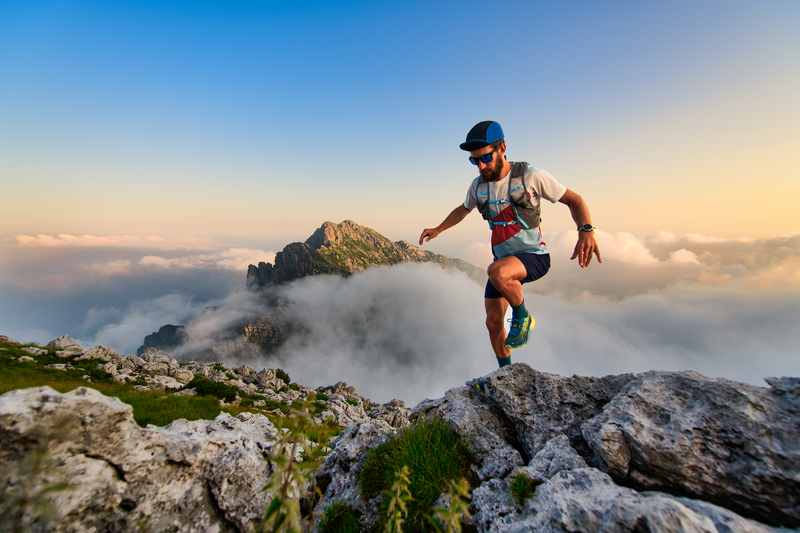
95% of researchers rate our articles as excellent or good
Learn more about the work of our research integrity team to safeguard the quality of each article we publish.
Find out more
ORIGINAL RESEARCH article
Front. Environ. Sci. , 11 August 2020
Sec. Water and Wastewater Management
Volume 8 - 2020 | https://doi.org/10.3389/fenvs.2020.00126
This article is part of the Research Topic Submarine Groundwater Discharge: Impacts on Coastal Ecosystem by Hidden Water and Dissolved Materials View all 11 articles
Stable isotopes of oxygen, nitrogen, and boron were used to identify the sources of nitrate (NO3–) in submarine groundwater discharge (SGD) into a large tidal estuary (Long Island Sound, NY, United States). Potential contaminants such as manure, septic waste and fertilizer overlap in δ15N and δ18O but have been shown to have distinctive δ11B in non-coastal settings. Two distinct subterranean estuaries were studied with different land-use up gradient, representative of (1) mixed medium-density residential housing and (2) agriculture. These sites have overlapping δ15N and δ18O measurements in NO3– and are unable to discriminate between different N sources. Boron isotopes and concentrations are measurably different between the two sites, with little overlap. The subterranean estuary impacted by mixed medium-density residential housing shows little correlation between δ11B and [B] or between δ11B and salinity, demonstrating that direct mixing relationships between fresh groundwater and seawater were unlikely to account for the variability. No two sources could adequately characterize the δ11B of this subterranean estuary. Groundwater N at this location should be derived from individual homeowner cesspools, although measured septic waste has much lower δ11B compared to the coastal groundwaters. This observation, with no trend in δ11B with [B] indicates multiple sources supply B to the coastal groundwaters. The agricultural subterranean estuary displayed a positive correlation between δ11B and [B] without any relationship with salinity. Binary mixing between sea spray and fertilizer can reasonably explain the distribution of B in the agricultural subterranean estuary. Results from this study demonstrate that δ11B can be used in combination with δ15N to trace sources of NO3– to the subterranean estuary if source endmember isotopic signatures are well-constrained, and if the influence of seawater on δ11B signatures can be minimized or easily quantified.
Submarine groundwater discharge (SGD) is an important vector for the delivery of nutrients to the coastal ocean (e.g., Taniguchi et al., 2019). While once thought to be a relatively minor component of the overall coastal nitrogen budget, studies have shown that non-point-sources of diffuse SGD can supply as much nitrogen into coastal waters as rivers (Slomp and Van Cappellen, 2004; Kroeger and Charette, 2008; Rodellas et al., 2015). The extent of SGD’s effect on coastal water quality has been difficult to establish because SGD consists of a fresh groundwater component and a seawater component that mix in a biogeochemically reactive subterranean estuary (STE; Moore, 1999; Burnett et al., 2003; Slomp and Van Cappellen, 2004). The terrestrial, fresh component of SGD (FSGD) represents a source of new N to coastal waters and, if significant, can help sustain primary production and even coastal algal blooms (Gobler and Sanudo-Wilhelm, 2001), thus fueling coastal eutrophication (Paerl, 1995; Howarth and Marino, 2006).
Isotopes of N and O in nitrate (NO3–) have a proven utility in sorting out the sources of nitrogen delivered to groundwaters (Aravena et al., 1993; Bateman and Kelly, 2007; Bannon and Roman, 2008; Kendall et al., 2008). In particular, δ15N-NO3– and δ18O-NO3– can distinguish between NO3– fertilizers, atmospheric NO3–, manure and sewage-derived NO3– (Kendall, 1998; Kendall et al., 2008). However, the overlapping δ15N signatures of soil N, animal and human waste, and nitrification of NH4+ in fertilizer and precipitation, as well as the biological modification of nitrogen and oxygen isotopes, can limit their utility in fully identifying anthropogenic sources of nitrogen. In addition, the speciation and attenuation of nitrogen may be modified in the STE before it reaches the coastal ocean (Kroeger and Charette, 2008; Erler et al., 2014). For example, changes in dissolved oxygen (DO) in groundwater may cause changes in N and O isotopic composition by nitrifying NH4+ to NO3– or denitrifying NO3– to N2. NH4+ volatilization and nitrogen remineralization may also alter N speciation (Charbonnier et al., 2013) and isotopic composition. Therefore, the groundwater isotopic signatures of N and O of an inland groundwater source may differ from that delivered to the coastal ocean, making it difficult to track anthropogenic sources of NO3– through the STE (Anschutz et al., 2016). The addition of other tracers may be useful in resolving these problems, including boron (B) isotopes.
Boron is added to groundwater both naturally and with contaminants such as NO3– in association with borate minerals used in fertilizers and detergents through septic systems or sewage (Barth, 1998; Vengosh, 1998; Vengosh et al., 1999). B is added to groundwater naturally via precipitation and water-rock interactions that include sorption reactions with clay minerals and iron hydroxides (Demetriou and Pashalidis, 2012). Anthropogenic sources of B to groundwaters include fertilizers and septic systems or sewage (Vengosh et al., 1999). Plants require B as an essential micronutrient for growth and therefore borate minerals are commonly added to commercial fertilizers (Shireen et al., 2018). Detergents and household cleaning products contain elevated [B] that is subsequently transported to septic systems.
Boron is highly soluble in groundwater and is therefore a co-migrant of groundwater NO3–, including fertilizer and septic sources. B is not removed by wastewater treatment nor is it affected by processes that alter N concentration, such as nitrification and denitrification; however, B speciation is controlled by pH. At low pH boric acid (BOH3) is the dominant species, while at high pH borate (BOH4–) dominates, with a pKa of 9.2 in fresh water (McPhail et al., 1972). There is an isotope fractionation between these species that makes borate isotopically enriched in 10B. Boric acid is conservative, but borate has a strong affinity for positively charged particle surfaces (e.g., clays) because of its negative charge (Palmer et al., 1987). This can result in changes in the isotope ratio as B can be fractionated by processes such as adsorption and desorption of borate onto iron oxides and clay minerals (McPhail et al., 1972; Goldberg and Glaubig, 1985; Glavee et al., 1995; Lemarchand et al., 2007; Demetriou and Pashalidis, 2012). However, these processes are pH dependent, where adsorption is greatest at pH 9 and decreases to less than 10% adsorption at pH 5 and lower (Bloesch et al., 1987; De La Fuente and Camacho, 2009; Demetriou and Pashalidis, 2012). The large relative mass differences between 11B and 10B means that isotope fractionation leads to a wide range of isotopic values in nature. The combined use of δ11B and δ15N to trace NO3– sources has been successfully applied in terrestrial and aquatic environments (Komor, 1997; Widory et al., 2004, 2005, 2013; Seiler, 2005; Bronders et al., 2012; Lindenbaum, 2012; Briand et al., 2013, 2017; Eppich et al., 2013; Saccon et al., 2013; Ransom et al., 2016; Guinoiseau et al., 2018; Kruk et al., 2020); however, the use of δ11B and δ15N has yet to be tested in the STE of coastal systems.
Long Island Sound (LIS) is a coastal estuary that lies between the south shore of Connecticut and the north shore of Long Island, NY, United States (Figure 1). The southern shoreline of LIS is cut into an unconfined, unconsolidated, sole-source aquifer (the Upper Glacial Aquifer) of glacial deposits with a hydraulic conductivity between 7 and 70 m d–1 (Buxton and Modica, 1992). The hydraulic gradient has been estimated to be 0.001 (McClymonds and Franke, 1972) with a vertical hydraulic gradient between 0.02 and 0.08 in the upper meter of sediment at the shoreline. Under these conditions, significant SGD has been documented along the shores of LIS (Durand, 2014; Garcia-Orellana et al., 2014; Bokuniewicz et al., 2015; Young et al., 2015; Tamborski et al., 2017a, b). FSGD-driven N loads to Smithtown Bay (1–13∗106 mol N y–1), an embayment of LIS, rivals that of the local Nissequogue River (4–10∗106 mol N y–1) and has been implicated as the primary new N source to LIS (Tamborski et al., 2017a).
Figure 1. Long Island, NY, United States (A) and the subterranean estuary (STE) study sites of Callahans Beach (B) and Iron Pier Beach (C), indicated by yellow stars. A survey of water samples from groundwater wells, spring-fed creeks (Mill Ponds) and precipitation-fed ponds (Kettle Ponds) are shown in panel (A).
This study focuses on two distinct STE’s along the north shore of Long Island at Callahans Beach (adjacent to Smithtown Bay) and at Iron Pier Beach (on the North Fork of Long Island; Figure 1). Anthropogenic contaminant sources including cesspools, septic systems and fertilizers are implicated in producing elevated NO3– levels seen in Long Island’s unconfined Upper Glacial Aquifer (Bellone, 2015). Land cover within the watershed of Callahans Beach is composed of medium-density residential housing (2770 people km–2; town of Fort Salonga); the beach itself is directly down-gradient from a golf course (Figure 1). This site has been well studied (Tamborski et al., 2015, 2017a,b) and was chosen to represent a shoreline impacted by mixed anthropogenic N sources (fertilizer, manure and/or septic); there was no evidence of groundwater denitrification or nitrogen attenuation in the STE (Tamborski et al., 2017a). At Callahans Beach, SGD rates vary tidally and can reach 50 cm d–1 near low tide (Tamborski et al., 2015). Land cover within the watershed of Iron Pier Beach is predominantly used for agriculture and this lower population density site (470 people km–2; town of Northville) was chosen to represent a shoreline impacted by a fertilizer N source. At Iron Pier Beach, SGD was measured at rates up to 75 cm d–1 via manual seepage meter measurements (Brown, 2018). Identifying the sources of nitrogen in groundwater is of particular importance to managers here because an effort to reduce nitrogen in Suffolk County, NY, United States, called “Reclaim our Water Initiative,” is predicated on nitrogen pollution from septic systems (Suffolk County Government, 2019).
Multi-level cluster wells were installed above the spring high tide elevation at Callahans Beach in 2014 at depths of 3.0 m, 5.0 m, 6.0 m, and 7.0 m below surface, and at Iron Pier Beach at 1.5 m, 3.0 m, and 4.5 m depths in 2017 using a track-mounted Geoprobe. Multi-level cluster-well installation at Callahans Beach is described in Tamborski et al. (2017b). Groundwater was collected from cluster wells monthly at both sites using a peristaltic pump, from June to December 2017, for nutrient analyses. Temperature, conductivity, salinity, DO, ORP and pH were measured in the field using a YSI-566 multi-probe. Groundwater samples for N, O, and B isotopic analysis were collected from June through October. Water samples for nutrient concentrations and N and O isotopes were filtered in the field (0.2 μm), collected in 15 mL falcon tubes and kept on ice. Samples were frozen upon returning to the lab and kept frozen until analyzed. The water samples for B isotope analysis were filtered in the field (0.2 μm), collected in 50 mL polypropylene centrifuge tubes and were kept refrigerated until analyzed.
A variety of possible endmembers were sampled to further constrain potential B sources. To estimate the contribution of B from seawater, we analyzed coastal seawater samples (n = 10) from Port Jefferson Harbor (Figure 1). Precipitation was collected from acid cleaned rain gauges on four separate occasions (at Stony Brook University). Surface waters were collected from protected precipitation-fed lakes (i.e., kettle ponds; Pine Barrens region; n = 3) and spring-fed ponds (Mill Pond and Setauket Pond; n = 8). Private wells (n = 5) in Smithtown and Old Field were sampled at the houses before the filter (which was disconnected). Septic waste (n = 3) was collected from private residences on Long Island with a peristaltic pump from a pump chamber (immediately downfield from the septic tank prior to the drain-field) and stored in 1 L plastic containers with no processing (e.g., no filtering, pH adjustment). All surface and groundwater samples were collected in acid cleaned 50 mL polypropylene bottles. The bottles were rinsed with the water that was being collected and then collected to avoid air space. Temperature, DO, and salinity were measured at the time of collection. Samples were prepared for B analyses in the same way that SGD samples described above were prepared.
In order to obtain δ11B and to estimate how much [B] could be expected from commercial products, tap water was used to leach five commonly applied fertilizers, one manure sample purchased locally and one locally collected Canada goose dropping. The tap water represents water that would be used for irrigation of lawns, making the leach a more realistic value for what might be delivered to groundwater. The fertilizers and manure were put in 50 mL centrifuge tubes up to the 5 mL mark, filled to the 50 mL mark with tap water and placed on a shaker table at room temperature for several hours.
Boron concentrations were measured with an Agilent 7500cx Quadrupole ICP-MS using a standard bracketing routine with background subtraction. After concentrations were established, approximately 250 ng of B was separated from the matrix using Amberlite IRA 743, a B-specific resin. Samples were adjusted to a pH of ∼9 before adding to the columns, washed with pH 9 adjusted DI water, and eluted with 2% nitric acid following a modified procedure from Lemarchand et al. (2002). Boron isotope ratios were measured using a Nu Instruments Plasma II multi-collector ICP-MS using a standard bracketing routine that included a background between each sample and standard. These background measurements were averaged and subtracted from the sample and standards before using the average of bracketing standards (NBS 951, which is taken as 0‰) to calculate δ11B. Measurement precision is between 0.5 and 1.0‰. Archived groundwater samples from Callahans Beach, collected during July 2014 and May 2015, were additionally analyzed for [B] and δ11B.
Coastal groundwater samples for nitrate concentration analysis were prepared using a modified vanadium (III) reduction procedure (Miranda et al., 2001; Doane and Howarth, 2003). Samples for the analysis of ammonium were prepared and analyzed using a mixed-reagent method (Presley and Claypool, 1971). Nutrient (NO3– and NH4+) concentrations were measured spectrophotometrically on a BPPBABTECH Omega series microplate reader. δ15N-NO3– and δ18O-NO3– were measured at the stable isotope facility at the University of California, Davis by bacterial denitrification assay. Isotope ratios were measured on a ThermoFinnigan GasBench + PreCon trace-gas concentration system interfaced to a ThermoScientific Delta V Plus isotope-ratio mass spectrometer with measurement precision of 0.4‰ for δ15N-NO3– and 0.5‰ for δ18O-NO3–. Nitrogen isotopes are referenced to air while oxygen isotopes are referenced to Vienna Standard Mean Ocean Water (VSMOW). Nitrite was removed prior to analysis (Granger and Sigman, 2009). November and December groundwater samples were not analyzed for δ15N-NO3– and δ18O-NO3–.
The survey of local water sources reveals a range in [B] and large variability in δ11B (Table 1). Rainwater varied from 6 to 13 ppb with a δ 11B between 12.7 and 33.4‰ (Table 1). Kettle ponds in the Pine Barrens region, which is a protected part of the Long Island watershed without farming and residences (Figure 1), demonstrated similar [B] as the rainwater samples (6–8 ppb) and somewhat higher δ11B values (27.5 to 32.7‰; Table 1). These protected ponds are fed by precipitation and should therefore represent the average annual precipitation δ11B and [B] endmember.
Table 1. Summary of B concentration and δ11B isotopic values for natural waters, including precipitation, protected precipitation-fed lakes, spring-fed ponds, groundwater wells, public tap water, and seawater.
Private wells to depths of 30 and 90 m in the Upper Glacial aquifer (town of Old Field; Figure 1) give a range of [B] of 18–47 ppb and a range of δ11B of 19.6 to 36.6‰ (Table 1). In contrast, one well from the town of Smithtown (Metcalf) was higher in [B] (67 ppb) and lower in δ11B (11‰; Table 1). Water collected along two spring-fed creeks (Setauket Mill Pond and Stony Brook Mill Pond) were enriched in [B] (17–25 ppb) and isotopically low (δ11B = 9.0–15.8‰; Setauket Pond 2E; Table 1) as compared to inland groundwaters. A total of 10 coastal seawater samples were collected along the shoreline of Port Jefferson Harbor; the mean (± standard deviation) δ11B is 39.4 ± 0.2‰ for a [B] of 3580 ppb and a salinity of 27 psu.
The public water supply is used for agricultural and homeowner irrigation; therefore, tap water was used to leach a variety of fertilizers and manure samples. The tap water has relatively low [B] (11 ppb) and elevated δ11B (34.9‰; Table 1). Leaching with tap water produced a range of [B] and while it is understood that farming and lawn practices are not mimicked by our leaching experiments and attenuation is expected, the δ11B values are used in ensuing mixing models (see section “Identifying Potential Sources of Groundwater Nitrate Using Boron Stable Isotopes”). The differences in [B] availability through these qualitative leaching experiments provides order of magnitude concentrations and shows differences among the various potential B sources. The δ11B values for five commonly applied commercial fertilizers (Scotts Thick’R Lawn, Holly Tone, 10–10–10, 5–10–5, and MilorganiteTM) analyzed ranged from −4.4 to 12.6‰ (Table 2). In contrast, commercial manure (animal source unknown) was higher in δ11B (25.9‰), and similar to that of Canada goose manure collected from Stony Brook University’s campus (25.8‰; Table 2). The δ11B of three septic samples ranged between −0.2 and 2.5‰ (Table 2). MilorganiteTM is the heat-treated residue from microbes that were used to break up solids in the public wastewater treatment for the city of Milwaukee, likely accounting for its similar composition (−4.4‰) to the septic samples. These analyses were conducted to better understand potential B endmembers, and N isotope values were not measured.
Table 2. Summary of potential contaminant endmember δ11B isotopic values analyzed, including fertilizers, animals waste and septic waste.
Groundwater salinity remained constant at depth (≤0.3 psu) and waters were well-oxygenated (with dissolved oxygen, [DO] > 5 mg L–1) at Callahans Beach (Table 3). DO was variable with depth and was generally lower in August and September than it was in the other 4 months. Groundwater pH generally increased with depth but showed some variability being lower in July (4.77–4.98) than at other times (4.80–5.89). [B] did not show a statistically significant correlation with salinity (R2 = 0.22, excluding a high outlier from 6 m depth in July) or with NO3– concentrations (Table 3 and Figure 2). B concentrations were variable with depth for each month, ranging from 14 to 47 ppb, with one elevated salinity sample at 73 ppb (Table 3). NO3– concentrations in groundwater from Callahans Beach ranged from 3.7 to 19.3 mg L–1 (as NO3–) with a mean (± standard deviation) of 12.7 ± 4.6 mg L–1 (Table 3 and Figure 2B). NO3– concentrations were elevated during June through September and decreased from October through December 2017. NH4+ was present at trace levels through the summer and increased slightly in November and December. These differences may have been driven by changes in regional precipitation. October 2017 had about 150 mm of total rainfall, while November and December 2017 had about 50 mm of total rainfall. Tamborski et al. (2017a) monitored these same wells over a 12-month period (2014–2015), during which NH4+ concentrations were negligible and NO3– was the dominant form of inorganic N.
Figure 2. (A) Coastal groundwater salinity vs. δ11B with point size reflecting B concentration (A); B concentration vs. δ11B with point size reflecting NO3– concentration (B) and NO3/B vs. δ11B with point size reflecting NO3– concentration (C). The range of seawater (∼0.5 mg N L–1) and precipitation (∼1 mg N L–1) in panel (C) are represented by dashed black and blue rectangles, respectively.
At Iron Pier Beach, groundwater salinity was relatively constant with depth (<0.5 psu), with slightly elevated salinities in July (<2 psu; Table 4). DO generally decreased with depth but all samples were well-oxygenated (>5 mg L–1); DO was slightly higher in summer than in winter. There was variability in pH with depth and pH was lower in June (4.98–5.07) than at other times of the year (5.10–5.92). On average, B concentrations at Iron Pier Beach were higher than those at Callahans Beach (16–98 ppb), variable with depth and were not correlated with salinity (Table 4 and Figure 2A). NO3– concentrations were consistently higher at Iron Pier Beach than those at Callahans Beach. The mean (± standard deviation) groundwater NO3– concentration was 28.2 ± 9.3 mg L–1 with a range of 9.3 to 52.6 mg L–1. NO3– concentrations showed no seasonal differences and generally increased with depth, with minor amounts of NH4+ (Table 4).
Groundwater samples from Callahans Beach collected in 2017 gave δ15N-NO3 values that ranged from 1.9‰ to 4.7‰ and δ18O-NO3 values that ranged from −0.4‰ to 2.2‰ (Figure 3A). δ15N-NO3 was slightly lower at 6 m depth (sample ID = CH4) than at other depths during August, September, and October (Table 3). δ18O-NO3 values were generally variable at depth without an obvious trend. The δ11B of groundwater at Callahans Beach ranged from 23.7‰ to 42.5‰ with a concurrent change at 6 m depth (Table 3). δ11B was significantly higher from July to October, but lower during June, and showed no trend with NO3– concentrations (Figure 2B).
Figure 3. (A) δ15N-NO3 vs. δ18O-NO3 of coastal groundwater samples. Callahans 2014/2015 data from Tamborski et al. (2017a). Northport data from Bleifuss et al. (2000). Source fields modified from Kendall et al. (2008). (B) δ15N-NO3 vs. δ11B. The δ15N-NO3 source fields are from Kendall et al. (2008) and the δ11B ranges are based on a survey of possible sources on Long Island as well as the ranges reported by Vengosh et al. (1994, 1999), Bassett et al. (1995); Komor (1997), Seiler (2005), Widory et al. (2005), Tirez et al. (2010), and Eppich et al. (2013). Only select samples from Callahans 2014/2015 are included (salinity ≤ 0.3).
Iron Pier Beach groundwaters had a similar range of δ15N-NO3 values (0.5‰ to 5.0‰) as Callahans Beach (Figure 3A). δ15N-NO3 values at Iron Pier Beach generally increased with depth (Table 4), while δ18O-NO3 values were highest at a depth of 3 m. δ11B values were generally lower at Iron Pier Beach than groundwater samples from Callahans Beach and ranged from −2.1‰ to 35.7‰ (Table 4 and Figure 2) and displayed a significant positive correlation with B concentration (R2 = 0.56; Figure 2B). Groundwater NO3/B ratios were significantly greater than seawater and precipitation endmembers for both sites (Figure 2C), demonstrating a significant input of anthropogenic NO3– to the STE.
Groundwater pH was low at both sites (Tables 3, 4) and was not correlated with [B] or δ11B. Therefore, B isotopic signatures are unlikely to be affected by sorption and should only reflect nitrogen source compositions or mixing processes. Coastal groundwaters at both sites were well oxygenated (Tables 3, 4); despite this, denitrification may occur in sedimentary anoxic micro-sites (Brandes and Devol, 1997). There was no evidence of denitrification in the STE (Figure 3A), assuming that denitrification results in a δ18O to δ15N ratio of 2:1 (Kendall et al., 2008). This is further supported by comparing δ15N with ln[NO3–] and 1/[NO3–] (Figure 4). Denitrification should result in trends distinctive from that induced by binary mixing. The narrow range of δ15N for the coastal groundwaters coupled with overlapping signatures of δ15N in potential endmembers (Figure 3A) precludes using N alone to untangle mixing sources (Figure 4).
Figure 4. Natural logarithm of NO3– vs. δ15N (A) and 1/[NO3–] vs. δ15N (B) of coastal groundwater samples.
There were no seasonal changes in δ15N-NO3– and δ18O-NO3– at Iron Pier Beach, but there were differences with depth (Table 4). The groundwater at 1.5 m depth had a lower δ15 N value relative to the other groundwater samples at Iron Pier Beach. Lower isotope values for samples at 1.5 m depth may be explained by nitrification processes within the STE where oxic conditions are persistent (Kroeger and Charette, 2008), especially at this shallow depth. Alternatively, differences may be related to geologic heterogeneity. At Callahans Beach, fresh groundwaters at 6 m depth were lower in δ15N-NO3– with variable δ11B, compared to shallower and deeper groundwaters (Table 3). This depth horizon is geologically distinct from neighboring sediments. Indeed, Tamborski et al. (2017b) observed significant radon, radium, and dissolved Mn2+ enrichments at this depth horizon. Geologic heterogeneity can act to both modify groundwater flow paths and enhance biogeochemical transformations within the STE (Heiss et al., 2020). However, denitrification cannot explain the differences in δ15N-NO3– and δ18O-NO3– compositions (Figures 3A, 4). In the ensuing analysis, we thus interpret changes in stable isotopes to reflect changes in groundwater N (and B) sources, rather than from a biogeochemical process or cycle, unless explicitly stated.
The nitrogen and oxygen isotope values of NO3– in groundwater from Callahans Beach show no seasonal trends (Table 3). The samples from this study are similar in δ15N-NO3– to previously measured values at that site from 2014 and 2015 (Tamborski et al., 2017a); however, the 2017 samples span a much narrower range in δ18O-NO3 (Figure 3A). Samples from both Callahans Beach and Iron Pier Beach plot in the overlapping fields of nitrification of NH4+ in fertilizer and precipitation, soil NH4+, manure and septic waste (Figure 3A). Previous studies at Callahans Beach attributed the δ15N and δ18O of groundwater NO3– to either nitrification of septic waste, or to mineral fertilizer (Tamborski et al., 2017a). Studies of drinking supply well samples collected in Northport, a sewered community to the southwest of Callahans Beach, have NO3– concentrations less than 10 mg L–1 (Bleifuss et al., 2000). These sewered community groundwaters have higher δ15N-NO3– values compared with those from Iron Pier and Callahans Beach (hollow black circles; Figure 3A). The δ15N-NO3– and δ18O-NO3– values from the Northport supply wells were attributed to nitrification of NH4+ in fertilizer, with small inputs from septic waste (Bleifuss et al., 2000); we note that the soil NH4+ and manure/septic waste source fields also overlap in δ15N-NO3– for these supply well samples (Figure 3A).
Residences in the watershed of Callahans Beach are unsewered and use individual, homeowner septic systems for wastewater disposal (i.e., cesspools); thus, a septic waste N signature is expected for Callahans Beach. Callahans Beach is located immediately down-gradient of a golf course, and in the vicinity of communities that fertilize their lawns (Figure 1), and so a N and B source from fertilizers is also expected. Based on the δ15N-NO3– and δ18O-NO3– data and the endmember source fields identified in Kendall et al. (2008), it is unclear what the dominant source of NO3– is to the groundwaters of the Callahans Beach STE (Figure 3A). Iron Pier Beach is located down-gradient from agricultural fields, so a fertilizer source for the NO3– was expected. Based on δ15N-NO3– and δ18O-NO3– is unclear, however, if the Iron Pier Beach samples are sourced from nitrification of NH4+ in fertilizer and precipitation, soil NH4+, manure or septic waste.
Stable isotope measurements of δ15N-NO3– and δ18O-NO3– are unable to discriminate NO3– sources in the STE, despite local knowledge of each study site’s primary NO3– sources. Comparison of δ15N and δ11B values has allowed the discrimination of anthropogenic NO3– sources in other terrestrial and aquatic-based studies (Komor, 1997; Widory et al., 2004, 2005, 2013; Seiler, 2005; Bronders et al., 2012; Lindenbaum, 2012; Briand et al., 2013, 2017; Eppich et al., 2013; Saccon et al., 2013; Guinoiseau et al., 2018; Kruk et al., 2020). Callahans Beach and Iron Pier Beach have distinct land-use patterns within their respective coastal watersheds (Figure 1). A posteriori knowledge of each site, and of the Long Island region (Bellone, 2015), provides a unique opportunity to compare “known” nitrogen sources to those estimated from δ11B and δ15N-NO3– versus δ18O-NO3–.
Comparison between δ11B and δ15N provides qualitative information on potential groundwater N sources (Figure 3B). Endmember source fields of δ11B from this study (Tables 1, 2), with additional constraints as summarized by Eppich et al. (2013), suggests that groundwater NO3– for Iron Pier Beach is derived from either nitrification of NH4+ in fertilizer or septic waste. In contrast, groundwater NO3– at Callahans Beach is interpreted to reflect either nitrification of NH4+ in precipitation or an animal manure source. Precipitation can supply, at most, 1 mg L–1 of NO3– to the groundwater system of Long Island; therefore, precipitation is not the primary source of the observed N concentrations (Table 3) or δ11B (Figures 2C, 5). Binary mixing models were used to investigate theoretical mixing between different sources of B in the STE. Assuming that B is not attenuated by in situ biogeochemical processes, then mixing between two different endmembers may be quantitatively approximated as:
Figure 5. Log of 1/B vs. δ11B of coastal groundwater samples and different endmembers analyzed in this study.
where X is the fraction of endmember 1 in the coastal groundwater, C1 and δ11B1 are the concentration and isotope composition of endmember 1 and C2 and δ11B2 are the concentration and isotope composition of endmember 2. Estimated endmembers (Figure 5) were used to investigate potential mixing relationships between natural sources (precipitation or seawater) with contaminant sources B (fertilizer, septic waste or animal waste). We note that endmember mixing models may be improved with a Bayesian statistical analysis, which accounts for the degree of overlap and uncertainty of different sources (Ransom et al., 2016); however, a more comprehensive analysis of potential endmembers is required here. The two different subterranean estuaries studied have relatively distinct ranges of δ11B (Figure 2) and therefore we treat each site as a separate system in the ensuing discussion.
Agriculture dominates the lower population density (470 people km–2) watershed of Iron Pier Beach. Groundwater NO3– at this site should be derived from mineral fertilizers. Fresh groundwater samples from Iron Pier Beach are systematically lower in δ11B compared to Callahans Beach (Figure 2). Iron Pier groundwater δ11B increases nearly linearly with increasing B concentration (Figure 2B), suggesting that binary mixing occurred between an isotopically enriched B source and an isotopically depleted, lower [B] source. Precipitation has isotopically high δ11B but is too low in [B] to explain the higher δ11B values of the Iron Pier groundwater (Figure 5). Animal manure is an alternative endmember; however, three coastal groundwater samples are higher in δ11B than the two measured manure samples (Figure 5). Either two samples are insufficient to adequately characterize the δ11B of animal manure (Eppich et al., 2013), or this is not an appropriate endmember for this site.
Seawater has elevated δ11B and [B] and may therefore constitute one endmember for Iron Pier Beach (Figure 5). Groundwater salinities are on the order of 0.3 psu (Table 4); direct mixing between a contaminant endmember and seawater (25–28 psu) would mean that seawater contributes between 1 and 2% of the observed salinity. This amount of seawater [B] could be delivered via sea spray that is diluted by rainfall. Septic waste and fertilizer endmembers (Table 2) are isotopically light enough to serve as the second endmember for Iron Pier Beach. However, B concentrations of the leaches are 1–3 orders of magnitude higher (lower 1/B; Figure 5) than the isotopically lowest δ11B groundwater sample (16 ppb; −2.1‰; Table 4). The [B] of the fertilizers determined from the leaching experiments are not representative of a natural system. Borate minerals are added to commercial fertilizers because B is an essential micro-nutrient for plant growth (Shireen et al., 2018); therefore, a significant fraction of the B from a fertilizer application should be retained by the plant-root system. Further, the leaching experiment used a small amount of water so the leach is much more concentrated than what would be found in irrigation water even if the plant roots were inefficient at utilizing the B.
Theoretical mixing between highly diluted sea spray and fertilizers reasonably explains the coastal groundwater B data of Iron Pier Beach (Figure 6A). These mixing curves represent an isotopically light fertilizer (Table 2) assuming that 5, 10 or 15% of the applied B is lost to the groundwater system. Note that a higher δ11B signature for fertilizer (12.6‰) would simply shift these mixing curves upward toward higher δ11B values, assuming that B concentration ranges remain the same (Figure 6A). Given these assumptions, groundwater N at Iron Pier Beach must be predominantly sourced from nitrification of NH4+ in fertilizer (Figures 3B, 6A), consistent with our working knowledge of this agricultural coastal system. Notably though, there is no trend in NO3– with [B] (Figure 2B), and therefore a simple two-component mix cannot explain the source of NO3– at Iron Pier Beach using N alone (Figure 4).
Figure 6. Log of 1/B vs. δ11B of coastal groundwater samples from Iron Pier Beach (A) and Callahans Beach (B). In panel (A), theoretical mixing between sea spray and fertilizer (orange curves) assumes that sea spray constitutes 5% of the B concentration of seawater, and that B retention from plants is between 85 and 95% of the applied fertilizer load. The horizontal orange dashed lines indicate the range of fertilizer δ11B (MilorganiteTM [–4.4‰] to Scotts Thick’R LawnTM [12.6‰]) over a B concentration range of 20–60 ppb. In panel (B), the dashed curves show mixing between coastal precipitation with possible contaminant endmembers, and solid curves show mixing between sea spray and possible contaminant endmembers, including fertilizer (9‰), septic waste (1.5‰) and animal manure (26‰). Coastal precipitation is set as the highest observed δ11B signature for Callahans Beach (15 ppb, 42.5‰). Manure B concentrations are 20% of the laboratory leached concentration. Fertilizer δ11B is the average of analyzed fertilizer leaches, excluding MilorganiteTM; septic waste δ11B is the average of the analyzed septic waste samples (Table 2).
Callahans Beach is directly down-gradient from medium-density residential housing (2770 people km–2) and a public golf course. Groundwater NO3– at this site should be derived from septic (i.e., cesspool) waste, with minor contributions from fertilizers. The coastal groundwater δ11B of Callahans Beach reflects a much heavier δ11B source compared to measured septic waste (−0.2 – 2.5‰) and fertilizer (Table 2). Anthropogenic B compounds used in detergents and cleaning products have a δ11B signature between −5 and 1‰ (Vengosh et al., 1999), such that the δ11B signature of the measured septic waste may be dominated by detergents, rather than human waste.
The highest δ11B signatures (>39‰) suggest a contribution of B from seawater, although coastal groundwater salinities are low (<0.3) and do not show a trend with [B] or δ11B (Figure 2A). Sea spray was invoked as the isotopically high δ11B endmember for Iron Pier Beach (Figure 6A). At Callahans Beach, B concentrations are systematically lower than Iron Pier Beach, and so despite appreciably high δ11B signatures, [B] cannot be explained by sea spray alone. δ11B signatures higher than seawater isotope values may be derived from boric acid volatilization of seawater (Chetelat et al., 2005). The highest δ11B we measured for rainwater is 33.4‰ (Table 1), but coastal precipitation can be much heavier (Chetelat et al., 2005). Therefore, we suggest that the high δ11B endmember of Callahans Beach results from boric acid volatilization of seawater, resulting in a higher δ11B coastal precipitation endmember (Figure 6B). The highest δ11B value for Callahans Beach, 42.5‰ (CH4, November 2017; Table 3) has relatively low NO3– concentrations (3.7 mg NO3– L–1 or 0.84 mg N L–1), similar to the N concentration of regional precipitation (Suffolk County Government, 2019). In the ensuing analysis, this sample is assumed to represent the B endmember of regional coastal precipitation.
Mixing relationships between sea spray and coastal precipitation were examined with respect to septic waste (1.5‰), animal manure (26‰) and fertilizer (9‰; Figure 6B). Sea spray B concentrations were assumed to represent 5% of measured seawater concentrations. Notably, because there is little change in [B] for a large range in δ11B, no single mixing curve will fit all of the Callahans Beach data. Therefore, it is not possible to demonstrate with certainty using B whether the N source is derived from fertilizer or septic waste (via individual homeowner cesspools). However, the range of values demonstrates that there is not one single endmember, consistent with our knowledge that groundwater NO3– within this watershed is derived from multiple non-point sources.
Coastal groundwaters collected during 2014 and 2015 are distinct in δ18O-NO3– and δ11B compared to samples from the same wells collected during 2017 (Figure 3). The B signatures of several 2015 samples match precipitation endmember B signatures, suggesting that recently recharged groundwaters obtain B and N from precipitation (Figure 6A). Importantly, this data demonstrates annual variability in groundwater flow and contaminant transport to LIS. While coastal groundwaters collected throughout 2017 showed relatively higher values of δ11B, it remains to be seen how contaminant delivery varies over longer time periods for this sole-source, unconfined aquifer.
In the coastal systems studied here, B and NO3– are added to the groundwater system in different ways, and are impacted by precipitation and seawater (sea spray versus boric acid volatilization) differently (Figure 7). Precipitation and seawater both have relatively low N concentrations (<1 mg L–1). Seawater is a distinct endmember, due to its high [B] and its isotopically high δ11B signature (Figure 7). Boric acid volatilization of seawater produces isotopically high B, and thus gives a very distinctive low B concentration endmember for coastal precipitation. It is interesting that two subterranean estuaries from similar settings have distinct sources of ‘uncontaminated’ waters. One has to consider differences in pathways for B from both fertilizer and septic waste. In the case of fertilizer, plants use B as a micro-nutrient, and so the concentration of B that is added to the groundwater system will be lower than what was applied to the land surface. In contrast, [B] in septic waste does not change. Therefore, homeowner cesspools likely reflect different sources of B and NO3– to the groundwater system (Figure 7). Septic waste δ11B integrates multiple contaminant sources, including detergents, commercial cleaning products and human waste. Given the low δ11B signature of septic waters (Table 2), B in detergents must dominate the B signature of septic waste. In contrast, human waste is the primary N source in septic waste and therefore B and N are decoupled in septic systems (Figure 7). Importantly, there is no relationship between B and NO3– concentrations in either of the studied subterranean estuaries (Figure 2).
Figure 7. Summary of δ11B (left) and δ15N (right) endmember isotope ratios of coastal groundwaters. Dotted boxes reflect natural sources or processes. Dashed boxes reflect anthropogenic contaminant sources. δ11B ratios constrained from Tables 1, 2. δ15N ratios from Kendall et al. (2008).
Application of boron isotopes in coastal settings is vulnerable to the influence of seawater (via sea spray), which has orders of magnitude higher boron concentrations compared to various contaminant sources. Identification and discrimination of NO3– and B sources in coastal settings thus requires (1) adequate characterization of local sources (both natural waters and contaminants) and (2) an understanding of biogeochemical processes within the STE. Coastal groundwaters are collected from beaches in SGD studies to integrate terrestrial groundwater flow paths over the entirety of the coastal watershed and to account for any biogeochemical processes (i.e., denitrification) in the STE that would influence N concentration and speciation prior to discharge. The advantage of collecting coastal groundwater to account for N transformation may thus hinder the utility of B as an auxiliary N source tracer if seawater significantly modifies subsurface geochemical signatures. The results from this study demonstrate the utility of multi-isotope tracing techniques to identify N sources in polluted coastal aquifers. Future studies should explicitly measure isotopic values of different possible endmembers in order to constrain local variability in N and B sources.
The datasets generated for this study are available on request to the corresponding author.
CB and JT collected the field data under the supervision of HB, JC, and ER. Boron isotopes were analyzed in the lab of ER. All authors participated in the data analysis and interpretation.
This research was funded by New York Sea Grant projects R/CMC-13 and R/CMC-13-NYCT. The MC-ICP-MS used for this work was funded through NSF-MRI 0959524.
The authors declare that the research was conducted in the absence of any commercial or financial relationships that could be construed as a potential conflict of interest.
JT acknowledges funding from the Canada First Research Excellence Fund, through the Ocean Frontier Institute. Katie Wooton, Deanna Downs, and Brooke Peritore (Stony Brook University FIRST lab) performed all of the boron isotope analyses for the fresh waters and potential contaminant endmembers.
Anschutz, P., Charbonnier, C., Deborde, J., Deirmendjian, L., Poirier, D., Mouret, A., et al. (2016). Terresrial groundwater and nutrient discharge along the 240-km-long aquitanian coast. Mar. Chem. 185, 38–47. doi: 10.1016/j.marchem.2016.04.002
Aravena, R., Evans, M. L., and Cherry, J. A. (1993). Stable isotopes of oxygen and nitrogen in source identification of nitrate from septic systems. Ground Water 31, 180–186. doi: 10.1111/j.1745-6584.1993.tb01809.x
Bannon, R. O., and Roman, C. T. (2008). Using stable isotopes to monitor anthropogenic nitrogen inputs to estuaries. Ecol. Appl. 18, 22–30. doi: 10.1890/06-2006.1
Barth, S. (1998). Application of boron isotopes for tracing sources of anthropogenic contamination in groundwater. Water Resour. 32, 685–690. doi: 10.1016/s0043-1354(97)00251-0
Bassett, R. L., Buszka, P. M., Davidson, G. R., and Chong-Diaz, D. (1995). Identification of groundwater solute sources using boron isotopic composition. Environ. Sci. Technol. 29, 2915–2922. doi: 10.1021/es00012a005
Bateman, A. S., and Kelly, S. D. (2007). Fertilizer nitrogen isotope signatures. Isotop. in Environ. Health Stud. 43, 237–247. doi: 10.1080/10256010701550732
Bellone, S. (2015). Suffolk County Comprehensive Water Resources Management Plan. Suffolk County, NY: Department of Health Services.
Bleifuss, P., Hanson, G., and Schoonen, M. (2000). Tracing Sources of Nitrate in the Long Island Aquifer System, SUNY-Stony Brook. Ph.D. Thesis, SUNY Stony Brook, New York, NY.
Bloesch, P., Bell, L., and Hughes, J. (1987). Adsorption and desorption of boron by goethite. Soil Res. 25, 377–390.
Bokuniewicz, H. J., Cochran, J. K., Garcia-Orellana, J., Rodellas, V., Daniel, J. W., and Heilbrun, C. (2015). Intertidal percolation through beach sands as a source of 224,223Ra to Long Island Sound, New York, and Connecticut, United States. J. Mar. Res. 73, 123–140. doi: 10.1357/002224015816665570
Brandes, J. A., and Devol, A. H. (1997). Isotopic fractionation of oxygen and nitrogen in coastal marine sediments. Geochim. Cosmochim. Acta 61, 1793–1801. doi: 10.1016/s0016-7037(97)00041-0
Briand, C., Plagnes, V., Sebilo, M., Louvat, P., Chesnot, T., Schneider, M., et al. (2013). Combination of nitrate (N, O) and boron isotopic ratios with microbiological indicators for the determination of nitrate sources in karstic groundwater. Environ. Chem. 10, 365–369.
Briand, C., Sebilo, M., Louvat, P., Chesnot, T., Vaury, V., Schneider, M., et al. (2017). Legacy of contaminant N sourves to the NO3-signature in rivers: a combined isotope delta N- 150NO3-, delta O-18- NO3-, delta B-11) and microbiological investigation. Sci. Rep. 7:41703.
Bronders, J., Tirez, K., Desmet, N., Widory, D., Petelet-Giraud, E., Bregnot, A., et al. (2012). Use of Compound-Specific Nitrogen (δ15N), Oxygen (δ18O), and Bulk Boron (δ11B) isotope ratios to identify sources of nitrate-contaminated waters: a guideline to identify polluters. Environ. Forens. 13, 32–38. doi: 10.1080/15275922.2011.643338
Brown, C. (2018). Using Boron to Trace Anthropogenic Sources of Nitrogen in Long Island Sound. Ph.D. Thesis, Stony Brook University, New Tork, NY.
Burnett, W. C., Bokuniewicz, H., Huettel, M., Moore, W. S., and Taniguchi, M. (2003). Groundwater and pore water inputs to the coastal zone. Biogeochemistry 66, 3–33. doi: 10.1023/b:biog.0000006066.21240.53
Buxton, H., and Modica, E. (1992). Patterns and rates of groundwater flow on Long Island. New York. Ground Water 30, 857–866. doi: 10.1111/j.1745-6584.1992.tb01568.x
Charbonnier, C., Anschutz, P., Poirier, D., Bujan, S., and Lecroart, P. (2013). Aerobic respiration in a high-energy sandy beach. Mar. Chem. 155, 10–21. doi: 10.1016/j.marchem.2013.05.003
Chetelat, B., Gaillardet, J., Freydier, R., and Négreic, Ph. (2005). Boron isotopes in precipitation: experimental constraints and field evidence from French Guiana. Earth Plan. Sci. Lett. 235, 16–30. doi: 10.1016/j.epsl.2005.02.014
De La Fuente, G. S. M. M., and Camacho, E. M. (2009). Boron removal by means of adsorption processes with magnesium oxide — Modelization and mechanism. Desalination 249, 626–634. doi: 10.1016/j.desal.2008.11.016
Demetriou, A., and Pashalidis, I. (2012). Adsorption of boron on iron-oxide in aqueous solutions. Desalin. Water Treat. 37/38, 315–320. doi: 10.1080/19443994.2012.661288
Doane, T. A., and Howarth, W. R. (2003). Spectrophotometric determination of nitrate with a single reagent. Analy. Lett. 36, 2713–2722. doi: 10.1081/al-120024647
Durand, J. (2014). Characterization of the Spatial and Temporal Variations of Submarine Groundwater Discharge Using Electrical Resistivity and Seepage Measurements. Ph.D. Thesis, Stony Brook University, New York, NY.
Eppich, G. R., Singleton, M. J., Wimpenny, J. B., Yin, Q.-Z., and Esser, B. K. (2013). California GAMA Special Study: Stable Isotopic Composition of Boron in Groundwater – San Diego County Domestic Well Data. LLNL-TR-533174. Livermore, CA: Lawrence Livermore National Lab.(LLNL), 22.
Erler, D. I., Santos, Y., Zhang, D., Tait, K., Befus, A., Hidden, L., et al. (2014). Nitrogen transformations within a tropical subterranean estuary. Mar. Chem. 164, 38–47. doi: 10.1016/j.marchem.2014.05.008
Garcia-Orellana, J. J. K., Bokuniewicz, C. H., Daniel, J. W. R., Rodellas, V., and Heilbrun, C. (2014). Geochim. Cosmochim. Acta 141, 314–330.
Glavee, G. N., Klabunde, K. J., Sorensen, C. M., and Hadjipanayis, G. C. (1995). Chemistry of borohydride reduction of iron(II) and iron(III) Ions in aqueous and nonaqueous media. formation of nanoscale Fe, FeB, and Fe2B powders. Inorga. Chem. 34, 28–35. doi: 10.1021/ic00105a009
Gobler, C., and Sanudo-Wilhelm, S. (2001). Temporal variability of groundwater seepage and brown tide blooms in a Long Island embayment. Mar. Ecol. Prog. Ser. 217, 299–309. doi: 10.3354/meps217299
Goldberg, S., and Glaubig, R. (1985). Boron adsorption on aluminum and iron oxide minerals. Soil Sci. Soc. Am. J. 49, 1374–1379. doi: 10.2136/sssaj1985.03615995004900060009x
Granger, J., and Sigman, D. M. (2009). Removal of nitrite with sulfamic acid for nitrate N and O isotope analysis with the denitrifier method. Rapid Commun. Mass Spectrom. 23, 3753–3762. doi: 10.1002/rcm.4307
Guinoiseau, D., Louvat, P., Paris, G., Chen, J. B., Cheelat, B., Rocher, V., et al. (2018). Are boron isotopes a reliable tracer of anthropogenic inputs to rivers over time? Sci. Total Environ. 626, 1057–1068. doi: 10.1016/j.scitotenv.2018.01.159
Heiss, J. W., Michael, H. A., and Koneshloo, M. (2020). Denitrification hotspots in intertidal mixing zones linked to geologic heterogeneity. Environ. Res. Lett. (in press). doi: 10.1088/1748-9326/ab90a6
Howarth, R. W., and Marino, R. (2006). Nitrogen as the limiting nutrient for eutrophication in coastal marine ecosystems: evolving views over three decades. Limnol. Oceanogr. 51, 364–376. doi: 10.4319/lo.2006.51.1_part_2.0364
Kendall, C., Elliott, E. M., and Wankel, S. D. (2008). “Tracing anthropogenic inputs of nitrogen to ecosystems,” in Stable Isotopes in Ecology and Environmental Science, ed. R. H. M. A. K. Lajtha (Hoboken,NY: Blackwell Publishing), 375–449. doi: 10.1002/9780470691854.ch12
Komor, S. C. (1997). boron contents and isotopic compositions of hog manure, selected fertilizers, and water in minnestoa. J. Environ. Qual. 26, 1212–1222. doi: 10.2134/jeq1997.00472425002600050004x
Kroeger, K. D., and Charette, M. A. (2008). Nitrogen biogeochemistry of submarine groundwater discharge. Limnol. Oceanogr. 53, 1025–1039. doi: 10.4319/lo.2008.53.3.1025
Kruk, M. K., Mayer, B., Nightingale, M., and Laceby, J. P. (2020). Tracing nitrate sources with a combined isotope approach (d15NNO3, d18ONO3 and d11B) in a large mixed-use watershed in southern Alberta. Canada. Sci. Total Environ. 703:135043. doi: 10.1016/j.scitotenv.2019.135043
Lemarchand, D., Gaillardet, J., Göpel, C., and Manhès, G. (2002). An optimized procedure for boron separation and mass spectrometry analysis for river samples. Chem. Geol. 182, 323–334. doi: 10.1016/S0009-2541(01)00329-1
Lemarchand, E., Schott, C., and Gaillardet, J. (2007). How surface complexes impact boron isotope fractionation: Evidence from Fe and Mn oxides sorption experiments. Earth Planet. Sci. Lett. 260, 277–296. doi: 10.1016/j.epsl.2007.05.039
Lindenbaum, J. (2012). Identification of Sources of Ammonium in Groundwater Using Stable Nitrogen and Boron Isotopes in Nam Du. Hanoi: Lund University.
McClymonds, N. E., and Franke, O. L. (1972). Water-transmitting properties of aquifers on Long Island, New York. Geolo. Survey Profess. Paper 627:24.
McPhail, M., Page, A., and Bingham, F. (1972). Adsorption interactions of monosilicic and boric acid on hydrous oxides of iron and aluminum. Soil Sci. Soc. Am. J. 36, 510–514. doi: 10.2136/sssaj1972.03615995003600030039x
Miranda, K. M., Espey, M. G., and Wink, D. A. (2001). A rapid, simple spectrophotometric method for simultaneous detection of nitrate and nitrite. Nitric Oxide 5, 62–71. doi: 10.1006/niox.2000.0319
Moore, W. S. (1999). The subterranean estuary: a reaction zone of ground water and sea water. Mar. Chem. 65, 111–125. doi: 10.1016/s0304-4203(99)00014-6
Paerl, H. W. (1995). Coastal eutrophication in relation to atmospheric nitrogen deposition: current perspectives. Ophelia 41, 237–259. doi: 10.1080/00785236.1995.10422046
Palmer, M. R., Spivack, A. J., and Edmond, J. M. (1987). Temperature and pH controls over isotopic fractionation during adsorption of boron on marine clay. Geochim. Cosmochim. Acta 51, 2319–2323. doi: 10.1016/0016-7037(87)90285-7
Presley, B., and Claypool, G. (1971). Techniques for analyzing interstitial water samples. Part 1, 1749–1755.
Ransom, K. M., Grote, M. N., Deinhart, A., Eppich, G., Kendall, C., Sanborn, M. E., et al. (2016). Bayesian nitrate source apportionment to individual groundwater wells in the Central Valley by use of elemental and isotopic tracers. Water Resour. Res. 52, 5577–5597. doi: 10.1002/2015WR018523
Rodellas, V., Garcia-Orellana, J., Masqué, P., Feldman, M., and Weinstein, Y. (2015). Submarine groundwater discharge as a major source of nutrients to the Mediterranean Sea. Proc. Natl. Acad. Sci. U.S.A. 112, 3926–3930. doi: 10.1073/pnas.1419049112
Saccon, P., Leis, A., Marca, A., Kaiser, J., Campisi, L., Bottcher, M. E., et al. (2013). Multi-isotope approach for the identification and characterization of nitrate pollution sources in the Marano lagoon (Italy) and parts of its catchment area. Appl. Geochem. 34, 75–89. doi: 10.1016/j.apgeochem.2013.02.007
Seiler, R. L. (2005). Combined use of 15N and 18O of nitrate and 11B to evaluate nitrate contamination in groundwater. Appl. Geochem. 20, 1626–1636. doi: 10.1016/j.apgeochem.2005.04.007
Shireen, F., Nawaz, M., Chen, C., Zhang, Q., and Zheng, Z. (2018). Boron: functions and approaches to enhance its availability in plants for sustainable agriculture. Int. J. Mol. Sci. 19:1856. doi: 10.3390/ijms19071856
Slomp, C. P., and Van Cappellen, P. (2004). Nutrient inputs to the coastal ocean through submarine groundwater discharge: controls and potential impact. J. Hydrol. 295, 64–86. doi: 10.1016/j.jhydrol.2004.02.018
Suffolk County Government (2019). Available online at: https://www.suffolkcountyny.gov/Departments/Economic-Development-and-Planning/Planning-and-Environment/ReclaimOurWaterInitiativeUpdate (accessed June 19, 2019).
Tamborski, J. J., Cochran, J. K., and Bokuniewicz, H. J. (2017a). Submarine groundwater discharge driven nitrogen fluxes to Long Island Sound, NY: Terrestrial vs. marine sources. Geochim. Cosmochim. Acta 218, 40–57. doi: 10.1016/j.gca.2017.09.003
Tamborski, J. J., Cochran, J. K., and Bokuniewicz, H. J. (2017b). Application of 224Ra and 222Rn for evaluating seawater residence times in a tidal subterranean estuary. Mar. Chem. 189, 32–45. doi: 10.1016/j.marchem.2016.12.006
Tamborski, J. J., Rogers, A. D., Bokuniewicz, H. J., Cochran, J. K., and Young, C. R. (2015). Identification and quantification of diffuse fresh submarine groundwater discharge via airborne thermal infrared remote sensing. Remote Sens. Environ. 171, 202–217. doi: 10.1016/j.rse.2015.10.010
Taniguchi, M., Dulai, H., Burnett, K. M. I, Santos, R., Sugimoto, R., Stieglitz, T., et al. (2019). Submarine groundwater discharge: updates on its measurement techniques, geophysical drivers, magnitudes, and effects. Front. Environ. Sci. 7:141. doi: 10.3389/fenvs.2019.00141
Tirez, K., Brusten, W., Widory, D., Petelet, E., Bregnot, A., Xue, D., et al. (2010). Boron isotope ratio (δ11B) measurements in Water Framework Directive monitoring programs: comparison between double focusing sector field ICP and thermal ionization mass spectrometry. J. Anal. Atom. Spectrom. 25, 964–974.
Vengosh, A. (1998). The isotopic composition of anthropogenic boron and its potential impact on the environment. Biolo. Trace Elem. Res. 66, 145–151. doi: 10.1007/bf02783134
Vengosh, A., Barth, S., Heumann, K. G., and Eisenhut, S. (1999). Boron isotopic composition of freshwater lakes from central europe and possible contamination sources. Acta Hydrochim. Hydrobiol. 27, 416–421. doi: 10.1002/(sici)1521-401x(199912)27:6<416::aid-aheh416>3.0.co;2-2
Vengosh, A., Heumann, K. G., Juraske, S., and Kasher, R. (1994). Boron isotope application for tracing sources of contamination in groundwater. Environ. Sci. Technol. 28, 1968–1974. doi: 10.1021/es00060a030
Widory, D., Kloppmann, W., Chery, L., Bonnin, J., Rochdi, H., and Guinamant, J. L. (2004). Nitrate in groundwater: an isotopic multi-tracer approach. J. Contam. Hydrol. 72, 165–188. doi: 10.1016/j.jconhyd.2003.10.010
Widory, D., Petelet-Giraud, E., Brenot, A., Bronders, J., Tirez, K., and Boeckx, P. (2013). Improving the management of nitrate pollution in water by the use of isotope monitoring: the δ15N, δ18O and δ11B triptych. Isotopes Environ. Health Stud. 49, 29–47. doi: 10.1080/10256016.2012.666540
Widory, D., Petelet-Giraud, E., Négrel, P., and Ladouche, B. (2005). Tracking the sources of nitrate in groundwater using coupled nitrogen and boron isotopes: a synthesis. Environ. Sci. Technol. 39, 539–548. doi: 10.1021/es0493897
Keywords: submarine groundwater discharge, boron, nitrogen, nitrate, fertilizer, wastewater, septic waste
Citation: Tamborski J, Brown C, Bokuniewicz H, Cochran JK and Rasbury ET (2020) Investigating Boron Isotopes for Identifying Nitrogen Sources Supplied by Submarine Groundwater Discharge to Coastal Waters. Front. Environ. Sci. 8:126. doi: 10.3389/fenvs.2020.00126
Received: 24 October 2019; Accepted: 16 July 2020;
Published: 11 August 2020.
Edited by:
Ryo Sugimoto, Fukui Prefectural University, JapanReviewed by:
David Widory, Université du Québec à Montréal, CanadaCopyright © 2020 Tamborski, Brown, Bokuniewicz, Cochran and Rasbury. This is an open-access article distributed under the terms of the Creative Commons Attribution License (CC BY). The use, distribution or reproduction in other forums is permitted, provided the original author(s) and the copyright owner(s) are credited and that the original publication in this journal is cited, in accordance with accepted academic practice. No use, distribution or reproduction is permitted which does not comply with these terms.
*Correspondence: Henry Bokuniewicz, aGVucnkuYm9rdW5pZXdpY3pAc3Rvbnlicm9vay5lZHU=
†Present address: Caitlin Brown, US Environmental Protection Agency, Boston, MA, United States
Disclaimer: All claims expressed in this article are solely those of the authors and do not necessarily represent those of their affiliated organizations, or those of the publisher, the editors and the reviewers. Any product that may be evaluated in this article or claim that may be made by its manufacturer is not guaranteed or endorsed by the publisher.
Research integrity at Frontiers
Learn more about the work of our research integrity team to safeguard the quality of each article we publish.