- 1Department of Land, Air, and Water Resources, University of California, Davis, Davis, CA, United States
- 2The Nature Conservancy, Arlington, VA, United States
Enhancing soil organic matter in agricultural soils has potential to contribute to climate mitigation while also promoting soil health and resilience. However, soil carbon (C) sequestration projects are rare in C markets. One concern surrounding soil C is uncertainty regarding the permanence of newly sequestered soil C. This scientific uncertainty is exacerbated by differences in terminology used by scientists and policymakers, which impedes the integration of new scientific findings regarding soil carbon longevity into evidence-based policies. Here, we review the evolution of understanding of soil C lifespan and the language used to describe it in both scientific and policy sectors. We find that recent scientific findings that have bearing on soil C lifespan are not part of discussions surrounding C policy, and conversely, policymaker concerns are not clearly addressed by scientific research. From a policy perspective, soil C is generally assumed to be a vulnerable pool at risk of being quickly lost via microbial degradation or other avenues of physical loss if soil C building practices are not maintained indefinitely. This assumption has been challenged by recent scientific advances demonstrating that microbial consumption and transformation of plant-derived C actually necessary for the long-term storage of soil organic matter. Here, we argue that soil C longevity can best be understood as resulting from continual movement and transformation of organic compounds throughout the soil matrix, and show that this definition is directly at odds with how soil C longevity is represented in current policies. Given current interest in new policies to promote soil C sequestration activities, resolving these definitions is critical. We further identify priority areas for future research in order to answer key policymaker questions about soil C lifespan, and to help develop new tools and benchmarks necessary to assess efficacy of agricultural soil C sequestration efforts.
Introduction
How permanently will carbon (C) stored in soil remain sequestered? This question is often posed in public policy concerned with C sequestration offsets (Smith, 2005; Thamo and Pannell, 2016; Smith et al., 2019), but is rarely considered by the scientific community in those terms, and therefore rarely addressed. Differences not only in terminology, but in conceptual models of C sequestration used by policymakers versus scientists, makes it challenging to clearly define science-based policies promoting long-term soil C sequestration. Concern that any soil C stored would be easily lost and re-emitted to the atmosphere was one reason that soil C sequestration projects were excluded in early C offset markets, such as those created under the Kyoto Protocol (von Unger and Emmer, 2018). Though soil C sequestration projects are now gaining a presence, particularly in voluntary C markets, permanence concerns remain difficult to directly address using scientific data because of differences in terminology, definitions, and focus between scientists and policymakers (Box 1). A clear understanding of definitions and research gaps related to soil C lifespans is both timely and necessary to catalyze more ambitious soil conservation practices as a part of broader climate change mitigation efforts.
BOX 1. Unpacking commonly used terms to describe permanence.
Language surrounding soil C longevity is inconsistent between C policymakers and soil scientists, muddying the communication between these two groups. Furthermore, across scientific disciplines, and even within disciplines, some of the same terms have different meanings, adding to confusion. Clarifying language that is used to describe how, and for how long, SOM is preserved in soils is critical for effectively communicating opportunities and challenges for soil C sequestration, as well as aligning scientific research goals with the needs of policy makers and other stakeholders.
Terminology:
Sequestration: The transfer and storage of atmospheric CO2 to other pools, such as soil or plant biomass (Lal, 2008). In most C financing markets, C sequestration projects are required to sequester more C than would normally be sequestered without the intervention, not result in the transfer of C emissions to a different region or sector, and permanent (see below).
Permanence: Permanence is a necessary condition for creditable CO2 emissions offsets. Sequestered C must remain sequestered during the period of the offset credits, which are typically issued for a 100-year period (although in newer markets, temporary credits can be issued for periods as short as 25 years). Critically, in practice, permanence typically refers to the duration for which a C-sequestering practice is carried out, rather than the soil C itself. It is assumed that once the management practice ends, any accumulated soil C will be quickly lost (Smith, 2005).
Turnover time: In soil science and biogeochemistry, the lifespan of soil C is often described as its turnover time, mathematically defined as the ratio of the total carbon stock in a particular soil pool to the output flux (Schimel et al., 1994; Sierra et al., 2017). It is colloquially used to describe the average length of time a single organic molecule remains in the soil before being lost via leaching or respiration. Traditionally, soil C has been represented in global biogeochemical models, e.g., with three pools with turnover times ranging from <1 year (active pool), 20–50 year (slow pool) to >1000 year (passive pool) (Parton, 1996). The passive C pool has been interpreted to be permanently stored soil C. However, this model presents an overly simplistic view of soil C, one which is now being challenged (see “Toward dynamic stability” section).
Humus, humic substances, and humification: Humus originally referred to SOM that had undergone degradation and secondary synthesis to form new organic compounds distinct from their plant or microbial origin (Waksman, 1925). The term is also used colloquially to describe SOM of all forms (Schlesinger and Bernhardt, 2013). The idea of humus or humic substances dates back to the 18th century, when an alkaline extraction method was introduced to separate the soil organic phase from the mineral phase. Humic substances were operationally defined to be the compounds soluble in alkaline solution. Though never observed directly in un-treated soils through spectroscopic methods, humic substances were thought to be large, irregular, aromatic organic molecules resistant to microbial decomposition. Humification refers to the series of abiotic condensation reactions which transform plant and microbial monomers into humic substances. Today, humic terminology is controversial, with many scientists dismissing the concept of humic substances entirely (Lehmann and Kleber, 2015; Kleber and Lehmann, 2019), others maintaining that it is still useful (Olk et al., 2019), and still others using the terminology of humic substances and humification to broadly refer to SOM and SOM production, irrespective of alkaline extraction (Kirkby et al., 2013).
Recalcitrant: Refers to SOM that is resistant to microbial and enzymatic degradation due to its chemical structure. Microbes were thought to selectively degrade organic matter based on its ease of oxidation, leaving behind organic matter that is increasingly difficult to break down (Sollins et al., 1996). Alkyl and aromatic functional groups are both theorized to contribute to chemical recalcitrance (Melillo et al., 1983).
Stability: Refers broadly to SOM resistance to decay, whether that results from humification, selective preservation of recalcitrant organic matter, and physicochemical interactions such as adsorption to mineral surfaces and occlusion within soil aggregates (Knorr et al., 2005; von Lutzow et al., 2006).
Stabilization: Broadly refers to processes resulting in SOM that is resistant to decay. Though our knowledge of specific processes involved have changed as scientific understanding of SOM preservation has grown, the term generally reflects the paradigm that there is some physical mechanism through which SOM becomes permanently resistant to decay.
Persistence: Refers to long-term preservation of SOM resulting from ecological, biological, and physicochemical conditions and interactions rather than any inherent chemical property of SOM (Schmidt et al., 2011). Recent research suggests that not even physicochemical interactions (e.g., occlusion in microaggregates or sorption to mineral surfaces) can explain long-term persistence, as they are reversible (e.g., Fontaine et al., 2007; Keiluweit et al., 2015). Here, we advocate for an understanding of SOM persistence as a property driven by continual movement and transformation of organic molecules within the heterogeneous soil environment via interactions with both soil microbes and the physical soil matrix.
Soils hold the largest store of organic carbon (C) on the planet (Batjes, 1996), but human activities have degraded soils worldwide, causing a gap between global soil C capacity and current storage (Scharlemann et al., 2014; Sanderman et al., 2018). This gap has been framed as an opportunity for soils to serve as C sinks and contribute to climate change mitigation (Sommer and Bossio, 2014; Lal, 2016; Zomer et al., 2017). Restoring C in agricultural soils particularly is seen as a win-win climate solution, since management practices that would restore soil C can improve soil health and food security (Paustian et al., 1997; Lal, 2004a, b; Minasny et al., 2017), reduce chemical fertilizer needs (Oldfield et al., 2018), while also providing a cost-effective natural solution to combat climate change (Fuss et al., 2018; Bossio et al., 2020).
Efforts such as the French Ministry of Agriculture’s 4 per 1000 Initiative (Minasny et al., 2017) have stimulated considerable discussion regarding the potential, globally, for agroecosystems to sequester significant C stocks. Models and CO2-enrichment studies show that supplies of nitrogen and phosphorus may constrain plant productivity and therefore pose limits to soil C accumulation (Hungate et al., 2003; van Groenigen et al., 2006; Wieder et al., 2015; Van Groenigen et al., 2017). Additional issues include biophysical constraints such as insufficient availability of organic biomass to add to soils and potential saturation of soil C storage (Poulton et al., 2018; Schlesinger and Amundson, 2018; White et al., 2018), as well as social and economic constraints that may hinder widespread adoption of C-sequestering agricultural practices (Amundson and Biardeau, 2018; Baveye et al., 2018). Regardless of how much C can be sequestered by agricultural soils, the issue of permanence is still of importance.
Despite these challenges, restoring organic matter to agricultural soils still provides an opportunity to improve soil health and global food security while drawing down atmospheric CO2. A better understanding of the longevity of sequestered soil C could increase confidence in the amount of C that is able to be sequestered in agricultural soils, enabling policymakers to develop appropriate incentive and C payment structures while simultaneously restoring critical soil ecological functions (Baveye et al., 2020).
Recently, significant strides have been made in illuminating how, and for how long, SOM persists in soils, which can help inform data-supported soil C sequestration efforts. Here, we describe the evolution of the scientific understanding of soil C longevity, and highlight how outdated scientific concepts have perpetuated an inaccurate understanding of soil C in the public sphere. Using our current understanding of soil C permanence, we outline key priorities for synergistic scientific and policy advances in support of long-term soil C sequestration.
The Som Paradigm Shift
Soil C is predominantly found in organic molecules collectively referred to as soil organic matter (SOM), which also contain other elements such as nitrogen, phosphorus, sulfur, potassium and calcium. SOM serves as both a substrate for microbial metabolism as well as a warehouse for long-term soil C storage, with SOM-C ages as old as several millennia detected in deep soils (Campbell et al., 1967; Scharpenseel and Becker-Heidmann, 1989; Krull and Skjemstad, 2003). A portion of SOM is labile and can be metabolized by microbes on time scales varying from days to decades, whereas other fractions persist in soils for thousands of years. Thus, most widely used ecosystem models (e.g., the CENTURY model) represent SOM as multiple, distinct pools with mean residence times ranging from a few years in the labile pool, to thousands of years in the passive or stable pool (Parton, 1996). Elucidating the biochemical nature of SOM in these theoretical pools, and the mechanisms by which the stable SOM pool forms, has been the subject of continual controversy and research over the past century.
Many of the recent developments in SOM research have been discussed extensively in previous reviews. Here, we present a summary of previous research most relevant to understanding the longevity of soil C sequestration. For more in-depth discussion of specific topics, we refer the reader to the following literature: Marschner et al. (2008), Schmidt et al. (2011), Lehmann and Kleber (2015) (controversy about humic substances and recalcitrance), Dungait et al. (2012) (role of physical inaccessibility in SOM longevity), Lavallee et al. (2019) (mineral-associated organic matter and particulate organic matter), Rumpel and Kögel-Knabner (2011) (subsoil C storage), Jilling et al. (2018), Bailey et al. (2019) (soil C destabilization).
Classical views in soil science held that stable SOM consisted of compounds known as humic substances that were inherently resistant to decay due to their complex, highly aromatic structures (Box 1). Humic macromolecules were hypothesized to form spontaneously from organic monomers via a series of abiotic condensation reactions through the process of humification (Waksman, 1925; Hedges, 1988; Tan, 2014). However, the presence in soil of humic substances, traditionally isolated and quantified using a series of harsh chemical extractions, has not been supported by non-destructive observation techniques, such as 2D-NMR and near-edge X-ray fine structure spectroscopy (NEXAFS) (Kelleher and Simpson, 2006; Lehmann et al., 2007, 2008). For example, an examination of a humic acid standard and the humic acid fraction extracted from soils using 2D-NMR could find no distinct chemical signature that could not be attributed to well-characterized plant and microbial compounds, including proteins, carbohydrates, and biomarker compounds such as lignin and cutin (Kelleher and Simpson, 2006).
Another hypothesis was that the stable SOM pool consisted of recalcitrant organic molecules, such as lignin, that were selectively preserved during plant tissue decay due to their complex chemical structure (Marschner et al., 2008). Long-term C stability was still assumed to be conferred by the chemistry of persistent organic molecules (Box 1). Yet, decomposition and 13C labeling studies show that compounds previously assumed to be recalcitrant, such as lignin, decay in soil at rates similar to those of simpler organic compounds (Dignac et al., 2005; Heim and Schmidt, 2007; Klotzbücher et al., 2011; Haddix et al., 2016). Supporting that, 13C and 14C dating methods reveal that soil lignin is not older than simple, labile molecules like amino sugars (von Lutzow et al., 2006). Similarly, recent 13C-NMR spectroscopy work examining the decomposition of the organic biomarkers suberin and cutin shows that both compounds can be decomposed quickly (Angst et al., 2016a). In several long-term agricultural experiments, 14C dating indicates that the turnover time of free particulate organic matter (POM), the low-density size fraction of SOM which should contain recalcitrant plant compounds, is only ∼50 years (John et al., 2005; Rethemeyer et al., 2005). Biochemical recalcitrance, it seems, prevents decomposition of plant matter for decades at most.
Recent advances in soil chemistry and microbiology over the past decade have dramatically shifted previous ideas about SOM formation. Direct characterization of SOM via NMR spectroscopy, scanning electron microscopy, NanoSIMS, and pyrolysis-GC-MS indicates that the majority of SOM is actually microbial in origin, consisting mainly of microbial necromass fragments such as proteins, lipids, and polysaccharides (Grandy and Neff, 2008; Simpson et al., 2008; Miltner et al., 2012; Gleixner, 2013; Plaza et al., 2013; Kallenbach et al., 2016; Kopittke et al., 2020). These observations are further supported by modeling work indicating that microbial necromass makes up as much as 80% of SOM (Liang and Balser, 2011; Liang et al., 2011), suggesting that microbial metabolism and transformation of organic matter is critical to long-term storage of SOM.
These partially decomposed microbial residues can be protected from further degradation via interactions with the soil matrix, such as sorption to mineral surfaces and incorporation into soil microaggregates (<53 μm) (Adu and Oades, 1978; Jastrow et al., 1996; Balesdent et al., 2000; Six et al., 2000; Kelleher and Simpson, 2006; Lehmann et al., 2007; Kögel-Knabner et al., 2008; Cotrufo et al., 2013, 2015; Six and Paustian, 2014); organic matter protected via interaction with soil mineral surfaces is referred to as mineral-associated organic matter (MAOM) (Cotrufo et al., 2019). Because soil microbes are typically associated with the surfaces of soil minerals, microbially derived residues and necromass are more likely to be sorbed to mineral surfaces than is unprocessed, plant-derived SOM (Pett-Ridge and Firestone, 2017). Thus, MAOM mainly consists of low-molecular weight compounds that have been processed by soil microbiota, via either incorporation into microbial biomass or extracellular enzymes (Christensen, 2001; Six et al., 2001; Sanderman et al., 2014; Cotrufo et al., 2015). MAOM has been measured to have 14C ages as old as centuries to millennia (Jastrow et al., 1996; Rasmussen et al., 2005; Kögel-Knabner et al., 2008; Kleber et al., 2011), suggesting that mineral association confers a high degree of stabilization.
Despite its physical protection and observed longevity, however, even MAOM is not inherently stable or permanent. Organo-mineral interactions are reversible; the complexes can be disrupted by root exudates such as oxalic acid that can outcompete MAOM for ligand interactions with mineral surfaces, freeing MAOM and making it susceptible to decomposition (Keiluweit et al., 2015). Fluctuations in soil pH can also promote desorption of C from mineral associations (Wagai and Mayer, 2007; Rasmussen et al., 2018). Furthermore, the addition of labile C compounds, either in incubations or via root exudation, has also been shown to stimulate the decomposition of native SOM (both MAOM and POM) that is old and theoretically stable, via the so-called priming effect (Fontaine et al., 2007; Finzi et al., 2015; Murphy et al., 2015; Bernal et al., 2016). Physical disturbance such as freeze-thaw cycles, wet-dry cycles, bioturbation, and tillage can also disrupt mineral-organic matter associations (Fierer and Schimel, 2002; Herrmann and Witter, 2002; Lavelle et al., 2006). Thus, not even MAOM, theoretically the best candidate for the hypothesized stable C pool, is permanently protected from decomposition and loss.
Toward Dynamic Stability
The suggestion that observed ancient SOM is not inherently stable presents a paradox – how can a thermodynamically unstable form of C be very old if it is not stabilized in some way (Hedges et al., 2000)? Current research suggests that the observed longevity of SOM results from biological, environmental, and physicochemical constraints on SOM decomposition, a concept that is described as persistence (Box 1, Schmidt et al., 2011). Understanding persistence requires an understanding of the complexity of the soil environment and the myriad constraints on the spatial distribution and metabolism of soil microbial communities. Soil is a complex environment, with substantial variation both horizontally and vertically, and a heterogeneous 3-dimensional structure (Young et al., 2001; Ettema and Wardle, 2002; Kinyangi et al., 2006; Nunan et al., 2007; Lehmann et al., 2008; Séger et al., 2009; Tarquis et al., 2009; Young and Bengough, 2018). Though soils are often depicted as teeming with microbial life, less than 1% of the soil surface is actually colonized by microbes (Young and Crawford, 2004), and soil microbes tend to congregate in specific, resource-rich areas like the rhizosphere, detritusphere, and along preferential flow pathways (Joergensen, 2000; Bundt et al., 2001b, a; McMahon et al., 2005; Chabbi et al., 2009; Angst et al., 2016b; Sokol and Bradford, 2018). Soil aggregation and micropore development further enhances heterogeneity in resources, oxygen diffusion, and organisms (Chenu et al., 2001; Smucker et al., 2007; Kravchenko et al., 2014; Bach and Hofmockel, 2016). The size, composition, and metabolic efficiency of the soil microbial community also varies in response to resource availability, moisture, temperature, redox conditions, and disturbances (Manzoni et al., 2012; Wallenstein and Hall, 2012; Sinsabaugh et al., 2013; Schmidt et al., 2018).
Heterogeneity leads to physical isolation of microbes from SOM, and places thermodynamic and ecological constraints on microbial populations even when they do have access to degradable SOM (Rovira and Ramón Vallejo, 2002; Ekschmitt et al., 2008; Salomé et al., 2010; Dungait et al., 2012; Manzoni et al., 2012). Soil organic matter is not evenly distributed within soil; direct observations using techniques such as NEXAFS and NanoSIMS shows that the distribution and identity of SOM compounds varies even at micro- and nanometer scales due to preferential MAOM accumulation in distinct submicron soil structures, particularly within microdomains of densely arranged clay minerals (Lehmann et al., 2007, 2008; Vogel et al., 2014; Steffens et al., 2017; Kopittke et al., 2020). Variable distribution of SOM and limited pore connectivity in these microdomains constrain microbial access to both SOM and nutrients, which in turn limits the activity of soil microbial communities (Nunan, 2017; Nunan et al., 2017; Steffens et al., 2017). A direct observation study pairing micro-scale enzyme mapping with X-ray microtomography suggests that heterogenous soil pore networks may be a particularly important influence on both microbial activity and SOM stabilization (Kravchenko et al., 2019).
New hypotheses based on these findings posit that rapid cycling can still result in long-term SOM persistence, with mineral interactions temporarily slowing the flow of SOM rather than conferring permanent protection (Cagnarini et al., 2019). Empirical support for this hypothesis comes from recent high-resolution mass spectrometry work demonstrating that as dissolved organic C moves downward through the soil profile, its composition shifts toward mid-weight, saturated molecules with a high degradation index (Roth et al., 2019). This indicates that microbial consumption and subsequent transformation is a key control on SOM composition and persistence. Recent process-based modeling efforts similarly find that observed distribution of SOM stocks and 14C ages can be reproduced without requiring the assumption of permanent protection of MAOM (Robertson et al., 2018; Woolf and Lehmann, 2019). Instead of being permanently sorbed to minerals, MAOM may de-sorb and re-enter soil solution as dissolved organic C, be transported downward in the soil profile, be consumed again by soil microbes and incorporated into biomass, and eventually become re-sorbed onto mineral surfaces (Woolf and Lehmann, 2019).
Given these advances, we advocate for an expanded definition of SOM persistence that explicitly recognizes the critical importance of the movement of soil C molecules for their long-term preservation (Box 1). Interactions between SOM and soil minerals, aggregates, and other physical protection mechanisms are not permanent; instead, SOM persistence is driven by its flow throughout the heterogeneous soil environment and its interactions with both soil microbes and the physical soil matrix (Box 1 and Figure 1).
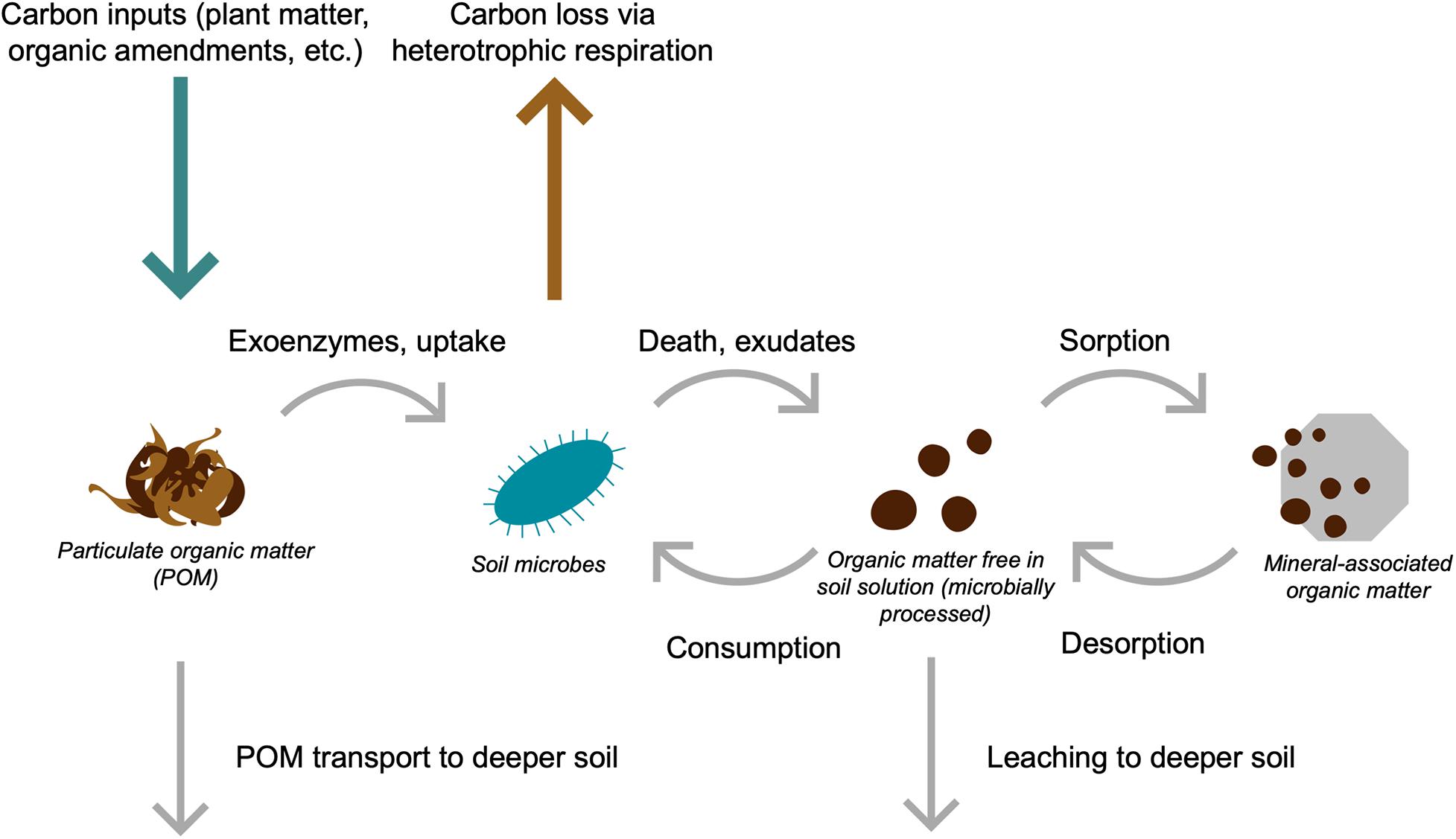
Figure 1. Conceptual illustration of soil carbon (C) flows. Carbon enters soil mainly via plant inputs (green arrow) and is lost via heterotrophic respiration (brown arrow). Plant C inputs are the precursor to particulate organic matter (POM), which can be depolymerized by microbes and taken up into biomass, or transported deeper into the soil profile as particulate matter. Microbial depolymerization, exudation, and turnover releases microbially processed C, which is free in soil solution and can sorb onto mineral surfaces to produce mineral-associated organic matter (MAOM). Mineral-associated organic matter can de-sorb from mineral surfaces and taken back up into microbial biomass or be transported to deeper soil profiles via leaching. Over time, these cycling processes result in the movement of both microbially processed C and POM into increasingly deeper soil horizons.
The soil regions most likely to accumulate microbially processed SOM are thus those where soil microbes, C substrates, and the conditions necessary for microbes to degrade those substrates do not co-occur. Such isolation is particularly pronounced in deep soils (below 30 cm), where microbial density and activity is markedly lower and highly variable (Nunan et al., 2003; Salomé et al., 2010), and SOM distribution is patchy (Chevallier et al., 2000; Don et al., 2007; Chabbi et al., 2009). At these depths, up to 90% of SOM is in the MAOM fraction, which significantly decreases its accessibility (Eusterhues et al., 2003; Moni et al., 2010; Jackson et al., 2017). Furthermore, constraints on microbial distribution and metabolism in deep soils can simultaneously suppress POM decomposition and increase its residence time (Hicks Pries et al., 2018; Sokol et al., 2018). Accordingly, significant quantities of old C can accumulate in deep soils despite low concentrations of soil C at depth, with the majority of C in the top meter of soil found deeper than 30 cm (Jobbagy and Jackson, 2000; Lorenz and Lal, 2005). Process-based models similarly predict the accumulation of very old C in deep soils when processes such as mineral sorption, microbial metabolism, and downward C transport are taken into account (Riley et al., 2014; Wieder et al., 2014; Woolf and Lehmann, 2019). However, research focusing on soil C sequestration in deeper soils is relatively rare, and should be a priority for future study, given the potential for subsoil horizons to accumulate persistent soil C.
Permanence in Policy
As interest in soil C sequestration projects deepens in the policy arena (e.g., Minasny et al., 2017; Bossio et al., 2020), it increases the need for timely translation of scientific knowledge of soil C longevity to inform effective policy. Currently, policy and science-based definitions of soil C permanence do not align. As described above, soil C longevity can best be conceptualized as persistence, in which the long-term sequestration of SOM results from the flow of C throughout a complex soil environment, where it can be transformed by soil microbes and interact with soil minerals and physicochemical structures (Box 1 and Figure 1).
In contrast, most C offset markets understand soil C longevity as resulting from the permanence of a particular C sequestration management practice (Box 1, Smith, 2005). This stems from difficulties in monitoring soil C stocks over long timeframes, and assumptions that (1) once the period of benefits/requirement is over, agricultural land managers will end any soil C sequestration practices (e.g., cover cropping, reduced tillage) (Hediger, 2009), and (2) once the C sequestration practice is ceased, C will be lost from soils at a rate faster than the C accrued (Smith, 2005). Because most C trading mechanisms require that offsets be maintained for a minimum of 100 years, this translates to a requirement that any management practices to sequester soil C be maintained for 100 years, a cumbersome and unrealistic expectation for most land managers (though there has been recent experimentation with shorter permanence requirements, see below). In recent years, incentives-based programs without such time requirements have become more common (e.g., the California Healthy Soils Incentive Program), but permanence requirements still remain a hurdle in offset markets (e.g., cap-and-trade programs) (von Unger and Emmer, 2018).
Clear translation of scientific knowledge could address policymaker concerns about the permanence of sequestered soil C, resulting in wider adoption of soil C sequestration efforts. In practice, changes in agricultural management are unlikely to result in loss of all soil C that was newly stored, calling into question the necessity and efficacy of 100-year permanence requirements. This has been demonstrated at the Rothamsted long-term agricultural experiment, wherein farmyard manure was applied to a cereal cropping system for twenty years in the late nineteenth century and then stopped. Nearly 150 years later, this soil still contains about 2.5 times as much SOM as soil that never received manure (Johnston et al., 2009). Similarly, studies focusing on conversion of no-till fields to conventional tillage have found that infrequent tillage (once a year or less) does not result in significant C loss relative to no-till (Conant et al., 2007; Dimassi et al., 2013). Translocation of C from topsoil into deeper soil horizons likely helps facilitate long-term C sequestration, even if management alters conditions in surface soils. Thus, a single tillage event is unlikely to cause the massive loss of soil C that is commonly assumed in economic models (Hediger, 2009). Sequestration practices that are centered around biochar application are also not likely to result in emissions reversals if the practice is ended (i.e., ceasing to apply further biochar is not likely to cause C emissions) (Lehmann, 2007). Even if ending a particular C-sequestering management practice did mean all the newly gained C would be lost, decay kinetics predict that it would take several years at a minimum (Schimel et al., 1994; Giardina and Ryan, 2000). The assumption that C will be lost so quickly as to be essentially immediate is therefore likely not accurate.
Synergistic efforts between scientists and policymakers to understand and address these soil C permanence questions are particularly critical now, as interest in soil C sequestration projects is increasing (von Unger and Emmer, 2018), necessitating the development of data-based policy mechanisms to overcome potential non-permanence of soil C sequestration management practices. The fact that C sinks in soil are not strictly permanent (i.e., there is potential risk of reversal) is not unusual among C sequestration solutions. Such risks also exist in reforestation and afforestation projects, where C is sequestered in aboveground forest biomass. The risk of sequestration reversal in forest biomass projects has been successfully managed through insurance-like schemes called buffers (Murray et al., 2007), suggesting that permanence is an important, but manageable, issue. A similar buffer scheme is used by the Alberta Offset System to protect C offset credits generated from conservation cropping against potential future practice reversal (Alberta Agriculture and Forestry, 2020). Shorter, 25-year permanence periods have also been successfully used in the Australian Carbon Farming Initiative, one of the few national-level C financing schemes to include improved soil management as a creditable offset (Australia Clean Energy Regulator, 2019). Projects opting for the shorter permanence period receive fewer credits than they would for a 100-year permanence period, but gain flexibility. These existing policy precedents, combined with a better scientific understanding of soil C permanence, vis-à-vis persistence, can help policymakers evaluate potential performance of climate projects on soil C sequestration and inform necessary funding structures (Bossio et al., 2020).
Discussion
In the flow-based model of C persistence developed here, SOM is simultaneously cycled through microbial biomass and stored via interactions with soil minerals that effectively introduce friction into the flow of C through the soil profile. These interactions between soil microbial communities and the physical soil matrix, with downward movement of SOM, ultimately result in the accumulation of persistent SOM stocks. Carbon cycling also supports soil health goals; one critical co-benefit of building SOM is improved nutrient availability and cycling, which can improve soil fertility and support higher crop yields while reducing the need for chemical fertilizers (Oldfield et al., 2018). Because nutrient release and cycling depends on microbial decomposition of SOM, this co-benefit has historically been understood to be at odds with intentions of sequestering soil C (Janzen, 2006). However, in a flow-based understanding of SOM persistence, consumption and transformation by soil microbes is crucial for producing MAOM and allowing movement and transformation of SOM. Thus, restoring soil biological function and C sequestration are fundamentally interwoven, rather than contradictory, goals.
Agricultural management strategies with potential to promote soil C storage have been detailed in several recent papers (Paustian et al., 2016; Chenu et al., 2019). Many of these strategies have potential to contribute to soil C flows as described in this paper. Understanding the specific mechanisms by which different management strategies may contribute to soil C flows is critical for informing selection of strategies most likely to result in persistent C sequestration (Table 1). We argue that optimal management strategies to enhance both the sequestration potential of agricultural soils and promote the co-benefits of increased SOM are those that support a robust biological C cycle and promote C flow. This, in turn, supports microbial processing of plant organic matter and subsequent translocation of microbially processed SOM to deeper soil horizons, building up a stock of persistent soil C over time. Based on this framework, the key principles for supporting C flows that efficiently produce persistent SOM are: 1) maintain C inputs to agricultural soils, particularly high-quality C substrates that support efficient microbial metabolism, and 2) reduce soil disturbances that disrupt biological and physicochemical interactions. Increasing plant inputs to soils, whether from living plants or their organic residues, increases resources available to soil microbes, promoting C flow. Partially decomposed organic amendments such as compost and manure are especially good C sources, as organic molecules with simple structures and relatively low C:N ratios are more efficiently assimilated into microbial biomass (Bradford et al., 2013; Cotrufo et al., 2013) and sorbed to mineral surfaces (Haddix et al., 2016). This has been supported empirically by several recent experiments in California showing that compost and winter cover crops additions result in significantly greater soil C stock gains than winter cover crops alone (Tautges et al., 2019; White et al., 2020). Maintaining nutrient availability, either via C inputs themselves (i.e., nutrient-rich compost) or with supplementary fertilizer additions may also be necessary to support efficient microbial metabolism (Kirkby et al., 2013). Soil disturbances that repeatedly disrupt soil aggregates, such as frequent tillage, are likely to suppress C flows by shifting the balance of interacting mechanisms sharply toward decomposition, resulting in C loss (Six et al., 2000; Lal, 2004b; Oost et al., 2007). Evidence suggests, however, that infrequent and/or less destructive tillage practices do not cause the same disruption to soil aggregates and do not result in significant C loss (Conant et al., 2007; Cooper et al., 2016).
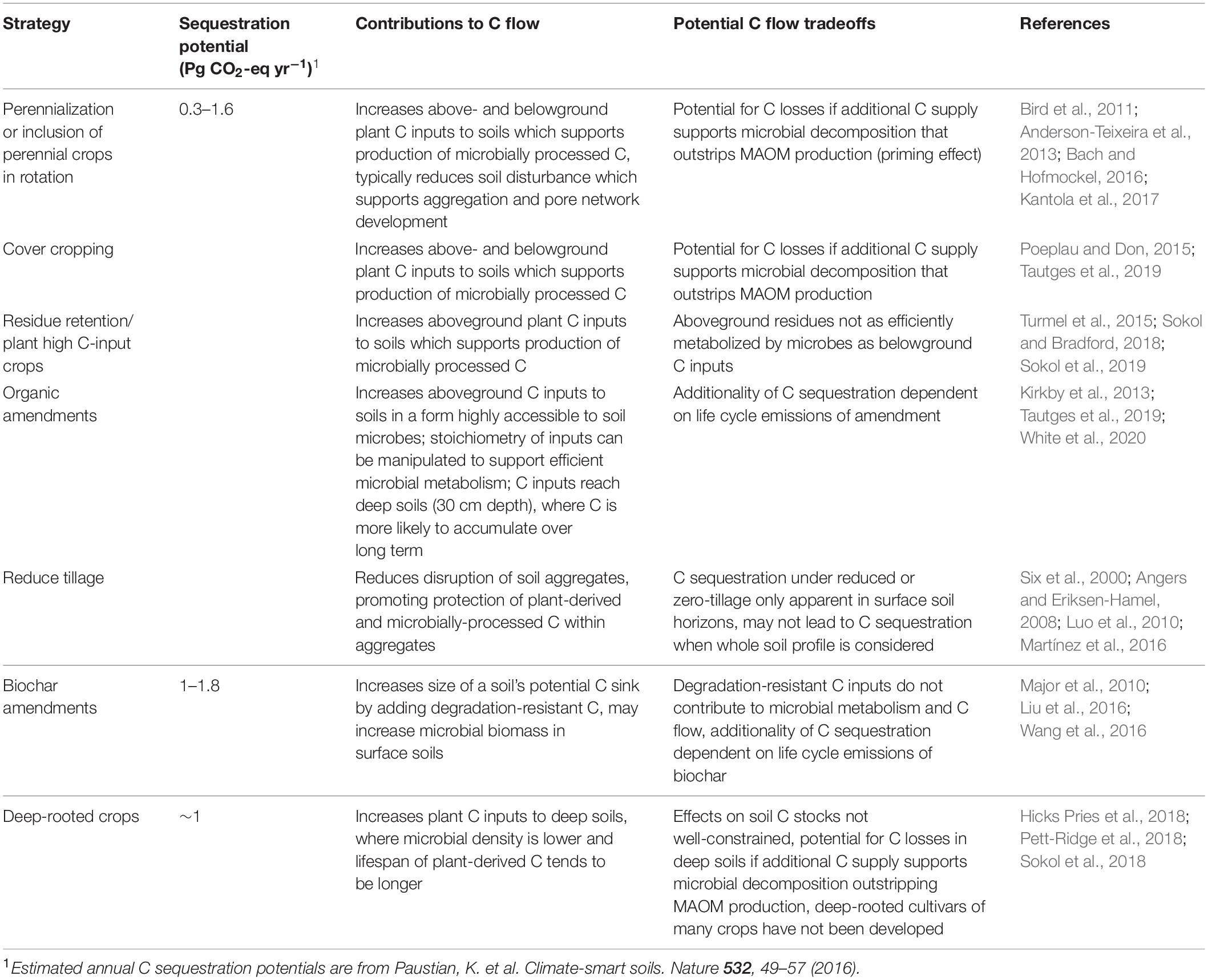
Table 1. Sequestration potential, contributions to C flow, and trade-offs/constraints of selected cropland management practices (compiled from Paustian et al., 2016 and Chenu et al., 2019) that are most related to the soil C flow conceptual model developed in this paper.
Several key differences emerge between the management practices associated with a flow-based model of soil C persistence and current policy representations of soil C storage, exemplifying how a unified understanding of soil C persistence is necessary to inform climate policy related to agricultural soil management. For example, the C flow model of persistence emphasizes the importance of microbially accessible C inputs in order to build SOM. However, currently an agricultural management practice with some support as a policy for climate change mitigation is incorporation of pyrogenic organic matter (biochar) (Whitman and Lehmann, 2009; Woolf et al., 2018; Pourhashem et al., 2019). Because pyrogenic organic matter is less readily degradable than many other carbon inputs (Glaser et al., 2002; Nguyen and Lehmann, 2009; Major et al., 2010; but see Hamer et al., 2004) it may effectively increase the size of a given soil’s potential C sink. Climate mitigation policies based on knowledge of C flows may thus prioritize management strategies that promote C flows, such as regular compost additions or planting perennial crops, in addition to inputs of less decomposable C such as biochar. Furthermore, fears about non-permanent implementation of soil C sequestration management practices, particularly zero-till, have been a major barrier to inclusion of soil projects in most C financing markets (Smith, 2005). Awareness of current scientific knowledge regarding mechanisms of soil C persistence could therefore inform which agricultural management practices should be prioritized, provide more confidence about the retention of SOM, and facilitate wider adoption.
Simultaneously, key policymaker questions have currently gone unanswered by scientists; harmonizing concepts and terminology across disciplines can facilitate scientific research into these critical unknowns. One lingering question is how long soil C will remain if a C-sequestering management practice is ceased. Though a few studies have indicated that loss of sequestered C may not be a concern in the case of occasional tillage (Conant et al., 2007; Cooper et al., 2016), very little field-based research has considered the lifespan of newly sequestered C when management practices are reverted.
Future research is also needed to understand land manager motivations in order to determine realistic expectations for time frames of management practice adoption, as well as identify appropriate incentives and support structures. Though incentives may be necessary to make adoption of C sequestration practices financially feasible, there is little evidence that land managers will immediately revert back to previous management practices when incentives periods end, as is commonly assumed in economic models. For farmers who have already adopted management practices that increase SOM, the agronomic benefits of these practices are often viewed as more important than short-term financial incentives (Conservation Technology Information Center., 2015). More recently, many farmers have been adopting soil C sequestration practices with the goal of supporting long-term soil health (Carlisle, 2016); soil health co-benefits associated with increasing SOM include increased water-holding capacity and fertility, which may boost agricultural yields, providing an economic incentive (Oldfield et al., 2018). In Australia’s Carbon Farming Initiative, early research suggests having access to information about the co-benefits of C sequestration, and knowing other growers are implementing these practices, is more important to farmers than financial incentives (Kragt et al., 2017).
Additional investigation to identify target regions for soil C sequestration based on sink capacity would also be valuable. There are concerns in both the soil science and public policy sectors that there is an upper limit to how much C soil can store – that is, the soil C sink can be saturated (Schlesinger, 2000; Smith, 2005; Stewart et al., 2007; Sommer and Bossio, 2014). Current evidence suggests that a soil’s capacity to accumulate MAOM is finite due to limited mineral surfaces for SOM adsorption. Mineral-associated organic matter capacity depends on physicochemical properties such as clay content and specific soil mineralogy (Six et al., 2002b; Cotrufo et al., 2019; Wiesmeier et al., 2019). Examples of soil with theoretically high capacity for MAOM sequestration include those containing clays with high surface area, such as vermiculites, as well as non-clay minerals with high specific surface areas such as allophane (Wiesmeier et al., 2019). Recent work examining thousands of soil profiles suggests that the physicochemical predictors of SOM storage vary across environments, with exchangeable calcium emerging as a strong predictor of SOM in arid environments, and iron and aluminum compounds becoming more important in wetter, more acidic environments. This calls for more nuanced research into relationships between soil mineralogy and MAOM capacity (Rasmussen et al., 2018). On the other hand, POM, while more readily available to soil microbes because it is unprotected, theoretically cannot saturate because POM accumulation does not require unoccupied mineral surfaces (Lavallee et al., 2019). Although many soil properties that promote C sequestration cannot easily be altered by management, this knowledge can help guide where to focus C sequestration incentives. Soils that could be considered good candidates for C sequestration projects are those with a large potential sink size; e.g., deep and high clay soils that are relatively depleted in SOM relative to their maximum sink size. Pedo-climatic context plays a large role in defining sink potential (Chenu et al., 2019); new work additionally suggests that deep soil horizons (>30 cm depth) may have high C sink potential. In a meta-analysis of 13C isotopes in 41 agricultural soil profiles, nearly a quarter of newly accumulated soil C was found below 30 cm within 20 years, and the turnover time of this C was four times slower than newly accumulated C in surface soils (Balesdent et al., 2017). Similarly, a recent long-term experiment in a California organic agricultural system found substantial C storage in the 60–200 cm depth in response to compost additions and winter cover crops (Tautges et al., 2019). This may be due to relatively greater mineral surface area available for MAOM adsorption than in surface soils, as well as greater constraints on microbial activity which limit POM degradation. Results such as these suggest that further research is necessary to understand the potential and to identify the opportunities for targeted efforts to build soil C stocks in subsoils.
Finally, although the scientific community has recently made great strides in understanding SOM persistence, there remains a lack of reliable indicators and tools for practitioners and policymakers to assess soil C flows and quantify the success of soil C sequestration efforts. Measurements that divide soil C into “labile” and “protected” fractions, such as density fractionation to separate MAOM from POM (Six et al., 2002a; Sollins et al., 2009; Lavallee et al., 2019), or permanganate-oxidizable C (Culman et al., 2012; Hurisso et al., 2016; Morrow et al., 2016; Wade et al., 2020), should provide insight into the flow and transformations of C throughout the soil system. Measurements that capture soil biological capacity, such as metabolic quotient, microbial biomass or microbial growth rate may also be useful indicators (Kallenbach et al., 2015; Bailey et al., 2018; Singh and Gupta, 2018), given the role of soil microbes as both producers and consumers of stabilized SOM. These dynamic properties need to be assessed across multiple soil depths in order to link knowledge of C transformations and movement to quantification of whole-profile C accumulation. Such efforts will also help elucidate which, if any, proxy measurements in surface soils might indicate C movement and accumulation in deeper horizons.
Though numerous terms have been used to describe SOM transformation and lifespan as our knowledge of the nature of soil C has evolved, scientists have generally assumed there to be some soil C pools with an extremely long turnover time (Box 1). This assumption has been codified into most carbon-climate models and facilitated a broader understanding that some forms of soil C can be stored more or less permanently, and other forms will be lost to microbial decomposition. However, current scientific understanding of soil C storage has largely moved beyond the idea of humified or recalcitrant C, and instead reveals a dynamic world dominated by spatial and temporal complexity, biotic and abiotic interactions, and the continuous C movement and transformation. In this flow-based model, C pools with infinitely long turnover times are no longer necessary to explain observed global patterns of soil C distribution and age (Woolf and Lehmann, 2019). We argue that a widespread understanding of soil C accumulation and persistence as the result of flow and transformation of C as it moves through the soil profile, and the use of terminology that reflects these mechanisms, can support policy development to promote agricultural management strategies that both build persistent soil C stocks and support soil health. Many of the practices that support soil biological activity and C flows, and therefore both soil health and persistent C accumulation, are not new suggestions and have been gaining in popularity in recent years (Vermeulen et al., 2019). Implementation can be supported by the development of policies that promote and incentivize management practices that increase soil biological capacity. Concurrently, scientific advances can support these goals by addressing policymaker questions and concerns, as well as defining useful indicators for assessing C flows. Harmonizing concepts and language used by both policymakers and scientists is thus essential to stimulate design of science-based policies.
Conclusion
Enhancing SOM in agricultural soils is an important and readily implementable practice that contributes to natural climate mitigation solutions (Smith, 2016; Griscom et al., 2017; Zomer et al., 2017; Bossio et al., 2020), and, critically, with significant soil health co-benefits for global agricultural yields and food security (Lal, 2004a). Currently we are not making use of soil’s full C sink potential, which is estimated to be as high as 5.3 Gt of CO2-equivalent yr–1 (Fuss et al., 2018), not including biochar. Implementing agricultural management practices that support soil microbial communities promotes active C cycling in surface soils, with benefits for soil fertility and structure, as well as long-term accumulation of SOM, particularly in deeper soil horizons.
Policymaker concerns about the permanence of sequestered soil C present a significant barrier to the wide-scale funding of soil C conservation projects as a climate mitigation tool (Smith et al., 2019). Different terminology and definitions used by scientists and policymakers to describe soil C longevity has both slowed down transfer of new scientific understanding of soil C sequestration to policymakers, as well as insulated scientists from targeting the research needs of policymakers. Integrating new scientific findings regarding soil carbon longevity into data-based C policies is critical for broader adoption of agricultural soil C sequestration projects that could expand the contribution of soils to climate change mitigation on a global scale.
Author’s Note
Any opinions, findings, conclusions, or recommendations expressed in this publication are those of the author(s) and do not necessarily reflect the view of the National Institute of Food and Agriculture (NIFA) or the United States Department of Agriculture (USDA).
Author Contributions
KD, DB, and KS contributed to the ideas presented in this manuscript. KD wrote the first draft. All authors contributed to manuscript revisions.
Funding
This work was supported in part by the USDA National Institute of Food and Agriculture, Hatch Project CA-2122-H and Grant #11925159. We also acknowledge support from the UC Office of the President’s Multi-Campus Research Programs and Initiatives (MR-15-328473). Additional support came from the Craig and Susan McCaw Foundation and the Nature Conservancy.
Conflict of Interest
The authors declare that the research was conducted in the absence of any commercial or financial relationships that could be construed as a potential conflict of interest.
References
Adu, J. K., and Oades, J. M. (1978). Physical factors influencing decomposition of organic materials in soil aggregates. Soil Biol. Biochem. 10, 109–115. doi: 10.1016/0038-0717(78)90080-9
Alberta Agriculture and Forestry (2020). Agricultural Carbon Offsets. Available online at: https://www.alberta.ca/agricultural-carbon-offsets.aspx (accessed May 4, 2020).
Amundson, R., and Biardeau, L. (2018). Opinion: soil carbon sequestration is an elusive climate mitigation tool. Proc. Natl. Acad. Sci. U.S.A. 115, 11652–11656. doi: 10.1073/pnas.1815901115
Anderson-Teixeira, K. J., Masters, M. D., Black, C. K., Zeri, M., Hussain, M. Z., Bernacchi, C. J., et al. (2013). Altered belowground carbon cycling following land-use change to perennial bioenergy crops. Ecosystems 16, 508–520. doi: 10.1007/s10021-012-9628-x
Angers, D. A., and Eriksen-Hamel, N. S. (2008). Full-inversion tillage and organic carbon distribution in soil profiles: a meta-analysis. Soil Sci. Soc. Am. J. 72, 1370–1370. doi: 10.2136/sssaj2007.0342
Angst, G., Heinrich, L., Kögel-Knabner, I., and Mueller, C. W. (2016a). The fate of cutin and suberin of decaying leaves, needles and roots – Inferences from the initial decomposition of bound fatty acids. Org. Geochem. 95, 81–92. doi: 10.1016/j.orggeochem.2016.02.006
Angst, G., Kögel-Knabner, I., Kirfel, K., Hertel, D., and Mueller, C. W. (2016b). Spatial distribution and chemical composition of soil organic matter fractions in rhizosphere and non-rhizosphere soil under European beech (Fagus sylvatica L.). Geoderma 264, 179–187. doi: 10.1016/j.geoderma.2015.10.016
Australia Clean Energy Regulator (2019). Permanence Obligations. Available online at: http://www.cleanenergyregulator.gov.au/ERF/Choosing-a-project-type/Opportunities-for-the-land-sector/Permanence-obligations#permanence (accessed March 26, 2020).
Bach, E. M., and Hofmockel, K. S. (2016). A time for every season: soil aggregate turnover stimulates decomposition and reduces carbon loss in grasslands managed for bioenergy. GCB Bioenergy 8, 588–599. doi: 10.1111/gcbb.12267
Bailey, V. L., Bond-Lamberty, B., DeAngelis, K., Grandy, A. S., Hawkes, C. V., Heckman, K., et al. (2018). Soil carbon cycling proxies: understanding their critical role in predicting climate change feedbacks. Glob. Change Biol. 24, 895–905. doi: 10.1111/gcb.13926
Bailey, V., Pries, C., and Lajtha, K. (2019). What do we know about soil carbon destabilization? Environ. Res. Lett. doi: 10.1088/1748-9326/ab2c11
Balesdent, J., Basile-Doelsch, I., Chadoeuf, J., Cornu, S., Fekiacova, Z., Fontaine, S., et al. (2017). Turnover of deep organic carbon in cultivated soils: an estimate from a review of isotope data. Biotechnol. Agron. Soc. Environ. 21, 181–190.
Balesdent, J., Chenu, C., and Balabane, M. (2000). Relationship of soil organic matter dynamics to physical protection and tillage. Soil Tillage Res. 53, 215–230. doi: 10.1016/S0167-1987(99)00107-5
Batjes, N. H. (1996). Total carbon and nitrogen in the soils of the world. Eur. J. Soil Sci. 47, 151–163. doi: 10.1111/j.1365-2389.1996.tb01386.x
Baveye, P. C., Berthelin, J., Tessier, D., and Lemaire, G. (2018). The “4 per 1000” initiative: a credibility issue for the soil science community? Geoderma 309, 118–123. doi: 10.1016/j.geoderma.2017.05.005
Baveye, P. C., Schnee, L. S., Boivin, P., Laba, M., and Radulovich, R. (2020). Soil organic matter research and climate change: merely re-storing carbon versus restoring soil functions. Front. Environ. Sci. 8:579904. doi: 10.3389/fenvs.2020.579904
Bernal, B., Mckinley, D. C., Hungate, B. A., White, P. M., Mozdzer, T. J., and Megonigal, J. P. (2016). Limits to soil carbon stability: deep, ancient soil carbon decomposition stimulated by new labile organic inputs. Soil Biol. Biochem. 98, 85–94. doi: 10.1016/j.soilbio.2016.04.007
Bird, J. A., Herman, D. J., and Firestone, M. K. (2011). Rhizosphere priming of soil organic matter by bacterial groups in a grassland soil. Soil Biol. Biochem. 43, 718–725. doi: 10.1016/j.soilbio.2010.08.010
Bossio, D. A., Cook-Patton, S. C., Ellis, P. W., Fargione, J., Sanderman, J., Smith, P., et al. (2020). The role of soil carbon in natural climate solutions. Nat. Sustain. 3, 1-8. doi: 10.1038/s41893-020-0491-z
Bradford, M. A., Keiser, A. D., Davies, C. A., Mersmann, C. A., and Strickland, M. S. (2013). Empirical evidence that soil carbon formation from plant inputs is positively related to microbial growth. Biogeochemistry 113, 271–281. doi: 10.1007/s10533-012-9822-0
Bundt, M., Jäggi, M., Blaser, P., Siegwolf, R., and Hagedorn, F. (2001a). Carbon and nitrogen dynamics in preferential flow paths and matrix of a forest soil. Soil Sci. Soc.Am. J. 65, 1529–1538. doi: 10.2136/sssaj2001.6551529x
Bundt, M., Widmer, F., Pesaro, M., Zeyer, J., and Blaser, P. (2001b). Preferential flow paths: biological ‘hot spots’ in soils. Soil Biol. Biochem. 33, 729–738. doi: 10.1016/S0038-0717(00)00218-2
Cagnarini, C., Blyth, E., Emmett, B. A., Evans, C. D., Griffiths, R. I., Keith, A., et al. (2019). Zones of influence for soil organic matter dynamics: a conceptual framework for data and models. Glob. Change Biol. 25, 3996–4007. doi: 10.1111/gcb.14787
Campbell, C. A., Paul, E. A., Rennie, D. A., and Mccallum, K. J. (1967). Applicability of the carbon-dating method of analysis to soil humus studies. Soil Sci. 104, 217–224. doi: 10.1097/00010694-196709000-00010
Carlisle, L. (2016). Factors influencing farmer adoption of soil health practices in the United States: a narrative review. Agroecol. Sustain. Food Syst. 40, 583–613. doi: 10.1080/21683565.2016.1156596
Chabbi, A., Kögel-Knabner, I., and Rumpel, C. (2009). Stabilised carbon in subsoil horizons is located in spatially distinct parts of the soil profile. Soil Biol. Biochem. 41, 256–261. doi: 10.1016/j.soilbio.2008.10.033
Chenu, C., Angers, D. A., Barré, P., Derrien, D., Arrouays, D., and Balesdent, J. (2019). Increasing organic stocks in agricultural soils: knowledge gaps and potential innovations. Soil Tillage Res. 188, 41–52. doi: 10.1016/j.still.2018.04.011
Chenu, C., Hassink, J., and Bloem, J. (2001). Short-term changes in the spatial distribution of microorganisms in soil aggregates as affected by glucose addition. Biol. Fertil. Soils 34, 349–356. doi: 10.1007/s003740100419
Chevallier, T., Voltz, M., Blanchart, E., Chotte, J. L., Eschenbrenner, V., Mahieu, M., et al. (2000). Spatial and temporal changes of soil C after establishment of a pasture on a long-term cultivated vertisol (Martinique). Geoderma 94, 43–58. doi: 10.1016/S0016-7061(99)00064-6
Christensen, B. T. (2001). Physical fractionation of soil and structural and functional complexity in organic matter turnover. Eur. J. Soil Sci. 52, 345–353. doi: 10.1046/j.1365-2389.2001.00417.x
Conant, R. T., Easter, M., Paustian, K., Swan, A., and Williams, S. (2007). Impacts of periodic tillage on soil C stocks: a synthesis. Soil Tillage Res. 95, 1–10. doi: 10.1016/j.still.2006.12.006
Conservation Technology Information Center. (2015). Report of the 2014–2015 cover crop survey. West Lafayette, IN: Joint publication of the CTIC and the North Central Region Sustainable Agriculture Research and Education Program.
Cooper, J., Baranski, M., Stewart, G., Nobel-de Lange, M., Bàrberi, P., Fließbach, A., et al. (2016). Shallow non-inversion tillage in organic farming maintains crop yields and increases soil C stocks: a meta-analysis. Agron. Sustain. Dev. 36:22. doi: 10.1007/s13593-016-0354-1
Cotrufo, M. F., Ranalli, M. G., Haddix, M. L., Six, J., and Lugato, E. (2019). Soil carbon storage informed by particulate and mineral-associated organic matter. Nat. Geosci. 12, 989–994. doi: 10.1038/s41561-019-0484-6
Cotrufo, M. F., Soong, J. L., Horton, A. J., Campbell, E. E., Haddix, M. L., Wall, D. H., et al. (2015). Formation of soil organic matter via biochemical and physical pathways of litter mass loss. Nat. Geosci. 8, 776–779. doi: 10.1038/ngeo2520
Cotrufo, M. F., Wallenstein, M. D., Boot, C. M., Denef, K., and Paul, E. (2013). The Microbial Efficiency-Matrix Stabilization (MEMS) framework integrates plant litter decomposition with soil organic matter stabilization: do labile plant inputs form stable soil organic matter? Glob. Change Biol. 19, 988–995. doi: 10.1111/gcb.12113
Culman, S. W., Snapp, S. S., Mirksy, S. B., Beniston, J., Franzluebbers, A. J., Lal, R., et al. (2012). Permanganate oxidizable carbon reflects a processed soil fraction that is sensitive to management. Soil Sci. Soc. Am. J. 76, 494–494. doi: 10.2136/sssaj2011.0286
Dignac, M.-F., Bahri, H., Rumpel, C., Rasse, D. P., Bardoux, G., Balesdent, J., et al. (2005). Carbon-13 natural abundance as a tool to study the dynamics of lignin monomers in soil: an appraisal at the Closeaux experimental field (France). Geoderma 128, 3–17. doi: 10.1016/j.geoderma.2004.12.022
Dimassi, B., Cohan, J. P., Labreuche, J., and Mary, B. (2013). Changes in soil carbon and nitrogen following tillage conversion in a long-term experiment in Northern France. Agricult. Ecosyst. Environ. 169, 12–20. doi: 10.1016/j.agee.2013.01.012
Don, A., Schumacher, J., Scherer-Lorenzen, M., Scholten, T., and Schulze, E.-D. (2007). Spatial and vertical variation of soil carbon at two grassland sites — Implications for measuring soil carbon stocks. Geoderma 141, 272–282. doi: 10.1016/j.geoderma.2007.06.003
Dungait, J. A. J., Hopkins, D. W., Gregory, A. S., and Whitmore, A. P. (2012). Soil organic matter turnover is governed by accessibility not recalcitrance. Glob. Change Biol. 18, 1781–1796. doi: 10.1111/j.1365-2486.2012.02665.x
Ekschmitt, K., Kandeler, E., Poll, C., Brune, A., Buscot, F., Friedrich, M., et al. (2008). Soil-carbon preservation through habitat constraints and biological limitations on decomposer activity. J. Plant Nutr. Soil Sci. 171, 27–35. doi: 10.1002/jpln.200700051
Ettema, C. H., and Wardle, D. A. (2002). Spatial soil ecology. Trends Ecol. Evol. 17, 51–72. doi: 10.1016/S0169-5347(02)02496-5
Eusterhues, K., Rumpel, C., Kleber, M., and Kögel-Knabner, I. (2003). Stabilisation of soil organic matter by interactions with minerals as revealed by mineral dissolution and oxidative degradation. Org. Geochem. 34, 1591–1600. doi: 10.1016/j.orggeochem.2003.08.007
Fierer, N., and Schimel, J. P. (2002). Effects of drying–rewetting frequency on soil carbon and nitrogen transformations. Soil Biol. Biochem. 34, 777–787. doi: 10.1016/S0038-0717(02)00007-X
Finzi, A. C., Abramoff, R. Z., Spiller, K. S., Brzostek, E. R., Darby, B. A., Kramer, M. A., et al. (2015). Rhizosphere processes are quantitatively important components of terrestrial carbon and nutrient cycles. Glob. Change Biol. 21, 2082–2094. doi: 10.1111/gcb.12816
Fontaine, S., Barot, S., Barré, P., Bdioui, N., Mary, B., and Rumpel, C. (2007). Stability of organic carbon in deep soil layers controlled by fresh carbon supply. Nature 450, 277–280. doi: 10.1038/nature06275
Fuss, S., Lamb, W. F., Callaghan, M. W., Hilaire, J., Creutzig, F., Amann, T., et al. (2018). Negative emissions - Part 2: costs, potentials and side effects. Environ. Res. Lett. 13:063002. doi: 10.1088/1748-9326/aabf9f
Giardina, C. P., and Ryan, M. G. (2000). Evidence that decomposition rates of organic carbon in mineral soil do not vary with temperature. Nature 404, 858–861. doi: 10.1038/35009076
Glaser, B., Lehmann, J., and Zech, W. (2002). Ameliorating physical and chemical properties of highly weathered soils in the tropics with charcoal – a review. Biol. Fertil. Soils 35, 219–230. doi: 10.1007/s00374-002-0466-4
Gleixner, G. (2013). Soil organic matter dynamics: a biological perspective derived from the use of compound-specific isotopes studies. Ecol. Res. 28, 683-695. doi: 10.1007/s11284-012-1022-9
Grandy, A. S., and Neff, J. C. (2008). Molecular C dynamics downstream: the biochemical decomposition sequence and its impact on soil organic matter structure and function. Sci. Total Environ. 404, 297–307. doi: 10.1016/j.scitotenv.2007.11.013
Griscom, B. W., Adams, J., Ellis, P. W., Houghton, R. A., Lomax, G., Miteva, D. A., et al. (2017). Natural climate solutions. Proc. Natl. Acad. Sci. U.S.A. 114, 11645–11650. doi: 10.1073/pnas.1710465114
Haddix, M. L., Paul, E. A., and Cotrufo, M. F. (2016). Dual, differential isotope labeling shows the preferential movement of labile plant constituents into mineral-bonded soil organic matter. Glob. Change Biol. 22, 2301–2312. doi: 10.1111/gcb.13237
Hamer, U., Marschner, B., Brodowski, S., and Amelung, W. (2004). Interactive priming of black carbon and glucose mineralisation. Org. Geochem. 35, 823–830. doi: 10.1016/j.orggeochem.2004.03.003
Hedges, J. I. (1988). Polymerization of humic substances in natural environments. Hum. Subst. Role Environ. 39, 605–625.
Hedges, J. I., Eglinton, G., Hatcher, P. G., Kirchman, D. L., Arnosti, C., Derenne, S., et al. (2000). The molecularly-uncharacterized component of nonliving organic matter in natural environments. Org. Geochem. 31, 945–958. doi: 10.1016/S0146-6380(00)00096-6
Hediger, W. (2009). “The non-permanence of optimal soil carbon sequestration,” in Proceedings of the 83rd Annual Conference of the Agricultural Economics Society, Dublin, 1–32.
Heim, A., and Schmidt, M. W. I. (2007). Lignin turnover in arable soil and grassland analysed with two different labelling approaches. Eur. J. Soil Sci. 58, 599–608. doi: 10.1111/j.1365-2389.2006.00848.x
Herrmann, A., and Witter, E. (2002). Sources of C and N contributing to the flush in mineralization upon freeze–thaw cycles in soils. Soil Biol. Biochem. 34, 1495–1505. doi: 10.1016/S0038-0717(02)00121-9
Hicks Pries, C. E., Sulman, B. N., West, C., O’Neill, C., Poppleton, E., Porras, R. C., et al. (2018). Root litter decomposition slows with soil depth. Soil Biol. Biochem. 125, 103–114. doi: 10.1016/j.soilbio.2018.07.002
Hungate, B. A., Dukes, J. S., Shaw, M. R., Luo, Y., and Field, C. B. (2003). Nitrogen and climate change. Science 302, 1512–1513. doi: 10.1126/science.1091390
Hurisso, T. T., Culman, S. W., Horwath, W. R., Wade, J., Cass, D., Beniston, J. W., et al. (2016). Comparison of permanganate-oxidizable carbon and mineralizable carbon for assessment of organic matter stabilization and mineralization. Soil Sci. Soc. Am. J. 80, 1352–1364. doi: 10.2136/sssaj2016.04.0106
Jackson, R. B., Lajtha, K., Crow, S. E., Hugelius, G., Kramer, M. G., and Piñeiro, G. (2017). The ecology of soil carbon: pools, vulnerabilities, and biotic and abiotic controls. Annu. Rev. Ecol. Evol. Syst. 48:annurev–ecolsys–112414–054234. doi: 10.1146/annurev-ecolsys-112414-054234
Janzen, H. H. (2006). The soil carbon dilemma: shall we hoard it or use it? Soil Biol. Biochem. 38, 419–424. doi: 10.1016/j.soilbio.2005.10.008
Jastrow, J. D., Miller, R. M., and Boutton, T. W. (1996). Carbon dynamics of aggregate-associated organic matter estimated by carbon-13 natural abundance. Soil Sci. Soc. Am. J. 60, 801–801. doi: 10.2136/sssaj1996.03615995006000030017x
Jilling, A., Keiluweit, M., Contosta, A. R., Frey, S., Schimel, J., Schnecker, J., et al. (2018). Minerals in the rhizosphere: overlooked mediators of soil nitrogen availability to plants and microbes. Biogeochemistry 139, 103–122. doi: 10.1007/s10533-018-0459-5
Jobbagy, E. G., and Jackson, R. B. (2000). The vertical distribution of soil organic carbon and its relation to climate and vegetation. Ecol. Appl. 10, 423–436. doi: 10.1890/1051-0761(2000)010[0423:tvdoso]2.0.co;2
Joergensen, R. G. (2000). Ergosterol and microbial biomass in the rhizosphere of grassland soils. Soil Biol. Biochem. 32, 647–652. doi: 10.1016/S0038-0717(99)00191-1
John, B., Yamashita, T., Ludwig, B., and Flessa, H. (2005). Storage of organic carbon in aggregate and density fractions of silty soils under different types of land use. Geoderma 128, 63–79. doi: 10.1016/j.geoderma.2004.12.013
Johnston, A. E., Poulton, P. R., and Coleman, K. (2009). Chapter 1 Soil Organic Matter. Its Importance in Sustainable Agriculture and Carbon Dioxide Fluxes. 1st edn. Amsterdam: Elsevier Inc, doi: 10.1016/S0065-2113(08)00801-8
Kallenbach, C. M., Frey, S. D., and Grandy, A. S. (2016). Direct evidence for microbial-derived soil organic matter formation and its ecophysiological controls. Nat. Commun. 7, 1–10. doi: 10.1038/ncomms13630
Kallenbach, C. M., Grandy, A. S., Frey, S. D., and Diefendorf, A. F. (2015). Microbial physiology and necromass regulate agricultural soil carbon accumulation. Soil Biol. Biochem. 91, 279–290. doi: 10.1016/j.soilbio.2015.09.005
Kantola, I. B., Masters, M. D., and DeLucia, E. H. (2017). Soil particulate organic matter increases under perennial bioenergy crop agriculture. Soil Biol. Biochem. 113, 184–191. doi: 10.1016/j.soilbio.2017.05.023
Keiluweit, M., Bougoure, J. J., Nico, P. S., Pett-Ridge, J., Weber, P. K., and Kleber, M. (2015). Mineral protection of soil carbon counteracted by root exudates. Nat. Climate Change 5, 588–595. doi: 10.1038/nclimate2580
Kelleher, B. P., and Simpson, A. J. (2006). Humic substances in soils: are they really chemically distinct? Environ. Sci. Technol. 40, 4605–4611. doi: 10.1021/es0608085
Kinyangi, J., Solomon, D., Liang, B., Lerotic, M., Wirick, S., and Lehmann, J. (2006). Nanoscale biogeocomplexity of the organomineral assemblage in soil. Soil Sci. Soc. Am. J. 70, 1708–1718. doi: 10.2136/sssaj2005.0351
Kirkby, C. A., Richardson, A. E., Wade, L. J., Batten, G. D., Blanchard, C., and Kirkegaard, J. A. (2013). Carbon-nutrient stoichiometry to increase soil carbon sequestration. Soil Biol. Biochem. 60, 77–86. doi: 10.1016/j.soilbio.2013.01.011
Kleber, M., and Lehmann, J. (2019). Humic substances extracted by alkali are invalid proxies for the dynamics and functions of organic matter in terrestrial and aquatic ecosystems. J. Environ. Qual. 48, 207–216. doi: 10.2134/jeq2019.01.0036
Kleber, M., Nico, P. S., Plante, A., Filley, T., Kramer, M., Swanston, C., et al. (2011). Old and stable soil organic matter is not necessarily chemically recalcitrant: implications for modeling concepts and temperature sensitivity. Glob. Change Biol. 17, 1097–1107. doi: 10.1111/j.1365-2486.2010.02278.x
Klotzbücher, T., Kaiser, K., Guggenberger, G., Gatzek, C., and Kalbitz, K. (2011). A new conceptual model for the fate of lignin in decomposing plant litter. Ecology 92, 1052–1062. doi: 10.1890/10-1307.1
Knorr, W., Prentice, I. C., House, J. I., and Holland, E. A. (2005). Long-term sensitivity of soil carbon turnover to warming. Nature 433, 298–301. doi: 10.1038/nature03226
Kögel-Knabner, I., Guggenberger, G., Kleber, M., Kandeler, E., Kalbitz, K., Scheu, S., et al. (2008). Organo-mineral associations in temperate soils: integrating biology, mineralogy, and organic matter chemistry. J. Plant Nutr. Soil Sci. 171, 61–82. doi: 10.1002/jpln.200700048
Kopittke, P. M., Dalal, R. C., Hoeschen, C., Li, C., Menzies, N. W., and Mueller, C. W. (2020). Soil organic matter is stabilized by organo-mineral associations through two key processes: the role of the carbon to nitrogen ratio. Geoderma 357:113974. doi: 10.1016/j.geoderma.2019.113974
Kragt, M. E., Dumbrell, N. P., and Blackmore, L. (2017). Motivations and barriers for Western Australian broad-acre farmers to adopt carbon farming. Environ. Sci. Pol. 73, 115–123. doi: 10.1016/j.envsci.2017.04.009
Kravchenko, A. N., Guber, A. K., Razavi, B. S., Koestel, J., Quigley, M. Y., Robertson, G. P., et al. (2019). Microbial spatial footprint as a driver of soil carbon stabilization. Nat. Commun. 10:3121. doi: 10.1038/s41467-019-11057-4
Kravchenko, A. N., Negassa, W. C., Guber, A. K., Hildebrandt, B., Marsh, T. L., and Rivers, M. L. (2014). Intra-aggregate pore structure influences phylogenetic composition of bacterial community in macroaggregates. Soil Sci. Soc. Am. J. 78, 1924–1939. doi: 10.2136/sssaj2014.07.0308
Krull, E. S., and Skjemstad, J. O. (2003). δ13C and δ15N profiles in 14C-dated Oxisol and Vertisols as a function of soil chemistry and mineralogy. Geoderma 112, 1–29. doi: 10.1016/S0016-7061(02)00291-4
Lal, R. (2004a). Soil carbon sequestration impacts on global change and food security. Science 304, 1623–1628. doi: 10.1126/science.1097396
Lal, R. (2004b). Soil carbon sequestration to mitigate climate change. Geoderma 123, 1–22. doi: 10.1097/ss.0b013e31815cc498
Lal, R. (2008). Carbon sequestration in soil. Perspect. Agricult. Vet. Sci. Nutr. Nat. Resour. 3, doi: 10.1079/PAVSNNR20083030
Lal, R. (2016). Soil health and carbon management. Food Energy Security 5, 212–222. doi: 10.1002/fes3.96
Lavallee, J. M., Soong, J. L., and Cotrufo, M. F. (2019). Conceptualizing soil organic matter into particulate and mineral-associated forms to address global change in the 21st century. Glob. Change Biol. 26, 261-273. doi: 10.1111/gcb.14859
Lavelle, P., Decaëns, T., Aubert, M., Barot, S., Blouin, M., Bureau, F., et al. (2006). Soil invertebrates and ecosystem services. Eur. J. Soil Biol. 42, S3–S15. doi: 10.1016/j.ejsobi.2006.10.002
Lehmann, J., Kinyangi, J., and Solomon, D. (2007). Organic matter stabilization in soil microaggregates: implications from spatial heterogeneity of organic carbon contents and carbon forms. Biogeochemistry 85, 45–57. doi: 10.1007/s10533-007-9105-3
Lehmann, J., and Kleber, M. (2015). The contentious nature of soil organic matter. Nature 528, 60–68. doi: 10.1038/nature16069
Lehmann, J., Solomon, D., Kinyangi, J., Dathe, L., Wirick, S., and Jacobsen, C. (2008). Spatial complexity of soil organic matter forms at nanometre scales. Nat. Geosci. 1, 238–242. doi: 10.1038/ngeo155
Liang, C., and Balser, T. C. (2011). Microbial production of recalcitrant organic matter in global soils: implications for productivity and climate policy. Nat. Rev. Microbiol. 9:75. doi: 10.1038/nrmicro2386-c1
Liang, C., Cheng, G., Wixon, D. L., and Balser, T. C. (2011). An Absorbing Markov Chain approach to understanding the microbial role in soil carbon stabilization. Biogeochemistry 106, 303–309. doi: 10.1007/s10533-010-9525-3
Liu, S., Zhang, Y., Zong, Y., Hu, Z., Wu, S., Zhou, J., et al. (2016). Response of soil carbon dioxide fluxes, soil organic carbon and microbial biomass carbon to biochar amendment: a meta-analysis. GCB Bioenergy 8, 392–406. doi: 10.1111/gcbb.12265
Lorenz, K., and Lal, R. (2005). The depth distribution of soil organic carbon in relation to land use and management and the potential of carbon sequestration in subsoil horizons. Adv.Agron. 88, 35–66. doi: 10.1016/S0065-2113(05)88002-2
Luo, Z., Wang, E., and Sun, O. J. (2010). Can no-tillage stimulate carbon sequestration in agricultural soils? A meta-analysis of paired experiments. Agricult. Ecosyst. Environ.t 139, 224–231. doi: 10.1016/j.agee.2010.08.006
Major, J., Lehmann, J., Rondon, M., and Goodale, C. (2010). Fate of soil-applied black carbon?: downward migration, leaching and soil respiration. Glob. Change Biol. 16, 1366–1379. doi: 10.1111/j.1365-2486.2009.02044.x
Manzoni, S., Taylor, P., Richter, A., Porporato, A., and Ågren, G. I. (2012). Environmental and stoichiometric controls on microbial carbon-use efficiency in soils. New Phytol. 196, 79–91. doi: 10.1111/j.1469-8137.2012.04225.x
Marschner, B., Brodowski, S., Dreves, A., Gleixner, G., Gude, A., Grootes, P. M., et al. (2008). How relevant is recalcitrance for the stabilization of organic matter in soils? J. Plant Nutr. Soil Sci. 171, 91–110. doi: 10.1002/jpln.200700049
Martínez, I., Chervet, A., Weisskopf, P., Sturny, W. G., Rek, J., and Keller, T. (2016). Two decades of no-till in the Oberacker long-term field experiment: part II. Soil porosity and gas transport parameters. Soil Tillage Res. 163, 130–140. doi: 10.1016/j.still.2016.05.020
McMahon, S. K., Williams, M. A., Bottomley, P. J., and Myrold, D. D. (2005). Dynamics of microbial communities during decomposition of carbon-13 Labeled Ryegrass Fractions in Soil. Soil Sci. Soc. Am. J. 69, 1238–1247. doi: 10.2136/sssaj2004.0289
Melillo, J. M., Aber, J. D., Muratore, J. F., and Jun, N. (1983). Nitrogen and lignin control of hardwood leaf litter decomposition dynamics. Ecology 63, 621–626. doi: 10.2307/1936780
Miltner, A., Bombach, P., Schmidt-Brücken, B., and Kästner, M. (2012). SOM genesis: microbial biomass as a significant source. Biogeochemistry 111, 41–55. doi: 10.1007/s10533-011-9658-z
Minasny, B., Malone, B. P., McBratney, A. B., Angers, D. A., Arrouays, D., Chambers, A., et al. (2017). Soil carbon 4 per mille. Geoderma 292, 59–86. doi: 10.1016/j.geoderma.2017.01.002
Moni, C., Rumpel, C., Virto, I., Chabbi, A., and Chenu, C. (2010). Relative importance of sorption versus aggregation for organic matter storage in subsoil horizons of two contrasting soils. Eur. J. Soil Sci. 61, 958–969. doi: 10.1111/j.1365-2389.2010.01307.x
Morrow, J. G., Huggins, D. R., Carpenter-Boggs, L. A., and Reganold, J. P. (2016). Evaluating measures to assess soil health in long-term agroecosystem trials. Soil Sci. Soc. Am. J. 80, 450–462. doi: 10.2136/sssaj2015.08.0308
Murphy, C. J., Baggs, E. M., Morley, N., Wall, D. P., and Paterson, E. (2015). Rhizosphere priming can promote mobilisation of N-rich compounds from soil organic matter. Soil Biol. Biochem.y 81, 236–243. doi: 10.1016/j.soilbio.2014.11.027
Murray, B. C., Sohngen, B., and Ross, M. T. (2007). Economic consequences of consideration of permanence, leakage and additionality for soil carbon sequestration projects. Clim. Change 80, 127–143. doi: 10.1007/s10584-006-9169-4
Nguyen, B. T., and Lehmann, J. (2009). Black carbon decomposition under varying water regimes. Org. Geochem. 40, 846–853. doi: 10.1016/j.orggeochem.2009.05.004
Nunan, N. (2017). The microbial habitat in soil: scale, heterogeneity and functional consequences. J. Plant Nutr. Soil Sci. 180, 425–429. doi: 10.1002/jpln.201700184
Nunan, N., Leloup, J., Ruamps, L. S., Pouteau, V., and Chenu, C. (2017). Effects of habitat constraints on soil microbial community function. Sci. Rep. 7, 1–10. doi: 10.1038/s41598-017-04485-z
Nunan, N., Wu, K., Young, I. M., Crawford, J. W., and Ritz, K. (2003). Spatial distribution of bacterial communities and their relationships with the micro-architecture of soil. FEMS Microbiol. Ecol. 44, 203–215. doi: 10.1016/S0168-6496(03)00027-8
Nunan, N., Young, I. M., Crawford, J. W., and Ritz, K. (2007). “Bacterial Interactions At The Microscale – Linking Habitat To Function In Soil,” in The Spatial Distribution of Microbes in the Environment, eds R. B. Franklin and A. L. Mills (Dordrecht: Springer), 61–85. doi: 10.1007/978-1-4020-6216-2_3
Oldfield, E. E., Bradford, M. A., and Wood, S. A. (2018). Global meta-analysis of the relationship between soil organic matter and crop yields. Soil 5, 15–32. doi: 10.5194/soil-2018-21
Olk, D. C., Bloom, P. R., Perdue, E. M., McKnight, D. M., Chen, Y., Farenhorst, A., et al. (2019). Environmental and agricultural relevance of humic fractions extracted by alkali from soils and natural waters. J. Environ. Qual. 48, 217–232. doi: 10.2134/jeq2019.02.0041
Oost, K. V., Govers, G., Gryze, S. D., Six, J., Harden, J. W., Ritchie, J. C., et al. (2007). The Impact of Agricultural Soil Erosion on the Global Carbon Cycle. Science 18147, 626–630.
Parton, W. J. (1996). “The CENTURY model,” in Evaluation of Soil Organic Matter Models NATO ASI Series, eds D. S. Powlson, P. Smith, and J. U. Smith (Berlin: Springer), 283–291. doi: 10.1007/978-3-642-61094-3_23
Paustian, K., Andrén, O., Janzen, H. H., Lal, R., Smith, P., Tian, G., et al. (1997). Agricultural soils as a sink to mitigate CO2 emissions. Soil Use Manag. 13, 230–244. doi: 10.1017/CBO9781107415324.004
Paustian, K., Lehmann, J., Ogle, S., Reay, D., Robertson, G. P., and Smith, P. (2016). Climate-smart soils. Nature 532, 49–57. doi: 10.1038/nature17174
Pett-Ridge, J., and Firestone, M. K. (2017). Using stable isotopes to explore root-microbe-mineral interactions in soil. Rhizosphere 3, 244–253. doi: 10.1016/j.rhisph.2017.04.016
Pett-Ridge, J., Nuccio, E., and Mcfarlane, K. (2018). “Deeply Rooted?: Evaluating Plant Rooting Depth as a Means for Enhanced Soil Carbon Sequestration,” in International Conference on Negative CO2 Emissions Gothenburg, Sweden, 1–13.
Plaza, C., Courtier-Murias, D., Fernández, J. M., Polo, A., and Simpson, A. J. (2013). Physical, chemical, and biochemical mechanisms of soil organic matter stabilization under conservation tillage systems: a central role for microbes and microbial by-products in C sequestration. Soil Biol. Biochem. 57, 124–134. doi: 10.1016/j.soilbio.2012.07.026
Poeplau, C., and Don, A. (2015). Carbon sequestration in agricultural soils via cultivation of cover crops - A meta-analysis. Agricult. Ecosyst. Environ. 200, 33–41. doi: 10.1016/j.agee.2014.10.024
Poulton, P., Johnston, J., Macdonald, A., White, R., and Powlson, D. (2018). Major limitations to achieving “4 per 1000” increases in soil organic carbon stock in temperate regions: evidence from long-term experiments at rothamsted research. U.K. Glob. Change Biol. 24, 2563–2584. doi: 10.1111/gcb.14066
Pourhashem, G., Hung, S. Y., Medlock, K. B., and Masiello, C. A. (2019). Policy support for biochar: review and recommendations. GCB Bioenergy 11, 364–380. doi: 10.1111/gcbb.12582
Rasmussen, C., Heckman, K., Wieder, W. R., Keiluweit, M., Lawrence, C. R., Berhe, A. A., et al. (2018). Beyond clay: towards an improved set of variables for predicting soil organic matter content. Biogeochemistry 137, 297–306. doi: 10.1007/s10533-018-0424-3
Rasmussen, C., Torn, M. S., and Southard, R. J. (2005). Mineral assemblage and aggregates control carbon dynamics in a california conifer forest. Soil Sci. Soc. Am. J. 69, 1711–1721. doi: 10.2136/sssaj2005.0040
Rethemeyer, J., Kramer, C., Gleixner, G., John, B., Yamashita, T., Flessa, H., et al. (2005). Transformation of organic matter in agricultural soils: radiocarbon concentration versus soil depth. Geoderma 128, 94–105. doi: 10.1016/j.geoderma.2004.12.017
Riley, W. J., Maggi, F., Kleber, M., Torn, M. S., Tang, J. Y., Dwivedi, D., et al. (2014). Long residence times of rapidly decomposable soil organic matter: application of a multi-phase, multi-component, and vertically resolved model (BAMS1) to soil carbon dynamics. Geosci. Model Dev. 7, 1335–1355. doi: 10.5194/gmd-7-1335-2014
Robertson, A. D., Paustian, K., Ogle, S., Wallenstein, M. D., Lugato, E., and Cotrufo, M. F. (2018). Unifying soil organic matter formation and persistence frameworks: the MEMS model. Biogeosci. Discuss. 16, 1225–1248. doi: 10.5194/bg-2018-430
Roth, V.-N., Lange, M., Simon, C., Hertkorn, N., Bucher, S., Goodall, T., et al. (2019). Persistence of dissolved organic matter explained by molecular changes during its passage through soil. Nat. Geosci. 12, 755–761. doi: 10.1038/s41561-019-0417-4
Rovira, P., and Ramón Vallejo, V. (2002). Mineralization of carbon and nitrogen from plant debris, as affected by debris size and depth of burial. Soil Biol. Biochem. 34, 327–339. doi: 10.1016/S0038-0717(01)00186-9
Rumpel, C., and Kögel-Knabner, I. (2011). Deep soil organic matter—a key but poorly understood component of terrestrial C cycle. Plant and Soil 338, 143–158. doi: 10.1007/s11104-010-0391-5
Salomé, C., Nunan, N., Pouteau, V., Lerch, T. Z., and Chenu, C. (2010). Carbon dynamics in topsoil and in subsoil may be controlled by different regulatory mechanisms. Glob. Change Biol. 16, 416–426. doi: 10.1111/j.1365-2486.2009.01884.x
Sanderman, J., Hengl, T., and Fiske, G. J. (2018). Soil carbon debt of 12,000 years of human land use. Proc. Natl. Acad. Sci. U.S.A. 115, E1700–E1700. doi: 10.1073/pnas.1800925115
Sanderman, J., Maddern, T., and Baldock, J. (2014). Similar composition but differential stability of mineral retained organic matter across four classes of clay minerals. Biogeochemistry 121, 409–424. doi: 10.1007/s10533-014-0009-8
Scharlemann, J. P. W., Tanner, E. V. J., Hiederer, R., and Kapos, V. (2014). Global soil carbon: understanding and managing the largest terrestrial carbon pool. Carbon Manag. 5, 81–91. doi: 10.4155/cmt.13.77
Scharpenseel, H.-W., and Becker-Heidmann, P. (1989). Shifts in 14C Patterns of soil profiles due to bomb carbon. including effects of morphogenetic and turbation processes. Radiocarbon 31, 627–636. doi: 10.1017/S0033822200012224
Schimel, D. S., Braswell, B. H., Holland, E. A., McKeown, R., Ojima, D. S., Painter, T. H., et al. (1994). Climatic, edaphic, and biotic controls over storage and turnover of carbon in soils. Glob. Biogeochem. Cycles 8, 279–293. doi: 10.1029/94GB00993
Schlesinger, W. H. (2000). Carbon sequestration in soils: some cautions amidst optimism. Agricult. Ecosyst. Environ. 82, 121–127. doi: 10.1016/S0167-8809(00)00221-8
Schlesinger, W. H., and Amundson, R. (2018). Managing for soil carbon sequestration: let’s get realistic. Glob. Change Biol. 25, 386-389. doi: 10.1111/gcb.14478
Schlesinger, W. H., and Bernhardt, E. S. (2013). “Chapter 5 - The Biosphere: The Carbon Cycle of Terrestrial Ecosystems,” in Biogeochemistry, 3rd Edn, eds W. H. Schlesinger and E. S. Bernhardt (Boston, MA: Academic Press), 135–172. doi: 10.1016/B978-0-12-385874-0.00005-4
Schmidt, M. W. I., Torn, M. S., Abiven, S., Dittmar, T., Guggenberger, G., Janssens, I. A., et al. (2011). Persistence of soil organic matter as an ecosystem property. Nature 478, 49–56. doi: 10.1038/nature10386
Schmidt, R., Gravuer, K., Bossange, A. V., Mitchell, J., and Scow, K. (2018). Long-term use of cover crops and no-till shift soil microbial community life strategies in agricultural soil. PLoS One 13:e0192953. doi: 10.1371/journal.pone.0192953
Séger, M., Cousin, I., Frison, A., Boizard, H., and Richard, G. (2009). Characterisation of the structural heterogeneity of the soil tilled layer by using in situ 2D and 3D electrical resistivity measurements. Soil Tillage Res. 103, 387–398. doi: 10.1016/j.still.2008.12.003
Sierra, C. A., Müller, M., Metzler, H., Manzoni, S., and Trumbore, S. E. (2017). The muddle of ages, turnover, transit, and residence times in the carbon cycle. Glob. Change Biol. 23, 1763–1773. doi: 10.1111/gcb.13556
Simpson, A. J., Simpson, M. J., Smith, E., and Kelleher, B. P. (2008). Microbially derived inputs to soil organic matter: are current estimates too low? Environ. Sci. Technol. 42, 3115–3115. doi: 10.1021/es8000932
Singh, J. S., and Gupta, V. K. (2018). Soil microbial biomass: a key soil driver in management of ecosystem functioning. Sci. Total Environ. 634, 497–500. doi: 10.1016/j.scitotenv.2018.03.373
Sinsabaugh, R. L., Manzoni, S., Moorhead, D. L., and Richter, A. (2013). Carbon use efficiency of microbial communities: stoichiometry, methodology and modeling. Ecol. Lett. 16, 930–939. doi: 10.1111/ele.12113
Six, J., Callewaert, P., Lenders, S., De Gryze, S., Morris, S. J., Gregorich, E. G., et al. (2002a). Measuring and understanding carbon storage in afforested soils by physical fractionation. Soil Sci. Soc. Am. J. 66, 1981–1987. doi: 10.2136/sssaj2002.1981
Six, J., Conant, R. T., Paul, E. A., and Paustian, K. (2002b). Stabilization mechanisms of soil organic matter: implications for C-saturation of soils. Plant Soil 241, 155–176. doi: 10.1023/A:1016125726789
Six, J., Elliott, E. T., and Paustian, K. (2000). Soil macroaggregate turnover and microaggregate formation: a mechanism for C sequestration under no-tillage agriculture. Soil Biol. Biochem. 32, 2099–2103. doi: 10.1016/S0038-0717(00)00179-6
Six, J., Guggenberger, G., Paustian, K., Haumaier, L., Elliott, E. T., and Zech, W. (2001). Sources and composition of soil organic matter fractions between and within soil aggregates. Eur. J. Soil Sci. 52, 607–618. doi: 10.1046/j.1365-2389.2001.00406.x
Six, J., and Paustian, K. (2014). Aggregate-associated soil organic matter as an ecosystem property and a measurement tool. Soil Biol. Biochem. 68, A4–A9. doi: 10.1016/j.soilbio.2013.06.014
Smith, P. (2005). An overview of the permanence of soil organic carbon stocks: influence of direct human-induced, indirect and natural effects. Eur. J. Soil Sci. 56, 673–680. doi: 10.1111/j.1365-2389.2005.00708.x
Smith, P. (2016). Soil carbon sequestration and biochar as negative emission technologies. Glob. Change Biol. 22, 1315–1324. doi: 10.1111/gcb.13178
Smith, P., Soussana, J.-F., Angers, D., Schipper, L., Chenu, C., Rasse, D. P., et al. (2019). How to measure, report and verify soil carbon change to realize the potential of soil carbon sequestration for atmospheric greenhouse gas removal. Glob. Change Biol. 26, 219-241. doi: 10.1111/gcb.14815
Smucker, A. J. M., Park, E.-J., Dorner, J., and Horn, R. (2007). Soil micropore development and contributions to soluble carbon transport within Macroaggregates. Vadose Zone J. 6, 282–290. doi: 10.2136/vzj2007.0031
Sokol, N. W., and Bradford, M. A. (2018). Microbial formation of stable soil carbon is more efficient from belowground than aboveground input. Nature Geosci. 12, 46–53. doi: 10.1038/s41561-018-0258-6
Sokol, N. W., Kuebbing, S. E., Karlsen-Ayala, E., and Bradford, M. A. (2019). Evidence for the primacy of living root inputs, not root or shoot litter, in forming soil organic carbon. New Phytol. 221, 233–246. doi: 10.1111/nph.15361
Sokol, N. W., Sanderman, J., and Bradford, M. A. (2018). Pathways of mineral-associated soil organic matter formation: integrating the role of plant carbon source, chemistry, and point of entry. Glob. Change Biol. 25, 12–24. doi: 10.1111/gcb.14482
Sollins, P., Homann, P., and Caldwell, B. A. (1996). Stabilization and destabilization of soil organic matter: mechanisms and controls. Geoderma 74, 65–105. doi: 10.1016/S0016-7061(96)00036-5
Sollins, P., Kramer, M. G., Swanston, C., Lajtha, K., Filley, T., Aufdenkampe, A. K., et al. (2009). Sequential density fractionation across soils of contrasting mineralogy: evidence for both microbial- and mineral-controlled soil organic matter stabilization. Biogeochemistry 96, 209–231. doi: 10.1007/s10533-009-9359-z
Sommer, R., and Bossio, D. (2014). Dynamics and climate change mitigation potential of soil organic carbon sequestration. J. Environ. Manage. 144, 83–87. doi: 10.1016/j.jenvman.2014.05.017
Steffens, M., Rogge, D. M., Mueller, C. W., Höschen, C., Lugmeier, J., Kölbl, A., et al. (2017). Identification of distinct functional microstructural domains controlling C storage in Soil. Environ. Sci. Technol. 51, 12182–12189. doi: 10.1021/acs.est.7b03715
Stewart, C. E., Paustian, K., Conant, R. T., Plante, A. F., and Six, J. (2007). Soil carbon saturation: concept, evidence and evaluation. Biogeochemistry 86, 19–31. doi: 10.1007/s10533-007-9140-0
Tan, K. H. (2014). Humic Matter in Soil and the Environment: Principles and Controversies, 2nd Edn. Boca Raton, FL: CRC Press.
Tarquis, A. M., Heck, R. J., Andina, D., Alvarez, A., and Antón, J. M. (2009). Pore network complexity and thresholding of 3D soil images. Ecol. Comp. 6, 230–239. doi: 10.1016/j.ecocom.2009.05.010
Tautges, N. E., Chiartas, J., Gaudin, A. C. M., O’Geen, A. T., Herrera, I., and Scow, M. K. (2019). Deep soil inventories reveal that impacts of cover crops and compost on soil carbon sequestration differ in surface and sub-surface soils. Glob. Change Biol. 25, 3753-3766. doi: 10.1111/gcb.14762
Thamo, T., and Pannell, D. J. (2016). Challenges in developing effective policy for soil carbon sequestration: perspectives on additionality, leakage, and permanence. Clim. Pol. 16, 973–992. doi: 10.1080/14693062.2015.1075372
Turmel, M.-S., Speratti, A., Baudron, F., Verhulst, N., and Govaerts, B. (2015). Crop residue management and soil health: a systems analysis. Agricult. Syst. 134, 6–16. doi: 10.1016/j.agsy.2014.05.009
Van Groenigen, J. W., Van Kessel, C., Hungate, B. A., Oenema, O., Powlson, D. S., and Van Groenigen, K. J. (2017). Sequestering soil organic carbon: a nitrogen dilemma. Environ. Sci. Technol. 51, 4738–4739. doi: 10.1021/acs.est.7b01427
van Groenigen, K.-J., Six, J., Hungate, B. A., de Graaff, M.-A., van Breemen, N., and van Kessel, C. (2006). Element interactions limit soil carbon storage. Proc. Natl. Acad. Sci. U.S.A. 103, 6571–6574. doi: 10.1073/pnas.0509038103
Vermeulen, S., Bossio, D., Lehmann, J., Luu, P., Paustian, K., Webb, C., et al. (2019). A global agenda for collective action on soil carbon. Nat. Sustain. 2, 2–4. doi: 10.1038/s41893-018-0212-z
Vogel, C., Mueller, C. W., Höschen, C., Buegger, F., Heister, K., Schulz, S., et al. (2014). Submicron structures provide preferential spots for carbon and nitrogen sequestration in soils. Nat. Commun. 5, 1–7. doi: 10.1038/ncomms3947
von Lutzow, M., Kogel-Knabner, I., Ekschmitt, K., Matzner, E., Guggenberger, G., Marschner, B., et al. (2006). Stabilization of organic matter in temperate soils: mechanisms and their relevance under different soil conditions - a review. Eur. J. Soil Sci. 57, 426–445. doi: 10.1111/j.1365-2389.2006.00809.x
von Unger, M., and Emmer, I. (2018). Carbon Market Incentives to Conserve, Restore and Enhance Soil Carbon. Arlington, VA: Silvestrum and The Nature Conservancy.
Wade, J., Maltais-Landry, G., Lucas, D. E., Bongiorno, G., Bowles, T. M., Calderón, F. J., et al. (2020). Assessing the sensitivity and repeatability of permanganate oxidizable carbon as a soil health metric: an interlab comparison across soils. Geoderma 366:114235. doi: 10.1016/j.geoderma.2020.114235
Wagai, R., and Mayer, L. M. (2007). Sorptive stabilization of organic matter in soils by hydrous iron oxides. Geochim. Cosmochim. Acta 71, 25–35. doi: 10.1016/j.gca.2006.08.047
Wallenstein, M. D., and Hall, E. K. (2012). A trait-based framework for predicting when and where microbial adaptation to climate change will affect ecosystem functioning. Biogeochemistry 109, 35–47. doi: 10.1007/s10533-011-9641-8
Wang, J., Xiong, Z., and Kuzyakov, Y. (2016). Biochar stability in soil: meta-analysis of decomposition and priming effects. GCB Bioenergy 8, 512–523. doi: 10.1111/gcbb.12266
White, K. E., Brennan, E. B., Cavigelli, M. A., and Smith, R. F. (2020). Winter cover crops increase readily decomposable soil carbon, but compost drives total soil carbon during eight years of intensive, organic vegetable production in California. PLoS One 15:e0228677. doi: 10.1371/journal.pone.0228677
White, R. E., Davidson, B., Lam, S. K., and Chen, D. (2018). A critique of the paper ‘Soil carbon 4 per mille’ by Minasny et al. (2017). Geoderma 309, 115–117. doi: 10.1016/j.geoderma.2017.05.025
Whitman, T., and Lehmann, J. (2009). Biochar—One way forward for soil carbon in offset mechanisms in Africa? Environ. Sci. Pol. 12, 1024–1027. doi: 10.1016/j.envsci.2009.07.013
Wieder, W. R., Cleveland, C. C., Smith, W. K., and Todd-Brown, K. (2015). Future productivity and carbon storage limited by terrestrial nutrient availability. Nat. Geosci. 8, 441–444. doi: 10.1038/ngeo2413
Wieder, W. R., Grandy, A. S., Kallenbach, C. M., and Bonan, G. B. (2014). Integrating microbial physiology and physio-chemical principles in soils with the MIcrobial-MIneral Carbon Stabilization (MIMICS) model. Biogeosciences 11, 3899–3917. doi: 10.5194/bg-11-3899-2014
Wiesmeier, M., Urbanski, L., Hobley, E., Lang, B., Lützow, M. V., Marin-spiotta, E., et al. (2019). Soil organic carbon storage as a key function of soils - A review of drivers and indicators at various scales. Geoderma 333, 149–162. doi: 10.1016/j.geoderma.2018.07.026
Woolf, D., and Lehmann, J. (2019). Microbial models with minimal mineral protection can explain long-term soil organic carbon persistence. Sci. Rep. 9, 6522–6522. doi: 10.1038/s41598-019-43026-8
Woolf, D., Lehmann, J., Cowie, A., Cayuela, M. L., Whitman, T., Sohi, S., et al. (2018). “Biochar for climate change mitigation: navigating from science to evidence-based policy,” in Soil and Climate (Boca Raton, Florida: CRC Press), 219–248.
Young, I. M., and Bengough, A. (2018). The search for the meaning of life in soil: an opinion. Eur. J. Soil Sci. 69, 31–38. doi: 10.1111/ejss.12514
Young, I. M., and Crawford, J. W. (2004). Interactions and self-organization in the soil-microbe complex. Science 304, 1634–1638. doi: 10.1126/science.1097394
Young, I. M., Crawford, J. W., and Rappoldt, C. (2001). New methods and models for characterising structural heterogeneity of soil. Soil Tillage Res. 61, 33–45. doi: 10.1016/S0167-1987(01)00188-X
Keywords: climate change, sustainable agriculture, soil carbon, carbon sequestration, microbial biomass
Citation: Dynarski KA, Bossio DA and Scow KM (2020) Dynamic Stability of Soil Carbon: Reassessing the “Permanence” of Soil Carbon Sequestration. Front. Environ. Sci. 8:514701. doi: 10.3389/fenvs.2020.514701
Received: 25 November 2019; Accepted: 19 October 2020;
Published: 13 November 2020.
Edited by:
Philippe C. Baveye, AgroParisTech Institut des sciences et industries du vivant et de l’environnement, FranceReviewed by:
Markus Steffens, Research Institute of Organic Agriculture (FiBL), SwitzerlandSamuel Abiven, University of Zurich, Switzerland
Copyright © 2020 Dynarski, Bossio and Scow. This is an open-access article distributed under the terms of the Creative Commons Attribution License (CC BY). The use, distribution or reproduction in other forums is permitted, provided the original author(s) and the copyright owner(s) are credited and that the original publication in this journal is cited, in accordance with accepted academic practice. No use, distribution or reproduction is permitted which does not comply with these terms.
*Correspondence: Katherine A. Dynarski, a2F0aGVyaW5lLmR5bmFyc2tpQHVtb250YW5hLmVkdQ==
†Present address: Katherine A. Dynarski, Department of Ecosystem and Conservation Sciences, University of Montana, Missoula, MT, United States