid loss of surface elevation in two contrasting Mississippi delta salt marshes: the role of sedimentation, autocompaction and sea-level rise. Ecol. Eng. 37, 229–240. doi: 10.1016/j.ecoleng.2010.11.021
Filley, T. R., Boutton, T. W., Liao, J. D., Jastrow, J. D., and Gamblin, D. E. (2008). Chemical changes to nonaggregated particulate soil organic matter following grassland-to-woodland transition in a subtropical savanna. J. Geophys. Res. Biogeosci. 113:G03009. doi: 10.1029/2007JG000564
Gaiser, E. (2009). Periphyton as an indicator of restoration in the Florida Everglades. Ecol. Indic. 9, S37–S45. doi: 10.1016/j.ecolind.2008.08.004
Haddad, R., Newell, S., Martens, C., and Fallon, R. (1992). Early diagenesis of lignin-associated phenolics in the salt marsh grass Spartina alterniflora. Geochim. Cosmochim. Acta 56, 3751–3764. doi: 10.1016/0016-7037(92)90168-I
Harfmann, J., Kurobe, T., Bergamaschi, B., Teh, S., and Hernes, P. (2019). Plant detritus is selectively consumed by estuarine copepods and can augment their survival. Sci. Rep. 9:9076. doi: 10.1038/s41598-019-45503-6
He, S., Malfatti, S. A., McFarland, J. W., Anderson, F. E., Pati, A., Huntemann, M., et al. (2015). Patterns in wetland microbial community composition and functional gene repertoire associated with methane emissions. mBio 6:e00066-15. doi: 10.1128/mBio.00066-15
Hedges, J. I., Blanchette, R. A., Weliky, K., and Devol, A. H. (1988). Effects of fungal degradation on the CuO oxidation products of lignin: a controlled laboratory study. Geochim. Cosmochim. Acta 52, 2717–2726. doi: 10.1016/0016-7037(88)90040-3
Hedges, J. I., Cowie, G. L., Ertel, J. R., Barbour, R. J., and Hatcher, P. G. (1985). Degradation of carbohydrates and lignins in buried woods. Geochim. Cosmochim. Acta 49, 701–711. doi: 10.1016/0016-7037(85)90165-6
Hedges, J. I., and Ertel, J. R. (1982). Characterization of lignin by gas capillary chromatography of cupric oxide oxidation products. Anal. Chem. 54, 174–178. doi: 10.1021/ac00239a007
Hedges, J. I., and Mann, D. C. (1979). Characterization of plant tissues by their lignin oxidation products. Geochim. Cosmochim. Acta 43, 1803–1807. doi: 10.1016/0016-7037(79)90028-0
Hedges, J. I., and Weliky, K. (1989). Diagenesis of conifer needles in a coastal marine environment. Geochim. Cosmochim. Acta 53, 2659–2673. doi: 10.1016/0016-7037(89)90137-3
Heim, A., and Schmidt, M. W. I. (2007). Lignin turnover in arable soil and grassland analysed with two different labelling approaches. Eur. J. Soil Sci. 58, 599–608. doi: 10.1111/j.1365-2389.2006.00848.x
Hernes, P. J., and Benner, R. (2002). Transport and diagenesis of dissolved and particulate terrigenous organic matter in the North Pacific Ocean. Deep Sea Res. Part Oceanogr. Res. Pap. 49, 2119–2132. doi: 10.1016/s0967-0637(02)00128-0
Hernes, P. J., and Benner, R. (2003). Photochemical and microbial degradation of dissolved lignin phenols: implications for the fate of terrigenous dissolved organic matter in marine environments. J. Geophys. Res. Oceans 108:3291. doi: 10.1029/2002JC001421
Hernes, P. J., Robinson, A. C., and Aufdenkampe, A. K. (2007). Fractionation of lignin during leaching and sorption and implications for organic matter “freshness.” Geophys. Res. Lett. 34:L17401. doi: 10.1029/2007GL031017
Hernes, P. J., Spencer, R. G. M., Dyda, R. Y., O’Geen, A. T., and Dahlgren, R. A. (2017). The genesis and exodus of vascular plant DOM from an oak woodland landscape. Front. Earth Sci 5:9. doi: 10.3389/feart.2017.00009
Hernes, P. J., Spencer, R. G. M., Dyda, R. Y., Pellerin, B. A., Bachand, P. A. M., and Bergamaschi, B. A. (2013). DOM composition in an agricultural watershed: assessing patterns and variability in the context of spatial scales. Geochim. Cosmochim. Acta 121, 599–610. doi: 10.1016/j.gca.2013.07.039
Janusz, G., Pawlik, A., Sulej, J., Swiderska-Burek, U., Jarosz-Wilkolazka, A., and Paszczynski, A. (2017). Lignin degradation: microorganisms, enzymes involved, genomes analysis and evolution. FEMS Microbiol. Rev. 41, 941–962. doi: 10.1093/femsre/fux049
Kastner, T. P., and Goni, M. A. (2003). Constancy in the vegetation of the amazon basin during the late pleistocene: evidence from the organic matter composition of amazon deep sea fan sediments. Geology 31, 291–294. doi: 10.1130/0091-7613(2003)031<0291:citvot>2.0.co;2
Matiasek, S. J., and Hernes, P. J. (2019). The chemical fingerprint of solubilized organic matter from eroded soils and sediments. Geochim. Cosmochim. Acta 267, 92-112. doi: 10.1016/j.gca.2019.09.016
Miller, R. L. (2011). Carbon Gas Fluxes in Re-Established Wetlands on Organic Soils Differ Relative to Plant Community and Hydrology. Wetlands 31, 1055–1066. doi: 10.1007/s13157-011-0215-2
Miller, R. L., Fram, M. S., Fujii, R., and Wheeler, G. (2008). Subsidence reversal in a re-established wetland in the Sacramento-San Joaquin Delta, California, USA. San Franc. Estuary Watershed Sci. 6, 20.
Miller, R. L., and Fujii, R. (2010). Plant community, primary productivity, and environmental conditions following wetland re-establishment in the Sacramento-San Joaquin Delta. California. Wetl. Ecol. Manag. 18, 1–16. doi: 10.1007/s11273-009-9143-9
Miller, R. L., and Fujii, R. (2011). “Re-Establishing Marshes Can Turn A Current Carbon Source Into A Carbon Sink In The Sacramento-San Joaquin Delta of California, USA,” in River Deltas: Types, Structures and Ecology. Hauppauge, NY: Nova Science Publishers, Inc, 1–34.
Opsahl, S., and Benner, R. (1993). Decomposition of senescent blades of the seagrass Halodule wrightii in a subtropical lagoon. Mar. Ecol. Prog. Ser. Oldendorf 94, 191–205. doi: 10.3354/meps094191
Opsahl, S., and Benner, R. (1995). Early diagenesis of vascular plant tissues: lignin and cutin decomposition and biogeochemical implications. Geochim. Cosmochim. Acta 59, 4889–4904. doi: 10.1016/0016-7037(95)00348-7
Orem, W. H., and Hatcher, P. G. (1987). Early diagenesis of organic matter in a sawgrass peat from the Everglades. Florida. Int. J. Coal Geol. 8, 33–54. doi: 10.1016/0166-5162(87)90021-8
Pellerin, B. A., Hernes, P. J., Saraceno, J., Spencer, R. G. M., and Bergamaschi, B. A. (2010). Microbial degradation of plant leachate alters lignin phenols and trihalomethane precursors. J. Environ. Qual. 39, 946–954. doi: 10.2134/jeq2009.0487
Schoellhamer, D. H., Wright, S. A., and Drexler, J. Z. (2013). Adjustment of the San Francisco estuary and watershed to decreasing sediment supply in the 20th century. Mar. Geol. 345, 63–71. doi: 10.1016/j.margeo.2013.04.007
Spencer, R. G. M., Aiken, G. R., Dyda, R. Y., Butler, K. D., Bergamaschi, B. A., and Hernes, P. J. (2010). Comparison of XAD with other dissolved lignin isolation techniques and a compilation of analytical improvements for the analysis of lignin in aquatic settings. Org. Geochem. 41, 445–453. doi: 10.1016/j.orggeochem.2010.02.004
Stumm, W., and Morgan, J. J. (1981). Aquatic Chemistry: An Introduction Emphasizing Chemical Equilibria In Natural Waters. New York, NY: Wiley.
Syvitski, J. (2003). Supply and flux of sediment along hydrological pathways: research for the 21st century. Glob. Planet. Change 39, 1–11. doi: 10.1016/S0921-8181(03)00008-0
Utz, I. J. (2016). Determining the Contribution of Aquatic Autotrophs to the Carbon Budgets of Restored Wetlands in the Sacramento-San Joaquin River Delta. B.S. thesis, University of California, Berkeley, CA.
Williams, C., Yavitt, J., Wieder, R., and Cleavitt, N. (1998). Cupric oxide oxidation products of northern peat and peat-forming plants. Can. J. Bot. 76, 51–62. doi: 10.1139/b97-150
Zaccone, C., Said-Pullicino, D., Gigliotti, G., and Miano, T. M. (2008). Diagenetic trends in the phenolic constituents of Sphagnum-dominated peat and its corresponding humic acid fraction. Org. Geochem. 39, 830–838. doi: 10.1016/j.orggeochem.2008.04.018
Zhang, X., Bianchi, T. S., Cohen, M. J., Martin, J. B., Quintero, C. J., Brown, A. L., et al. (2019). Initiation and development of wetlands in southern florida karst landscape associated with accumulation of organic matter and vegetation evolution. J. Geophys. Res. Biogeosci. 124, 1604–1617. doi: 10.1029/2018JG004921
Keywords: lignin biomarkers, wetland restoration, litterbag, cattail, tule, decomposition
Citation: Hernes PJ, Miller RL, Dyda RY and Bergamaschi BA (2020) Vegetation vs. Anoxic Controls on Degradation of Plant Litter in a Restored Wetland. Front. Environ. Sci. 8:564603. doi: 10.3389/fenvs.2020.564603
Received: 22 May 2020; Accepted: 14 September 2020;
Published: 08 October 2020.
Edited by:
Jiang Helong, Nanjing Institute of Geography and Limnology (CAS), ChinaReviewed by:
Huacheng Xu, Nanjing Institute of Geography and Limnology (CAS), ChinaBaoli Wang, Tianjin University, China
Copyright © 2020 Hernes, Miller, Dyda and Bergamaschi. This is an open-access article distributed under the terms of the Creative Commons Attribution License (CC BY). The use, distribution or reproduction in other forums is permitted, provided the original author(s) and the copyright owner(s) are credited and that the original publication in this journal is cited, in accordance with accepted academic practice. No use, distribution or reproduction is permitted which does not comply with these terms.
*Correspondence: Peter J. Hernes, cGpoZXJuZXNAdWNkYXZpcy5lZHU=