- Department of Chemistry, Mississippi State University, Starkville, MS, United States
Gasifier Biochar (GBC) and Chitosan-Coated Gasifier Biochar (CGBC) derived from pine wood was used to remove Cu2+ and Cd2+ from water. Chitosan-Coated Gasifier Biochar was made by mixing GBC with aqueous acetic acid chitosan solution followed by treatment with NaOH. Both CGBC and GBC were characterized using FT-IR, scanning electron microscopy, surface area measurement (BET), elemental analysis, thermogravimetric analysis, and point of zero charge. Chitosan accounts for 25% of the weight of the CGBC. Thermogravimetric analysis showed chitosan decomposes sharply at 225–270°C and then more slowly thereafter. The BET surface areas of GBC and CGBC were 34.1 and 4.61 m2/g, respectively. Batch adsorption studies performed at pH values of 2–5 followed Cu2+ and Cd2+ adsorption quantitatively using atomic absorption spectrophotometry. Sorption was evaluated using the Freundlich, Langmuir, and Sips isotherm models. Cu2+ adsorption on CGBC fit best the Sips model (capacity 111.5 mg/g) and Cd2+ with the Langmuir model (capacity 85.8 mg/g). Langmuir adsorption capacities on GBC were 83.7 and 68.6 mg/g for Cu2+ and Cd2+ respectively. CGBC removed more Cu2+(25.8 mg/g) and Cd2+(17.2 mg/g) than GBC because chitosan modification generates amine coordination sites that enhance metal adsorption. Adsorption on CGBC and GBC of both metal ions followed pseudo-second order kinetics.
Introduction
Cadmium and copper ions are toxic and are a concern in the treatment of wastewaters (Fu and Wang, 2011). These metals can enter the environment from metal plating facilities (Kadirvelu et al., 2001), mining operations (Razo et al., 2004), fertilizer plants (Sabiha et al., 2009), paper industries (Nriagu and Pacyna, 1988), batteries (Liu et al., 2014), and some preservatives and pesticides (Mohan et al., 2007). Their toxicity at low concentrations led the World Health Organization (WHO) to set guidelines for maximum amount of Cu2+ and Cd2+ levels in drinking water at 0.003 and 2 ppm, respectively (World Health Organization, 2011). Cadmium exposure will damage kidneys, cause nausea, salivation, cramps, diarrhea, chronic pulmonary problems, and bone decay (Mohan and Singh, 2002). Where copper is handled or stored industrially and can come in contact with storm water, this water must be monitored and remediated before releasing into the environment. Site-specific permits vary, but some locations require Cu2+ concentration to be as low at 5 ppb before it can be legally released. Although copper is an essential nutrient, chronic high-level exposure can cause gastrointestinal distress and other health problems (Stern, 2010).
Many procedures have been developed to remove metals from contaminated water. Industrial techniques include chemical precipitation (Matlock et al., 2002; González-Muñoz et al., 2006; Huisman et al., 2006), ion exchange (Vaaramaa and Lehto, 2003; Dañbrowski et al., 2004), membrane filtration (Blöcher et al., 2003; Qdais and Moussa, 2004), electrochemical treatment (Hunsom et al., 2005), and adsorption (Bailey et al., 1999; Basci et al., 2004). Adsorption is the most widely employed because of its flexibility and reversibility (Fu and Wang, 2011). Activated carbon is the most popular adsorbent for remediating heavy metals, but its high cost has increased interest in novel alternatives (Babel and Kurniawan, 2003). Many low-cost adsorbents have been well studied (Babel and Kurniawan, 2003), including zeolites (Erdem et al., 2004), clays (Bereket et al., 1997), mosses (Brown et al., 2000), algae (Mallick, 2002), coal (Mohan and Gandhimathi, 2009), chitosan (Wan Ngah et al., 2011), and biochar (Mohan et al., 2014). Biochar and chitosan have received recent attention due to their biodegradable and bio-recyclable properties, but their joint use is less known.
Biochar is produced from biomass by three different processes; slow pyrolysis (350–800°C), fast pyrolysis (400–550°C), and gasification (700–1500°C) (Mohan et al., 2014). Pyrolysis biochars have been extensively studied for heavy metal removal while, gasification biochars are much less studied. During gasification at 700–1500°C, solid biomass partially combusts, producing syngas and an ash-like biochar as a byproduct (Mohan et al., 2014). Gasifier biochars (GBC) have higher carbonization and metal oxide (ash) percentages (Brewer et al., 2009).
Chitosan is an aminopolysaccharide biopolymer which is biodegradable, biocompatible, non-toxic and renewable. Therefore, chitosan is widely used as an emerging adsorbent for wastewater treatment (Crini, 2005), chromatographic supports (Muzzarelli and Tubertini, 1969), and enzyme immobilization (Krajewska, 2004). It has several features that make it amenable to environmental applications. Its precursor, chitin is abundant, the second most natural polymer on earth, non-toxic, biocompatible, biodegradable and has antibacterial properties (Ravi Kumar, 2000). Chitin can be extracted from various crustaceous shells such as shrimps, prawns, crabs, and some fungi (Ngah and Isa, 1998). Chitosan is generally prepared by alkali or enzymatic deacetalyzation of chitin consisting of unbranched chains of β-(1-4)-2- acetoamido-2-deoxy-D-glucose. Chitosan and chitosan derived composites have been extensively studied for heavy metal and dye removal as it makes active sites for chelation or adsorption by amine and hydroxyl functional groups. Previously, metal adsorption on chitosan has been studied using hydrogels (Liu et al., 2012), beads (Wan Ngah et al., 2002), and other chitosan-coated supports (Boddu et al., 2008; Popuri et al., 2009).
Biosorbents in their natural form are typically very soft and tend to agglomerate in aqueous solutions or they form gel-like structures that reduce potential commercial applications (Boddu et al., 2003). A solid substrate is often needed to physically support the biosorbent and make it more available for the binding of metal ions. Chitosan deprotonates at high pH. This deprotonation reduces chitosan’s solubility in aqueous media, and the chitosan congeals to form a coating on the substrate surface. Oxalic acid has been found to be a promising linker between chitosan and substrates such as alumina and perlite. Oxalic acid forms hydrogen bonds or strong chelates with alumina or perlite and ionic bonds with amine groups in chitosan (Dobson and McQuillan, 1999). Removal of hexavalent chromium from aqueous medium was reported using a composite made from chitosan-coated onto alumina. It was also reported that the coating improved the adsorption capacity of chitosan for Cr(VI) by exposing more binding sites on the composite biosorbent to adsorb heavy metals (Boddu et al., 2003).
Chitosan immobilized on perlites have shown higher removal capacities of Cd(II) even at low pH 2–4.5 while both pure perlite or chitosan showed no removal of cadmium at pH < 4 (Hasan et al., 2006). Chitosan/sand composite made from the coating of chitosan on montmorillonite clay particles has improved the adsorption of tungsten ions from aqueous medium. This three-dimensional sorbent used exposed amine functional groups to coordinate with metal cations to make complexes (Gecol et al., 2006). Poly(vinyl alcohol) is a non-toxic, biocompatible polymer that has been employed to immobilize chitosan to prepare poly(vinyl alcohol)-chitosan composite for the removal of Cu(II) and Cd(II) from waste water (Kumar et al., 2009).
Chitosan-modified pyrolysis biochars have been previously used to remove heavy metals from water (Zhou et al., 2013; Zhang et al., 2015; Huang et al., 2016). Here, we evaluate both uncoated and chitosan-coated gasified pine wood biochar for their copper and cadmium removal capabilities. Green, GBC coated with chitosan can be produced at low cost because both components are inexpensive and readily available.
Materials and Methods
Preparation of Chitosan-Coated Gasifier Biochar
All chemicals used in this study were analytical grade from Sigma Aldrich, USA and used as received unless specified. Biochar was formed in a down-draft gasifier (BioMax 25, Community Power Corp., Littleton, CO, United States) at Mississippi State University at 700–900°C under nitrogen flow (10°C/min) with a residence time of 5–10 s fed with pine wood chips. Chitosan flakes (prepared by 85% deacylation of chitin), 3.0 g, from Dungeness Environmental was dissolved into 180 mL of 2% acetic acid. The pine wood gasifier bio-char (3 g) was then added to the chitosan/acetic acid solution and stirred for 30 min. The resulting mixture was added dropwise into 900 mL of a 1.2% NaOH solution and then aged for 24 h. The pH after aging was ∼10. Deprotonation of chitosan rendered it insoluble, and it complexes to form a coating on the biochar. The chitosan-coated biochar was then filtered and washed with 100 mL of deionized water and allowed to dry at 90°C in air for 24 h. The final weight of the dried sample was 4 g. The 3 g of biochar complexed 1 g of chitosan resulting is a 25/75 wt% chitosan/biochar complex.
Char Characterization
The chemical composition (C, H, N) of both GBC and Chitosan-Coated Gasifier Biochar (CGBC) was determined via combustion analysis. Ash content was also calculated for both biochars. The organic oxygen content was determined by subtracting the sum of the %C, %H, %N, plus the ash weight from the initial sample. The ash contains additional oxygen in the form of metal oxides. BET surface areas, pore volumes, and averaged pore diameters of both adsorbents were examined using a Micromeritics TriStar II Plus 3030 surface area analyzer using nitrogen adsorption isotherms at −196°C (BET). The FT-IR transmission spectra of both chars were collected using an FT-IR fixed with a diamond AT-IR unit Bruker Alpha II, United States. Thermogravimetric analysis (TGA) was done under air at a heating rate of 10°C/min from 32 to 1000°C for both GBC and CGBC using a TA Instrument’s Q50 thermogravimetric analyzer (United States). Surface morphologies of the two adsorbents were examined by scanning electron microscopy (SEM) using a JEOL JSM-6500F FE-SEM at 5 kV. Energy-dispersive X-ray spectroscopy (EDX) was carried out on both biochars using a Zeiss, EVO 40 scanning electron microscope containing a BRUKER EDX system. The point of zero charge (PZC) of both GBC and CGBC was determined using 0.01 M NaCl aqueous solutions with pH values ranging from 2 to 10 at pH intervals of 2. The solutions (25 mL) were stirred at 200 rpm for 8 h with 0.025 g of suspended adsorbent. After adsorbent removal, the pH of the supernatant was measured using an ORION model 210 pH meter. The PZC was obtained by plotting pH of the initial solution against pH of the final solution.
Adsorption Studies
All chemical reagents were analytical grade. Water for stock solutions was filtered with a Millipore Milli-Q Academic system using a Quantum EX Ultrapure Organex Cartridge. Batch sorption studies for GBC and CGBC char were conducted by varying Cu2+ and Cd2+ concentrations from 25 to 300 mg/L using deionized water at pH 5. A 0.025 g quantity of char was added to 45 mL vials each containing 25 mL solutions of Cu2+ or Cd2+ at different concentrations. The solutions were shaken for 24 h at 200 rpm to reach equilibrium. Biochars were then removed by filtration using Whatman #1 qualitative filter papers, and the adsorbate concentrations remaining in the filtrate were determined using atomic absorption spectroscopy. All experiments were repeated, and the presented result error bars represent the standard deviation of three replicates. The adsorbate amount removed per gram of char (Qe) was calculated using: . Here, Co and Ce are initial and equilibrium analyte solution concentrations in the solution (mg/L), V is the solution volume (L), and M is the total mass of adsorbent added (g).
The Cu2+ and Cd2+ uptake rate kinetics of the two biochars were determined at pH 5 and 25°C at time intervals between 2 min and 16 h. The concentrations of Cu2+ and Cd2+ were 100 ppm. The total volume was 25 mL, and the mass of each adsorbent used was 0.010 g.
Results and Discussion
Characterization of Chitosan Coated Biochar
The BET surface areas of GBC and CGBC along with their elemental analyses (combustion and ash) are presented in Table 1. The surface area of GBC (34.1 m2/g) is within the expected range for gasified biochar (Mallick, 2002; Son et al., 2018). Coating with chitosan causes a dramatic decrease in the surface area of CGBC (4.61 m2/g). This decrease is due to the chitosan coating which covers pore openings and is consistent with previous studies (Zhou et al., 2013). Chitosan coating also decreases the pore volume and average pore size (Huang et al., 2016). Material C, O, N and ash combustion analysis is included in Table 1. Metal oxide composition cannot be analyzed from this method but both GBC and CGBC have high ash content, 56.3 and 22.6% for GBC and CGBC, respectively, which is common for GBCs (Yongcheng et al., 2013). Gasifier biochars traditionally have a high ash content because of the high preparation temperatures and times. The ash content is much reduced during the chitosan coating process. This is likely due to a combination of washing away of some of the biochar ash and the additions of ash-free chitosan. The biochars also show low%C composition compared to pyrolysis biochars (Mallick, 2002). The addition of chitosan resulted in an increase in the %N composition from GBC to CGBC. The %N composition for CGBC was found to be 3.25% which equates to a 25/75 wt% ratio of chitosan to biochar in agreement with the gravimetric analysis obtained from the synthesis of CGBC.
The FT-IR spectra of GBC and CGBC are shown in Figure 1. The CGBC band at about 3280 cm–1 is due to NH stretching of chitosan amino groups coated on the biochar surface. This band is not found in the GBC. The 2872.2 cm–1 band of CGBC is attributed to C–H stretching of –CH and CH2 groups of chitosan, while bands at 1653.3 and 1559.0 cm–1 are due to amide carbonyl stretching vibration of unhydrolyzed amide functional groups remaining in chitosan (Udaybhaskar et al., 1990; Zhang et al., 2015; Huang et al., 2016; Afzal et al., 2018; Ifthikar et al., 2018). The band near 1405 cm–1 in both GBC and CGBC is typical of C–O–H bending of phenols found in most biochars (Cantrell et al., 2012; Chauhan et al., 2012).
Scanning electron microscopy images of GBC and CGBC (Figure 2) illustrate large pore channels of GBC left over from the pine wood’s original morphology with clusters of metal oxide or salt particles (ash) dispersed throughout the channels (Figure 2A). Figure 2B is at a lower magnification and shows a very smooth surface with large pores. In contrast, in CGBC the pore channels are covered and blocked by the thick chitosan coating (Figures 2C,D). This accounts for the low BET surface area of CGBC. Figure 2D shows that the overall surface morphology has changed to a rough chitosan coated biochar surface. The EDX spectra (Supplementary Figures 4–7) for both Cu2+ and Cd2+ experiments show the presence of these two elements on both adsorbents’ surfaces after adsorption. EDX mapping analysis (Figures 2E–H) revealed the adsorption of Cu2+ on the surfaces of GBC and CGBC. EDX mapping images of Cd2+ adsorbed on both biochars are shown in Supplementary Figure 3. Variable mesh sized sieves were used to calculate the percentage fraction of biochar under each particle size. Sieving experiment results (Table 2) confirmed that the biochar particle size increased due to chitosan coating.
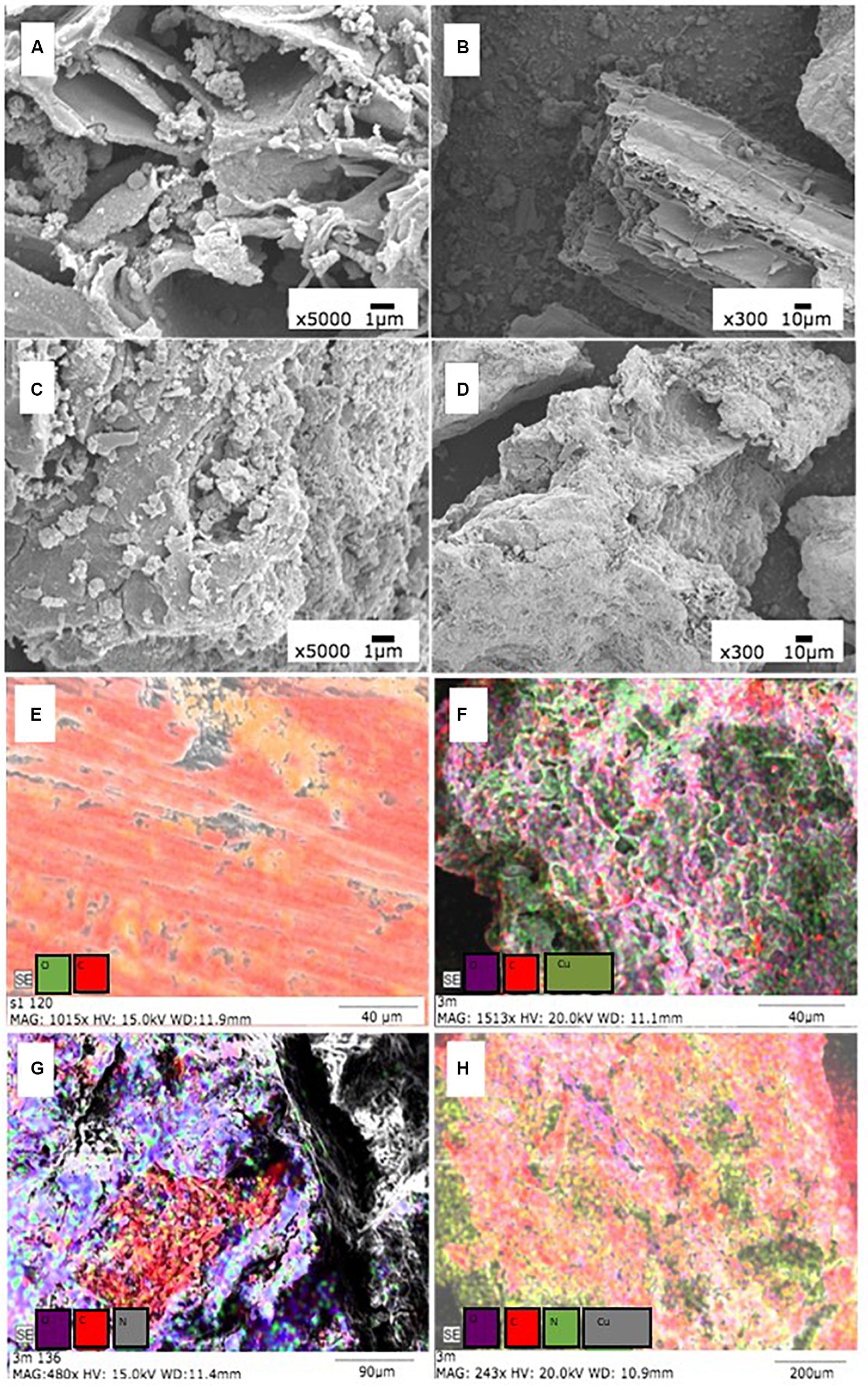
Figure 2. SEM images of GBC (A,B), CGBC (C,D) and EDX analysis of (E) GBC before adsorption and (F) GBC after Cu2+ adsorption. Panel (G) is CGBC before adsorption and panel (H) is CGBC after Cu2+ adsorption.
Differences in the TGA curves (Supplementary Figure 1) of GBC and CGBC, when heated at 10°C/min in air, supports the presence of chitosan on the CGBC surface. GBC is thermally stable to approximately 540°C and then quickly loses about 18% of its weight between 550–700°C (Zhou et al., 2013). This is due to the loss of carbon in the form of CO or CO2 in this range. The CGBC has a lower temperature weight loss region (225–270°C)
where chitosan thermally degrades (Hong et al., 2007), followed by a slower loss of chitosan residue up to ∼540°C. From 540 to 1000°C, CGBC exhibits more weight loss than GBC due to the continued weight loss from chitosan residues along with the underlying biochar decomposition and the lower ash content of the CGBC. These weight loss changes for GBC and CGBC are comparable to what is found in the literature (Zhou et al., 2013; Dewage et al., 2018).
The PZC is the pH at which the net charge of the biochar surface is zero. The PZC (Supplementary Figure 2) drops upon chitosan coating (11.2 for GBC and 10.2 for CGBC). The PZC of GBC is higher than fast pyrolysis biochars and almost all slow pyrolysis biochars, with consequences for heavy metal adsorption (Wan Ngah and Hanafiah, 2008; Hu et al., 2019). This high pH is a result of the relatively high concentration of surface metal oxides and metal hydroxides, which may form on water uptake, as well as metal carbonate formation. All this is a consequence of high gasification temperatures. Biomass combustion converts most carbon to gas, lowering the GBC carbon content, while leaving behind a high ash fraction. Gasification also decarboxylates R-COOH functions and lowers organic hydroxyl content.
Effect of Solution pH
The adsorption of Cu2+ and Cd2+ was quantified over a pH range of 2–5 (Figure 3). Initial metal ion concentrations were 100 mg/L and adsorption was allowed to reach equilibrium (24 h). Adsorption capacities rose with increasing pH for each metal ion. The largest increase occurred with a pH change of 2–3. An increase in adsorption with rising pH as observed previously with chitosan (Vold et al., 2003; Krajewska, 2004). Figure 3 shows that the amount, mg of ion/g for char, of Cu2+ and Cd2+ adsorbed are similar at higher pH for both GBC and CGBC. The pH dependence of metal ion adsorption is closely connected with the adsorbents’ surface charge (Guibal, 2004; Krajewska, 2004; Huang et al., 2016; Xiao et al., 2019). At low pH, both adsorbents will be more highly positively charged. Metal ions and protons from H3O+ compete for the amine groups on CGBC, and protons also compete with the metal ions for biochar adsorption sites. As the pH is raised, adsorption sites will become progressively deprotonated (Supplementary Table 1) resulting in decreased electrostatic repulsion of positively charged metal ions (Wan Ngah and Hanafiah, 2008).
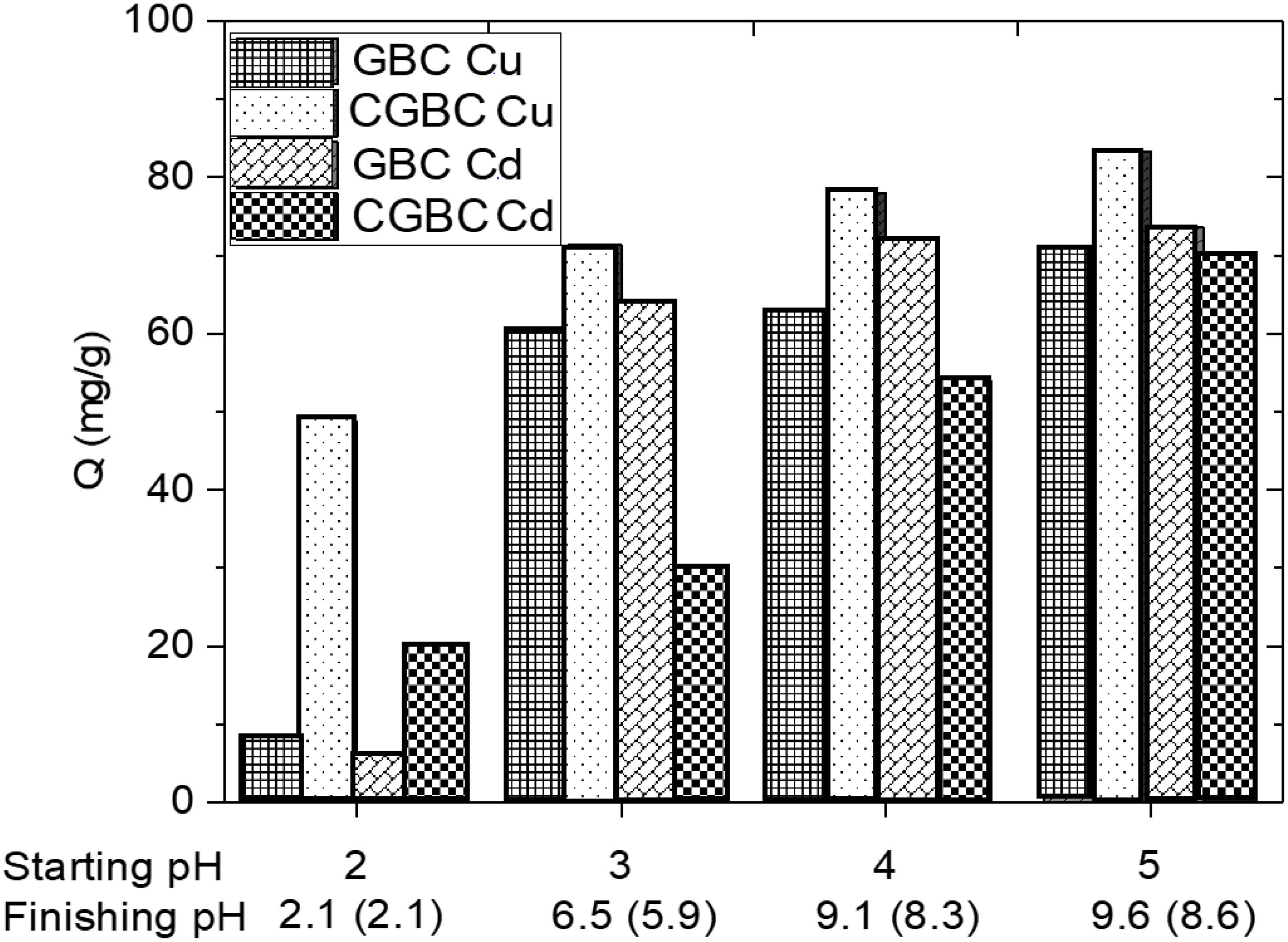
Figure 3. Effect of pH on Cu2+ and Cd2+ ion adsorption onto GBC and CGBC using 0.025 g of adsorbent and 25 mL 100 ppm solutions of each metal ion at the desired pH. Both starting and finishing pH values for GBC (CGBC) are shown.
Effect of Contact Time
Adsorption vs time on GBC and CGBC for each metal ion is shown in Figure 4. Biochar (0.01 g) was stirred at 200 rpm with 25 mL of 100 ppm solutions of each metal individually. Metal adsorption was rapid in the first hour for each metal ion. Equilibrium was reached for both biochars in 6 h and 2 h for Cu2+ and Cd2+, respectively. An increase in the adsorption capacity from GBC to CGBC occurs for Cu2+ but not Cd2+. This is believed to be due to a lower binding constant of Cd2+ to chitosan compared to Cu2+ (Krajewska, 2004).
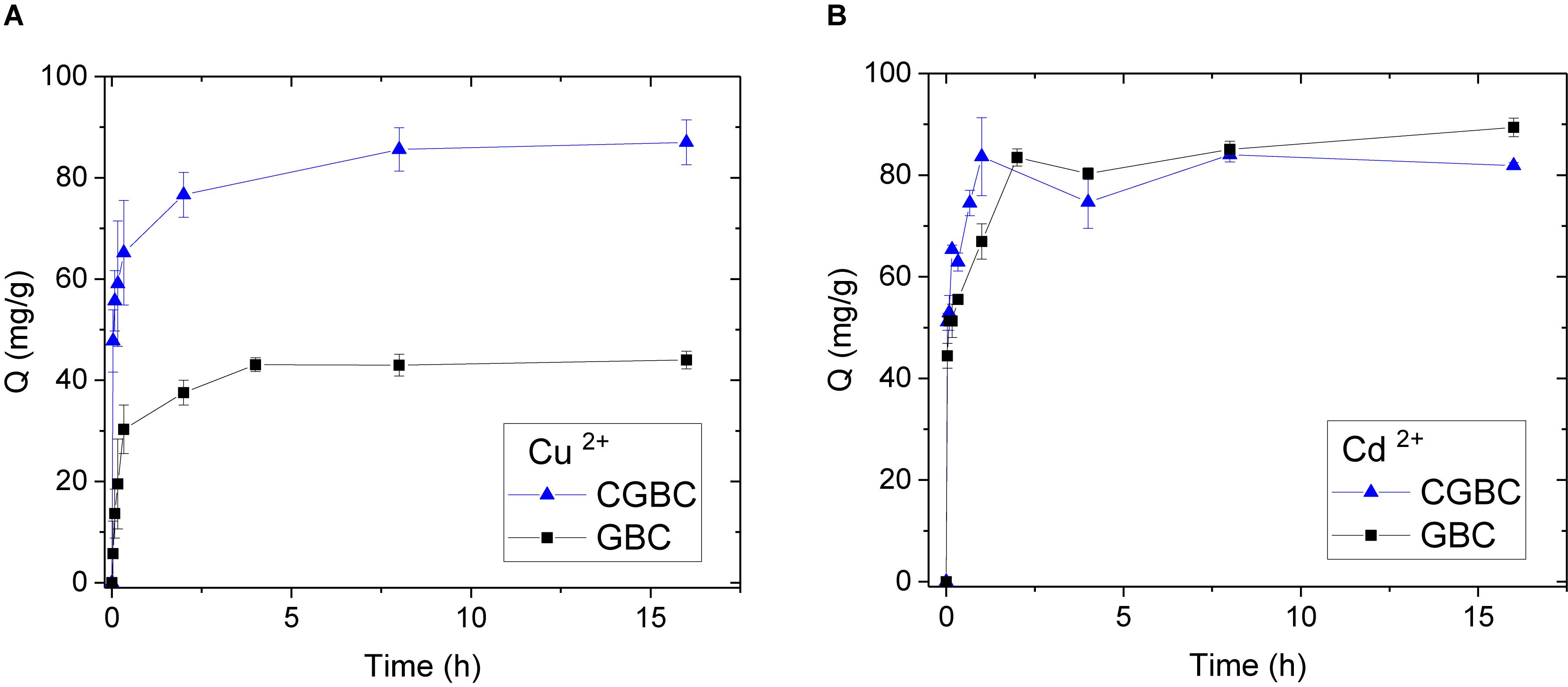
Figure 4. Effect of contact time of (A) Cu2+ and (B) Cd2+ adsorption onto GBC and CGBC. Standard deviation error bars are from three replicates.
Adsorption Kinetics
Pseudo first and second order linear kinetics models were used to fit the adsorption vs time data for Cu2+ and Cd2+. The first order model (eq. 1)
gives qt as the amount of metal adsorbed at time, t; qe is the amount adsorbed at equilibrium; and k1 (h–1) is the first order adsorption rate constant. The pseudo first order plots of log(qe − qt) verses t (Supplementary Figure 8) exhibited very poor fits and correlation coefficients, 0.45–0.95 (Table 3).
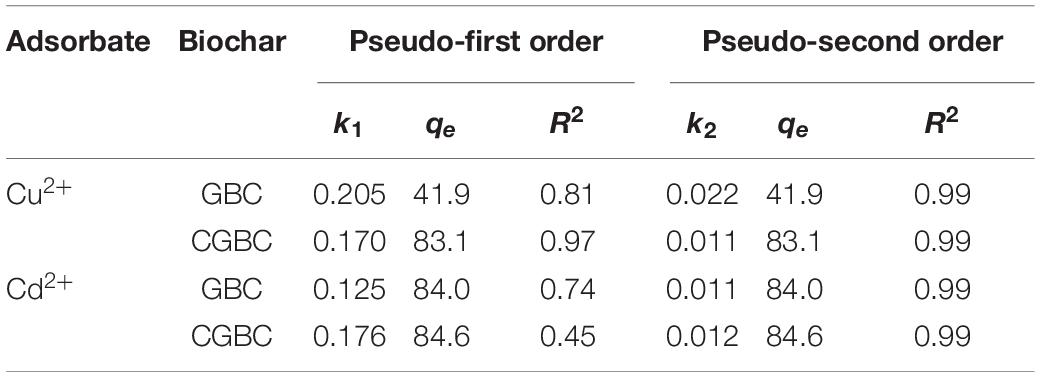
Table 3. Pseudo-first and second order parameters for Cu2+ and Cd2+ adsorption of at pH of 5 and 100 ppm of metal adsorbate using GBC and CGBC.
The pseudo second order linear model (eq. 2) is:
where qt is the amount of metal adsorbed at time, t; qe is the amount adsorbed at equilibrium; and k2 (h–1) is the second order adsorption rate constant. The plots of t/qt versus t produced excellent linear fits (Figure 5). The pseudo second order kinetic parameters for Cu2+ and Cd2+ are provided in Table 3 with correlation coefficients of 0.99. Both GBC and CGBC adsorb Cu2+ and Cd2+ via pseudo second order kinetics.
Adsorption Isotherm Models
To aid the design of GBC and CGBC applications, Freundlich (Freundlich, 1906), Langmuir (Langmuir, 1918), and Sips (Sips, 1948) models were employed to evaluate the maximum adsorption properties of both adsorbents. The three model equations are:
where Ce (mg/L) is the equilibrium concentration in the solution; qe (mg/g) is the adsorbate removed by an amount of adsorbent at equilibrium; qmax (mg/g) is the maximum adsorption capacity; n is the degree of non-linearity; KL (L/mg), KF [(mg/g)(L/mg)1/n], and KLF [mg/g (mg/L)–1/n] are the constants for Langmuir, Freundlich, and Sips models, respectively. Origin Pro 2018 software was used to fit the isotherm data. Adsorption isotherm studies were conducted over 25–300 mg/L for both Cd2+ and Cu2+ to simulate the concentrations of toxic metals released in industrial effluents and to provide data for adsorption capacities.
Figure 6 shows the adsorption isotherm fittings for each metal ion with both GBC and CGBC at 30°C. Table 4 presents the summarized values of the fitting parameters with the corresponding correlation coefficients obtained for each metal ion adsorbate onto the biochars. The adsorption data for Cd2+ gave similar fits to both the Langmuir and Freundlich models with R2 values over 0.93. The Cu2+ data was also found to fit the Freundlich model very well. The Sips model also fits the Cu2+ well with R2 values >0.94. The good fit for both Langmuir and Sips models suggests a monolayer adsorption mechanism could operate for both metals onto GBC and CGBC. Monolayer adsorption is consistent with other claims of heavy metal ion adsorption onto chitosan-functionalized materials (Krajewska, 2004; Deng et al., 2017). However, our isotherm studies are not definitive on this point.
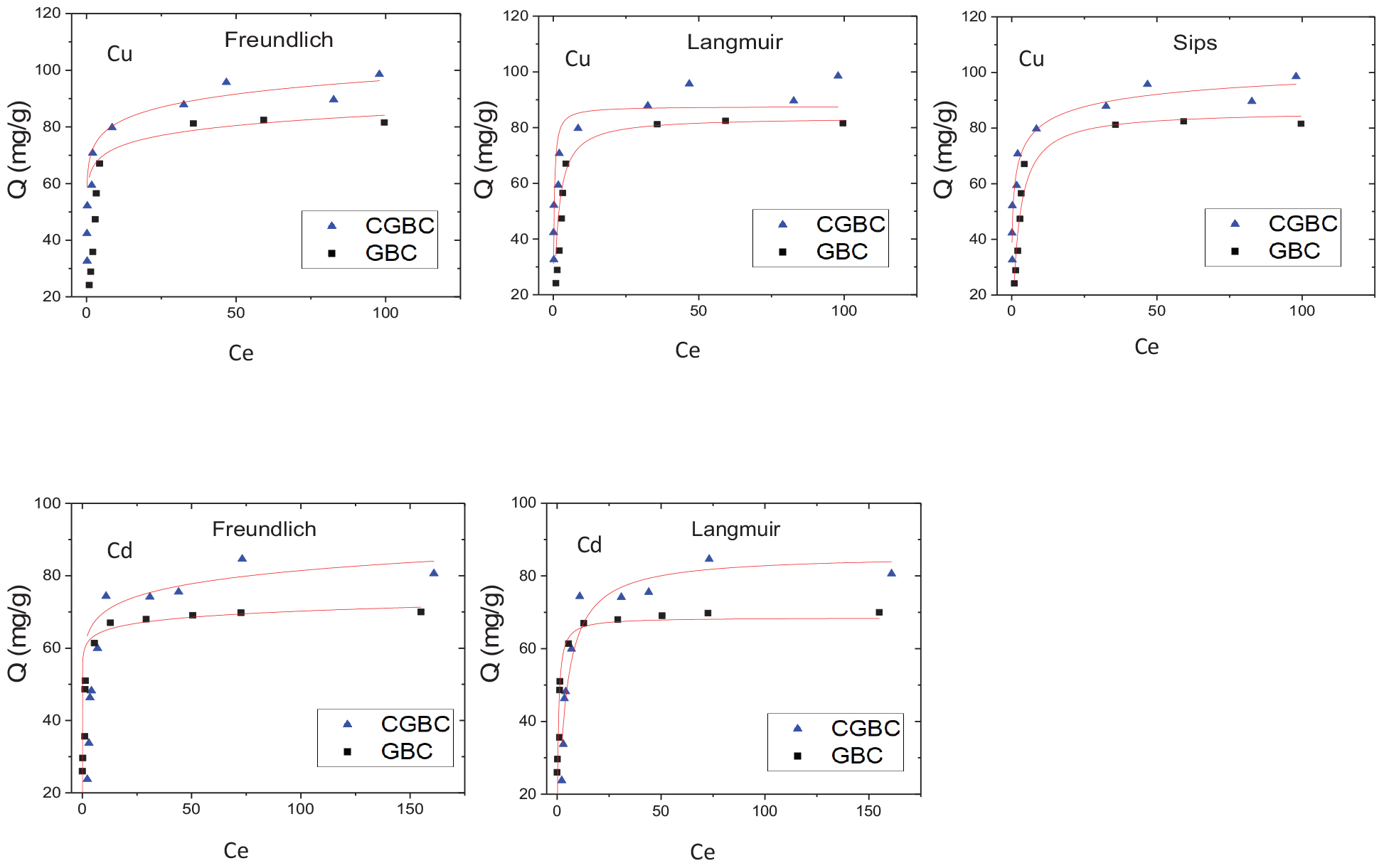
Figure 6. Adsorption isotherms for Cu2+ and Cd2+ removal by GBC and CGBC plotted using non-linear least squares curve fitting.
The maximum GBC adsorption capacities for Cd2+ and Cu2+on GBC were given, respectively, by the Langmuir (70.5 mg/g for Cd2+) and Sips (89.2 mg/g for Cu2+) models. Using CGBC provided an increase in the maximum adsorption capacities to 80.8 mg/g for Cd2+ (Langmuir model) and 112 mg/g for Cu2+ (Sips model). Chitosan deposition likely acted to increase both Cu2+ and Cd2+ removal ability due to the formation of strong metal ion/amine coordination. Adsorption capacities compare favorably to those reported in the literature for other biochar adsorbents (Mohan et al., 2014). Addition of the chitosan decreased the surface area by 86%, but increased in the overall Cu2+ and Cd2+ adsorption capacities. Table 5 compares these capacities to other biochars, chitosan, and activated carbon. Capacities of the adsorbents are given as both mg adsorbed/g adsorbent and as mg adsorbed/m2 of the surface area measured by BET.
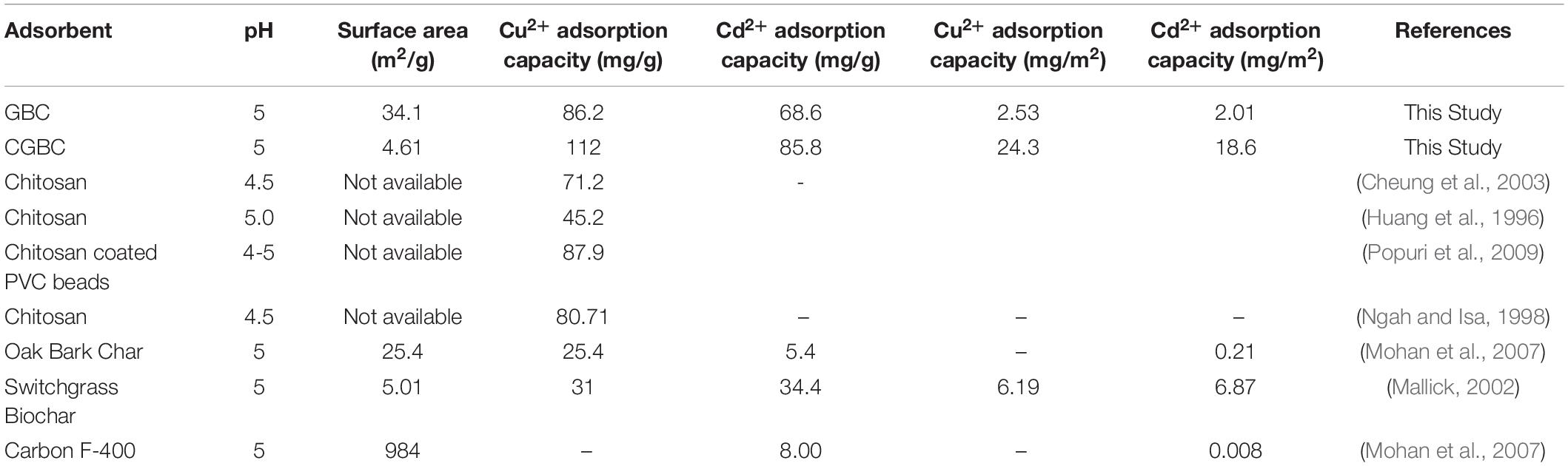
Table 5. Comparison of GBCs’ and CGBCs’ metal adsorption capacity with other biochars, chitosan, and activated carbon.
Conclusion
Gasifier pine wood biochar, GBC, was modified by surface deposition of 25% wt chitosan (CGBC). CGBC and GBC were characterized by FTIR, SEM, BET surface area, elemental analysis (EA), TGA, and PZC. The surface area of CGBC was 4.61 m2/g, which was decreased from GBC, 34.1 m2/g, due to pore blocking by chitosan. Batch adsorption studies were performed at pH of 2–5 to find the optimum pH. The maximum metal adsorption occurred at pH 5. Pseudo-second order kinetics provided the best fit with regression coefficients of 0.99. The max adsorption capacity was studied using the Freundlich, Langmuir, and Sips isotherm models. Cd2+ adsorption was best fit using the Freundlich (GBC) and Langmuir (CGBC) models. Cu2+ was best fit with Freundlich but showed good fitting to the Langmuir (GBC) and Sips (CGBC) models. GBC removed the Cu2+ and Cd2+ ions from water with good capacities which increased upon coating with chitosan (producing CGBC). The chitosan amine groups increase adsorption capacity by metal ion coordination. The adsorption capacities for Cu2+ and Cd2+ (mg/m2) are better than other biochars and activated carbons that were reported earlier (Table 5).
This work demonstrates that byproduct biochars from gasification can successfully adsorb Cu2+ and Cd2+, and that this adsorption can be augmented with chitosan-coating of the biochar. Production of high ash biochars from gasification is growing with the increasing use of biomass as an alternative energy source. This work highlights an application for low cost, high ash gasified biochar.
Data Availability Statement
The datasets generated for this study are available on request to the corresponding author.
Author Contributions
GB led the program. AH and GC contributed with sample characterizations. DB and SP helped with data collection. TM and CP advised. All authors contributed to the article and approved the submitted version.
Conflict of Interest
The authors declare that the research was conducted in the absence of any commercial or financial relationships that could be construed as a potential conflict of interest.
Acknowledgments
The authors would like to acknowledge support from the University Grants Commission for the Joint Research Program entitled “Indo-US initiatives on Cleaner Energy and Water 17 Research” between Jawaharlal Nehru University and Mississippi State University Chemistry Department and the National Science Foundation under Award Number 1659830 for the INFEWS REU: Food Energy and Water Security. The authors would also like to acknowledge Drs. Jason Street and Fei Yu (Mississippi State University) for providing raw gasifier biochar and Dungeness Environmental for providing chitosan.
Supplementary Material
The Supplementary Material for this article can be found online at: https://www.frontiersin.org/articles/10.3389/fenvs.2020.541203/full#supplementary-material
References
Afzal, M. Z., Sun, X.-F., Liu, J., Song, C., Wang, S.-G., and Javed, A. (2018). Enhancement of ciprofloxacin sorption on chitosan/biochar hydrogel beads. Sci. Total Environ. 639, 560–569. doi: 10.1016/j.scitotenv.2018.05.129
Babel, S., and Kurniawan, T. A. (2003). Low-cost adsorbents for heavy metals uptake from contaminated water: a review. J. Hazardous Mater. 97, 219–243. doi: 10.1016/s0304-3894(02)00263-7
Bailey, S. E., Olin, T. J., Bricka, R. M., and Adrian, D. D. (1999). A review of potentially low-cost sorbents for heavy metals. Water Res. 33, 2469–2479. doi: 10.1016/s0043-1354(98)00475-8
Basci, N., Kocadagistan, E., and Kocadagistan, B. (2004). Biosorption of copper (II) from aqueous solutions by wheat shell. Desalination 164, 135–140. doi: 10.1016/s0011-9164(04)00172-9
Bereket, G., Arog, A. Z., and Özel, M. Z. (1997). Removal of Pb(II), Cd(II), Cu(II), and Zn(II) from Aqueous Solutions by Adsorption on Bentonite. J. Colloid Interface Sci. 187, 338–343. doi: 10.1006/jcis.1996.4537
Blöcher, C., Dorda, J., Mavrov, V., Chmiel, H., Lazaridis, N. K., and Matis, K. A. (2003). Hybrid flotation—membrane filtration process for the removal of heavy metal ions from wastewater. Water Res. 37, 4018–4026. doi: 10.1016/s0043-1354(03)00314-2
Boddu, V. M., Abburi, K., Talbott, J. L., and Smith, E. D. (2003). Removal of hexavalent chromium from wastewater using a new composite chitosan biosorbent. Environ. Sci. Technol. 37, 4449–4456. doi: 10.1021/es021013a
Boddu, V. M., Abburi, K., Talbott, J. L., Smith, E. D., and Haasch, R. (2008). Removal of arsenic (III) and arsenic (V) from aqueous medium using chitosan-coated biosorbent. Water Res. 42, 633–642. doi: 10.1016/j.watres.2007.08.014
Brewer, C. E., Schmidt-Rohr, K., Satrio, J. A., and Brown, R. C. (2009). Characterization of biochar from fast pyrolysis and gasification systems. Environ. Progress Sustain. Energy 28, 386–396. doi: 10.1002/ep.10378
Brown, P. A., Gill, S. A., and Allen, S. J. (2000). Metal removal from wastewater using peat. Water Res. 34, 3907–3916. doi: 10.1016/s0043-1354(00)00152-4
Cantrell, K. B., Hunt, P. G., Uchimiya, M., Novak, J. M., and Ro, K. S. (2012). Impact of pyrolysis temperature and manure source on physicochemical characteristics of biochar. Bioresour. Technol. 107, 419–428. doi: 10.1016/j.biortech.2011.11.084
Chauhan, D., Jaiswal, M., and Sankararamakrishnan, N. (2012). Removal of cadmium and hexavalent chromium from electroplating waste water using thiocarbamoyl chitosan. Carbohydrate Polymers 88, 670–675. doi: 10.1016/j.carbpol.2012.01.014
Cheung, W., Ng, J., and McKay, G. (2003). Kinetic analysis of the sorption of copper (II) ions on chitosan. J. Chem. Technol. Biotechnol. 78, 562–571. doi: 10.1002/jctb.836
Crini, G. (2005). Recent developments in polysaccharide-based materials used as adsorbents in wastewater treatment. Progr. Polymer Sci. 30, 38–70. doi: 10.1016/j.progpolymsci.2004.11.002
Dañbrowski, A., Hubicki, Z., Podkościelny, P., and Robens, E. (2004). Selective removal of the heavy metal ions from waters and industrial wastewaters by ion-exchange method. Chemosphere 56, 91–106. doi: 10.1016/j.chemosphere.2004.03.006
Deng, J., Liu, Y., Liu, S., Zeng, G., Tan, X., Huang, B., et al. (2017). Competitive adsorption of Pb (II), Cd (II) and Cu (II) onto chitosan-pyromellitic dianhydride modified biochar. J. Colloid Interface Sci. 506, 355–364. doi: 10.1016/j.jcis.2017.07.069
Dewage, N. B., Fowler, R. E., Pittman, C. U., Mohan, D., and Mlsna, T. (2018). Lead (Pb 2+) sorptive removal using chitosan-modified biochar: batch and fixed-bed studies. RSC Adv. 8, 25368–25377. doi: 10.1039/c8ra04600j
Dobson, K. D., and McQuillan, A. J. (1999). In situ infrared spectroscopic analysis of the adsorption of aliphatic carboxylic acids to TiO2, ZrO2, Al2O3, and Ta2O5 from aqueous solutions. Spectrochim. Acta Part A Mol. Biomol. Spectroscopy 55, 1395–1405. doi: 10.1016/s1386-1425(98)00303-5
Erdem, E., Karapinar, N., and Donat, R. (2004). The removal of heavy metal cations by natural zeolites. J. Colloid Interface Sci. 280, 309–314. doi: 10.1016/j.jcis.2004.08.028
Fu, F., and Wang, Q. (2011). Removal of heavy metal ions from wastewaters: a review. J. Environ. Manag. 92, 407–418. doi: 10.1016/j.jenvman.2010.11.011
Gecol, H., Miakatsindila, P., Ergican, E., and Hiibel, S. R. (2006). Biopolymer coated clay particles for the adsorption of tungsten from water. Desalination 197, 165–178. doi: 10.1016/j.desal.2006.01.016
González-Muñoz, M. J., Rodríguez, M. A., Luque, S., and Álvarez, J. R. (2006). Recovery of heavy metals from metal industry waste waters by chemical precipitation and nanofiltration. Desalination 200, 742–744. doi: 10.1016/j.desal.2006.03.498
Guibal, E. (2004). Interactions of metal ions with chitosan-based sorbents: a review. Separat. Purificat. Technol. 38, 43–74. doi: 10.1016/j.seppur.2003.10.004
Hasan, S., Krishnaiah, A., Ghosh, T. K., Viswanath, D. S., Boddu, V. M., and Smith, E. D. (2006). Adsorption of divalent cadmium (Cd (II)) from aqueous solutions onto chitosan-coated perlite beads. Industrial Eng. Chem. Res. 45, 5066–5077. doi: 10.1021/ie0402620
Hong, P.-Z., Li, S.-D., Ou, C.-Y., Li, C.-P., Yang, L., and Zhang, C.-H. (2007). Thermogravimetric analysis of chitosan. J. Appl. Polymer Sci. 105, 547–551. doi: 10.1002/app.25920
Hu, Z., Zhang, L., Zhong, L., Zhou, Y., Xue, J., and Li, Y. (2019). Preparation of an antibacterial chitosan-coated biochar-nanosilver composite for drinking water purification. Carbohydr. Polym. 219, 290–297. doi: 10.1016/j.carbpol.2019.05.017
Huang, C., Chung, Y.-C., and Liou, M.-R. (1996). Adsorption of Cu (II) and Ni (II) by pelletized biopolymer. J. Hazardous Mater. 45, 265–277. doi: 10.1016/0304-3894(95)00096-8
Huang, X., Liu, Y., Liu, S., Tan, X., Ding, Y., Zeng, G., et al. (2016). Effective removal of Cr (VI) using β-cyclodextrin–chitosan modified biochars with adsorption/reduction bifuctional roles. RSC Adv. 6, 94–104. doi: 10.1039/c5ra22886g
Huisman, J. L., Schouten, G., and Schultz, C. (2006). Biologically produced sulphide for purification of process streams, effluent treatment and recovery of metals in the metal and mining industry. Hydrometallurgy 83, 106–113. doi: 10.1016/j.hydromet.2006.03.017
Hunsom, M., Pruksathorn, K., Damronglerd, S., Vergnes, H., and Duverneuil, P. (2005). Electrochemical treatment of heavy metals (Cu2+, Cr6+, Ni2+) from industrial effluent and modeling of copper reduction. Water Res. 39, 610–616. doi: 10.1016/j.watres.2004.10.011
Ifthikar, J., Jiao, X., Ngambia, A., Wang, T., Khan, A., Jawad, A., et al. (2018). Facile one-pot synthesis of sustainable carboxymethyl chitosan–sewage sludge biochar for effective heavy metal chelation and regeneration. Bioresour. Technol. 262, 22–31. doi: 10.1016/j.biortech.2018.04.053
Kadirvelu, K., Thamaraiselvi, K., and Namasivayam, C. (2001). Removal of heavy metals from industrial wastewaters by adsorption onto activated carbon prepared from an agricultural solid waste. Bioresour. Technol. 76, 63–65. doi: 10.1016/s0960-8524(00)00072-9
Krajewska, B. (2004). Application of chitin- and chitosan-based materials for enzyme immobilizations: a review. Enzyme Microbial Technol. 35, 126–139. doi: 10.1016/j.enzmictec.2003.12.013
Kumar, M., Tripathi, B. P., and Shahi, V. K. (2009). Crosslinked chitosan/polyvinyl alcohol blend beads for removal and recovery of Cd (II) from wastewater. J. Hazardous Mater. 172, 1041–1048. doi: 10.1016/j.jhazmat.2009.07.108
Langmuir, I. (1918). The adsorption of gases on plane surfaces of glass, mica and platinum. J. Am. Chem. Soc. 40, 1361–1403. doi: 10.1021/ja02242a004
Liu, G., Yu, Y., Hou, J., Xue, W., Liu, X., Liu, Y., et al. (2014). An ecological risk assessment of heavy metal pollution of the agricultural ecosystem near a lead-acid battery factory. Ecol. Indicat. 47, 210–218. doi: 10.1016/j.ecolind.2014.04.040
Liu, Z., Wang, H., Liu, C., Jiang, Y., Yu, G., Mu, X., et al. (2012). Magnetic cellulose-chitosan hydrogels prepared from ionic liquids as reusable adsorbent for removal of heavy metal ions. Chem. Commun. 48, 7350–7352. doi: 10.1039/c2cc17795a
Mallick, N. (2002). Biotechnological potential of immobilized algae for wastewater N. P and metal removal: a review. Biometals 15, 377–390.
Matlock, M. M., Howerton, B. S., and Atwood, D. A. (2002). Chemical precipitation of heavy metals from acid mine drainage. Water Res. 36, 4757–4764. doi: 10.1016/s0043-1354(02)00149-5
Mohan, D., Pittman, C. U. Jr., Bricka, M., Smith, F., Yancey, B., Mohammad, J., et al. (2007). Sorption of arsenic, cadmium, and lead by chars produced from fast pyrolysis of wood and bark during bio-oil production. J. Colloid Interface Sci. 310, 57–73. doi: 10.1016/j.jcis.2007.01.020
Mohan, D., Sarswat, A., Ok, Y. S., and Pittman, C. U. Jr. (2014). Organic and inorganic contaminants removal from water with biochar, a renewable, low cost and sustainable adsorbent – A critical review. Bioresour. Technol. 160, 191–202. doi: 10.1016/j.biortech.2014.01.120
Mohan, D., and Singh, K. P. (2002). Single- and multi-component adsorption of cadmium and zinc using activated carbon derived from bagasse—an agricultural waste. Water Res. 36, 2304–2318. doi: 10.1016/s0043-1354(01)00447-x
Mohan, S., and Gandhimathi, R. (2009). Removal of heavy metal ions from municipal solid waste leachate using coal fly ash as an adsorbent. J. Hazardous Mater. 169, 351–359. doi: 10.1016/j.jhazmat.2009.03.104
Muzzarelli, R. A. A., and Tubertini, O. (1969). Chitin and chitosan as chromatographic supports and adsorbents for collection of metal ions from organic and aqueous solutions and sea-water. Talanta 16, 1571–1577. doi: 10.1016/0039-9140(69)80218-3
Ngah, W. W., and Isa, I. (1998). Comparison study of copper ion adsorption on chitosan. Dowex A-1, and Zerolit 2. J. Appl. Polym. Sci. 67, 1067–1070. doi: 10.1002/(sici)1097-4628(19980207)67:6<1067::aid-app14>3.0.co;2-y
Nriagu, J. O., and Pacyna, J. M. (1988). Quantitative assessment of worldwide contamination of air, water and soils by trace metals. Nature 333, 134–139. doi: 10.1038/333134a0
World Health Organization (2011). Guidelines for Drinking-Water Quality. Geneva: World Health Organization.
Popuri, S. R., Vijaya, Y., Boddu, V. M., and Abburi, K. (2009). Adsorptive removal of copper and nickel ions from water using chitosan coated PVC beads. Bioresour. Technol. 100, 194–199. doi: 10.1016/j.biortech.2008.05.041
Qdais, H. A., and Moussa, H. (2004). Removal of heavy metals from wastewater by membrane processes: a comparative study. Desalination 164, 105–110. doi: 10.1016/s0011-9164(04)00169-9
Ravi Kumar, M. N. V. (2000). A review of chitin and chitosan applications. React. Funct. Polym. 46, 1–27.
Razo, I., Carrizales, L., Castro, J., Díaz-Barriga, F., and Monroy, M. (2004). Arsenic and Heavy Metal Pollution of Soil. Water and Sediments in a Semi-Arid Climate Mining Area in Mexico. Water Air Soil Pollut. 152, 129–152. doi: 10.1023/b:wate.0000015350.14520.c1
Sabiha, J., Mehmood, T., Chaudhry, M. M., Tufail, M., and Irfan, N. (2009). Heavy metal pollution from phosphate rock used for the production of fertilizer in Pakistan. Microchem. J. 91, 94–99. doi: 10.1016/j.microc.2008.08.009
Son, E.-B., Poo, K.-M., Mohamed, H. O., Choi, Y.-J., Cho, W.-C., and Chae, K.-J. (2018). A novel approach to developing a reusable marine macro-algae adsorbent with chitosan and ferric oxide for simultaneous efficient heavy metal removal and easy magnetic separation. Bioresour. Technol. 259, 381–387. doi: 10.1016/j.biortech.2018.03.077
Stern, B. R. (2010). Essentiality and toxicity in copper health risk assessment: overview. update and regulatory considerations. J. Toxicol. Environ. Health Part A 73, 114–127. doi: 10.1080/15287390903337100
Udaybhaskar, P., Iyengar, L., and Rao, A. V. S. P. (1990). Hexavalent chromium interaction with chitosan. J. Appl. Polym. Sci. 39, 739–747. doi: 10.1002/app.1990.070390322
Vaaramaa, K., and Lehto, J. (2003). Removal of metals and anions from drinking water by ion exchange. Desalination 155, 157–170. doi: 10.1016/s0011-9164(03)00293-5
Vold, I. M. N., Vårum, K. M., Guibal, E., and Smidsrød, O. (2003). Binding of ions to chitosan—selectivity studies. Carbohydr. Polym. 54, 471–477. doi: 10.1016/j.carbpol.2003.07.001
Wan Ngah, W. S., Endud, C. S., and Mayanar, R. (2002). Removal of copper(II) ions from aqueous solution onto chitosan and cross-linked chitosan beads. React. Funct. Polym. 50, 181–190. doi: 10.1016/s1381-5148(01)00113-4
Wan Ngah, W. S., and Hanafiah, M. A. K. M. (2008). Removal of heavy metal ions from wastewater by chemically modified plant wastes as adsorbents: a review. Bioresour. Technol. 99, 3935–3948. doi: 10.1016/j.biortech.2007.06.011
Wan Ngah, W. S., Teong, L. C., and Hanafiah, M. A. K. M. (2011). Adsorption of dyes and heavy metal ions by chitosan composites: a review. Carbohydr. Polym. 83, 1446–1456. doi: 10.1016/j.carbpol.2010.11.004
Xiao, F., Cheng, J., Cao, W., Yang, C., Chen, J., and Luo, Z. (2019). Removal of heavy metals from aqueous solution using chitosan-combined magnetic biochars. J. Colloid Interface Sci. 540, 579–584. doi: 10.1016/j.jcis.2019.01.068
Yongcheng, Z., Caixia, W., Fei, Y., Qi, L., and Philip, H. S. (2013). Studies of biochars generated from pilot-scale downdraft gasification. Trans. ASABE 56, 995–1001. doi: 10.13031/trans.56.9671
Zhang, M.-M., Liu, Y.-G., Li, T.-T., Xu, W.-H., Zheng, B.-H., Tan, X.-F., et al. (2015). Chitosan modification of magnetic biochar produced from Eichhornia crassipes for enhanced sorption of Cr(vi) from aqueous solution. RSC Adv. 5, 46955–46964. doi: 10.1039/c5ra02388b
Keywords: sorption, chitosan, gasified biochar, copper, cadmium, metal ions removal 2
Citation: Burk GA, Herath A, Crisler GB II, Bridges D, Patel S, Pittman CU Jr and Mlsna T (2020) Cadmium and Copper Removal From Aqueous Solutions Using Chitosan-Coated Gasifier Biochar. Front. Environ. Sci. 8:541203. doi: 10.3389/fenvs.2020.541203
Received: 07 March 2020; Accepted: 09 September 2020;
Published: 25 November 2020.
Edited by:
Baharak Sajjadi, University of Mississippi, United StatesReviewed by:
Danuta Leszczynska, Jackson State University, United StatesVijayasankar Raman, University of Mississippi, United States
Copyright © 2020 Burk, Herath, Crisler, Bridges, Patel, Pittman and Mlsna. This is an open-access article distributed under the terms of the Creative Commons Attribution License (CC BY). The use, distribution or reproduction in other forums is permitted, provided the original author(s) and the copyright owner(s) are credited and that the original publication in this journal is cited, in accordance with accepted academic practice. No use, distribution or reproduction is permitted which does not comply with these terms.
*Correspondence: Todd Mlsna, dG02MTNAbXNzdGF0ZS5lZHU=