- 1Department of Chemistry and Biochemistry, University of Minnesota Duluth, Duluth, MN, United States
- 2Department of Process Engineering, Eawag, Swiss Federal Institute of Aquatic Science and Technology, Dübendorf, Switzerland
Characterizing the impact of nanoplastics to organism health is important to understand the consequences of the environmental plastic waste problem. This article examines the impact of nano-polystyrene (nano-PS; 159 ± 0.9 nm diameter) to ecologically relevant bacteria Shewanella oneidensis. Bacterial viability was evaluated using a growth-based assay. Riboflavin secretion is a critical cell function of S. oneidensis, serving as an electron mediator in anaerobic respiration and/or as a signaling molecule when the bacteria are under stress. Thus, changes in cellular function were monitored through riboflavin secretion in order to evaluate toxic responses that may not result in cell death. Under aerobic and anaerobic exposures (4, 8, or 12 h), the viability of the S. oneidensis was minimally changed as compared to the control, while the concentration of riboflavin secreted varied with exposure dose. In order to determine if this was a specific response to nanoplastic particles, opposed to a response to either particles or plastic more generally, we exposed the system to colloidal TiO2 nanoparticles and polystyrene and polyethylene thin films. We confirmed that riboflavin secretion trends were specific to nano-PS and not to these other materials, which showed no significant changes. We investigated the association of the nano-PS with ICP-MS using Pd that was chemically incorporated into the model nanoplastics. While 59.2% of the nano-PS were found in the non-cellular culture media, 7.0 and 6.6% was found associated with the loosely and tightly bound extracellular polymeric substance, respectively. There was significantly more nano-PS (10.9%) strongly associated with the cells. Taken together, we found that nano-PS had minimal impacts to viability but caused a significant change in the function of S. oneidensis that can be related to the nano-PS attached or in proximity to the bacterium. These trends are consistent between aerobic and anaerobic cultures, signifying that the stress response of S. oneidensis can be generalized between different environmental compartments. This work highlights that the association of nanoplastic materials with microorganisms may modify the cellular function that could ultimately be an impact to ecosystem health.
Introduction
Plastic-particle distributions in marine and freshwater systems are an active area of research, with most current work centered on the size distribution, polymer type, and number of particles above 1 micron (Wagner et al., 2014; Zbyszewski et al., 2014; Driedger et al., 2015; Hendrickson et al., 2018; Allen et al., 2019), and estimates suggest there could be up to 125 trillion microplastics in ocean water (Lindeque et al., 2020). Particulate plastics (nano- and microplastic particles, fragments and fibers) pose a concern for organisms in our environment because they can cause a myriad of health outcomes, such as impacting the gill cavity of crab Carcinus maenas causing altered O2 consumption (Watts et al., 2016) or causing inflammation of the liver tissue of zebrafish (Lu et al., 2016). Exposure to microplastics is often through the ingestion pathway because the size range overlaps with that of phytoplankton in both fresh and salt waters, which has resulted in the ingestion of microplastic particles by invertebrates and fish (Imhof et al., 2016). Ingestion is thought to pose challenges to various organisms such as false satiation, leading to nutritional deficits, (Welden and Cowie, 2016; Yin et al., 2018) tissue inflammation (Lu et al., 2016) or toxicity of additives leaching from plastic into tissue (Rochman et al., 2012; Tanaka et al., 2015). While environmental microplastic hazards are concerning, there is growing worry for the impact of nanoplastics in the environment as laboratory studies have shown that they are likely prevalent within these natural systems (Koelmans et al., 2015; Gangadoo et al., 2020; Hebner and Maurer-Jones, 2020). Nanoplastics are particularly concerning with regard to ecosystem health because they have the potential to permeate cell membranes and disrupt cellular behavior (Rossi et al., 2014) while also having a larger surface area-to-volume ratio, increasing the additive leaching potential (Bouwmeester et al., 2015; Hermabessiere et al., 2017).
Nanoparticle toxicity, largely focused on engineered inorganic materials, has been widely studied with the growing demand of novel nanomaterials along with their incorporation into commercially available products. Efforts in this arena have established the framework for studying the interaction between nanoparticles and organisms at various trophic levels (Maurer-Jones et al., 2013b; Zhang et al., 2018) and there are substantial efforts to have the toxicity response to inorganic nanomaterials inform nanoparticle design (Buchman et al., 2019a). Yet, there is much less understood about the toxicity of nanoplastics. The most studied nanoplastic in terms of toxicity is nanoparticle polystyrene (nano-PS) because of its commercial availability with a variety of controlled size ranges and surface chemistries. Many nano-PS toxicity studies have focused on a range of ecologically relevant, multi-cellular model organisms (Besseling et al., 2014; Della Torre et al., 2014; Greven et al., 2016; Chen et al., 2017; Pitt et al., 2018) but there is a gap in knowledge in the toxicity of nano-PS to single-celled organisms.
Toxicity evaluation of single-celled microorganisms is critical because these organisms provide a bedrock of the ecosystems. To date, studies of nano-PS toxicity has primarily focused on algal species. For example, algae exposed to nano-PS have demonstrated a decrease in photosynthetic activity due to their adsorption onto the surface of the algae, and thus screening sunlight (Bhattacharya et al., 2010). Beyond the toxic implications for the algae itself, sorption to the surface can also be the first step within the food web for bioaccumulation of nanoplastic, moving from algae to water fleas to carp (Cedervall et al., 2012). These studies of photosynthetic activity changes and trophic transfer highlight the important role that microorganisms play within the context of nanoplastic hazards to larger organisms. It is expected, due to the close algal-bacterial relationship (Ramanan et al., 2016), that the influence of nano-PS to bacteria systems could be similar, including changes to the primary productivity from these organisms, the transfer of nano-PS to larger organisms through ingestion, or changes in health to other organisms that rely on the cellular function of the microorganisms (Azam and Malfatti, 2007). Yet, there is not a wide understanding of the impact of nano-PS to ecologically relevant bacteria. In bacteria models, Fu et al. (2018) showed nano-PS causes a decrease in growth and metabolism of Acetobacteroides hydrogenigenes, a species relevant to anaerobic digestion. Qu and coworkers studied the impact of micro- and nano-PS to marine bacteria Halomonas alkaliphilia and showed both sizes of particles inhibited growth at high concentrations but that nano-PS also negatively impacted the ammonia conversion efficiencies of the bacteria (Sun et al., 2018). There is still a lot to be understood about the impact of nano-PS to other ecologically relevant bacteria, including where the nanoplastics are in relationship to the bacteria (i.e., associated to or localized within the bacteria) and/or the response to nano-PS from a bacterial species in a range of growing conditions such as aerobic and anaerobic settings.
Shewanella oneidensis is an ideal model bacterium for nanoplastic studies because they are a facultative anaerobe (i.e., they can switch between anaerobic and aerobic respiration), are distributed worldwide and live in varied environmental conditions (e.g., variable salt concentrations and/or temperatures) (Hau and Gralnick, 2007). The fact that these bacteria live in such diverse conditions means it is likely that the organism will encounter nano-PS in a natural system. Additionally, their adaptability may aid in ecosystem modeling as their response may inform our understanding of the impact of nano-PS to similar organisms. In anaerobic conditions, Shewanellae have a unique ability to respire on compounds found in the environment, such as reducing iron to its bioavailable state [Fe(III) to Fe(II)] (Valls and De Lorenzo, 2002; Hau and Gralnick, 2007; Shi et al., 2007), which contribute to the process of metal cycling that other organisms within the environment rely on for their survival (Sheng and Fein, 2014; Wang et al., 2016). The metal reduction function is facilitated through the secretion of flavin mediators, including riboflavin and flavin mononucleotide. Riboflavin secretion is a critical cell function of S. oneidensis, serving as both an electron mediator in anaerobic respiration and as a signaling molecule when the bacteria are under stress in both aerobic and anaerobic environments (Brutinel et al., 2013; Oram and Jeuken, 2019). Finally, this model organism has been used in previous nanoparticle toxicity assessments and thus provides precedent benchmarks for toxicity responses that go beyond only live versus dead cell counts, with evaluation of how substances induce changes of cellular function and gene expression (Maurer-Jones et al., 2013a, c; Zhi et al., 2019; Buchman et al., 2020; Clement et al., 2020). Taken together, these advantages provide an improved understanding as to what cellular processes are most impacted and how this will in turn influence the surrounding environment.
To address the gaps in knowledge of nanoplastic toxicity to single-celled organisms, we evaluated the impacts of nano-PS on the model bacteria S. oneidensis MR-1. The toxicity evaluation included assessments of the viability and riboflavin secretion and iron reduction upon exposure to varied concentrations of nano-PS under both aerobic and anaerobic culture conditions. These sublethal endpoints are critical to more completely evaluate the environmental risk of nanoplastics. While previous work discussed above had characterized inorganic nanoparticle toxicity in aerobic conditions, this is the first study of toxicity to S. oneidensis under anaerobic conditions. We hypothesized that the markers of respiration (i.e., riboflavin secretion) would be varied between the two culture conditions, thus suggesting that nano-PS interrupts the signaling and iron reduction capabilities. To better understand the changes observed in cellular function, we evaluated the association of the nano-PS with the cells using ICP-MS, taking advantage of a Pd-label incorporated into the particle. While increasing understanding of nano-PS impacts to bacteria, this work provides an example of toxicity evaluation that considers diverse culture conditions (aerobic and anaerobic) that could enable generalization of the nano-PS toxicity response.
Materials and Methods
A suite of analyses was used to assess the impact of nano-PS to S. oneidensis. The approach of our analyses is shown in Figure 1.
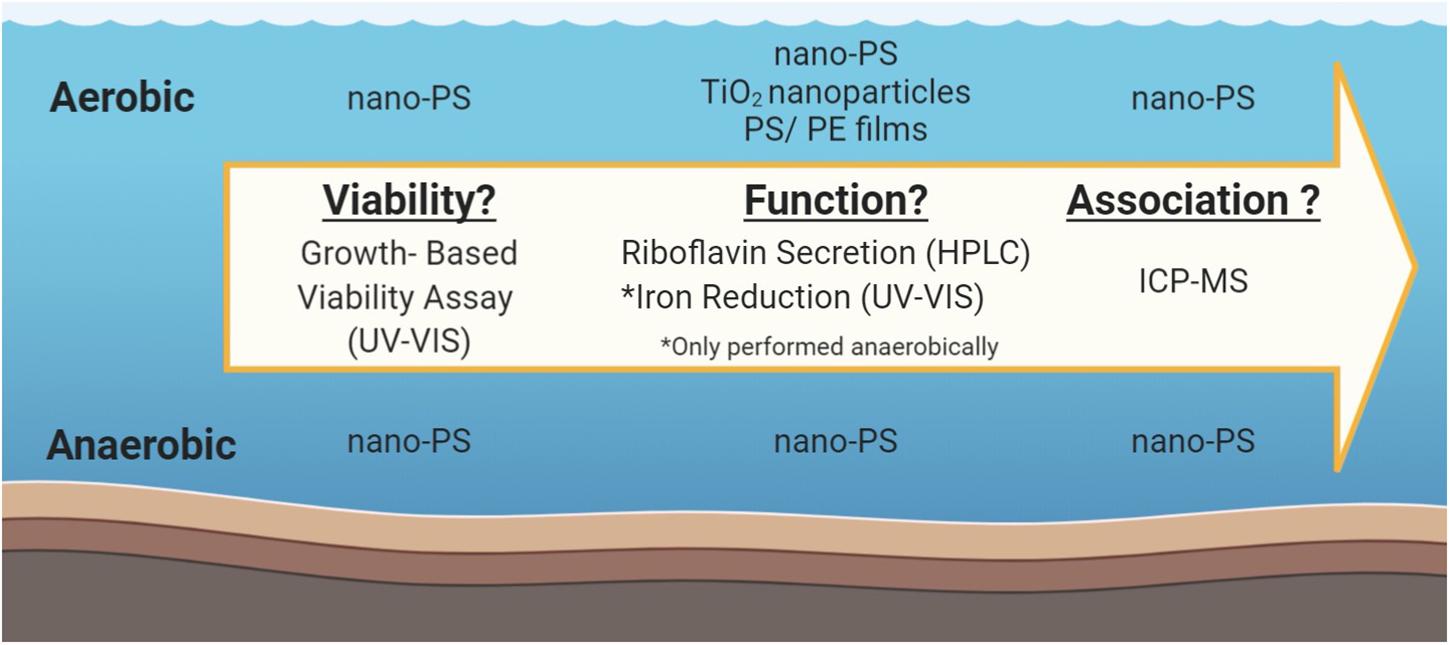
Figure 1. Schematic of experimental workflow, including the exposure materials studied in the various assays.
Exposure Materials
Nano-PS was synthesized and characterized as previously described in Mitrano et al. (2019). Briefly, a polyacrylonitrile material containing a chemically entrapped Pd-tracer was capped with crosslinked polystyrene shell, ultimately resulting in a core/shell nanoplastic, which was approximately 160 nm in diameter. On average, the nano-PS contained 0.49% Pd, which equated to 5.1 × 10–9 ng Pd per nano-PS particle. The nanoplastic particle demonstrated a high stability, with no leaching of the Pd tracer in complex matrices. Therefore, we are confident the interaction of the bacteria is with the polystyrene shell material and all impacts to bacterial cell viability and function is due to the plastic, and not the metal tracer. S. oneidensis were exposed to other materials including TiO2 nanoparticles (25 nm, anatase; Aldrich, Burlington, MA, United States) and polystyrene (30 μm thick) and polyethylene (25 μm thick) thin films (Goodfellow, Huntington England). Thin films were pre-processed by soaking in n-hexane, methanol, and water, each for 24 h prior to bacteria experiments to remove potential contaminants from the commercially acquired films that could cause bacterial toxicity. A single 1 × 1 cm piece (∼3 mg) of pre-soaked and dried film was placed directly into the bacterial culture.
Bacterial Cell Culture
S. oneidensis MR-1(ATCC BAA-1096, Manassas VA, United States) was inoculated in Luria-Burtani (LB, Fisher Scientific) growth media overnight at 30°C with shaking at 200 rpm under aerobic conditions. After inoculation, cells were washed in triplicate with M4 growth media via centrifugation at 1500 rpm for 15 min followed by resuspension in fresh media. After washing, cells were diluted with M4 to a specific optical density by monitoring the absorbance at 600 nm (OD600). M4 broth is a media with little bioavailable carbon and is optimized to sustain bacteria, though does not encourage growth (detailed preparation of M4 in Supplementary Information). M4 was chosen to prevent artificially nutrient rich conditions of the LB and also encourage an interaction of S. oneidensis with the nano-PS because there it has minimal bioavailable carbon.
Growth Based Viability Assay
Using an adapted growth-based viability assay developed by Qiu et al. (2017) the viability of S. oneidensis was quantified after exposure to nano-PS. Briefly, the assay relates the onset of exponential growth of S. oneidensis to calibrate viability, using a mathematical model to fit the exponential growth phase. Bacteria exposure was performed under aerobic and anaerobic environments with nano-PS suspended in MQ water at concentrations ranging from 18.75 to 300 mg/L for periods of 4, 8, or 12 h. These dosages were chosen as they cover the exposure ranges previously studied in other nanoplastic toxicity evaluations (Sun et al., 2018; Shen et al., 2019). The exposure times were chosen because the bacteria were able to be sustained for this period of time in the M4 broth without displaying stress caused from lack of bioavailable carbon. For aerobic growth studies, cells were diluted to an OD600 of 0.5 in M4 broth, which corresponds to approximately 5 × 108 cells/mL. For anaerobic growth, where the doubling rate of S. oneidensis is significantly slowed, the M4 media was supplemented with 15 mM lactate to allow for cell survival during the prolonged experiments, while the LB was supplemented with 20 mM lactate and 20 mM fumarate to allow for anaerobic growth. Anaerobic conditions were achieved by purging samples with an N2/CO2/H2 [85:10:5] gas mixture in an anaerobic chamber (Coy Lab Products, Grass Lake MI, United States) and cells were equilibrated for 4 h prior to exposure. Growth was monitored by optical density readings at 600 nm. A Biotek Synergy two (Winooski, VT, United States) plate reader was used to take optical density measurements every 20 min for 13 h under aerobic environments. Alternatively, anaerobic growth measurements were observed approximately every 3 h for 48 h with a SpectraMax Plus 384 Microplate Reader (Molecular Devices, San Jose CA, United States). Anaerobic measurements required the transfer of plates from the anaerobic chamber to aerobic conditions and a subsequent transfer back to the anaerobic chamber. Anaerobic conditions in the 96-well plates were maintained by sealing the plates with an adhesive film cover.
Growth curves were modeled using R coding (RStudio, Boston, MA, United States). Under aerobic conditions the exponential growth phase of bacterial growth was modeled to evaluate viability through the onset of exponential growth (Qiu et al., 2017). Anaerobic growth lacked a noticeable lag phase, requiring an alternative model. A Gompertz model was chosen and viability was evaluated using the end of exponential growth (Zwietering et al., 1990). A detailed data analysis code is provided in the Supplementary Information along with example growth curves for 12 h exposure conditions (Supplementary Figure S1). Results of nano-PS exposure were compared to a negative control where the nano-PS exposure volume was replaced by an equivalent volume of MQ water without nano-PS, as the nano-PS stock was suspended in MQ water. A serial dilution of the negative control was performed after the exposure period for the construction of a calibration curve.
Cellular Function
The impact of nano-PS to bacterial cell function was evaluated by secretion of the external electron mediator, riboflavin. Suspended cells were diluted to an OD600 of 0.1 in media containing 1 mM iron hydroxide to a final volume of 3.2 mL. Aerobic studies were performed with LB broth, while anaerobic studies were performed with M4 broth supplement 9 mM lactate. After a 5-day exposure to nano-PS with concentrations ranging from 18.75 to 300 mg/L, riboflavin concentration in the supernatant was determined by isolating the non-cellular media by centrifugation for 5 min at 13000 rpm (Eppendorf Minispin Microcentrifuge Z606235) and quantifying using a Dionex UltiMate 3000 Series UHPLC (LPG-3400SD) with a 50 × 4.6 mm ID, Phenomenex Gemini C18 (3 μm particles) column. The mobile phase consisted of 85% Milli-Q water/15% acetonitrile v/v (Millipore Sigma; Sigma-Aldrich, Darmstadt Germany) with a flow rate of 0.5 mL/min. Riboflavin eluted after approximately 10 min and detected using fluorescence with excitation and emission wavelengths of 450 and 525 nm, respectively. A comparative analysis of riboflavin secretion after exposure to the same mass-based concentrations (18.75–300 mg/L) of TiO2 nanoparticles was performed under aerobic conditions. The cell density of the bacteria after exposure was measured through changes in the OD600 in comparison to the control after exposure and used as an estimation of viability.
Beyond quantification of the riboflavin, the anaerobic cultures described above were also used to evaluate the reduction of iron. That is, while 1 mL sample of each exposure was taken for riboflavin and optical density analysis, the remainder of the culture was used to evaluate the reduction of iron. Iron reduction was halted before analysis by the addition of 0.56 mL of 12 M HCl. Reduced iron concentrations in the supernatants of lysed cells were quantified by the ferrozine (Sigma-Aldrich, Darmstadt Germany) assay, which used UV–Visible spectroscopy to selectively monitor the formation of a Fe(II)-ferrozine complex at 562 nm (Lies et al., 2005).
Sample Preparation and Digestion for ICP-MS Analysis
Various components of the cellular culture were evaluated for the presence of nano-PS. Specifically, nano-PS was quantified in the non-cellular portions of the growth media, lightly bound extracellular polymeric substance (LB-EPS), tightly bound extracellular polymeric substance (TB-EPS), and in the cell biomass, using the Pd-label as means for detection of the nano-PS with ICP-MS analysis. Collection of the ICP-MS samples relied on the physical extraction of the TB-EPS maintained intact cells, as opposed to chemical extractions that increase the risk of lysing the cells. Initially, the non-cellular growth broth solution was collected via centrifugation at 5000 × g for 10 min. The cell-containing pellet was then washed twice with 0.9% NaCl at 5000 × g for 10 min each, with the supernatant of each NaCl wash being collected and analyzed as the LB-EPS. The cell-containing pellet was resuspended in 0.9% NaCl and incubated at 30°C for 30 min to dislodge the TB-EPS from the cells. Samples were centrifuged at 5000 × g for 10 min and the supernatant containing the TB-EPS was collected while the pellet was resuspended in M4 growth media and collected as the cell biomass.
Upon collection of the culture components, the samples were digested to dissolve and homogenize the bacteria and nano-PS. Specifically, an acid digestion was performed where 8 mL of each component of the cellular culture were mixed with 4.8 mL of a 1:10:1 (H2O2 (30%, Fisher): HNO3(69.2% Fischer): H2SO4(95%, Arcos) acid mixture. Samples were added to a glass digestion tube (N9308049, Perkin Elmer) with H2O2 and allowed to stand for 30 min to initiate the digestion of the organic matter. HNO3 was then added, followed by a 30-min standing period to allow for gas formation to slow. Finally, H2SO4 was added followed by a final 30-min standing period. H2SO4 was added slowly as it reacts with H2O2 to produce perania acid, which causes off-gassing and bubbling. The samples were then covered with aluminum foil and placed into the autoclave with the chamber temperature set at 270°C and a pressure of approximately 15 psi for 135 min. During the heating phase, the temperature fluctuated around 250°C. Two heating phases, which were deemed necessary upon visual inspection of the samples for particle presence, were performed on each sample.
A Shimadzu ICP-MS-2030 was used to detect the Pd label of the nano-PS. Pd standards (Inorganic Ventrures, Christiansburg VA, United States) were prepared at a range of 0.5–50 ppb from a 1000 ppb stock to a final volume of 10 mL. Culture components were quantified as a percent recovery of Pd by normalizing results to nano-PS control samples analyzed with ICP-MS that were of the same nano-PS concentration as the exposure. For some samples, a conversion factor of 5.1 × 10–9 ng Pd/nano-PS particle was used to quantify the number of nano-PS particles within each sample.
Statistical Analysis
Numerical representations of the data are presented as mean ± standard error. Single factor ANOVA analyses (alpha = 0.95) were used to determine the overall statistical significance of the varied concentrations for the different exposure conditions. ANOVA analyses that were statistically different (i.e., p < 0.05) were subsequently evaluated with Tukey posthoc to determine the pairwise p-values between the different exposure levels.
Results and Discussion
Viability of S. oneidensis
The impact to S. oneidensis viability was evaluated with a growth-based viability assay (Qiu et al., 2017). The viability of the suspended bacteria was determined by calibrating to a point on the bacterial growth curve, either at the onset or at the end of exponential phase for aerobic and anaerobic growth, respectively. The viability of S. oneidensis exposed to increasing doses of nano-PS for varying exposure times showed minimal changes compared to the control (Figure 2), where values were normalized to the calibration samples for the 100% viability. In aerobic conditions (Figure 2A), there are slight, but significant (p < 0.05), decreases in viability between control viability (dashed line) and the viability of S. oneidensis aerobically exposed to 75, 150, and 300 mg/L nano-PS for 8 h. However, this decrease in viability was not observed with the other aerobic exposure times, so it was concluded the impact of nano-PS on aerobically grown S. oneidensis is small. For anaerobic conditions, there were no significant changes to the cell viability as compared to the control (Figure 2B). There is larger error associated with the viabilities determined under anaerobic conditions that could be the result of small, but variable amounts of O2 entering the sealed samples over the course of the experiment. Considering both aerobic and anaerobic cultures, nano-PS caused minimal changes to cell survival under either growth environment. Previously, bacterial viability has shown a significant negative correlation with the presence of nano-PS. Both marine bacterium Halomonas alkaliphila, after 2 h of exposure (Sun et al., 2018), and fermentation bacterium Acetobacteroides hydrogenigenes, after over 3 days of exposure (Fu et al., 2018), experienced significant decreases in growth or viability upon exposure to 55 nm nano-PS. Our findings are not consistent with these observations as there is not a significant decrease in the viability observed consistently for the all exposure conditions. Due to the lack of single-species literature studies, we cannot conclude if our results vary from literature because of experimental parameters such as nanoparticle size, exposure length, or species differences.
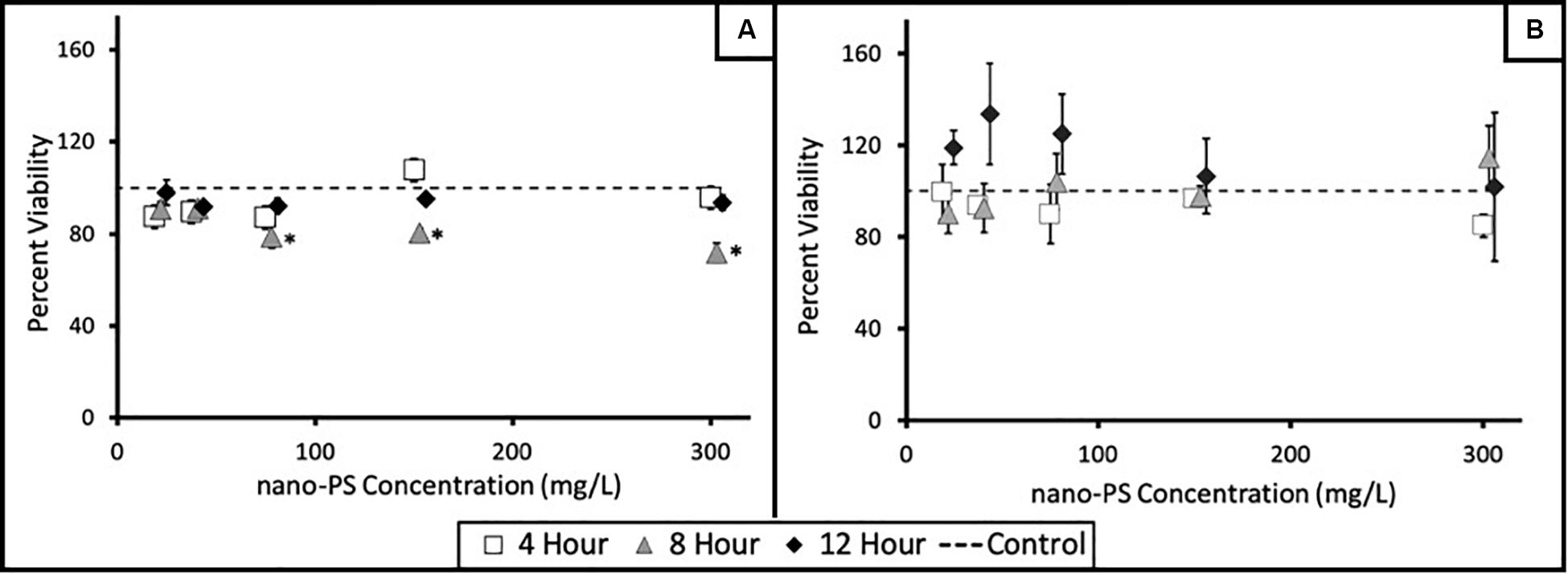
Figure 2. Viability of S. oneidensis exposed to nano-PS as measured with a growth-based viability assay. Cells were exposed to varied concentrations of nano-PS (18.75–300 mg mg/L) for varying exposure lengths (4, 8, 12 h) in (A) aerobic and (B) anaerobic conditions. Markers at each of the nano-PS exposure concentrations are slightly staggered to highlight the viabilities for exposure length. The dashed line represents the control wells, which were normalized to the 100% viable calibration wells (*p < 0.05). Biological triplicates were performed for each exposure condition, where one assay was considered a single biological replicate containing four exposure wells for each nano-PS exposure concentration. Error bars represent the SEM of biological triplicates.
Riboflavin Secretion
While the viability of S. oneidensis was minimally affected by the presence of nano-PS in our exposure conditions, we sought to also evaluate changes in cellular function. The exuded riboflavin concentration upon exposure to various concentrations of nano-PS in aerobic and anaerobic growth conditions is shown in Figure 3A. Aerobic exposure conditions (diagonal lines) showed significant increases in riboflavin secretion of cells with doses up to and including 150 mg/L nano-PS, but there was a significant (p < 0.05) decrease at the highest (300 mg/L) exposure concentration as compared to the control. It should be noted that 300 mg/L would be an unexpectedly high dose in ecologically relevant conditions. This increase in riboflavin secretion with a decrease at 300 mg/L nano-PS exposure was also observed in anaerobic conditions (solid gray bars), although the effect size was much smaller overall when compared to aerobic conditions. It is interesting to note that while the absolute value of riboflavin per cell was different, secretion trends were similar for aerobic and anaerobic conditions. S. oneidensis exudes riboflavin and other flavin mediators for a number of bacterial functions (Marsili et al., 2008; Mcanulty and Wood, 2014; Kim et al., 2016). Primarily, these flavin mediators act as part of the anaerobic respiratory pathway resulting in the reduction of a variety of metals. However, upon quantifying the bacterially reduced iron in anaerobic conditions, we observed no significant change in iron reduction in the presence of the nano-PS (Figure 3B). This signifies that there is not a correlation between riboflavin secretion and iron reduction after exposure to nano-PS. Therefore, we conclude that riboflavin is being secreted by S. oneidensis for a purpose beyond acting as an electron mediator itself. There is evidence that S. oneidensis also uses riboflavin as a signaling molecule, where riboflavin activates the cells for a number of different cellular behaviors, including repulsion of foreign bodies (Moriyama et al., 2008; Brutinel et al., 2013) or chemotaxis of other S. oneidensis bacteria (Li et al., 2012; Kim et al., 2016; Oram and Jeuken, 2019). To elucidate the purpose of the flavin response, future transcriptomics work is being pursued. In comparing aerobic and anaerobic exposures, we have shown that the observed stress response to nano-PS is consistent, no matter the culture conditions. Consequently, we hypothesize that the interactions (e.g., association) of the nano-PS with S. oneidensis would be similar between culture conditions.
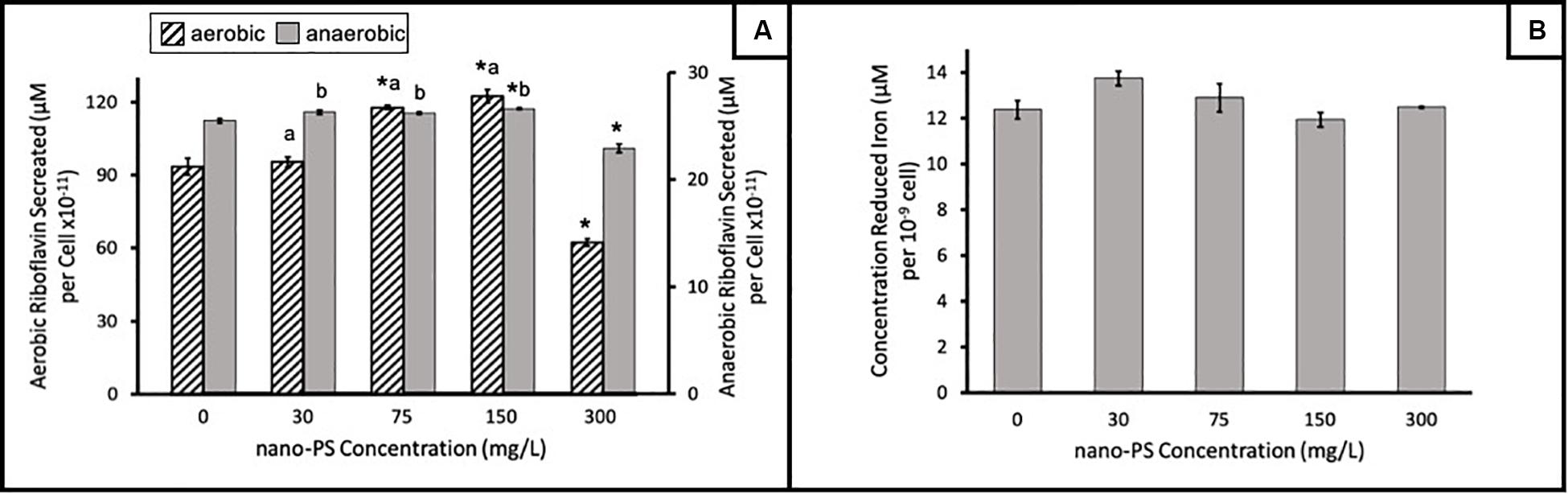
Figure 3. (A) Riboflavin secreted per cell from S. oneidensis as a function of exposure concentration of nano-PS in aerobic (diagonal lines) and anaerobic (solid gray) conditions. A significant increase (*p < 0.05) was observed as compared to the control (0 mg/L nano-PS) for aerobic 75, and 150 mg/L nano-PS and anaerobic 150 mg/mL. However, 300 mg/mL was significantly decreased riboflavin secreted per cell as compared to the control. The highest exposure concentration, 300 mg/L nano-PS, was significantly different (a or bp < 0.05) in secreted riboflavin compared to the other exposure concentration. The SEM among triplicate trials is represented by the error bars. (B) Iron reduced by S. oneidensis under anaerobic conditions exposed to varying concentrations of nano-PS does not show significant differences from the control (i.e., non-exposed) cells (single-factor ANOVA, p = 0.26). Error bars present the SEM of triplicate trials.
To confirm that these cellular responses were the result of the particulate polymer, and not simply a response to particulate matter in solution more generally, aerobic riboflavin production was quantified in the presence of TiO2 nanoparticles (Figure 4A). The optical density of the bacteria was measured to roughly estimate changes in viability at the end of exposure for all exposure doses. Like the nano-PS, TiO2 did not induce a change in optical density as compared to the control (see Supplementary Figure S5), which is interpreted as minimal changes in viability, but unlike nano-PS, there was no significant difference in the secretion of riboflavin. The difference in chemical makeup and size could potentially contribute to the observed response, but previous work with nickel manganese cobalt oxide(NMC) nanosheets (Mitchell et al., 2019) and iron oxide nanoparticles (Buchman et al., 2019b) were similar, where exposure did not result in a significant changes in the amount of riboflavin secretion from S. oneidensis. Considering these inorganic nanomaterials, with varied sizes and shapes, cause a similar secretion behavior from S. oneidensis, our results of nano-PS significantly changing the riboflavin secretion is unique. This suggests that the nano-PS material itself, and not simply bacterial interactions with particles, is important in inducing changes in cellular behavior for S. oneidensis.
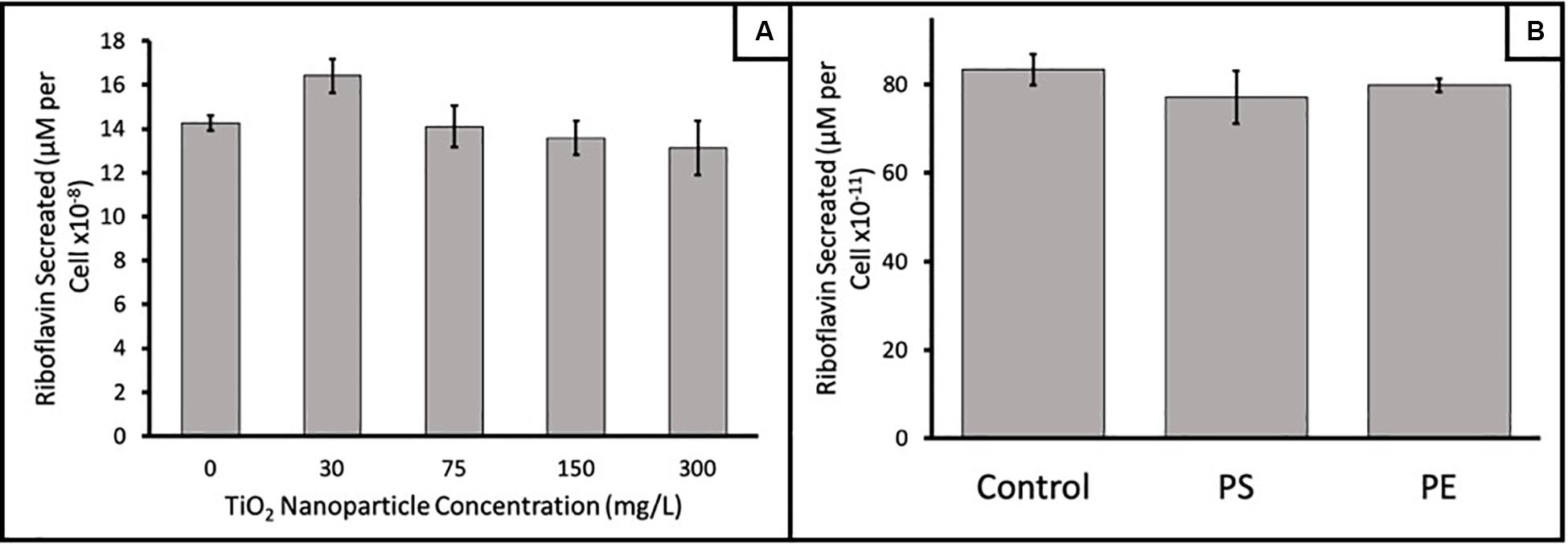
Figure 4. (A) Riboflavin secreted from S. oneidensis upon aerobic exposure to varying concentrations of 25 nm TiO2 nanoparticles were not statistically different than control cultures (single-factor ANOVA, p = 0.69). (B) Riboflavin secreted by S. oneidensis after aerobic exposure to sheets of polystyrene (PS) and polyethylene (PE) thin films. No statistical difference was observed (single-factor ANOVA, p = 0.58). Trials were performed in triplicate; error bars represent the SEM in triplicate values. Note the different order of magnitudes on the riboflavin secretion for panels (A,B).
Beyond particulate matter, no significant differences in the secretion of riboflavin was measured when bacteria were exposed to polystyrene or polyethylene thin films, indicating that the material and/or size in which the bacteria are exposed to the material impacts its response (Figure 4B). For context of the size comparison, the surface area of the thin films is 2.0 × 1014 nm2 is very similar to the nano-PS exposure concentration of 75 mg/L that has a surface area of 2.2 × 1014 nm2. This highlights the significance of the elevated riboflavin secretion in the presence of nano-PS. While the thin film polystyrene and polyethylene induced a similar result, it remains unclear if nano-polyethylene would elicit the same size-dependent response that was observed with nano-PS. Polystyrene and polyethylene are both considered to have fairly recalcitrant backbones and would be harder for microorganisms to degrade compared to polymers such as polylactic acid (Amaral-Zettler et al., 2020). Based on this similarity, we would predict that nano-polyethylene may similarly increase riboflavin secretion, although studies of natural microbial communities show that microbes respond differently to these polymers (Zettler et al., 2013; Debroas et al., 2017).
Characterizing Association of Nano-PS With S. oneidensis
To understand the association of nano-PS with respect to the bacteria in an effort to interpret the observed changes in functionality, we analyzed various components of the bacterial culture and organisms by ICP-MS. After exposure, the majority of the nano-PS remained in the non-cellular culture media under all experimental conditions (averaging 59.2 ± 15.7% of the recovered nano-PS), which was significantly greater (p < 0.05) than all the other culture components (Figure 5). The remaining fractions of nano-PS were found in the LB-EPS (7.0 ± 0.6%), TB-EPS (6.6 ± 0.6%), and directly associated with the cellular biomass (10.9 ± 1.1%) (Figure 5, inset). The mass balance for nano-PS was ranged between 66 and 106% of the total nano-PS in the exposure, which was the result of efforts to separate the aggregated nano-PS from the pelleted bacteria cell (see Supplementary Figure S6). Collectively, our results suggest that nano-PS interact both indirectly with the bacteria through entrapment in the EPS and directly with the bacterial membranes. Particles which were associated with the membrane were presumed to be strongly bound because the associated nano-PS remained attached after a series of cell washing steps. Bacteria exude EPS as a mechanism for improved chemical reactions, nutrient capture, and protection (Costa et al., 2018). This work supports previous nanoparticle toxicity studies, where nanoparticles are shown associated with the EPS, likely a protective response by the bacteria (Kang et al., 2014; Nomura et al., 2016). Interestingly, though, there is a significant increase (p < 0.05) of nano-PS associated with the bacterial biomass as was as compared to the LB-EPS or TB-EPS samples. While it would be unusual for a particle with a diameter of 160 nm to cross the bacterial membranes (Neal, 2008; Ivask et al., 2014), an association with the membrane is supported by previous work with a variety of materials and bacterial organisms (Ivask et al., 2012, 2014; Zhao et al., 2013). Specifically, for S. oneidensis, Jacobson et al. (2015) has shown that Au nanoparticles (12.8 nm) associate with the bacteria as a function of the lipopolysaccharide (LPS) within the outer cell membrane, we would hypothesize S. oneidensis would have a similar mechanism for our nano-PS results. The exposure times did not influence the percent of associated nano-PS, suggesting that the interaction with the EPS and membranes occurs within the first 4 h of exposure. Other nanoparticle association studies have suggested that this association could be even faster, within the first 60 min of exposure (Butler et al., 2014; Moore et al., 2017).
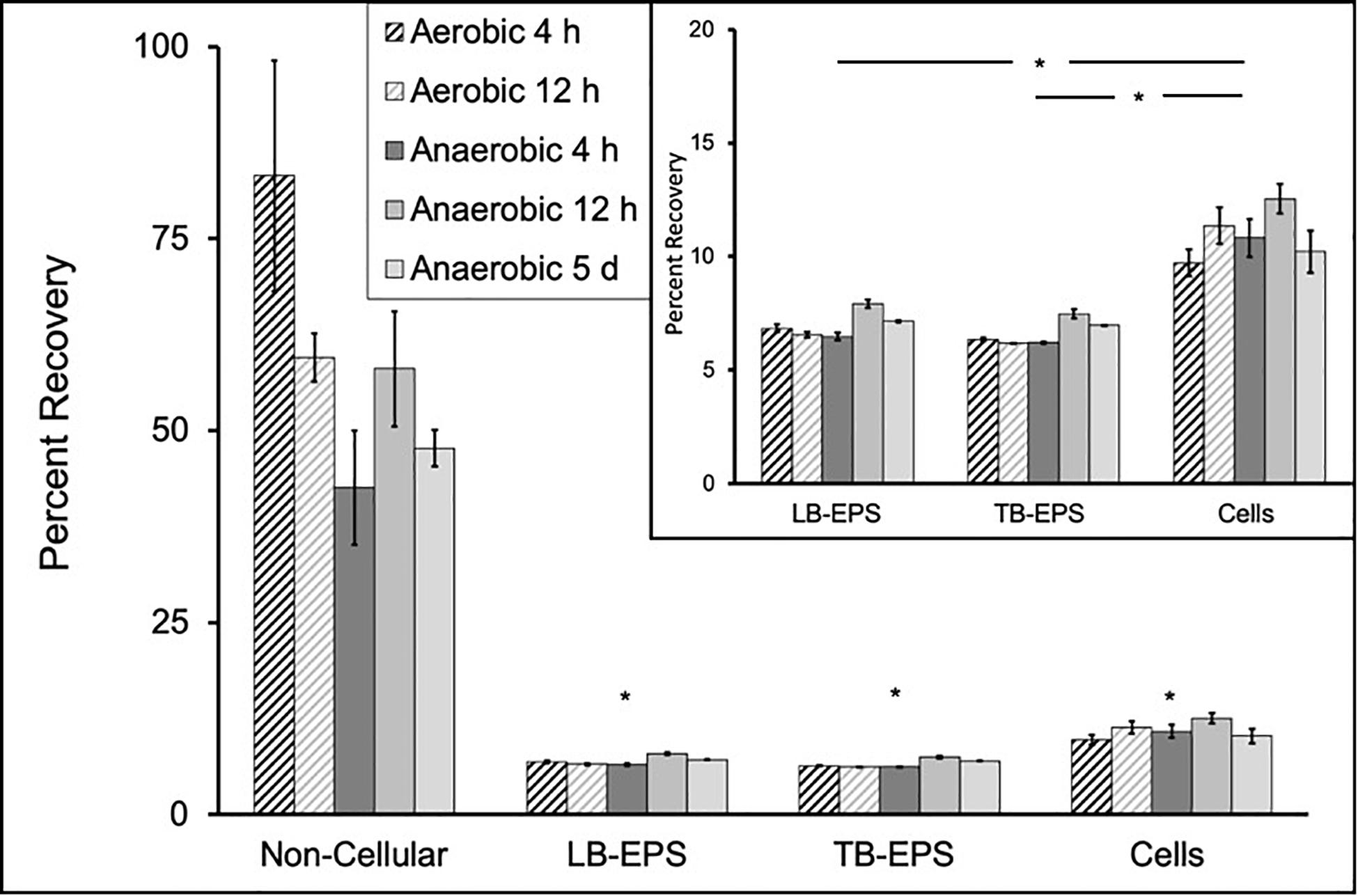
Figure 5. The distribution of nano-PS in the four components of the exposed bacterial culture after exposure to 150 mg/L nano-PS, including the non-cellular growth broth, which is significantly greater (p < 0.05) than lightly bound (LB)-EPS, tightly bound (TB)-EPS and association with cells. Inset shows statistical comparison of the nano-PS in LB-EPS and TB-EPS to the cells (*p < 0.05). Error bars represent the SEM of triplicate biological replicates.
The presence of nano-PS associated to the cell biomass indicates that a direct interaction occurs between the nano-PS and the bacteria. To determine if there is a correlation between the amount of nano-PS particles associated with the cell and the changes in riboflavin secretion, we quantified the amount of nano-PS in the cell biomass upon increased exposure doses (Figure 6). An increase in the number of nano-PS associated with the cell was observed with increased exposure concentrations. There was a significant increase in the nanoparticles per cell at 300 mg/L in comparison to the 75 and 150 mg/L exposure results, although we did not observe a doubling in membrane nano-PS concentration with a doubled exposure concentration. The 300 mg/L exposure concentration correlates with the nano-PS exposure concentration in which the riboflavin secretion had significant decreases in comparison to the control. Based on the determined low number of nano-PS/cell (12–15 nano-PS particles/cell), we do not anticipate nano-PS aggregates on the cell surface but that the particles would be individually bound onto the cell membrane. This association behavior has been observed by Jahnke et al. (2016) in which S. oneidensis was exposed to 50 and 100 nm gold nanoparticle at similar nanoparticles/cell dosages. The corresponding surface area coverage between the cells exposed to 150 mg/L and 300 mg/L nano-PS was 13.4 and 15.2%, respectively. The significant increase in the nano-PS coverage of membrane surface area paired with the observed significant decrease of riboflavin secretion, suggests that there is a concentration at which the association of nano-PS with the bacterial membrane results in alterations of the bacteria’s riboflavin secretion ability. Nanoparticles have been shown to perturb the structural integrity of the cell membranes, resulting in changes within the local lipid environment (Rossi et al., 2014; Contini et al., 2018). Membrane proteins are often sensitive to protein-lipid interactions that may be altered by changes in the morphology of the membrane caused by nanoparticle presence (Lee, 2004; Dowhan and Bogdanov, 2011; Battle et al., 2015; Muller et al., 2019). While the experimental dosages are higher than what would be expected in natural systems, the increase in the number of nano-PS particles associated with cells at higher concentrations may result in the disruption of the membrane shape impacting protein function responsible for riboflavin secretion.
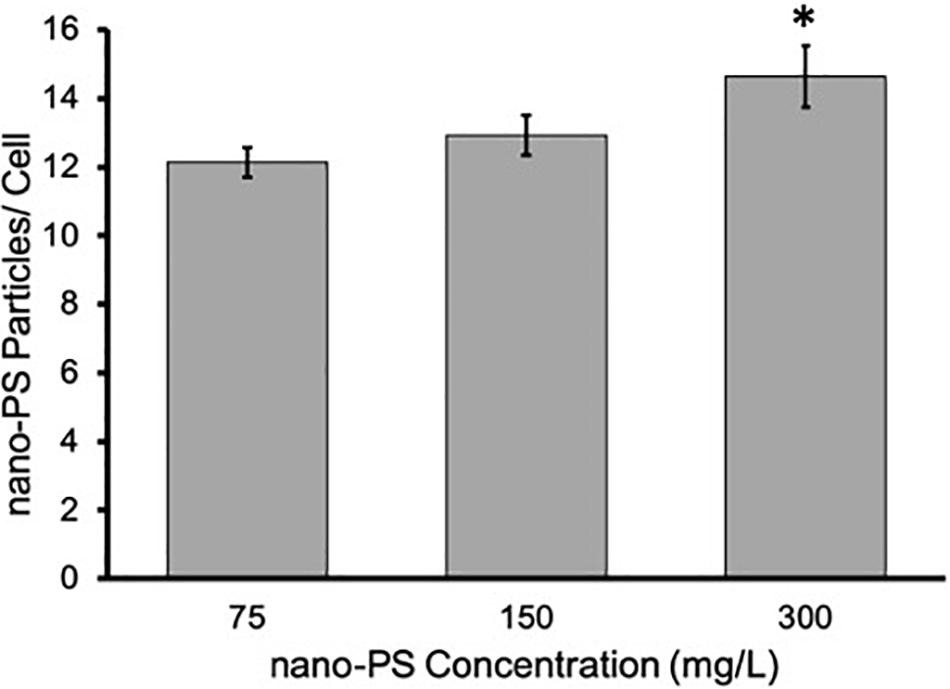
Figure 6. Comparison of the number of nano-PS normalized to the number of cells for samples exposed to 75, 150, and 300 mg/L nano-PS in anaerobic conditions for 12 h. A significant increase (∗p < 0.05) in the number of nano-PS particles/cell for samples exposed to 300 mg/L nano-PS was observed in comparison to bacteria exposed to 75 and 150 mg/L nano-PS. Error bars represent SEM of biological triplicates.
Conclusion
Overall, exposing S. oneidensis to nano-PS causes a stress response that is not related to viability. Specifically, riboflavin secretion, a critical cell function, is altered in the presence of nano-PS. This work highlights the importance of this riboflavin stress response because it is observed in both anaerobic and aerobic conditions, indicating that the secretion behavior is not a result of its metal reducing function. The observed similar aerobic and anaerobic response is interesting as it indicates that the interaction with nano-PS will be consistent in many different environmental compartments. Furthermore, we reveal that nano-PS associates indirectly with the EPS and directly with the bacteria membranes. This is the first study to characterize S. oneidensis nanoplastic or nanoparticle response in both aerobic and anaerobic cultures. By understanding the response of this adaptable single-celled organism under varied conditions, this work has contributed to a better understanding of the impact of plastic waste to the bedrock of our ecosystems.
Data Availability Statement
The datasets presented in this study can be found in online repositories. The names of the repository/repositories and accession number(s) can be found below: https://conservancy.umn.edu/handle/11299/166578.
Author Contributions
VF contributed the data collection and analysis of growth-based viability assays and ICP-MS. LF contributed the data collection and analysis of the riboflavin secretion and iron reduction assays. DM synthesized and characterized nano-PS particles. MM-J and DM contributed the conception of the work. All authors contributed to data interpretation and writing and editing of the manuscript.
Funding
Financial support of this work was provided to MM-J by the University of Minnesota and the Swiss National Science Foundation, Ambizione Grant number PZP002_168105 to DM.
Conflict of Interest
The authors declare that the research was conducted in the absence of any commercial or financial relationships that could be construed as a potential conflict of interest.
Acknowledgments
We gratefully acknowledge Jessica Sieber for use of her lab space and discussion of results, Zhihua Xu for providing the TiO2 nanoparticles, Amanda Klein for use of her lab space, and the Shama Mirza research group for performing ICP-MS measurements.
Supplementary Material
The Supplementary Material for this article can be found online at: https://www.frontiersin.org/articles/10.3389/fenvs.2020.00097/full#supplementary-material
References
Allen, S., Allen, D., Phoenix, V. R., Le Roux, G., Durántez Jiménez, P., Simonneau, A., et al. (2019). Atmospheric transport and deposition of microplastics in a remote mountain catchment. Nat. Geosci. 12, 339–344. doi: 10.1038/s41561-019-0335-5
Amaral-Zettler, L. A., Zettler, E. R., and Mincer, T. J. (2020). Ecology of the plastisphere. Nat. Rev. Microbiol. 18, 139–151. doi: 10.1038/s41579-019-0308-0
Azam, F., and Malfatti, F. (2007). Microbial structuring of marine ecosystems. Nat. Rev. Microbiol. 5, 782–791. doi: 10.1038/nrmicro1747
Battle, A. R., Ridone, P., Bavi, N., Nakayama, Y., Nikolaev, Y. A., and Martinac, B. (2015). Lipid-protein interactions: lessons learned from stress. Biochim. Biophys. Acta Biomemb. 18, 1744–1756. doi: 10.1016/j.bbamem.2015.04.012
Besseling, E., Wang, B., Lürling, M., and Koelmans, A. A. (2014). Nanoplastic affects growth of S. obliquus and reproduction of D. magna. Environ. Sci. Technol. 48, 12336–12343. doi: 10.1021/es503001d
Bhattacharya, P., Lin, S., Turner, J. P., and Ke, P. C. (2010). Physical adsorption of charged plastic nanoparticles affects algal photosynthesis. J. Phys. Chem. C 114, 16556–16561. doi: 10.1021/jp1054759
Bouwmeester, H., Hollman, P. C. H., and Peters, R. J. B. (2015). Potential health impact of environmentally released micro- and nanoplastics in the human food production chain: experiences from nanotoxicology. Environ. Sci. Technol. 49, 8932–8947. doi: 10.1021/acs.est.5b01090
Brutinel, E. D., Dean, A. M., and Gralnick, J. A. (2013). Description of a riboflavin biosynthetic gene variant prevalent in the phylum Proteobacteria. J. Bacteriol. 195:5479. doi: 10.1128/jb.00651-13
Buchman, J. T., Bennett, E. A., Wang, C., Abbaspour Tamijani, A., and Bennett, J. W. (2020). Nickel enrichment of next-generation NMC nanomaterials alters material stability, causing unexpected dissolution behavior and observed toxicity to S. oneidensis MR-1 and D. magna. Environ. Sci. Nano 7, 571–587. doi: 10.1039/c9en01074b
Buchman, J. T., Hudson-Smith, N. V., Landy, K. M., and Haynes, C. L. (2019a). Understanding nanoparticle toxicity mechanisms to inform redesign strategies to reduce environmental impact. Acc. Chem. Res. 52, 1632–1642. doi: 10.1021/acs.accounts.9b00053
Buchman, J. T., Pho, T., Rodriguez, R. S., Feng, Z. V., and Haynes, C. L. (2019b). Coating iron oxide nanoparticles with mesoporous silica reduces their interaction and impact on S. oneidensis MR-1. Chemosphere 237:124511. doi: 10.1016/j.chemosphere.2019.124511
Butler, K. S., Casey, B. J., Garborcauskas, G. V. M., Dair, B. J., and Elespuru, R. K. (2014). Assessment of titanium dioxide nanoparticle effects in bacteria: association, uptake, mutagenicity, co-mutagenicity and DNA repair inhibition. Mutat. Researchagen. 768, 14–22. doi: 10.1016/j.mrgentox.2014.04.008
Cedervall, T., Hansson, L. A., Lard, M., Frohm, B., and Linse, S. (2012). Food chain transport of nanoparticles affects behaviour and fat metabolism in fish. PLoS One 7:e32254. doi: 10.1371/journal.pone.0032254
Chen, Q., Yin, D., Jia, Y., Schiwy, S., Legradi, J., Yang, S., et al. (2017). Enhanced uptake of BPA in the presence of nanoplastics can lead to neurotoxic effects in adult zebrafish. Sci. Total Environ. 609, 1312–1321. doi: 10.1016/j.scitotenv.2017.07.144
Clement, P. L., Kuether, J. E., Borgatta, J. R., Buchman, J. T., Cahill, M. S., Qiu, T. A., et al. (2020). Cobalt release from a nanoscale multiphase lithiated cobalt phosphate dominates interaction with Shewanella oneidensis MR-1 and Bacillus subtilis SB491. Chem. Res. Toxicol. 33, 806–816. doi: 10.1021/acs.chemrestox.9b00465
Contini, C., Schneemilch, M., Gaisford, S., and Quirke, N. (2018). Nanoparticle-membrane interactions. J. Exp. Nanosci. 13, 62–81.
Costa, O. Y. A., Raaijmakers, J. M., and Kuramae, E. E. (2018). Microbial extracellular polymeric substances: ecological function and impact on soil aggregation. Front. Microbiol. 9:1636. doi: 10.3389/fmicb.2018.01636
Debroas, D., Mone, A., and Ter Halle, A. (2017). Plastics in the North Atlantic garbage patch: a boat-microbe for hitchhikers and plastic degraders. Sci. Total Environ. 59, 1222–1232. doi: 10.1016/j.scitotenv.2017.05.059
Della Torre, C., Bergami, E., Salvati, A., Faleri, C., Cirino, P., Dawson, K. A., et al. (2014). Accumulation and embryotoxicity of polystyrene nanoparticles at early stage of development of sea urchin embryos Paracentrotus lividus. Environ. Sci. Technol. 48, 12302–12311. doi: 10.1021/es502569w
Dowhan, W., and Bogdanov, M. (2011). Lipid-protein interactions as determinants of membrane protein structure and function. Biochem. Soc. Trans. 39, 767–774. doi: 10.1042/bst0390767
Driedger, A. G. J., Dürr, H. H., Mitchell, K., and Van Cappellen, P. (2015). Plastic debris in the laurentian great lakes: a review. J. Great Lakes Res. 41, 9–19. doi: 10.1016/j.jglr.2014.12.020
Fu, S.-F., Ding, J.-N., Zhang, Y., Li, Y.-F., Zhu, R., Yuan, X.-Z., et al. (2018). Exposure to polystyrene nanoplastic leads to inhibition of anaerobic digestion system. Sci. Total Environ. 625, 64–70. doi: 10.1016/j.scitotenv.2017.12.158
Gangadoo, S., Owen, S., Rajapaksha, P., Plaisted, K., Cheeseman, S., Haddara, H., et al. (2020). Nano-plastics and their analytical characterisation and fate in the marine environment: from source to sea. Sci. Total Environ. 732:138792. doi: 10.1016/j.scitotenv.2020.138792
Greven, A.-C., Merk, T., Karagöz, F., Mohr, K., Klapper, M., Jovanoviæ, B., et al. (2016). Polycarbonate and polystyrene nanoplastic particles act as stressors to the innate immune system of fathead minnow (Pimephales promelas). Environ. Toxicol. Chem. 35, 3093–3100. doi: 10.1002/etc.3501
Hau, H. H., and Gralnick, J. A. (2007). Ecology and biotechnology of the genus Shewanella. Annu. Rev. Microbiol. 61, 237–258.
Hebner, T. S., and Maurer-Jones, M. A. (2020). Characterizing microplastic size and morphology of photodegraded polymers placed in simulated moving water conditions. Environ. Sci. Process. Impacts 22, 398–407. doi: 10.1039/c9em00475k
Hendrickson, E., Minor, E. C., and Schreiner, K. (2018). Microplastic abundance and composition in western lake superior as determined via microscopy, Pyr-GC/MS, and FTIR. Environ. Sci. Technol. 52, 1787–1796. doi: 10.1021/acs.est.7b05829
Hermabessiere, L., Dehaut, A., Paul-Pont, I., Lacroix, C., Jezequel, R., Soudant, P., et al. (2017). Occurrence and effects of plastic additives on marine environments and organisms: a review. Chemosphere 182, 781–793. doi: 10.1016/j.chemosphere.2017.05.096
Imhof, H. K., Laforsch, C., Wiesheu, A. C., Schmid, J., Anger, P. M., Niessner, R., et al. (2016). Pigments and plastic in limnetic ecosystems: a qualitative and quantitative study on microparticles of different size classes. Water Res. 98, 64–74. doi: 10.1016/j.watres.2016.03.015
Ivask, A., Kurvet, I., Kasemets, K., Blinova, I., Aruoja, V., Suppi, S., et al. (2014). Size-dependent toxicity of silver nanoparticles to bacteria, yeast, algae, crustaceans and mammalian cells in vitro. PLoS One 9:e102108. doi: 10.1371/journal.pone.00102108
Ivask, A., Suarez, E., Patel, T., Boren, D., Ji, Z., and Holden, P. (2012). Genome-wide bacterial toxicity screening uncovers the mechanisms of toxicity of a cationic polystyrene nanomaterial. Environ. Sci. Technol. 46, 2398–2405. doi: 10.1021/es203087m
Jacobson, K. H., Gunsolus, I. L., Kuech, T. R., Troiano, J. M., Melby, E. S., and Lohse, S. E. (2015). Lipopolysaccharide density and structure govern the extent and distance of nanoparticle interaction with actual and model bacterial outer membranes. Environ. Sci. Technol. 49, 10642–10650. doi: 10.1021/acs.est.5b01841
Jahnke, J. P., Cornejo, J. A., Sumner, J. J., Schuler, A. J., Atanassov, P., and Ista, L. K. (2016). Conjugated gold nanoparticles as a tool for probing the bacterial cell envelope: the case of Shewanella oneidensis MR-1. Biointerphases 11:011003. doi: 10.1116/1.4939244
Kang, F., Alvarez, P. J., and Zhu, D. (2014). Microbial extracellular polymeric substances reduce Ag+ to silver nanoparticles and antagonize bactericidal activity. Environ. Sci. Technol. 48, 316–322. doi: 10.1021/es403796x
Kim, B. J., Chu, I., Jusuf, S., Kuo, T., Teravest, M. A., Angenent, L. T., et al. (2016). Oxygen tension and riboflavin gradients cooperatively regulate the migration of Shewanella oneidensis MR-1 revealed by a hydrogel-based microfluidic device. Front. Microbiol. 7:1438. doi: 10.3389/fmicb.2018.01438
Koelmans, A. A., Besseling, E., and Shim, W. J. (2015). “Nanoplastics in the aquatic environment. critical review,” in Marine Anthropogenic Litter, eds M. Bergmann, L. Gutow, and M. Klages (Cham: Springer International Publishing), 325–340. doi: 10.1007/978-3-319-16510-3_12
Lee, A. G. (2004). How lipids affect the activities of integral membrane proteins. Biochim. Biophys. Acta 1666, 62–87. doi: 10.1016/j.bbamem.2004.05.012
Li, R., Tiedje, J. M., Chiu, C., and Worden, R. M. (2012). Soluble electron shuttles can mediate energy taxis toward insoluble electron acceptors. Environ. Sci. Technol. 46, 2813–2820. doi: 10.1021/es204302w
Lies, D. P., Hernandez, M. E., Kappler, A., Mielke, R. E., Gralnick, J. A., and Newman, D. K. (2005). Shewanella Oneidensis MR-1 uses overlapping pathways for iron reduction at a distance and by direct contact under conditions relevant for biofilms. Appl. Environ. Microbiol. 71:4414. doi: 10.1128/aem.71.8.4414-4426.2005
Lindeque, P. K., Cole, M., Coppock, R. L., Lewis, C. N., Miller, R. Z., and Watts, A. J. R. (2020). Are we underestimating microplastic abundance in the marine environment? A comparison of microplastic capture with nets of different mesh-size. Environ. Pollut. 2020:114721. doi: 10.1016/j.envpol.2020.114721
Lu, Y., Zhang, Y., Deng, Y., Jiang, W., Zhao, Y., Geng, J., et al. (2016). Uptake and accumulation of polystyrene microplastics in zebrafish (Danio rerio) and toxic effects in liver. Environ. Sci. Technol. 50, 4054–4060. doi: 10.1021/acs.est.6b00183
Marsili, E., Baron, D. B., Shikhare, I. D., Coursolle, D., Gralnick, J. A., and Bond, D. R. (2008). Shewanella secretes flavins that mediate extracellular electron transfer. Proc. Natl. Acad. Sci. U.S.A. 105:3968. doi: 10.1073/pnas.0710525105
Maurer-Jones, M. A., Gunsolus, I. L., Meyer, B. M., Christenson, C. J., and Haynes, C. L. (2013a). Impact of TiO2 nanoparticles on growth, biofilm formation, and flavin secretion in Shewanella oneidensis. Analyt. Chem. 85, 5810–5818. doi: 10.1021/ac400486u
Maurer-Jones, M. A., Gunsolus, I. L., Murphy, C. J., and Haynes, C. L. (2013b). Toxicity of engineered nanoparticles in the environment. Analyt. Chem. 85, 3036–3049.
Maurer-Jones, M. A., Mousavi, M. P. S., Chen, L. D., Bühlmann, P., and Haynes, C. L. (2013c). Characterization of silver ion dissolution from silver nanoparticles using fluorous-phase ion-selective electrodes and assessment of resultant toxicity to Shewanella oneidensis. Chem. Sci. 4, 2564–2572.
Mcanulty, M. J., and Wood, T. K. (2014). YeeO from Escherichia coli exports flavins. Bioengineered 5, 386–392. doi: 10.4161/21655979.2014.969173
Mitchell, S. L., Hudson-Smith, N. V., Cahill, M. S., Reynolds, B. N., Frand, S. D., Green, C. M., et al. (2019). Chronic exposure to complex metal oxide nanoparticles elicits rapid resistance in Shewanella oneidensis MR-1. Chem. Sci. 10, 9768–9781. doi: 10.1039/c9sc01942a
Mitrano, D. M., Beltzung, A., Frehland, S., Schmiedgruber, M., Cingolani, A., and Schmidt, F. (2019). Synthesis of metal-doped nanoplastics and their utility to investigate fate and behaviour in complex environmental systems. Nat. Nanotechnol. 14, 362–368. doi: 10.1038/s41565-018-0360-3
Moore, J. D., Avellan, A., Noack, C. W., Guo, Y., Lowry, G. V., and Gregory, K. B. (2017). Time-dependent bacterial transcriptional response to CuO nanoparticles differs from that of Cu2+ and provides insights into CuO nanoparticle toxicity mechanisms. Environ. Sci. Nano 4, 2321–2335. doi: 10.1039/c7en00600d
Moriyama, Y., Hiasa, M., Matsumoto, T., and Omote, H. (2008). Multidrug and toxic compound extrusion (MATE)-type proteins as anchor transporters for the excretion of metabolic waste products and xenobiotics. Xenobiotica 38, 1107–1118. doi: 10.1080/00498250701883753
Muller, M. P., Jiang, T., Sun, C., Lihan, M., Pant, S., Mahinthichaichan, P., et al. (2019). Characterization of lipid-protein interactions and lipid-mediated modulation of membrane protein function through molecular simulation. Chem. Rev. 119, 6086–6161. doi: 10.1021/acs.chemrev.8b00608
Neal, A. L. (2008). What can be inferred from bacterium-nanoparticle interactions about the potential consequences of environmental exposure to nanoparticles? Ecotoxicology 17:362. doi: 10.1007/s10646-008-0217-x
Nomura, T., Fujisawa, E., Itoh, S., and Konishi, Y. (2016). Comparison of the cytotoxic effect of polystyrene latex nanoparticles on planktonic cells and bacterial biofilms. J. Nanopart. Res. 18:157.
Oram, J., and Jeuken, L. J. C. (2019). Tactic response of Shewanella oneidensis MR-1 toward insoluble electron acceptors. mBio 10:e002490-18.
Pitt, J. A., Kozal, J. S., Jayasundara, N., Massarsky, A., Trevisan, R., and Geitner, N. (2018). Uptake, tissue distribution, and toxicity of polystyrene nanoparticles in developing zebrafish (Danio rerio). Aquat. Toxicol. 194, 185–194. doi: 10.1016/j.aquatox.2017.11.017
Qiu, T. A., Nguyen, T. H. T., Hudson-Smith, N. V., Clement, P. L., Forester, D. C., Frew, H., et al. (2017). Growth-based bacterial viability assay for interference-free and high-throughput toxicity screening of nanomaterials. Analyt. Chem. 89, 2057–2064. doi: 10.1021/acs.analchem.6b04652
Ramanan, R., Kim, B. H., Cho, D. H., Oh, H. M., and Kim, H. S. (2016). Algae-bacteria interactions: Evolution, ecology and emerging applications. Biotechnol. Adv. 34, 14–29. doi: 10.1016/j.biotechadv.2015.12.003
Rochman, C. M., Hoh, E., Hentschel, B. T., and Kaye, S. (2012). Long-term field measurement of sorption of organic contaminants to five types of plastic pellets: implications for plastic marine debris. Environ. Sci. Technol. 47, 1646–1654.
Rossi, G., Barnoud, J., and Monticelli, L. (2014). Polystyrene nanoparticles perturb lipid membranes. J. Phys. Chem. Lett. 5, 241–246. doi: 10.1021/jz402234c
Shen, M., Zhang, Y., Zhu, Y., Song, B., Zeng, G., Hu, D., et al. (2019). Recent advances in toxicological research of nanoplastics in the environment: a review. Environ. Pollut. 252, 511–521. doi: 10.1016/j.envpol.2019.05.102
Sheng, L., and Fein, J. B. (2014). Uranium reduction by Shewanella oneidensis MR-1 as a function of nahco3 concentration: surface complexation control of reduction kinetics. Environ. Sci. Technol. 48, 3768–3775. doi: 10.1021/es5003692
Shi, L., Squier, T. C., Zachara, J. M., and Fredrickson, J. K. (2007). Respiration of metal (hydr)oxides by Shewanella and Geobacter: a key role for multihaem c-type cytochromes. Mol. Microbiol. 65, 12–20. doi: 10.1111/j.1365-2958.2007.05783.x
Sun, X., Chen, B., Li, Q., Liu, N., Xia, B., Zhu, L., et al. (2018). Toxicities of polystyrene nano- and microplastics toward marine bacterium Halomonas alkaliphila. Sci. Total Environ. 642, 1378–1385. doi: 10.1016/j.scitotenv.2018.06.141
Tanaka, K., Takada, H., Yamashita, R., Mizukawa, K., Fukuwaka, M.-A., and Watanuki, Y. (2015). Facilitated leaching of additive-derived PBDEs from plastic by seabirds’ stomach oil and accumulation in tissues. Environ. Sci. Technol. 49, 11799–11807. doi: 10.1021/acs.est.5b01376
Valls, M., and De Lorenzo, V. (2002). Exploiting the genetic and biochemical capacities of bacteria for the remediation of heavy metal pollution. FEMS Microbiol. Rev. 26, 327–338. doi: 10.1016/s0168-6445(02)00114-6
Wagner, M., Scherer, C., Alvarez-Muñoz, D., Brennholt, N., Bourrain, X., Buchinger, S., et al. (2014). Microplastics in freshwater ecosystems: what we know and what we need to know. Environ. Sci. Eur. 26:12.
Wang, J., Wu, M., Lu, G., and Si, Y. (2016). Biotransformation and biomethylation of arsenic by Shewanella oneidensis MR-1. Chemosphere 145, 329–335. doi: 10.1016/j.chemosphere.2015.11.107
Watts, A. J. R., Urbina, M. A., Goodhead, R., Moger, J., Lewis, C., and Galloway, T. S. (2016). Effect of microplastic on the gills of the shore crab Carcinus maenas. Environ. Sci. Technol. 50, 5364–5369. doi: 10.1021/acs.est.6b01187
Welden, N. A. C., and Cowie, P. R. (2016). Long-term microplastic retention causes reduced body condition in the langoustine, Nephrops norvegicus. Environ. Pollut. 218, 895–900. doi: 10.1016/j.envpol.2016.08.020
Yin, L., Chen, B., Xia, B., Shi, X., and Qu, K. (2018). Polystyrene microplastics alter the behavior, energy reserve and nutritional composition of marine jacopever (Sebastes schlegelii). J. Hazard. Mater. 360, 97–105. doi: 10.1016/j.jhazmat.2018.07.110
Zbyszewski, M., Corcoran, P. L., and Hockin, A. (2014). Comparison of the distribution and degradation of plastic debris along shorelines of the Great Lakes, North America. J. Great Lakes Res. 40, 288–299. doi: 10.1016/j.jglr.2014.02.012
Zettler, E. R., Mincer, T. J., and Amaral-Zettler, L. A. (2013). Life in the “Plastisphere”: microbial communities on plastic marine debris. Environ. Sci. Technol. 47, 7137–7146. doi: 10.1021/es401288x
Zhang, W., Xiao, B., and Fang, T. (2018). Chemical transformation of silver nanoparticles in aquatic environments: mechanism, morphology and toxicity. Chemosphere 191, 324–334. doi: 10.1016/j.chemosphere.2017.10.016
Zhao, J., Wang, Z., Dai, Y., and Xing, B. (2013). Mitigation of CuO nanoparticle-induced bacterial membrane damage by dissolved organic matter. Water Res. 47, 4169–4178. doi: 10.1016/j.watres.2012.11.058
Zhi, B., Yang, Y., Hudson-Smith, N. V., Kortshagen, U. R., and Haynes, C. L. (2019). Bacterial toxicity of germanium nanocrystals induced by doping with boron and phosphorus. ACS Appl. Nano Mater. 2, 4744–4755. doi: 10.1021/acsanm.9b00525
Keywords: nanoplastic, viability, functional response, polystyrene, association
Citation: Fringer VS, Fawcett LP, Mitrano DM and Maurer-Jones MA (2020) Impacts of Nanoplastics on the Viability and Riboflavin Secretion in the Model Bacteria Shewanella oneidensis. Front. Environ. Sci. 8:97. doi: 10.3389/fenvs.2020.00097
Received: 09 April 2020; Accepted: 09 June 2020;
Published: 30 June 2020.
Edited by:
Alexandre J. Poulain, University of Ottawa, CanadaReviewed by:
Ilaria Corsi, University of Siena, ItalyAndrew Mark Osborn, RMIT University, Australia
Copyright © 2020 Fringer, Fawcett, Mitrano and Maurer-Jones. This is an open-access article distributed under the terms of the Creative Commons Attribution License (CC BY). The use, distribution or reproduction in other forums is permitted, provided the original author(s) and the copyright owner(s) are credited and that the original publication in this journal is cited, in accordance with accepted academic practice. No use, distribution or reproduction is permitted which does not comply with these terms.
*Correspondence: Melissa A. Maurer-Jones, bWF1am9uZXNAZC51bW4uZWR1