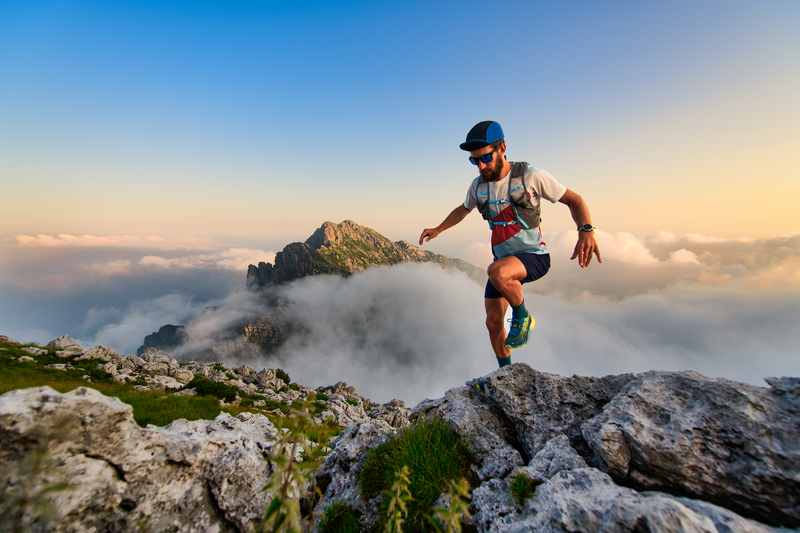
95% of researchers rate our articles as excellent or good
Learn more about the work of our research integrity team to safeguard the quality of each article we publish.
Find out more
ORIGINAL RESEARCH article
Front. Environ. Sci. , 25 January 2021
Sec. Conservation and Restoration Ecology
Volume 8 - 2020 | https://doi.org/10.3389/fenvs.2020.563431
This article is part of the Research Topic Environmental DNA Innovations for Conservation View all 19 articles
Recent research on environmental DNA (eDNA), genetic material shed by organisms into their environment that can be used for sensitive and species-specific detection, has focused on the ability to collect airborne eDNA released by plants and carried by the wind for use in terrestrial plant populations, including detection of invasive and endangered species. Another possible application of airborne eDNA is to detect changes in plant communities in response to activity or changes on a landscape-scale. Therefore, the goal of this study was to demonstrate how honey mesquite, blue grama, and general plant airborne eDNA changes in response to human activity on a landscape-scale. We monitored airborne eDNA before, during, and after a rangeland restoration effort that included honey mesquite removal. As expected, restoration activity resulted in a massive increase in airborne honey mesquite eDNA. However, we also observed changes in abundance of airborne eDNA from the grass genus Bouteloua, which was not directly associated with the restoration project, and we attribute these changes to both human activity and seasonal trends. Overall, we demonstrate for the first time that activity and changes on a landscape-scale can be tracked using airborne eDNA collection, and we suggest that airborne eDNA has the potential to help monitor and assess ecological restoration projects, track changes due to global warming, or investigate community changes in response to encroachment by invasive species or extirpation of threatened and endangered species.
Monitoring is a critical component of successful restoration before, during, and after management actions (Walters 1986; Lake 2001; Galatowitsch 2012). For example, conventional field-based plant community monitoring is generally accomplished via methods such as the line-point intercept, belt transect, and gap intercept, using quadrats, transects, or points (Herrick et al., 2005a; Elzinga et al., 1998). Monitoring methods can provide detailed information about a study site, but they can also be time consuming and withdraw logistical and financial resources from low budget projects. In addition, conventional monitoring activity typically incites elevated disturbance to target areas, which may be counterproductive to restoration, and the results can vary based on the intensity of the sampling (Herrick et al., 2005a; Herrick et al., 2005b; Garrard et al., 2008).
A novel method that could address the limitations of conventional monitoring relies upon collection and analysis of environment DNA (eDNA), the genetic material shed by an organism into its environment and collected by researchers from environmental samples such as soil, water, or air (Thomsen et al., 2012; Barnes and Turner 2016). A primary benefit of eDNA monitoring is the ability to detect organisms without the need of a captured target species or direct tissue sample. Indeed, recent reviews by Ruppert et al. (2019) and Makiola et al. (2020) have predicted an increasingly large role for eDNA analysis and metabarcoding in community surveillance and monitoring landscape changes in response to activities such as ecological restoration.
Research into eDNA has focused primarily on aquatic and sediment samples, including assessments on invasive species detection, endangered species monitoring, and the ecology of eDNA (Willerslev et al., 2007; Goldberg et al., 2011; Lodge et al., 2012; Taberlet et al., 2012; Barnes et al., 2014; Barnes and Turner 2016). Recently, Johnson et al. (2019a) demonstrated that airborne eDNA samples, genetic material collected from air, could detect both anemophilous and non-anemophilous plant species. These findings portend the utility of airborne eDNA analysis for broad conservation, management, and research applications such as whole plant community monitoring. However, the ecology of airborne eDNA, including spatial and temporal dynamics of airborne eDNA abundance relative to seasonal changes and other activity on the landscape, remain unexplored but represent critical gaps in current understanding that should be addressed before the potential management and conservation impact of airborne eDNA monitoring can be maximized.
Like eDNA research in general, the existing research addressing how eDNA responds to biotic and abiotic landscape changes primarily comes from aquatic systems. For example, Bista et al. (2017) found that macroinvertebrate eDNA levels displayed community level shifts in diversity throughout the year due to changes in species ecology, biotic, and abiotic factors. Additionally, Buxton et al. (2018) found, when examining eDNA of the great crested newt (Triturus cristatus) in aquatic and sediment samples, that detection varied throughout the year based on habitat suitability and species ecology. Work has also been done in ocean systems with studies ranging from examining shark diversity response to anthropogenic disturbance to understanding how anthropogenic activities such as oil spills and development impact micro and macro coastal communities (Bakker et al., 2017; Xie et al., 2018; DiBattista et al., 2020). Sun et al. (2019) used eDNA metabarcoding to understand Dipteria and other organism populations in human caused roadside stormwater ponds. Additionally, Klymus et al. (2017) examined how anthropogenic uranium containment ponds impacted the biodiversity of vertebrate species. Early applications also include monitoring of the re-introduction of Rhine sculpin (Cottus rhenanus) into its native range (Hempel et al., 2020) and at-risk, pre-restoration coral community monitoring (Nichols and Marko 2019).
Despite the apparent focus on aquatic systems to date, similar eDNA applications will likely also benefit conservation and management in terrestrial habitats. For example, airborne eDNA analysis could be used to track the changes in plant community composition throughout a restoration project and afterward to provide information for robust adaptive restoration. Airborne eDNA analysis could also assess the impacts of climate change, assist in identifying the spread and location of rangeland invasive species, track endangered species, and detect disease within a restored site or before a restoration (Dejean et al., 2012; Huver et al., 2015; Scriver et al., 2015; Barnes and Turner 2016). With the use of airborne eDNA, tedious surveys that require large amounts of time could be replaced by a network of airborne eDNA traps. However, a critical first step in the evaluation of such methods is to examine the extent to which airborne eDNA reflects landscape changes. Human activity on a landscape-scale encompasses a large variety of activities that could influence airborne eDNA dynamics, from building roads, farming, construction, habitat fragmentation, and invasive species introduction to name a few. We believe that airborne eDNA (both species specific and global) can be used reflect landscape-scale changes from human activity.
In our study, we used ecological restoration as one example of human activity on the landscape. Our goal was to demonstrate the use of airborne eDNA as a surveillance tool during removal of honey mesquite (Prosopis glandulosa) on the Texas Tech University native short-grass prairie. Specifically, we examined whether airborne eDNA changed in response to activity on the landscape by: 1) tracking the removal of honey mesquite during a rangeland restoration project; and 2) monitoring changes within the plant community using the Bouteloua genus and global plant eDNA as surrogates. The results of this work will help quantify the feasibility of using airborne eDNA to monitor human activities such as restoration and other landscape management.
The 130-acre Texas Tech University Native Rangeland (33.60327 N, −101.9003 W) acts as a natural area for teaching and research within the Department of Natural Resources Management (Figure 1). The site consists of short-grass prairie, with a large variety of bunchgrasses, forbs, and cacti, and a large population of honey mesquite due to the suppression of fire and grazing (Ansley et al., 2001). This site, despite being fragmented and isolated within an urban matrix, represents a native short-grass prairie in post-climax seral stage, and has not been disked, plowed, or reseeded.
FIGURE 1. The Texas Tech University Native Rangeland (Lubbock County, TX, United States) study site where the restoration and airborne eDNA sampling took place. The hatched lines show the two different restoration methods used and the clusters of points represent the three groupings of airborne eDNA Big Spring Number Eight Dust Traps.
A rangeland restoration project was performed by the Texas Tech Student Chapter of the Wildlife Society, students from the NRM 4309: Range Wildlife Habitat course, and Texas A&M Forest Service in November and December 2017. During the restoration, honey mesquite, a thorny shrub/tree that can re-sprout and form multi-stem thickets (Ansley et al., 1997), was targeted for removal due to its negative impact on forage production, grazing, and local grass biodiversity (Mohamed et al., 2011). The project was completed in two treatments, each lasting 3 days. The first, 1.2-hectare treatment began on November 18, 2017. This treatment consisted of cutting off the entirety of the honey mesquite aboveground biomass (i.e., main stem and adjacent minor stems), and then chipping the cut material on site and treating the stump with 25% Triclopyr and 75% diesel with a 1% blue marker dye. This first effort was completed on the eastern side of our study site (Figure 1). The second, 1.4-hectare treatment began on December 2, 2017 and was changed to a reduced treatment due to logistical constraints, where only the larger mesquite trees (i.e., main and adjacent minor stems) were targeted and removed. As with the previous treatment, the cut material was chipped, and the stump was treated with the same herbicide mix. This reduced treatment occurred directly west and adjacent to the first total treatment (Figure 1). Both treatments attempted to kill the sprout buds of the underground main stem with both cutting and herbicide (Fisher, 1950).
To examine how airborne eDNA responds to human activity on a landscape-scale, we collected airborne eDNA before, during, and after the restoration project. To collect airborne eDNA, we set up three eDNA sampling locations: one south of the restoration taking place (“restoration traps”), one plot along the northwest edge of the study site (“north traps”) and one plot to the east of the restoration treatments (“east traps”; Figure 1). Within each sampling location, three Big Spring Number Eight (BSNE) passive dust traps were deployed. Johnson et al. (2019b) demonstrated that BSNE traps performed well compared to other passive dust traps. The BSNE traps (Figure 2) consisted of two triangular traps 0.914 and 0.406 m above the ground. Each trap has an opening at the tip of the triangular piece of metal and a metal mesh vent on top, allowing air to enter the through the opening and flow out through the metal mesh on top, depositing carried material into a collection tray below (Zobeck 2006). Each triangular trap is attached to a metal sheet that acts as sail to consistently orient them into the wind to maximize the amount of material collected.
FIGURE 2. The Big Spring Number Eight dust traps that were used to collect airborne eDNA in this experiment.
Traps were sampled four times between November 3 and December 8, 2017. Sampling Events I (November 10th) and II (November 17th) took place 8 and 1 day before the first total treatment restoration, respectively. Event III (November 27th) occurred 9 days after the first total treatment restoration, and Event IV (December 8th) occurred 6 days after the reduced treatment restoration. The collection of repeated samples through time allowed us to examine the response in airborne eDNA to activity associated with the restoration effort. At each sampling event, each trap was rinsed with approximately 1 L deionized water, and the water was collected into individual, sterile 1 L bottles. Since the BSNE arrays each have two collection traps, each tray at a single trap was washed and combined into a single water sample to avoid pseudoreplication (Hurlbert 1984). Rinse water samples were then transported to the laboratory within a cooler and vacuum filtered with 1 µm Isopore membrane filters. Filters were stored at −20 °C for approximately 1 month. Next, DNA extractions were completed using a DNeasy PowerPlant Pro DNA Isolation Kit, which has demonstrated high efficiency in previous airborne eDNA analyses (Johnson et al., 2019b). We followed the manufacturer’s protocol, except we added an extra grinding step with a sterile plastic pestle and frequent vortex agitation to ensure homogenization (Johnson et al., 2019b). Extracted genomic DNA was stored at −20 °C until analysis (approximately 6 months later). To confirm that there was no contamination throughout this process, extraction blanks (i.e., sterile samples extracted alongside experimental samples as negative controls with just buffer and no filter) were processed with every extraction event. Additionally, we used sterile containers, bleached all laboratory surfaces, and used sterile gloves. Due to the nature of airborne eDNA, we could not develop confident field or filtration controls (i.e., we have not developed a method in which control samples are not exposed to the air representing our sample). As a result, we have only included extraction and PCR negative controls (“blanks”).
To broadly characterize changes in airborne eDNA in response to the restoration activity, we used three different quantitative polymerase chain reaction (qPCR) assays: a honey mesquite species-specific primer, a Bouteloua genus assay, and a global plant primer. Species- and genus-specificity for honey mesquite and Bouteloua assays, respectively, were confirmed in silico using NCBI Primer-BLAST (Ye et al., 2012) as well as in-lab PCR confirmation using tissues of the nine most common plants found within our study site. We quantified both the limits of detection and quantification as the lowest amount of DNA our primers detect any of the technical replicates and where all the technical replicates were detected, respectively. Operationally, we followed the recommendations of low-copy qPCR analysis of Ellison et al. (2006) and assigned all non-detections a value of zero rather than omitting them from the analysis or missing out on information provided by samples in which fewer than all technical replicates amplified. We previously observed high rates of PCR inhibition in airborne eDNA samples (Johnson et al., 2019a; Johnson et al., 2019b), which led us to dilute samples in this study by 1:10 with pure water. All qPCR reactions were completed on a QuantStudio 3 Real-Time qPCR machine (ThermoFisher Scientific, Foster City, California). The honey mesquite assay targeted the focal species of the restoration effort using 25 µl reactions with 1x PowerSYBR Green qPCR Master Mix, 1 µM forward and reverse primer (Johnson et al., 2019a; Table 1) 2 µl diluted genomic DNA template. The thermocycling program for the honey mesquite assay began with an initial 95 °C step for 10 min followed by 40 cycles of 15 s at 95 °C and 1 min at 70.1 °C, and a final melt curve analysis. The Bouteloua genus assay targeted grasses that represents the most common wind pollinated species on our study site. Each 25 µl qPCR reaction contained 1x PowerSYBR Green qPCR Master Mix, 1 µM forward and reverse primer (Johnson et al., 2019a; Table 1), and 2 µl diluted genomic DNA template. The thermocycling program used an initial 95 °C step for 10 min followed by 40 cycles of 15 s at 95 °C and 1 min at 66 °C, and a final melt curve analysis. Reactions in the honey mesquite and Bouteloua genus assays were quantified using five-point standard curves based on a 1:10 serial dilution of tissue-derived DNA from honey mesquite and Bouteloua gracilis, respectively. Non-detections were treated as zeros when averaged with other technical replicates (Ellison et al., 2006). For both assays, samples were run in triplicate and non-template controls were included to ensure no contamination occurred.
TABLE 1. The three different primer sets (forward and reverse) used over the course of this experiment. The honey mesquite and Bouteloua genus assays were produced by Johnson et al., 2019a. The trnL global chloroplast was taken from Taberlet et al. (2007).
Finally, as an indicator of changes in the overall plant community beyond the two focal groups, honey mesquite and Bouteloua spp., we amplified all plant eDNA in our samples with a global plant assay targeting the chloroplast trnL gene (Taberlet et al., 2007; Table 1). For this assay, each 25-µl qPCR reaction contained 1x PowerSYBR Green qPCR Master Mix, 1 µM forward and reverse primer concentrations, and 2 µl diluted genomic DNA template. The thermocycling program began with an initial 95 °C step for 10 min followed by 32 cycles of 2 min at 94 °C, 1 min at 55 °C, and 30 s at 72 °C, and a final extension at 72 °C for 2 min (Craine et al., 2016). Since amplification with the global plant assay could be the result of a variable mix of plant eDNA sources, we could not create a standard curve for quantification. Therefore, rather than absolute quantification of eDNA concentration in each reaction, we relied on comparison of the average number of cycles needed for the samples to display enough fluorescence to be considered positive (cycle threshold, determined using the default settings of the QuantStudio three Real-Time qPCR machine and abbreviated CT; Heid et al., 1996).
To analyze whether airborne eDNA changed in response to restoration activity, we completed three separate repeated measures analyses of variance (rmANOVAs) with IBM SPSS statistics (IBM Corp. 2017), separately analyzing honey mesquite, Bouteloua genus, and global plant eDNA results. In each analysis, sampling units consisted of nine total BSNE traps, and three replicate traps at each plot were combined into experimental units to make comparisons between locations. Sampling event represented the repeated measure in our experimental design, we interpreted Wilks’ Lambda as our test statistic, and we assumed α = 0.05 for determination of statistical significance. Following significant rmANOVAs, we used Tukey-Kramer post-hoc tests to examine how the different trap locations varied from one another for each sampling event.
When collecting airborne eDNA, it is common to see very small leaf fragments in the samples; however, occasionally large plant leaves can be collected which in turn results in extremely high amounts of target DNA in the traps. While these detections are real, the extremely large amounts of airborne eDNA may obfuscate underlying trends or patterns. Therefore, we removed two outliers from consideration: one from the global eDNA assay during Event III in the east traps (>100x more eDNA than the other two traps at the same site), and the other from the honey mesquite assay from Event III, also in the east traps (600x more eDNA than replicate traps).
We found during Events I and II that the average honey mesquite eDNA quantities for all three trap locations were consistently low (Table 2). After the total restoration treatment, the average quantity of honey mesquite eDNA spiked in the restoration traps but remained low in the east and undetected in north traps (Table 2). After Event IV, we observed honey mesquite eDNA in high concentrations in the restoration traps with low concentrations for the east traps and no detections for the north traps (Table 2). Overall, when comparing the amounts of airborne eDNA for each of our three sampling locations, eDNA significantly differed between trap locations (rmANOVA F3,19 = 7.36, p = 0.0018; Figure 3). Pairwise comparisons revealed that for Events I and II, the east traps were significantly different from both the restoration (p = 0.0052 and p < 0.0001, respectively) and north trap locations (p = 0.0052 and p < 0.0001), but the north and restoration traps were not significantly different from each other for either Event (Table 3). However, after the first restoration treatment (Event III), the restoration traps were significantly different from both the north traps (p < 0.0001) and the east traps (p < 0.0001), and the north and east traps were not significantly different (p = 0.98; Table 3). Lastly, during Event IV, the restoration traps were again significantly different from both the north (p < 0.0001) and east (p < 0.0001) trap locations while the north and east traps did not significantly differ from one another (p = 0.996; Table 3). Amplification percentage (i.e., number of samples displaying any amplification, regardless of eDNA quantification) for Events III and IV showed a large spike for the restoration traps, rising from 33% to 100% for both Events III and IV (Figure 4A). This spike was mirrored slightly in the east traps but not in the north traps.
TABLE 2. The amounts of honey mesquite, Bouteloua genus, and global plant airborne eDNA collected for each trap location and all four sampling events. The honey mesquite and Bouteloua genus units are mean concentration ± standard deviation. The global plant eDNA shows the mean cycle threshold ± standard deviation. The complete raw results can be found in the Supplementary Table.
FIGURE 3. The quantity of honey mesquite eDNA for all three trap locations over four sampling Events. Honey mesquite eDNA increased following restoration activity, especially within the restoration site.
TABLE 3. The p values for the Tukey-Kramer post-hoc comparison tests for each event examining the honey mesquite, Bouteloua genus and global plant eDNA.
FIGURE 4. The percentage of significant amplification/detections for the traps from all three sampling locations (East, North, and Restoration). The primers focused on honey mesquite airborne eDNA (A), Bouteloua airborne eDNA (B), and global plant airborne eDNA (C).
Across all honey mesquite qPCR plates, negative controls failed to amplify as expected, and qPCR efficiencies were on average 67% with an average R-squared of 0.99. The limit of detection and limit of quantification for the honey mesquite primer were both 3.8 × 10−4 ng/μL.
The quantity of Bouteloua airborne eDNA changed over time as well. At Event I the quantity of Bouteloua varied greatly between trap locations, with the east traps having a larger quantity than the restoration and north traps. Between Events I and II the amount of Bouteloua eDNA declined (Table 2). After the first full treatment restoration event, increases in the quantity of Bouteloua DNA were detected in the east and northern traps while the restoration traps stayed the same. After the reduced treatment, there were no Bouteloua detections other than by the restoration traps (Table 2). Across all four Events, we observed significant differences in the amount of Bouteloua eDNA between each trap location (rmANOVA F3,22 = 329.85, p < 0.0001; Figure 5). At Event I the east traps were significantly different from both the restoration (p = 0.0069) and north (p = 0.0198) traps, whereas the restoration and north traps did not significantly differ (p = 0.8934; Table 3). At Event II, none of the trap locations significantly differed from one another (Table 3). At Event III, the east traps were significantly different from both restoration (p < 0.0001) and north (p < 0.0001) traps, while the north and restoration traps did not differ from one another (Table 3). Lastly, at Event IV, the restoration traps were significantly different from both the east (p = 0.0319) and north (p = 0.0319) traps, while the east and north traps were not significantly different (Table 3). Bouteloua amplification percentages were consistently 66% or higher in Events I and II, and Event III notably had 100% amplification for all groups (Figure 4B). At Event IV, we only observed Bouteloua amplification in restoration traps (Figure 4B).
FIGURE 5. The quantity of Bouteloua genus eDNA collected for all three trap locations over four sampling Events. Bouteloua genus eDNA concentrations generally decreased over time with notable spikes after the restoration treatments.
Across all Bouteloua qPCR plates, negative controls failed to amplify as expected, and qPCR efficiencies were an average of 83% with an average R-squared of 0.99. The Bouteloua genus primer’s limit of detection and limit of quantification were both 6.7 × 10−6 ng/μl.
For the first two sampling events, the average cycle threshold (CT; note that decreasing CT values indicate increasing eDNA concentrations) for all three trap locations were consistent and averaged between approximately 29.1 and 30.1 cycles (Table 2). After the total treatment restoration, all three traps detected more global plant eDNA with a large spike in the amount of restoration trap airborne eDNA. Lastly, the average CT values for the samples taken after the reduced treatments were lower compared to those in Event III (Table 2). We found a significant effect of sampling Event on the amount of global eDNA (rmANOVA F3,19 = 8,158.88, p < 0.0001; Figure 6). Pairwise analyses revealed that at Event I, none of the sampling events were significantly different from each other (Table 3). At Event II, the restoration traps were significantly different from both the east (p = 0.0142) and north (p < 0.0001), while the north and east traps also differed significantly (p = 0.0251; Table 3). At Event III, following the first restoration, the restoration traps were significantly different from both the north (p < 0.0001) and east (p < 0.0001) traps, while the north and east traps did not differ from one another (Table 3). Finally, at Event IV, the restoration traps were again significantly different from both the north (p < 0.0001) and east (p < 0.0001) traps, and the north and east traps remained non-significantly different (Table 3). All three trap locations amplified global plant airborne eDNA 100% of the time across all four events (Figure 4C). Across all global plant qPCR plates, negative controls failed to amplify, as expected.
FIGURE 6. The global plant eDNA collected for all three trap locations for all four sampling Events. The global plant eDNA is measured as cycle thresholds (CT) where decreasing values indicate more eDNA. Global plant eDNA increased in response to restoration activity.
Using three different qPCR assays targeting honey mesquite, Bouteloua genus, and a global plant chloroplast gene, we demonstrated that airborne eDNA is affected by human activity during a restoration event, and we argue that these changes track intuitively through time with different stages of the restoration. Notably, we found that airborne eDNA from species that were not even the target of the restoration changed over time. Collectively, our observations demonstrate that airborne eDNA reflects human activity and phenological changes on a landscape-scale and point to an expanding role that airborne eDNA surveillance may play in terrestrial conservation.
Since the restoration focused on the removal of honey mesquite, we first quantified how the amount of honey mesquite changed over the course of the restoration. We found that there was a significant difference between the restoration eDNA traps and the east and north traps. Before the total treatment restoration took place, mesquite eDNA quantity and detection was low. It is useful to consider the ecology of honey mesquite to put these results into context. Honey mesquite is insect-pollinated and flowers in the spring before losing its leaves and going dormant for the winter months (Lopez-Portillo et al., 1993; Golubov et al., 1999). At the time of our restoration effort, honey mesquite was inactive with most of their leaves gone and no flowering or pollination occurring. Accordingly, a “typical” paucity of airborne honey mesquite eDNA was illustrated by the low concentrations and detection percentages for this species in our first two sampling Events (Figure 3 and Figure 4A). However, after the first restoration event, Event III demonstrated a large increase in the concentration of honey mesquite airborne eDNA for the restoration traps. A spike in airborne eDNA occurred again at Event IV but was not as large, likely because the second restoration was farther away and lower intensity. Together, these results demonstrate the potential for airborne eDNA analysis to distinguish between different types or intensities of activity on a landscape-scale. Additionally, airborne eDNA analysis may reveal spatial information. The amplification percentage of honey mesquite detected for each restoration group trap jumped to 100% for both Events III and IV (Figure 4A). We simultaneously observed amplification percentage in east traps (i.e., away from the site of the restoration activity), though not in as high concentrations as shown in the restoration traps, increase after the restorations, suggesting that honey mesquite eDNA traveled downwind to the east trap grouping.
On the other hand, the concentration of Bouteloua airborne eDNA, which was not the target of restoration activities, also changed over time. In general, Bouteloua eDNA decreased throughout our study. Of the four Bouteloua species on our study site - blue grama (Bouteloua gracilis), buffalo grass (Bouteloua dactyloides), sideoats grama (Bouteloua curtipendula), and sixweeks grama (Bouteloua barbata) - blue grama is by far the most common species. The steady decreasing trend that was observed corresponds to the ecology of blue grama, which is summer-active, releases pollen in early fall, and then goes dormant for the winter months (Riegal 1941; Anderson 2003). Johnson et al. (2019a) monitored the changes in Bouteloua genus eDNA for the early fall and showed there was a spike in Bouteloua airborne eDNA associated with their early fall pollination event. However, the results in the present study track the opposite direction and appear to document that Bouteloua airborne eDNA concentrations decline in alignment with approaching winter dormancy.
Therefore, if not impacted, Bouteloua airborne eDNA would likely have decreased uniformly throughout the study period. This trend was observed for the first two sampling events, especially in the eastern traps where large amounts of blue grama grow. However, after the first total treatment restoration we observed a modest increase in Bouteloua airborne eDNA concentrations during Event III. To give further evidence that Bouteloua airborne eDNA was impacted, we can examine the concentrations in conjunction with the percentage of traps that significantly detected Bouteloua DNA (Figure 4B and Figure 5). In addition to the increase in Bouteloua eDNA concentration after the total treatment, we saw each trap location detect Bouteloua eDNA 100% of the time, which should be unlikely to occur naturally since blue grama is becoming dormant for the year at the time of this study. For Event IV, the more limited restoration activity did not promote Bouteloua detection in either north or east trap locations, but we observed 67% amplification at the restoration trap location. Again, this pattern points to a spatial interpretation of airborne eDNA results, with restoration traps closest to the restoration activity demonstrating impacts from the reduced treatment and farther east and north traps remaining unaffected.
We observed that Bouteloua eDNA appeared to impact all three trap locations whereas honey mesquite eDNA, the subject of the restoration, impacted only the restoration traps and moderately the eastern traps. This could be a result of the eDNA content being released into the air. The Bouteloua DNA is assumed to come primarily from blue grama which is at the tail end of its pollination season. Any disruption would result in a plume of leftover pollen being released. Bouteloua pollen is designed to travel on the wind so it would be logical for it to travel greater distances and effect the eastern and northern trap groupings. The honey mesquite on the other hand, is insect pollinated and had sparse leaves available when the restoration took place. As a result, the DNA being released into the air was most likely living wood fragments, cells, and a small amount of leaf material. This type of DNA is not designed to travel on the wind, so only seeing a spike at the closest trap site and slight increases in detection percentage elsewhere would make sense.
Finally, we observed a significant change in the global plant airborne eDNA in our traps closest to the restoration treatments over time. In this study, the global plant airborne eDNA acts as a qualitative surrogate for the plant community. Species specific primers are still rare for most plant species so without a metabarcoding approach, global plant eDNA allows us to monitor general patterns across all plants in addition to focusing on single species (Wallinger et al., 2012). For the first two Events, the global assay showed consistent levels of airborne eDNA across all three sites. After the total treatment restoration occurred, there was a large spike in restoration trap site eDNA and smaller increases in both the north and east traps. After the limited restoration activity, the restoration trap site showed a higher amount of airborne eDNA compared to the other two trap locations, which were unaffected. As shown in the Bouteloua analysis, the amount of Bouteloua eDNA (the most common genus on the landscape) was steadily dropping over the study period which is reflected by the east and north traps showing less global eDNA amounts. This again points to spatial information contained within airborne eDNA analyses as well as the fact that airborne eDNA analysis can distinguish between different activity intensities.
Overall, we have shown that airborne eDNA can assist with the evaluation of current species on a landscape-scale, and that airborne eDNA reflects human activity and seasonal changes on a landscape-scale. In a conservation or management setting, we believe airborne eDNA analysis can aid site selection and monitoring, which will prove especially valuable if it can supplement or even provide an alternative to time-consuming and potentially disruptive conventional plant community surveys. To maximize the conservation potential of airborne eDNA analysis, further examination of the ability of airborne eDNA analysis to detect rare species on a landscape-scale is warranted. Furthermore, combination of airborne eDNA analysis and metabarcoding approaches could allow airborne eDNA to act as a plant community monitoring tool, further increasing its utility for conservation, management, and research.
The original contributions presented in the study are included in the article/Supplementary Material, further inquiries can be directed to the corresponding author.
MJ designed the research, collected and analyzed the data, and wrote the manuscript. RC collected the data and commented on the manuscript. BG performed the restoration, analyzed the data, and commented on the manuscript. DL performed the restoration and commented on the manuscript. MB designed the research, collected and analyzed the data, commented on the manuscript, and provided funding.
This work was funded by Texas Tech University as startup funding to MB.
The authors declare that the research was conducted in the absence of any commercial or financial relationships that could be construed as a potential conflict of interest.
Peter Dotray, Karin and Ardon-Dryer provided guidance as members of MJ’s doctoral dissertation committee. Robert Scott Van Pelt provided the BSNE dust collector traps from the United States Department of Agriculture. Elizabeth Roesler, Sadie Roth, Hayden Hays, Kristin Kabat, and two anonymous reviewers provided comments on an earlier draft of this manuscript. Use of product names within this manuscript does not constitute endorsement.
The Supplementary Material for this article can be found online at: https://www.frontiersin.org/articles/10.3389/fenvs.2020.563431/full#supplementary-material.
Anderson, M. (2003). Bouteloua gracilis. Fire effects information system. Washington, DC: U.S. Department of Agriculture, Forest Service, Rocky Mountain Research Station, Fire Science Laboratory.
Ansley, R. J., Huddle, J. A., and Kramp, B. A. (1997). Mesquite ecology. Vernon, Tx: Texas A&M Agrilife Extension, Texas Agricultural Experiment Station.
Ansley, R. J., Wu, B., and Kramp, B. A. (2001). Observation: long-term increases in mesquite canopy cover in a North Texas savanna. J. Range Manag. 54, 171–176. doi:10.2307/4003179
Bakker, J., Wangensteen, O. S., Chapman, D. D., Boussarie, G., Buddo, D., Guttridge, T. L., et al. (2017). Environmental DNA reveals tropical shark diversity in contrasting levels of anthropogenic impact. Sci. Rep. 7, 16886. doi:10.1038/s41598-017-17150-2
Barnes, M. A., Turner, C. R., Jerde, C. L., Renshaw, M. A., Chadderton, W. L., and Lodge, D. M. (2014). Environmental conditions influence eDNA persistence in aquatic systems. Environ. Sci. Technol. 48, 1819–1827. doi:10.1021/es404734p
Barnes, M. A., and Turner, C. R. (2016). The ecology of environmental DNA and implications for conservation genetics. Conserv. Genet. 17, 1–17. doi:10.1007/s10592-015-0775-4
Bista, I., Carvalho, G. R., Walsh, K., Seymour, M., Hajibabaei, M., Lallias, D., et al. (2017). Annual time-series analysis of aqueous eDNA reveals ecologically relevant dynamics of lake ecosystem biodiversity. Nat. Commun. 8, 14087. doi:10.1038/ncomms14087
Block, W. M., Franklin, A. B., Ward, J. P., Ganey, J. L., and White, G. C. (2001). Design and implementation of monitoring studies to evaluate the success of ecological restoration on wildlife. Restor. Ecol. 9, 293–303. doi:10.1046/j.1526-100x.2001.009003293.x
Buxton, A., Groombridge, J. J., and Griffiths, R. A. (2018). Seasonal variation in environmental DNA detection in sediment and water samples. PloS One 13, e0191737. doi:10.1371/journal.pone.0191737
Clewell, A. F., and Aronson, J. (2013). Ecological restoration. The science and practice of ecological restoration. Washington, DC: Island Press.
Craine, J. M., Barberan, A., Lynch, R. C., Menninger, H. L., Dunn, R. R., and Fierer, N. (2016). Molecular analysis of environmental plant DNA in house dust across the United States. Aerobiologia 33, 71–86. doi:10.1007/s10453-016-9451-5
Dejean, T., Valentini, A., Miquel, C., Taberlet, P., Bellemain, E., and Miaud, C. (2012). Improved detection of an alien invasive species through environmental DNA barcoding: the example of the American bullfrog Lithobates catesbeianus. J. Appl. Ecol. 49, 953–959. doi:10.1111/j.1365-2664.2012.02171.x
DiBattista, J. D., Reimer, J. D., Stat, M., Masucci, G. D., Biondi, P., De Brauwer, M., et al. (2020). Environmental DNA can act as a biodiversity barometer of anthropogenic pressures in coastal ecosystems. Sci. Rep. 10, 8365. doi:10.1038/s41598-020-64858-9
Ellison, S. L., English, C. A., Burns, M., and Keer, J. T. (2006). Routes to improving the reliability of low level DNA analysis using real-time PCR. BMC Biotechnol. 6, 33. doi:10.1186/1472-6750-6-33
Elzinga, C. L., Salzer, D. W., and Willoughby, J. W. (1998). “Measuring and monitoring plant populations,” in USDI-BLM Technical Reference 1730-1. (Denver, CO: USDI-BLM).
Galatowitsch, S. M. (2012). Ecological restoration. Sunderland, mass. Sunderland, MA: Sinauer Associates.
Garrard, G. E., Bekessy, S. A., McCarthy, M. A., and Wintle, B. A. (2008). When have we looked hard enough? A novel method for setting minimum survey effort protocols for flora surveys. Austral. Ecol. 33, 986–998. doi:10.1111/j.1442-9993.2008.01869.x
Goldberg, C. S., Pilliod, D. S., Arkle, R. S., and Waits, L. P. (2011). Molecular detection of vertebrates in stream water: a demonstration using rocky mountain tailed frogs and Idaho giant salamanders. PLoS One 6, e22746. doi:10.1371/journal.pone.0022746
Golubov, J., Mandujano, M. D. C., Franco, M., Montana, C., Eguiarte, L. E., and Lopez-Portillo, J. (1999). Demography of the invasive woody perennial Prosopis glandulosa (honey mesquite). J. Ecol. 87, 955–962. doi:10.1046/j.1365-2745.1999.00420.x
Heid, C. A., Stevens, J., Livak, K. J., and Williams, P. M. (1996). Real time quantitative PCR. Genome Res. 6, 986–994. doi:10.1101/gr.6.10.986
Hempel, C. A., Peinert, B., Beermann, A. J., Elbrecht, V., Macher, J.‐N., Macher, T.-H., et al. (2020). Using environmental DNA to monitor the reintroduction success of the Rhine sculpin (Cottus rhenanus) in a restored stream. Front. Ecol. Evol. 8, 81. doi:10.3389/fevo.2020.0008
Herrick, J. E., Van Zee, J. W., Havstad, K. M., Burkett, L. M., and Whitford, W. G. (2005b). Monitoring manual for grassland, shrubland and savanna ecosystems volume II. Tucson, AZ: USDA-ARS Jornada Experimental Range University of Arizona Press.
Herrick, J. E., Van Zee, J. W., McCord, S. E., Courtright, E. M., Karl, J. W., and Burkett, L. M. (2005a). Monitoring manual for grassland, shrubland and savanna ecosystems volume I. Tucson, AZ: USDA-ARS Jornada Experimental Range University of Arizona Press.
Hurlbert, S. H. (1984). Pseudoreplication and the design of ecological field experiments. Ecol. Monogr. 54, 187–211. doi:10.2307/1942661
Huver, J. R., Koprivnikar, J., Johnson, P. T., and Whyard, S. (2015). Development and application of an eDNA method to detect and quantify a pathogenic parasite in aquatic ecosystems. Ecol. Appl. 25, 991–1002. doi:10.1890/14-1530.1
Johnson, M. D., Cox, R. D., and Barnes, M. A. (2019a). The detection of a non-anemophilous plant species using airborne eDNA. PLoS One 14, e0225262. doi:10.1371/journal.pone.0225262
Johnson, M. D., Cox, R. C., and Barnes, M. A. (2019b). Analyzing airborne environmental DNA: a comparison of extraction methods, primer type, and trap type on the ability to detect airborne eDNA from terrestrial plant communities. Environmental DNA 1, 176–185. doi:10.1002/edn3.19
Klymus, K. E., Richter, C. A., Thompson, N., and Hinck, J. E. (2017). Metabarcoding of environmental DNA samples to explore the use of uranium mine containment ponds as a water source for wildlife. Diversity 9, 54. doi:10.3390/d9040054
Lake, P. S. (2001). On the maturing of restoration: linking ecological research and restoration. Ecol. Manag. Restor. 2, 110–115. doi:10.1046/j.1442-8903.2001.00074.x
Lodge, D. M., Turner, C. R., Jerde, C. L., Barnes, M. A., Chadderton, L., Egan, S. P., et al. (2012). Conservation in a cup of water: estimating biodiversity and population abundance from environmental DNA. Mol. Ecol. 21, 2555–2558. doi:10.1111/j.1365-294X.2012.05600.x
Lodge, D. M., Simonin, P. W., Burgiel, S. W., Keller, R. P., Bossenbroek, J. M., Jerde, C. L., et al. (2016). Risk analysis and bioeconomics of invasive species to inform policy and management. Annu. Rev. Environ. Resour. 41, 453–488. doi:10.1146/annurev-environ-110615-085532
Lopez-Portillo, J., Eguiarte, L., and Montana, C. (1993). Nectarless honey mesquites. Funct. Ecol. 7, 452–461. doi:10.2307/2390032
Makiola, A., Compson, Z. G., Baird, D. J., Barnes, M. A., Boerlijst, S. P., Bouchez, A., et al. (2020). Key questions for next-generation biomonitoring. Frontiers in Environmental Science 7, 197. doi:10.3389/fenvs.2019.00197
Mohamed, A. H., Holecheck, J. L., Bailey, D. W., Campbell, C. L., and DeMers, M. N. (2011). Mesquite encroachment impact on southern New Mexico rangelands: remote sensing and geographic information systems approach. J. Appl. Remote Sens. 5. 3514. doi:10.1117/1.3571040
Nichols, P. K., and Marko, P. B. (2019). Rapid assessment of coral cover from environmental DNA in Hawai’i. Environmental DNA 1, 40–53. doi:10.1002/edn3.8
Pimental, D., Zuniga, R., and Morrison, D. (2005). Update on the environmental and economic costs associated with alien-invasive species in the United States. Ecol. Econ. 52, 273–288. doi:10.1016/j.ecolecon.2004.10.002
Ruppert, K. M., Kline, R. J., and Rahman, M. S. (2019). Past, present, and future perspectives of environmental DNA (eDNA) metabarcoding: a systematic review in methods, monitoring, and applications of global eDNA. Glob. Ecol. Conserv. 17, e00547. doi:10.1016/j.gecco.2019.e00547
Scriver, M., Marinich, A., Wilson, C., and Freeland, J. (2015). Development of species-specific environmental DNA (eDNA) markers for invasive aquatic plants. Aquat. Biol. 122, 27–31. doi:10.1016/j.aquabot.2015.01.003
Sun, Z., Majaneva, M., Sokolova, E., Rauch, S., Meland, S., and Ekrem, T. (2019). DNA metabarcoding adds valuable information for management of biodiversity in roadside stormwater ponds. Ecol. Evol. 9, 9712–9722. doi:10.1002/ece3.5503
Taberlet, P., Coissac, E., Pompanon, F., Gielly, L., Miquel, C., Valentini, A., et al. 2007). Power and limitations of the chloroplast trnL (UAA) intron for plant DNA barcoding. Nucleic Acids Res. 35, e14. doi:10.1093/nar/gkl938
Taberlet, P., Prud'Homme, S., Campione, E., Roy, J., Miquel, C., Shehzad, W., et al. (2012). Soil sampling and isolation of extracellular DNA from large amount of starting material suitable for metabarcoding studies. Mol. Ecol. 21, 1816–1820. doi:10.1111/j.1365-294X.2011.05317.x
Thomas, C. D., Cameron, A., Green, R. E., Bakkenes, M., Beaumont, L. J., Collingham, Y. C., et al. (2004). Extinction risk from climate change. Nature 427, 145–148. doi:10.1038/nature02121
Thomsen, P. F., Kielgast, J., Iversen, L. L., Wiuf, C., Rasmussen, M., Gilbert, M. T., et al. (2012). Monitoring endangered freshwater biodiversity using environmental DNA. Mol. Ecol. 21, 2565–2573. doi:10.1111/j.1365-294X.2011.05418.x
Wallinger, C., Juen, A., Staudacher, K., Schallhart, N., Mitterrutzner, E., Steiner, E. M., et al. 2012). Rapid plant identification using species- and group-specific primers targeting chloroplast DNA. PLoS One 7, e29473. doi:10.1371/journal.pone.0029473
Willerslev, E., Cappellini, E., Boomsma, W., Nielsen, R., Hebsgaard, M., Brand, T. B., et al. (2007). Ancient biomolecules from deep ice cores reveal a forested Southern Greenland. Science 317, 111–114. doi:10.1126/science.1141758
Xie, Y., Zhang, X., Yang, J., Kim, S., Hong, S., Giesy, J. P., et al. (2018). eDNA-based bioassessment of coastal sediments impacted by an oil spill. Environ. Pollut. 238, 739–748. doi:10.1016/j.envpol.2018.02.081
Ye, J., Coulouris, G., Zaretskaya, I., Cutcutache, I., Rozen, S., and Madden, T. (2012). Primer-BLAST: a tool to design target-specific primers for polymerase chain reaction. BMC Bioinf. 13, 134. doi:10.1186/1471-2105-13-134
Zavaleta, E. S., Hobbs, R. J., and Mooney, H. A. (2001). Viewing invasive species removal in a whole-ecosystem context. Trends Ecol. Evol. 16, 454–459. doi:10.1016/S0169-5347(01)02194-2
Keywords: environmental DNA, airborne, human impact, restoration, qPCR
Citation: Johnson MD, Cox RD, Grisham BA, Lucia D and Barnes MA (2021) Airborne eDNA Reflects Human Activity and Seasonal Changes on a Landscape Scale. Front. Environ. Sci. 8:563431. doi: 10.3389/fenvs.2020.563431
Received: 18 May 2020; Accepted: 07 December 2020;
Published: 25 January 2021.
Edited by:
Hideyuki Doi, University of Hyogo, JapanReviewed by:
Kevin Leempoel, Royal Botanic Gardens, Kew, United KingdomCopyright © 2021 Johnson, Cox, Grisham, Lucia and Barnes. This is an open-access article distributed under the terms of the Creative Commons Attribution License (CC BY). The use, distribution or reproduction in other forums is permitted, provided the original author(s) and the copyright owner(s) are credited and that the original publication in this journal is cited, in accordance with accepted academic practice. No use, distribution or reproduction is permitted which does not comply with these terms.
*Correspondence: Mark D. Johnson, bWFyay5qb2huc29uQHR0dS5lZHU=
Disclaimer: All claims expressed in this article are solely those of the authors and do not necessarily represent those of their affiliated organizations, or those of the publisher, the editors and the reviewers. Any product that may be evaluated in this article or claim that may be made by its manufacturer is not guaranteed or endorsed by the publisher.
Research integrity at Frontiers
Learn more about the work of our research integrity team to safeguard the quality of each article we publish.