- 1Urat Desert-Grassland Research Station, Northwest Institute of Eco-Environment and Resources, Chinese Academy of Sciences, Lanzhou, China
- 2Naiman Desertification Research Station, Northwest Institute of Eco-Environment and Resources, Chinese Academy of Sciences, Lanzhou, China
- 3Ecological Environmental Supervision and Administration Bureau of Gansu Province, Lanzhou, China
- 4Health and Environmental Science Department, Xi’an Jiaotong Liverpool University, Suzhou, China
Litter mass loss and nutrient release are key links in the material cycling and energy flowing in ecosystems and of special ecological significance in maintaining ecosystem stability, improving soil structure, and promoting vegetation restoration in the arid and semi-arid regions. Furthermore, litter mass loss could be affected by the change in precipitation patterns. However, currently, most studies on the response of litter mass loss to precipitation pattern change focus on the precipitation amount much more than the precipitation frequency. Therefore, we conducted a 3-year manipulative research in a desert steppe to assess the effects of decreasing precipitation amount and increasing precipitation interval on the litter mass loss of Stipa klemenzii and their relationships with litter chemical traits [contents of carbon (C), nitrogen (N), phosphorus (P), potassium (K), lignin and ash, C/N ratio, and lignin/N ratio] and abiotic factors (light intensity and temperature and humidity of soil and air). The results showed that (1) both treatments have negative effects on litter mass loss; (2) for abiotic factors, both treatments affected only soil moisture; for biotic factors, both treatments decreased the litter lignin contents; the increased precipitation interval treatment decreased the litter N and K contents, but increased the litter C/N ratio and lignin/N ratio; (3) the main control of litter mass loss was due to our manipulation of drought regime and its effects on the soil decomposition environment, rather than to other factors such as litter quality or light intensity; (4) compared to decreased precipitation amount, increased precipitation interval has more impact on litter mass loss, and this was caused by the increased litter C/N ratio in increased precipitation interval treatment. We speculated that increased precipitation interval was a harsher abiotic factor for the decomposer, and more research on this should be conducted in the future.
Introduction
Recently, global climate change has altered precipitation pattern and extended time intervals between precipitation events (Easterling et al., 2000; Intergovernmental Panel on Climate Change [IPCC], 2014). In arid and semi-arid regions, precipitation is the most important source of water (Easterling et al., 2000), and its spatial and temporal distribution determines plant colonization and growth, biomass production and distribution, litter mass loss, and nutrient release (Hobbie et al., 2001; Salinas et al., 2011). This, in turn, restricts the formation and development of vegetation communities and affects climate change by changing the cycle of carbon and water (Davidson and Janssens, 2006; Bonan et al., 2013). However, the phenomenon of extreme drought is frequent, represented by an increase in the interval of precipitation events or decreasing amount of precipitation (Min et al., 2011; Intergovernmental Panel on Climate Change [IPCC], 2014; Knapp et al., 2017).
Among the biogeochemical cycles affected by precipitation, litter mass loss and nutrient release are key links in the material and energy cycle within an ecosystem and play a decisive role in soil properties and plant productivity (Zhang et al., 2013). Moreover, a small change in the rate of decomposition can significantly affect soil carbon budget, soil fertility status, and land-atmosphere carbon exchange (Currie et al., 2010; Potthast et al., 2012; Wang et al., 2014). Previous studies showed that more than 90% of nitrogen and phosphorus required for plant growth were derived from the nutrient release during litter decomposition (Chapin et al., 2002), indicating that litter decomposition is important for maintaining ecosystem nutrient balance (Aerts et al., 2003). Compared with other ecosystems, the soils in drylands are very poor in organic matter, and the element cycling and energy flow are slow (Poulter et al., 2014). Thus, plant biomass product is strongly dependent on leaf litter decomposition (Killingbeck, 1996). Therefore, in the arid and semi-arid regions, there is a special ecological significance of litter decomposition in maintaining ecosystem stability, improving soil structure, and promoting vegetation restoration (Cornwell et al., 2008).
Litter decomposition is a complex process, which is controlled by both biotic and abiotic factors (Berg and McClaugherty, 2003; Jacotot et al., 2019). Among abiotic factors, precipitation is the most important factor affecting litter decomposition and nutrient release (Pucheta et al., 2006; Brandt et al., 2007). First, precipitation can directly accelerate the decomposition of litter by nutrient leaching (Clein and Schimel, 1994; Franklin et al., 2020). Second, precipitation can also affect decomposition and nutrient release from litter by affecting the activities of soil microbes and soil animals (Berg, 1986; Clein and Schimel, 1994). Third, precipitation can indirectly affect the rate of decomposition by changing the chemical composition of litter (Pastor and Post, 1988; Austin and Vitousek, 2000). Because rainfall and high temperatures are mostly synchronous in arid and semi-arid regions, this is conducive to higher activity of soil microbes, thus accelerating the decomposition of litter (Wang et al., 2013). However, there are significant variations in the availability of water in reality, which in turn makes the biotic and abiotic functions significantly different, leading to differences in the effects of precipitation on litter decomposition (Austin et al., 2004). Some researchers have stated that drought can reduce litter decomposition rate in arid and semi-arid ecosystems. For instance, Whitford et al. (1995) conducted water removal and addition experiments in the Chihuahuan Desert. They found that the decomposition of litter had different responses to dry and wet treatments and that only the water removal treatment reduced the decomposition rate of litter. In the Patagonian steppe, reducing precipitation by 30% in the rainless season significantly reduced the decomposition rate of Stipa speciosa litter (Yahdjian et al., 2006). However, other studies have suggested that reducing precipitation had little effect on the decomposition of litter in arid and semi-arid regions, because microorganisms in these areas can adjust their own C/N to ensure the decomposition of litter (Parton et al., 2007).
The effect of precipitation on the decomposition of litter can also vary as a result of changes in the amount of annual precipitation, but observations on this aspect are not consistent either (McCulley et al., 2005). Some studies have found that the decomposition rate of litter is positively correlated with precipitation in areas with high precipitation, but in areas with annual precipitation below 300 mm, the decomposition rate is not related to actual precipitation (Austin and Vitousek, 2000; Epstein et al., 2002). Other studies have pointed that when the precipitation is less than 100 mm (the soil is in extreme drought for most of the time) the decomposition of litter is basically controlled by precipitation (Jacobson and Jacobson, 1998). Finally, when precipitation is larger than 200 mm, the soil water content can sustain substantial soil microbial activity, so that the influence of pulsed precipitation on the decomposition of litter is reduced (Xu and Hirata, 2005). Furthermore, most studies on the response of litter mass loss and nutrient release to precipitation changes focus on the change in the net amount of precipitation, but fewer consider changes of precipitation frequency (Beier et al., 2012).
Thus, climate change plays an important role in controlling decomposition of litter (Aerts, 1997). Compared with other factors, precipitation has a great spatial and temporal variability in arid and semi-arid areas. This makes it complex to predict climate change effects on litter decomposition in arid and semi-arid regions. Therefore, we manipulated extreme drought conditions (decreased precipitation amount and increased precipitation interval) in an arid ecosystem for more than 3 years to evaluate the impacts of climate change on litter decomposition. We hypothesized that (1) leaf litter that under extreme drought conditions will decompose slower than litter under natural condition due to precipitation is conductive to litter mass loss by increasing the soil moisture (Clein and Schimel, 1994); (2) compared to the decrease in total precipitation amount, increase in precipitation intervals will have more negative impact on the litter mass loss due to the latter being harsher on the living conditions of the decomposer (Allison et al., 2013).
Materials and Methods
Experimental Site and Materials
The study was conducted on the Urat Desert-Grassland Research Station of the Chinese Academy of Sciences, a 340-ha fenced desert steppe station located in Urat Houqi County (106°58′ E, 41°25′ N, altitude ∼1,520 m), the western part of Inner Mongolia, China, a transitional area from grassland to desert (Figure 1). The average annual air temperature is 3.7–4.6°C. The annual mean sunshine duration is 3,110–3,300 h, and the frost-free period is ∼126 days. The annual precipitation is 100–150 mm, whereas the annual evapotranspiration is 3,032–3,179 mm, and the wetting coefficient is from 0.15 to 0.30 (Qu et al., 2014).
The vegetation is dominated by Stipa klemenzii, a perennial gramineous plant, which is mainly distributed in northern central Asia and represents the main vegetation type of the desert steppe. Other species in the desert steppe include Achnatherum splendens, Peganum harmala, Salsola collina, Allium mongolicum, Allium polyrhizum, Corispermum macrocarpum, Bassia dasyphylla, Setaria viridis, Reaumuria songarica, and Oxytropis aciphylla.
We selected leaf litter (as it accounts for > 60% of total litter, and its decomposition rate is faster than that of other litters, Huysen et al., 2013) of the dominated species S. klemenzii as the experimental litter material. Stipa klemenzii is a short herb, usually 1.5–13.5 cm high. It is drought tolerant and plays a vital role in environmental protection, and the conservation of water and soil in the dessert steppe (Qu et al., 2019).
Experimental Platform
The Urat Desert-Grassland Research Station is equipped with an experimental platform for studying the response of ecosystems to extreme drought (Figure 2). It is a standard experiment setup in six major grassland ecosystems in the United States since 2012. Since 2014, China and the United States have conducted a network research to study the response of grassland ecosystems to the extreme drought.
The experimental platform uses a canopy to reduce or exclude the rainwater. This type of shelter has been used by many other ecological peers (Knapp et al., 2017) and is technically feasible. There is a shelter area of 36 m2, and the top of the shelter is arched to facilitate the flow of the intercepted water and reduce the damage of the wind. The shelter is made of transparent acrylic plastic plate with easy disassembly, high light transmission, and high UV penetration, which completely shields the precipitation and avoids the influence of light.
There are 12 sets of experimental facilities in Urat Desert-Grassland Research station, and there are also six natural precipitation control plots with the same area. Therefore, there are 18 plots in total and each covering 36 m2. Every plot is separated by 5 m; the surrounding area is deeply digging 1–1.5 m and separated by a 6-m-long plastic baffle to prevent mutual interference of moisture lateral movement. The 4 × 4-m2 area in the center of each plot is the experimental area, and the surrounding 1 m is a buffer zone to facilitate sample collection during the experiment and reduce the marginal effect. The experimental platform has good controllability and can conveniently carry out the precipitation interval processing of different durations.
Experimental Design
Based on the same experimental approach to that setup in the United States and the historical precipitation situation, we set our precipitation modification experiment with three treatments: a natural background precipitation control, and two extreme drought treatments: (1) decreased precipitation amount by two-thirds of that in the growing season (May 1–August 31) and (2) increased precipitation interval in the early stage of growth season (May 1–June 30). There were six repetitions of each treatment; thus, there are 18 experimental plots in total.
The litter bag method (Knacker et al., 2003; Githaiga et al., 2019) was used for estimating litter decomposition dynamics. We used a litter bag size of 15 × 10 cm and a mesh size of 1 × 1 mm. The experiment was conducted for 3 years, and the field collection of leaf litter was completed in the autumn of 2015. All the collected litter was cleaned and dried at 65°C until constant weight. Based on previous experience in the study area (Qu et al., 2019), we placed 10 g leaf litter of S. klemenzii in every nylon bag. Litter bags were equipped with zippers to prevent the litter from leaking out and then were placed into the precipitation interval experimental platform on April 1, 2016. According to a previous study, the decomposition of litter is faster in the initial stage and slower in the later stage (Qu et al., 2010); thus, samples were taken every 2 months in the first year (June, August, October, and December in 2016) and then every 3 months in the rest of the experimental period (March, June, September, and December in 2017 and 2018), and six litter bags were sampled each time in each plot. Therefore, there are 12 sampling times in total. The total of litter bags placed was 3 (treatments) × 6 (plots) × 6 (replicates) × 12 (sampling times) = 1,296; all litter bags are placed on the ground surface.
Data Collection
Abiotic Factors
The soil surface temperature, soil humidity, and light intensity were measured every 10 days at the same time (11:00 AM) during the experimental period and determined using geothermometers (HH82; Exphil Calibration Labs, Bohemia, NY, United States) and hygrometers (TRIME-FM;, IMKO, GmbH, Ettlingen, Germany) and illuminometer (Testo 545, Schwarzwald, Germany), respectively. The data of precipitation (Supplementary Table S1), air temperature, and air humidity were provided by the standard weather station in the experimental field. The differences in air temperature (Supplementary Figure S1A), air humidity (Supplementary Figure S1B), soil temperature (Supplementary Figure S1C), and light intensity (Supplementary Figure S2) among control and different treatments were not significant (P > 0.05). However, the soil humidity of control, decreased precipitation amount treatment, and increased precipitation interval treatment showed different trends during the experimental period (Supplementary Figure S1D).
Mass Loss
The sampled litter bags were carefully washed with clean water every time to remove the mud and debris and then dried at 65°C to constant weight to calculate the mass loss. The litter mass was determined as ash free dry mass (AFDM) after the combustion of samples at 550°C in a muffle furnace for 4 h, and all litter chemical traits were calculated on the basis of AFDM (Duan et al., 2013).
The leaf litter mass loss was measured by Equation (1):
where W0 is the initial litter weight (10 g), and W1 refers to the measured weight after each sampling.
Leaf Litter Traits
The leaf litter traits were measured three times during the experiment period (initial, after 1 year of decomposition, and by the end of the experiment, respectively). The total carbon content (C) and total nitrogen content (N) of leaf litters were measured by using an elemental analyzer (vario MACRO cube; Elementar, Langenselbol, Germany). The phosphorus (P) and potassium (K) contents were determined with an inductively coupled plasma emission spectrometer after digestion of samples in concentrated nitric acid. The lignin content was determined by gravimetry using hot sulfuric acid digestion (Flora et al., 1996). The ash content was determined by a muffle furnace with litter samples burning at 550°C to constant weight.
Statistical Analysis
Data were examined to determine if they satisfied the assumptions of parametric statistics. SPSS version 20.0 (SPSS Inc., Chicago, IL, United States) was used to perform analysis of variance (ANOVA). If the data did not meet the prerequisites for ANOVA, conversion of logarithmic was conducted. We used two approaches to analyze our mass loss data. First, to test whether the drought treatments affected mass loss differently, we conducted a repeated-measurement ANOVA. Second, to test the importance of the different factors on mass loss (e.g., factors related to our treatments such as soil moisture, see below), we used a model selection approach. We analyzed controls of litter mass loss using mixed linear effect models to test out hypotheses whether the main control on mass loss was due either to our treatment manipulation (and variables related to it, mainly soil moisture) or to variables partially related to the treatments (litter quality, represented by the lignin/N ratio), or variables not related to the experimental manipulation (represented by light intensity). We built models that included only the effect of either our factors or litter quality or light intensity at once, and a full model. We compared the plausibility of each model by the Akaike’s information criterion (AIC). Given that our data acquisition for litter chemistry variables was limited to three particular sampling dates, we used environmental and litter-bag mass data close to those dates for our analysis of controls on decomposition. Additionally, because some variables covary with time for these sampling dates on a “non-causal basis” (see below), we included sampling time as a factor in our analysis to avoid spurious results on the effects on those variables and mass loss. For instance, both litter mass and light intensity decreased monotonically with time, but light did it only as a result of our particular selection of sampling dates (two spring dates and a winter sampling date with much lower intensity values). For all mixed models, the drought treatments were the between-subjects factor; time of sampling was the repeated variable (fix effects), and plot was treated as the random factor (within subjects). Other covariates were used as fixed factors in the model selection approach. Post hoc Tukey test was performed after the ANOVA to differentiate among drought treatments if applicable. All data were transformed. These analyses were carried out with R using the nlme library (Pinheiro et al., 2013). All statistical tests were conducted at a 5% significance level.
Results
Mass Loss of Leaf Litter
The mass loss of S. klemenzii leaf litter in control was significantly more than that in increased precipitation interval treatment for every sampling (P < 0.05), and the difference with decreased precipitation amount treatment was not significant in the initial stage of experiment (P > 0.05), whereas after the sixth sampling, the litter in control was decomposed significantly faster than that in increased precipitation interval treatment (P < 0.05). The difference of mass loss between the litter in decreased precipitation amount treatment and in increased precipitation interval treatment was not significant in the first sampling (P > 0.05); with the experiment continued, the litter in decreased precipitation amount treatment was decomposed significantly faster than that in increased precipitation interval treatment (P < 0.05), whereas their differences were not significant in the seventh, eighth, and ninth sampling (P > 0.05), however, the litter in decreased precipitation amount treatment was decomposed significantly faster than that in increased precipitation interval treatment again since the 10th sampling to the end of the experiment (P < 0.05). The mass losses of S. klemenzii litter after 3 years were 21.0% (control) > 18.1% (decreased precipitation amount treatment) > 14.0% (increased precipitation interval treatment) (Figure 3).
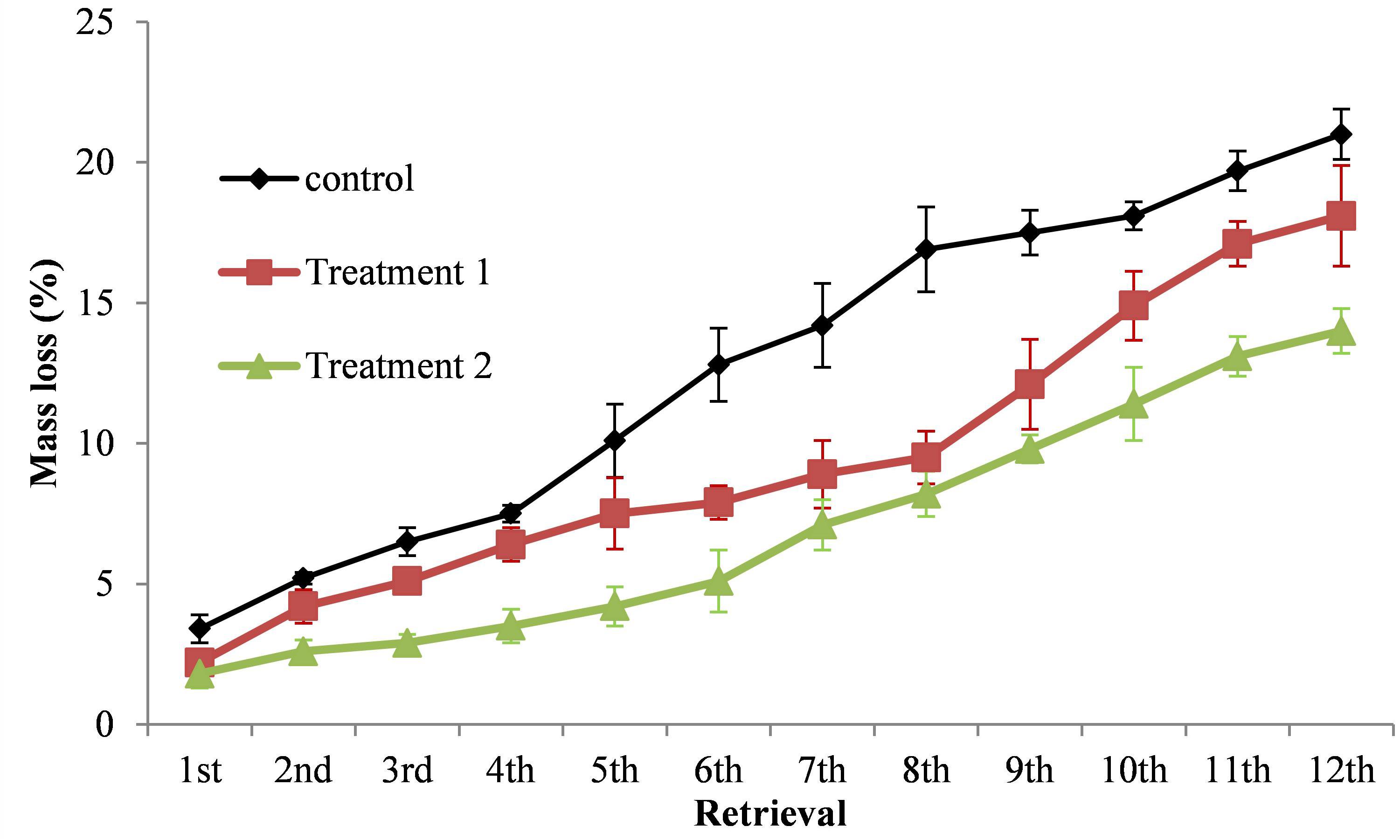
Figure 3. Comparative leaf litter mass loss of different extreme drought treatments at different retrieval times. Bar heights are means ± 1 SE for the error bars. Treatment 1, decreased precipitation amount by 2/3 in the growth season (May 1–August 31); Treatment 2, increased precipitation interval in the early stage of growth season (May 1–June 30); control, natural precipitation.
Changes of Leaf Litter Traits With Decomposition
The initial leaf litter C content of the S. klemenzii was between 462.72 and 465.47 g/kg. After 1 year of the experiment, litter C contents of different treatments were between 478.78 and 502.23 g/kg, and the differences were not significant among treatments or with the initial (P > 0.05). There was no obvious change of the leaf litter C contents of different treatments to the end of the experiment, and the differences among the treatments and in different periods were not significant (P > 0.05) (Figure 4A).
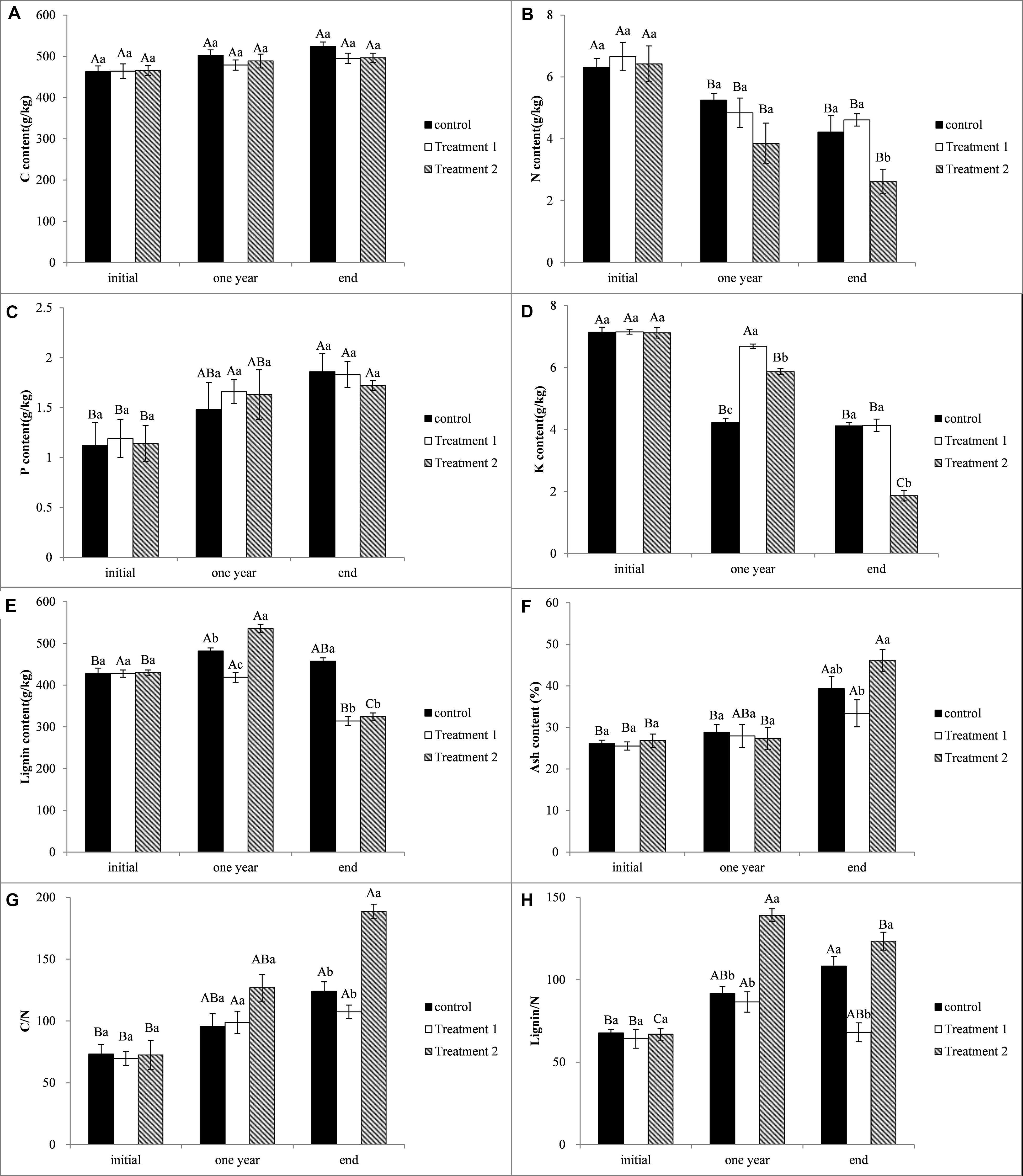
Figure 4. Initial traits of Stipa klemenzii leaf litter. (A) C content; (B) N content; (C) P content; (D) K content; (E) Lignin content; (F) Ash content; (G) C/N ratio; (H) Lignin/N ratio. Bar heights (means) ± error bars (1 SE). Mean values with different lowercase letters are significantly different among the different treatments at P < 0.05; mean values with different uppercase letters are significantly different among the different retrieval times at P < 0.05. Treatment 1, decreased precipitation amount by 2/3 in the growth season (May 1–August 31); Treatment 2, increased precipitation interval in the early stage of growth season (May 1–June 30); control, natural precipitation.
The initial leaf litter N content was between 6.31 and 6.66 g/kg. After 1 year of the experiment, the N contents of all the control, decreased precipitation amount treatment, and increased precipitation interval treatment were decreased significantly (P < 0.05), whereas the differences among different treatments were not significant (P > 0.05). By the end of the experiment, the differences of the N contents compared with those in the first year of the experiment were not significant (P > 0.05), whereas the N content of increased precipitation interval treatment was significantly lower than that of the control and the decreased precipitation amount treatment (P < 0.05) (Figure 4B).
The initial leaf litter P content was between 1.12 and 1.19 g/kg. And not similar to the C and N contents, the P content was increased with the experiment time, and by the end of the experiment, the P contents in the control, decreased precipitation amount treatment, and increased precipitation interval treatment were significantly higher than that in the initial, respectively (P < 0.05). However, the differences of P contents among the control, decreased precipitation amount treatment, and increased precipitation interval treatment were not significant during the whole experiment (P > 0.05) (Figure 4C).
The initial leaf litter K content was between 7.12 and 7.15 g/kg. After 1 year of the experiment, the K content in decreased precipitation amount treatment was 6.69 g/kg, significantly higher than that in control and increased precipitation interval treatment, and the K content in increased precipitation interval treatment was significantly higher than that in decreased precipitation amount treatment (P < 0.05). By the end of the experiment, the K content in increased precipitation interval treatment was decreased obviously and significantly lower than that in the control and decreased precipitation amount treatment (P < 0.05), the differences between control and decreased precipitation amount treatment were not significant (P > 0.05). The initial K content of the control was significantly higher than the contents after 1 year and by the end of the experiment (P < 0.05), and the difference of the K contents between that after 1 year and by the end of the experiment was not significant (P > 0.05) (Figure 4D).
The initial leaf litter lignin content was between 427.45 and 429.95 g/kg. After 1 year of the experiment, the lignin content in the control and increased precipitation interval treatment was increased significantly to 481.84 and 535.73 g/kg, respectively, whereas the lignin content in decreased precipitation amount treatment was decreased to 418.78 g/kg. Thus, the lignin contents in control and increased precipitation interval treatment were significantly higher than that in decreased precipitation amount treatment (P < 0.05). By the end of the experiment, the lignin contents in decreased precipitation amount treatment and increased precipitation interval treatment were decreased significantly to 314.24 and 324.61 g/kg, respectively, significantly lower than that in the control (457.22 g/kg) (P < 0.05). The lignin content after 1 year of the experiment in increased precipitation interval treatment was significant higher than that in the initial and by the end of the experiment, and the lignin content by the end of the experiment in decreased precipitation amount treatment was significantly lower than that in control and increased precipitation interval treatment (P < 0.05) (Figure 4E).
The initial leaf litter ash content was between 25.53 and 26.82 g/kg. After 1 year of the experiment, there was no obvious change of the ash contents in the control and the two treatments, and the differences were not significant (P > 0.05). By the end of the experiment, the ash contents of control and increased precipitation interval treatment were increased significantly, and the ash content in decreased precipitation amount treatment was significantly lower than that in increased precipitation interval treatment (P < 0.05) (Figure 4F).
The initial C/N of the S. klemenzii leaf litter was between 69.70 and 73.33. With the experiment continued, the C/N showed an increased trend, and all the C/N ratios in the control and the two treatments by the end of the experiment were significantly higher than that in the initial. And the C/N in the increased precipitation interval treatment was 188.72 by the end of the experiment, significantly higher than that in the control (124.11) and the decreased precipitation amount treatment (107.39, P < 0.05) (Figure 4G).
The initial lignin/N ratio of the S. klemenzii leaf litter was between 64.18 and 67.78. After 1 year of the experiment, all the lignin/N ratios in the control and the two treatments were increased, and the lignin/N in the increased precipitation interval treatment was significantly higher than that in control and decreased precipitation amount treatment (P < 0.05). By the end of the experiment, the lignin/N ratios in decreased precipitation amount treatment and increased precipitation interval treatment were decreased, whereas that in the control increased, and the lignin/N ratio in the decreased precipitation amount treatment was 68.16, significantly lower than that in the control (108.35) and increased precipitation interval treatment (123.43) (P < 0.05) (Figure 4H).
Effects of Treatments on Litter Mass Loss, Litter Traits, and Abiotic Factors
The repeated-measurement ANOVA was performed to test the significance of the extreme drought treatments, sampling times, and their interactions on litter mass losses. Results showed that the litter mass losses were affected by the extreme drought treatments (D), sampling times (T), and the T × D interaction. The litter C, N, K, and lignin contents; C/N ratio; and lignin/N ratio were related to sampling times, drought treatments, and the T × D interaction. The litter P contents were affected only by sampling times (Table 1).
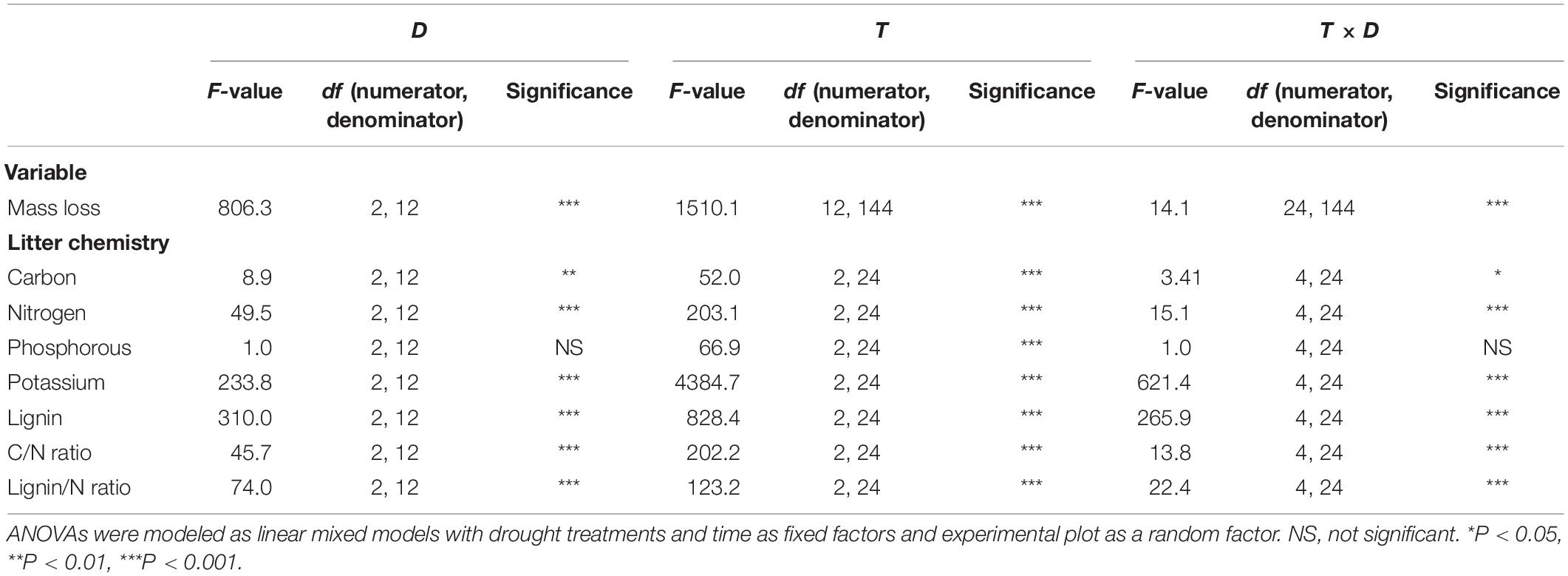
Table 1. Results of the repeated-measurement ANOVAs on the response of variables to the imposed drought treatments (D), time of sampling (T), and their interaction (T × D).
Our linear mixed-model analysis showed that our treatment and its effects on soil environment were the main factor explaining variation on litter mass. In this way, the models with either only our rainfall treatments or treatments plus soil moisture showed that our manipulation was highly significant on mass litter, and these models had the lowest AICs [-224.2 with 11 degrees of freedom (df), and -222.6 with 12 df, respectively]. In contrast, models including either only lignin/N ratio or light intensity had the lowest AICs (-194.4 with 6 df and -190.8 with 6 df), and the effect litter mass was significant only for the model including litter chemistry, not for that with light intensity.
Discussion
Many studies evaluating the impact of precipitation manipulation on litter decomposition in terrestrial ecosystems have been conducted (Chapin et al., 2002; Aerts et al., 2003; Currie et al., 2010; Zhang et al., 2013). However, the main drivers of litter mass loss in the extreme drought condition are yet not fully understood, even though drylands accounted for 41% of the global land area (Poulter et al., 2014). In our study, we conducted a simulation test in order to answer the question: as two of the most common phenomena in precipitation patterns under global climate change, is increased precipitation interval equivalent to decreased precipitation amount at litter mass loss?
The first hypothesis was supported by our findings, leaf litter under extreme drought conditions decomposed slower than litter under natural condition and showed decreased precipitation amount treatment < increased precipitation interval treatment < control after 3 years, and their differences in most samples (especially the later samples) are significant (Figure 3). The analysis also indicated that the main effect on litter mass loss is related to our treatments (and their effects on soil moisture are most important) and secondarily to litter chemistry and almost nothing to light intensity, suggesting that precipitation is conductive to litter mass loss. This is in line with many studies dealing with litter decomposition that reported significant reductions in litter mass loss with precipitation reduction (Sardans et al., 2008; Araujo and Austin, 2015; Bastida et al., 2017). And this negative impact was directly attributed to the decreased biomass and activity of soil microbial communities caused by decreased precipitation and the subsequent reduction in soil and litter moisture under drought conditions, which were important to litter mass loss (Baldrian et al., 2013; Maynard et al., 2018).
The second hypothesis was also supported by our study; compared to decreased precipitation amount, increased precipitation interval has more impact on the litter mass loss; the former decomposed 4.1% more than did the latter after 3 years, and the difference was significant (Figure 3). This may be because the infrequent rainfall events induce lower litter moisture compared to smaller but more frequent ones (Jolya et al., 2019), and litter moisture is a very important factor as we illustrated above (Baldrian et al., 2013). We also found that the C/N ratio was increased in increased precipitation interval treatment, but it was not found in decreased precipitation amount treatment (Figure 4G). This is in agreement with previous research that reported litter mass loss showed a negative relationship with the C/N ratio (Zhou et al., 2015), because microbes transitioned from C to N limitation when the C/N ratio of plants increased (Averill and Waring, 2018). These results indicated that increased precipitation interval was harsher on the living conditions of the decomposer than decreased precipitation amount (Allison et al., 2013), because the altered abiotic environment would select for more drought-resistant microbial communities (Yuste et al., 2011). It also should be noticed that the lignin contents were lower in both extreme drought treatments than that in control (Figure 4E); this is inconsistent with a previous study that found litter with lower lignin content will decompose faster because lignin is difficult to decompose (Zhou et al., 2012; Qu et al., 2019). However, similar with Prieto et al. (2019), the potential positive effect of lower lignin content in our study may be overwhelmed by climate-induced reductions in litter decomposition in the extreme drought treatments.
Conclusion
We conclude that the main control of litter mass loss was due to our manipulation of drought regime and its effects on the soil decomposition environment, rather than to other factors such as litter quality or light intensity. We found that as two of the most common phenomena in precipitation patterns under global climate change, both increased precipitation interval and decreased precipitation amount have negative effects on litter mass loss in the desert steppe because of the reduced soil and litter humidity. However, the effects of the two treatments on litter mass loss are different, compared to decreased precipitation amount, the litter mass loss under increased precipitation interval is less significant. Furthermore, we found that the C/N ratio of litters in the increased precipitation interval treatment was significantly higher than that in the control, but this is not observed in the decreased precipitation amount treatment. This indicated that increased precipitation interval can prevent litter mass loss by increasing the C/N ratio; therefore, it is harsher on the living conditions of the decomposer than decreased precipitation amount treatment. Based on this, we proposed that more studies should focus on the decomposer change in relation to litter mass loss in extreme drought conditions in the future.
Data Availability Statement
All datasets generated for this study are included in the article/Supplementary Material.
Author Contributions
HQ: conceptualization, methodology, and writing – original draft preparation. XZ: conceptualization and methodology. JL: visualization and investigation. XT: supervision. XW and EM-R: software, reviewing, and editing. All authors contributed to the article and approved the submitted version.
Funding
This work was supported by the National Natural Science Foundation of China (41877540), the Visiting Scholar Research Program of China Scholarship Council (201804910131), the Key Research and Development Plan of Ning Xia Province, China (2020BBF02003), the National Key Research and Development Plan of China (2016YFC0500506), and the Second Tibetan Plateau Scientific Expedition and Research program (2019QZKK0305).
Conflict of Interest
The authors declare that the research was conducted in the absence of any commercial or financial relationships that could be construed as a potential conflict of interest.
Acknowledgments
We thank all members of Naiman Desertification Research Station and Urat Desert-Grassland Research Station, Chinese Academy of Sciences (CAS), for their help in field and laboratory work.
Supplementary Material
The Supplementary Material for this article can be found online at: https://www.frontiersin.org/articles/10.3389/fenvs.2020.00088/full#supplementary-material
FIGURE S1 | Air temperature (A), air humidity (B) and soil temperature (C), soil humidity (D) of different extreme drought treatments during the experimental period. Values were assigned as mean ± SE. The soil surface temperature and soil humidity were measured every 10 days at the same time (11:00 AM) during the experimental period. The data of air temperature and air humidity were provided by the standard weather station in the experimental field. Jul-16: July 2016. Treatment 1, decreased precipitation amount by 2/3 in the growth season (May 1–August 31); Treatment 2, increased precipitation interval in the early stage of growth season (May 1–June 30); control, natural precipitation.
FIGURE S2 | Light intensity of different extreme drought treatments during the experimental period. Values were assigned as mean ± SE. The data were measured every 10 days at the same time (11:00 AM) during the experimental period. Jul-16: July 2016. Treatment 1: decreased precipitation amount by 2/3 in the growth season (May 1–August 31); Treatment 2: increased precipitation interval in the early stage of growth season (May 1–June 30); control, natural precipitation.
References
Aerts, R. (1997). Climate, leaf litter chemistry and leaf litter decomposition in terrestrial ecosystems: a triangular relationship. Oikos 79, 439–449. doi: 10.2307/3546886
Aerts, R., Caluwede, H., and Beltman, B. (2003). Plant community mediated vs. nutritional controls on litter decomposition rates in grasslands. Ecology 84, 3198–3208. doi: 10.1890/02-0712
Allison, S. D., Lu, Y., Weihe, C., Goulden, M. L., Martiny, A. C., Treseder, K. K., et al. (2013). Microbial abundance and composition influence litter decomposition response to environmental change. Ecology 94, 714–725. doi: 10.1890/12-1243.1
Araujo, P. I., and Austin, A. T. (2015). A shady business: pine afforestation alters the primary controls on litter decomposition along a precipitation gradient in Patagonia, Argentina. J. Ecol. 103, 1408–1420. doi: 10.1111/1365-2745.12433
Austin, A. T., and Vitousek, P. M. (2000). Precipitation, decomposition and litter decomposability of Metrosideros polymorpha in native forests on Hawai’i. J. Ecol. 88, 129–138. doi: 10.1046/j.1365-2745.2000.00437.x
Austin, A. T., Yahdjian, L., Stark, J. M., Belnap, J., Porporato, A., Norton, U., et al. (2004). Water pulses and biogeochemical cycles in arid and semiarid ecosystems. Oecologia 141, 221–235. doi: 10.2307/40005683
Averill, C., and Waring, B. (2018). Nitrogen limitation of decomposition and decay: how can it occur? Glob. Chang. Biol. 24, 1417–1427. doi: 10.1111/gcb.13980
Baldrian, P., Šnajdr, J., Merhautová, V., Dobiášová, P., Cajthaml, T., and Valášková, V. (2013). Responses of the extracellular enzyme activities in hardwood forest to soil temperature and seasonality and the potential effects of climate change. Soil Biol. Biochem. 56, 60–68. doi: 10.1016/j.soilbio.2012.01.020
Bastida, F., Torres, I. F., Andrés-Abellán, M., Baldrian, P., López-Mondéjar, R., Vìtrovskı, T., et al. (2017). Differential sensitivity of total and active soil microbial communities to drought and forest management. Glob. Chang. Biol. 23, 4185–4203. doi: 10.1111/gcb.13790
Beier, C., Beierkuhnlein, C., Wohlgemut, T., Penuelas, J., Emmett, B., Körner, C., et al. (2012). Precipitation manipulation experiments-challenges and recommendations for the future. Ecol. Lett. 15, 899–911. doi: 10.1111/j.1461-0248.2012.01793.x
Berg, B. (1986). Nutrient release from litter and humus in coniferous forest soils-a mini review. Scand. J. Forest Res. 1, 359–369. doi: 10.1080/02827588609382428
Berg, B., and McClaugherty, C. (2003). Plant Litter Decomposition, Humus Formation, carbon Sequestration. New York, NY: Springer-Verlag Press.
Bonan, G. B., Hartman, M. D., Parton, W. J., and Wieder, W. R. (2013). Evaluating litter decomposition in earth system models with long-term litterbag experiments: an example using the community land model version 4 (CLM4). Glob. Chang. Biol. 19, 957–974. doi: 10.1111/gcb.12031
Brandt, L. A., King, J. Y., and Milchunas, D. G. (2007). Effects of ultraviolet radiation on litter decomposition depend on precipitation and litter chemistry in a shortgrass steppe ecosystem. Glob. Chang. Biol. 13, 2193–2205. doi: 10.1111/j.1365-2486.2007.01428.x
Chapin, F. S., Matson, P. A., and Mooney, H. A. (2002). Principles of Terrestrial Ecosystem Ecology. New York, NY: Springer-Verlag Press.
Clein, J. S., and Schimel, J. P. (1994). Reduction in microbial activity in birch litter due to repeated drying and rewetting events. Soil Biol. Biochem. 26, 403–406. doi: 10.1016/0038-0717(94)90290-9
Cornwell, W. K., Cornelissen, J. H. C., Amatangelo, K., Dorrepaal, E., Eviner, V. T., Godoy, O., et al. (2008). Plant species traits are the predominant control on litter decomposition rates within biomes worldwide. Ecol. Lett. 11, 1065–1071. doi: 10.1111/j.1461-0248.2008.01219.x
Currie, W. S., Harmon, M. E., Burke, I. C., Hart, S. C., Parton, W. J., and Silver, W. (2010). Cross-biome transplants of plant litter show decomposition models extend to a broader climatic range but lose predictability at the decadal time scale. Glob. Chang. Biol. 16, 1744–1761. doi: 10.1111/j.1365-2486.2009.02086.x
Davidson, E. A., and Janssens, I. A. (2006). Temperature sensitivity of soil carbon decomposition and feedbacks to climate change. Nature 440, 165–173. doi: 10.1038/nature04514
Duan, J. C., Wang, S. P., Zhang, Z. H., Xu, G., Luo, C., Chang, X., et al. (2013). Non-additive effect of species diversity and temperature sensitivity of mixed litter decomposition in the alpine meadow on tibetan plateau. Soil Biol. Biochem. 57, 841–847. doi: 10.1016/j.soilbio.2012.08.009
Easterling, D. R., Meehl, G. A., Parmesan, C., Changnon, S. A., Karl, T. R., and Mearns, L. O. (2000). Climate extremes: observations, modeling, and impacts. Science 28, 2068–2074. doi: 10.1126/science.289.5487.2068
Epstein, H. E., Burke, I. C., and Lauenroth, W. K. (2002). Regional patterns of decomposition and primary production rates in the U.S. great plains: regional ecological analysis. Ecology 83, 320–327. doi: 10.2307/2680016
Flora, A. R., Amalia, V. D. S., Berg, B., Alfani, A., and Fioretto, A. (1996). Lignin decomposition in decaying leaves of Fagus sylvatica L. and needles of Abies alba Mill. Soil Biol. Biochem. 28, 101–106. doi: 10.1016/0038-0717(95)00120-4
Franklin, H. M., Carroll, A. R., Chen, C. R., Maxwell, P., and Burford, M. A. (2020). Plant source and soil interact to determine characteristics of dissolved organic matter leached into waterways from riparian leaf litter. Sci. Total Environ. 703:134530. doi: 10.1016/j.scitotenv.2019.134530
Githaiga, M. N., Frouws, A. M., Kairo, J. G., and Huxham, M. (2019). Seagrass removal leads to rapid changes in fauna and loss of carbon. Front. Ecol. Evol. 7:62. doi: 10.3389/fevo.2019.00062
Hobbie, S. E., Schimel, J. P., Trumbore, S. E., and Randerson, J. A. (2001). Controls over carbon storage and turnover in high latitude soils. Glob. Chang. Biol. 6, 196–210. doi: 10.1046/j.1365-2486.2000.06021.x
Huysen, T. L., Harmon, H. E., Perakis, S. S., and Chen, H. (2013). Decomposition and nitrogen dynamics of 15N-labeled leaf, root, and twig litter in temperate coniferous forests. Oecologia 173, 1563–1573. doi: 10.1007/s00442-013-2706-8
Intergovernmental Panel on Climate Change [IPCC] (2014). Intergovernmental Panel on Climate Change. doi: 10.1016/B978-0-12-375067-9.00128-5
Jacobson, K. M., and Jacobson, P. J. (1998). Rainfall regulates decomposition of buried cellulose in the Namib Desert. J. Arid Environ. 38, 571–583. doi: 10.1006/jare.1997.0358
Jacotot, A., Marchand, C., and Allenbach, M. (2019). Increase in growth and alteration of C:N ratios of avicennia marina and Rhizophora stylosa subject to elevated CO2 concentrations and longer tidal flooding duration. Front. Ecol. Evol. 7:98. doi: 10.3389/fevo.2019.00098
Jolya, F. X., Weibela, A. K., Coulisb, M., and Throopa, H. L. (2019). Rainfall frequency, not quantity, controls isopod effect on litter decomposition. Soil Biol. Biochem. 135, 154–162. doi: 10.1016/j.soilbio.2019.05.003
Killingbeck, K. T. (1996). Nutrients in senesced leaves: keys to the search for potential resorption and resorption proficiency. Ecology 77, 1716–1727. doi: 10.2307/2265777
Knacker, T., Forstera, B., Rombkea, J., and Frampton, G. K. (2003). Assessing the effects of plant protection products on organic matter breakdown in arable fields-litter decomposition test systems. Soil Biol. Biochem. 35, 1269–1287. doi: 10.1016/S0038-0717(03)00219-0
Knapp, A. K., Ciais, P., and Smith, M. D. (2017). Reconciling inconsistencies in precipitation-productivity relationships: implications for climate change. New Phytol. 214, 41–47. doi: 10.1111/nph.14381
Maynard, D. S., Covey, K. R., Crowther, T. W., Sokol, N. W., Morrison, E. W., Frey, S. D., et al. (2018). Species associations overwhelm abiotic conditions to dictate the structure and function of wood-decay fungal communities. Ecology 99, 801–811. doi: 10.1002/ecy.2165
McCulley, R. L., Burke, I. C., Nelson, J. A., Lauenroth, W. K., Knapp, A. K., and Kelly, E. F. (2005). Regional patterns in carbon cycling across the great plains of North America. Ecosystems 8, 106–121. doi: 10.1007/s10021-004-0117-8
Min, S., Zhang, X., Zwiers, F. W., and Hegerl, G. C. (2011). Human contribution to more-intense precipitation extremes. Nature 470, 378–381. doi: 10.1038/nature09763
Parton, W., Silver, W. L., Burke, I. C., Grassens, L., Harmon, M. E., and Currie, W. S. (2007). Global-scale similarities in nitrogen re-lease patterns during long-term decomposition. Science 315, 361–364. doi: 10.1126/science.1134853
Pastor, J., and Post, W. M. (1988). Response of northern forests to CO2-induced climate change. Nature 334, 55–57. doi: 10.1038/334055a0
Pinheiro, J., Bates, D., DebRoy, S., and Sarkar, D. (2013). nlme: Linear and Nonlinear Mixed Effects Models. R Package Version 3.1-111. Vienna: R Foundation.
Potthast, K., Hamer, U., and Makeschin, H. F. (2012). Land-use change in a tropical mountain rainforest region of southern Ecuador affects soil microorganisms and nutrient cycling. Biogeochemistry 2012, 151–167. doi: 10.2307/23359735
Poulter, B., Frank, D., Ciais, P., Myneni, R. B., Andela, N., Bi, J., et al. (2014). Contribution of semi-arid ecosystems to interannual variability of the global carbon cycle. Nature 509, 600–603. doi: 10.1038/nature13376
Prieto, I., Almagro, M., Bastida, F., and Querejeta, J. I. (2019). Altered leaf litter quality exacerbates the negative impact of climate change on decomposition. J. Ecol. 107, 2364–2382. doi: 10.1111/1365-2745.13168
Pucheta, E., Llanos, M., and Meglioli, C. (2006). Litter decomposition in a sandy monte desert of western argentina: influences of vegetation patches and summer rainfall. Austral Ecol. 31, 808–816. doi: 10.1111/j.1442-9993.2006.01635.x
Qu, H., Pan, C. C., Zhao, X. Y., Lian, J., Wang, S. K., Wang, X. Y., et al. (2019). Initial lignin content is an indicator of predicting leaf litter decomposition and the mixed effects of two perennial gramineous plants in a desert steppe: a 5-year long-term study. Land Degrad. Dev. 30, 1645–1654. doi: 10.1002/ldr.3343
Qu, H., Zhao, X. Y., Wang, S. K., Huang, W. D., and Mao, W. (2014). Effects of different vegetation communities on soil carbon and nitrogen contents in Urat desert steppe. Pratacul. Sci. 31, 355–360. doi: 10.11829/j.issn.1001-0629.2013-0249
Qu, H., Zhao, X. Y., Zhao, H. L., and Wang, S. K. (2010). Research progress of litter decomposition in terrestrial ecosystems. Pratacult. Sci. 27, 44–51.
Salinas, N., Malhi, Y., Meir, P., Silman, M., Cuesta, R. R., Huaman, J., et al. (2011). The sensitivity of tropical leaf litter decomposition to temperature: results from a large-scale leaf translocation experiment along an elevation gradient in Peruvian forests. New Phytol. 189, 967–977. doi: 10.2307/29783383
Sardans, J., Peñuelas, J., and Estiarte, M. (2008). Changes in soil enzymes related to C and N cycle and in soil C and N content under prolonged warming and drought in a Mediterranean shrubland. Appl. Soil Ecol. 39, 223–235. doi: 10.1016/j.apsoil.2007.12.011
Wang, H., Liu, S. R., Wang, J. X., Shi, Z. M., Lu, L. H., Guo, W. F., et al. (2013). Dynamics and speciation of organic carbon during decomposition of leaf litter and fine roots in four subtropical plantations of China. Forest Ecol. Manag. 300, 43–52. doi: 10.1016/j.foreco.2012.12.015
Wang, Y. K., Chang, S. X., Fang, S. Z., and Tian, Y. (2014). Contrasting decomposition rates and nutrient release patterns in mixed vs singular species litter in agroforestry systems. J. Soil Sediment 14, 1071–1081. doi: 10.1007/s11368-014-0853-0
Whitford, W. G., Martínez-Turanzas, G., and Martínez-Meza, E. (1995). Persistence of desertified ecosystems: explanations and implications. Environ. Monit. Assess. 37, 319–332. doi: 10.1007/BF00546898
Xu, X., and Hirata, E. (2005). Decomposition patterns of leaf litter of seven common canopy species in a subtropical forest: N and P dynamics. Plant Soil 273, 279–289. doi: 10.1007/s11104-004-8069-5
Yahdjian, L., Sala, O. E., and Austin, A. T. (2006). Differential controls of water input on litter decomposition and nitrogen dynamics in the Patagonian steppe. Ecosystems 9, 128–141. doi: 10.1007/s10021-004-0118-7
Yuste, J. C., Peñuelas, J., Estiarte, M., Garcia-Mas, J., Mattana, S., Ogaya, R., et al. (2011). Drought-resistant fungi control soil organic matter decomposition and its response to temperature. Glob. Chang. Biol. 17, 1475–1486. doi: 10.1111/j.1365-2486.2010.02300.x
Zhang, B., Wang, H. L., Yao, S. H., and Bi, L. D. (2013). Litter quantity confers soil functional resilience through mediating soil biophysical habitat and microbial community structure on an eroded bare land restored with mono Pinus massoniana. Soil Biol. Biochem. 57, 556–567. doi: 10.1016/j.soilbio.2012.07.024
Zhou, H. C., Tam, N. F., Lin, Y. M., Wei, S. D., and Li, Y. Y. (2012). Changes of condensed tannins during decomposition of leaves of Kandelia obovata in a subtropical mangrove swamp in China. Soil Biol. Biochem. 44, 113–121. doi: 10.1016/j.soilbio.2011.09.015
Keywords: litter decomposition, precipitation patterns, arid region, C/N ratio, lignin content
Citation: Qu H, Zhao X, Lian J, Tang X, Wang X and Medina-Roldán E (2020) Increasing Precipitation Interval Has More Impacts on Litter Mass Loss Than Decreasing Precipitation Amount in Desert Steppe. Front. Environ. Sci. 8:88. doi: 10.3389/fenvs.2020.00088
Received: 08 February 2020; Accepted: 29 May 2020;
Published: 30 June 2020.
Edited by:
Xian Xue, Northwest Institute of Eco-Environment and Resources (CAS), ChinaReviewed by:
Guodong Han, Inner Mongolia Agricultural University, ChinaJianshuang Wu, Chinese Academy of Agricultural Sciences, China
Copyright © 2020 Qu, Zhao, Lian, Tang, Wang and Medina-Roldán. This is an open-access article distributed under the terms of the Creative Commons Attribution License (CC BY). The use, distribution or reproduction in other forums is permitted, provided the original author(s) and the copyright owner(s) are credited and that the original publication in this journal is cited, in accordance with accepted academic practice. No use, distribution or reproduction is permitted which does not comply with these terms.
*Correspondence: Hao Qu, cXVoYW9AbHpiLmFjLmNu; Eduardo Medina-Roldán, ZWR1YXJkby5tZWRpbmEtcm9sZGFuQHhqdGx1LmVkdS5jbg==