- 1School for Environment and Sustainability, Cooperative Institute for Great Lakes Research, University of Michigan, Ann Arbor, MI, United States
- 2Department of Biology, Institute for Great Lakes Research, Central Michigan University, Mount Pleasant, MI, United States
Manganese (Mn), an element sensitive to redox conditions in aquatic environments, plays a role in numerous biogeochemical cycles. Although the speciation of Mn in lakes largely tracks the availability of dissolved oxygen, direct oxidation of Mn by molecular oxygen occurs slowly and, instead, the majority of Mn oxidation occurs via biotic and abiotic mechanisms involving microbes, organic matter, light, reactive oxygen species, and mineral surfaces. While each of these mechanisms is either known or likely to occur in freshwater, the relative balance and interaction among these biogeochemical pathways in an intact plankton system remains uncertain. We investigated potential abiotic and biotic mechanisms contributing to Mn oxide formation in Lake Erie, which experiences seasonal hypoxia and accumulation of Mn during seasonal stratification. Overall, Mn oxidation rates were much higher in the shallow and highly productive western basin (up to 1.5 μmoles L−1 d−1) compared to the deeper and less productive central basin, where we observed very little Mn oxidation over 7 days. Our experiments suggest that abiotic mechanisms involving mineral surfaces played a larger role than biotic mechanisms, particularly in the light where Mn oxidation was highest. Reactive oxygen species exhibited antagonistic roles: hydrogen peroxide acted as a net reductant for manganese oxides and completely masked oxidation of Mn by the superoxide free radical. These findings show that multiple mechanisms may exert control over the fate of Mn and suggest that Mn released from sediments during hypoxia can potentially remain dissolved in the water for an extended period of time. Since the severity of Lake Erie hypoxia has increased in recent years, and we are becoming increasingly aware of the health effects of Mn in drinking water sources, these findings should help inform efforts to predict when and where Mn will accumulate in the lake water.
Introduction
Lake Erie, one of the Laurentian Great Lakes, plays a valuable role to the ecology, economy, and population of the Great Lakes region and supplies drinking water to over 11 million people. It is unique among the Laurentian Great Lakes as it is the shallowest lake and its three distinct basins (eastern, central, and western) vary in depth, productivity, susceptibility to thermal stratification, and terrestrial inputs (Beletsky et al., 2012; Scavia et al., 2014; Cory et al., 2016; Prater et al., 2017). The western basin is shallow and most affected by riverine inputs of nutrients, which sustain high primary production and recurrent cyanobacterial blooms (Bertani et al., 2016; Scavia et al., 2016). The eastern basin is deepest and least productive. The central basin is intermediate in depth and productivity, but is characterized by widespread seasonal hypoxia in bottom waters (Scavia et al., 2014; Zhou et al., 2015). One of the consequences of hypoxia for water quality is the release of dissolved manganese (Mn) from the sediments into the water, which occurs not only in Lake Erie (Burns and Nriagu, 1976; Lum and Leslie, 1983), but other lakes and reservoirs (Davison, 1981; Munger et al., 2019). Hydrodynamic upwellings in the central basin have the potential to transport hypoxic water toward shore (Rowe et al., 2019), which can result in elevated Mn concentrations entering intake pipes for public water utilities (Ruberg et al., 2008). Mn is a secondary contaminant in drinking water owing to aesthetic effects (United States Environmental Protection Agency, 1996) and it is now known that micromolar concentrations of Mn in drinking water can cause neurologic impairments, particularly in children (Bouchard et al., 2011; Khan et al., 2012). Elevated Mn in seasonally anoxic bottom waters of Lake Erie has been documented previously, and with increased spatial extent of hypoxia in recent years (Zhou et al., 2013), there is a need to understand the biogeochemical cycling and fate of Mn in Lake Erie.
Mn in aquatic environments exhibits three oxidation states, with each one impacting different ecological and biogeochemical processes. Mn(II) is the most reduced form of Mn and it is a soluble antioxidant within aquatic ecosystems (Wuttig et al., 2013) and inside bacterial cells (Daly et al., 2004). Mn(IV) is insoluble and forms numerous different oxide minerals (reviewed in Post, 1999; Tebo et al., 2004). Mn(III) is an unstable intermediate that forms ligand complexes with dissolved organic matter and metal oxides (Oldham et al., 2017) and serves as both an oxidant and reductant (Yakushev et al., 2009; Madison et al., 2013; Oldham et al., 2019). Mn(VII) is soluble and a powerful oxidant, but to date, is not known to be an important Mn species in aquatic environments (Sunda et al., 1983; Hansel and Learman, 2015; Hansel, 2017) and would likely react rapidly with organic matter. Mn(III,IV) oxides are important in the environment as they have a high affinity to sorb other heavy metals (e.g., lead and copper), bind nutrients like phosphorus (Yao and Millero, 1996; Post, 1999), and also can oxidize complex organic molecules (Tebo et al., 2004; Spiro et al., 2009). Mn (III, IV) oxides are also important as they can serve as terminal electron acceptors for microbes (Nealson and Saffarini, 1994; Tebo et al., 2005) and facilitate anaerobic degradation of organic matter.
The distribution and fate of Mn in aquatic systems depends on various factors, the predominant one being oxidative and reductive processes. In particular, oxidation of Mn(II) to Mn(IV) leads to the formation of insoluble (oxy)hydroxide minerals that tend to accumulate in sediments (Tipping et al., 1984; Post, 1999). Thus, the oxidation of Mn is key to understanding persistence of dissolved Mn(II) in the water column of lakes (Davison, 1981), rivers (Anderson et al., 2011), caves (Carmichael et al., 2013), and the ocean (Sunda and Huntsman, 1988, 1990). Homogenous oxidation of Mn(II) by molecular oxygen is thermodynamically favorable at the pH of most lakes, but kinetically limited in the absence of a biotic or abiotic catalyst and will occur slowly (Diem and Stumm, 1984). However, abiotic oxidation of Mn(II) by molecular oxygen be can catalyzed via complexation with organics compounds (Nico et al., 2002; Duckworth and Sposito, 2005) or by Mn oxide mineral surfaces (Diem and Stumm, 1984; Davies and Morgan, 1989; Junta and Hochella, 1994). Biotic Mn(II) oxidation can be catalyzed enzymatically by a taxonomically diverse group of bacteria and fungi (Boogerd and De Vrind, 1987; Miyata et al., 2006; Dick et al., 2008; Anderson et al., 2009; Geszvain et al., 2011; Butterfield et al., 2013). Biotic oxidation of Mn(II) is important both in contemporary (Sunda and Huntsman, 1990; Tebo, 1991) and geological timescales (Ossa Ossa et al., 2018) and occurs directly via enzymes such as multicopper oxidases or heme peroxidases (Dick et al., 2008; Anderson et al., 2009; Butterfield et al., 2013; Nakama et al., 2014), indirectly due to high pH micro-environments produced by photosynthesis (Chaput et al., 2019), and indirectly through the biological production of the superoxide free radical (O2–) (Learman et al., 2011a; Hansel et al., 2012). In addition to producing superoxide, many microbes are known to produce hydrogen peroxide as a metabolism byproduct (Dixon et al., 2013; Marsico et al., 2015), which could serve to reduce Mn oxides and potentially compete with Mn oxidation caused by superoxide (Sunda and Huntsman, 1994; Learman et al., 2013). Other abiotic processes include photo-reduction of Mn oxides (Sherman, 2005) and interactions with organic matter ligands (Oldham et al., 2017; Oldham et al., 2019).
Much of our understanding of the mechanisms of Mn oxidation comes from marine and estuarine systems, but far less is known about these mechanisms in freshwater ecosystems. A longstanding conceptual model in freshwater has been that the distribution and speciation of Mn within the water column is a simple balance between Mn reduction by microbes, which is thought to occur only in anoxic sediments or water, and abiotic Mn mineralization, which occurs rapidly in oxygenated water and results in sedimentation of Mn oxy(hydr)oxides (Davison, 1981, 1993). This conceptual model is based on vertical patterns of Mn, redox gradients, and dissolved oxygen in lakes and reservoirs subject to seasonal or permanent stratification (Mortimer, 1971; Burns and Nriagu, 1976; Hongve, 1997; Munger et al., 2017). This understanding of Mn cycling has been useful in applied lake management – artificial aeration of bottom water in reservoirs effectively slows Mn release and results in decreased concentrations of Mn in the water (Zaw and Chiswell, 1999; Gantzer et al., 2009; Gerling et al., 2014; Munger et al., 2016). Studies in freshwater have shown that Mn is oxidized more rapidly than Fe(II) (Hsiung and Tisue, 1994; Stumm and Morgan, 1996) and also that rates of Mn oxidation measured in lake water are higher than expected from homogeneous oxidation by molecular oxygen (Delfino and Lee, 1968; Diem and Stumm, 1984; Hsiung and Tisue, 1994; Zaw and Chiswell, 1999). This disparity in rates has led to the hypothesis that Mn oxidation in marine (Tebo, 1991) and freshwater ecosystems is a primarily mediated by biological processes (Chapnick et al., 1982; Tipping, 1984; Hsiung and Tisue, 1994; Aguilar and Nealson, 1998).
While all of the mechanisms described above have been demonstrated individually, the relative contribution of different biotic and abiotic mechanisms to Mn oxidation in freshwater systems is poorly understood (but see Nealson et al., 1988; Tebo, 1991). Further, these different mechanisms have not been examined simultaneously in experiments with an intact plankton system which includes phototrophs, heterotrophs, mineral particles, and dissolved organic matter (DOM). Due to the relevance of Mn cycling to water quality in lakes, such as Lake Erie, there is a need to examine the relative impact of different proposed mechanisms in a real biological system.
A greater understanding of the fate of Mn in Lake Erie would not only provide new insights into the cycling of this metal in freshwater ecosystems, it would also provide data for water managers as higher levels of Mn in water intakes are becoming more frequent. Here, we present the results of an experiment designed to test whether these proposed mechanisms occur in an intact freshwater plankton system. We used water from Lake Erie and applied experimental manipulations to examine the roles of: particles (using filtration); biological particles (using formaldehyde to kill microbes); photosynthetic metabolism (using light vs. dark); superoxide (using the enzyme superoxide dismutase to scavenge superoxide); and hydrogen peroxide (using the enzyme catalase to remove hydrogen peroxide). As our experiments were performed in an intact plankton system, where multiple mechanisms are occurring simultaneously, we use Table 1 to outline the hypothesized mechanisms for Mn oxidation, the representative reactions, and our expectations in terms of Mn oxide concentrations and Mn oxide production rates.
Materials and Methods
Sample Stations and Collection
We collected water from two biogeochemically contrasting areas of Lake Erie: the western basin, which is characterized by high biological production, sediment resuspension, and generally oxic conditions and the central basin, which is deeper and has lower biological production and undergoes seasonal hypoxia in the hypolimnion. We sampled on June 17 and July 8, 2019 in the western basin aboard the National Oceanic and Atmospheric Administration (NOAA) vessel R4108 at NOAA Great Lakes Environmental Research Laboratory station WE2 (41.762°N, 83.33°W). In the central basin, we sampled from two different stations located within 15 km (Figure 1). On June 4, 2019, we sampled station NOAA station CB2 (41.7588°N, 82.1352°W) aboard the NOAA vessel R5503. On June 27, 2019, we sampled at EPA station ER43 (41.7883°N, 81.9450°W) aboard the R/V Lake Guardian. Hereafter, we will refer to these locations collectively as the western and central basins. All water samples were collected in Niskin bottles from a depth of 1–2 m below the surface. Samples were stored in the dark at the same ambient temperature as the lake (Table 2). Although we collected water from the surface mixed layer for these experiments, it is important to note that bottom water was not hypoxic at the time of collection (i.e., >2 mg L−1 dissolved oxygen). We initiated the experiments within 24 h of collecting the samples.
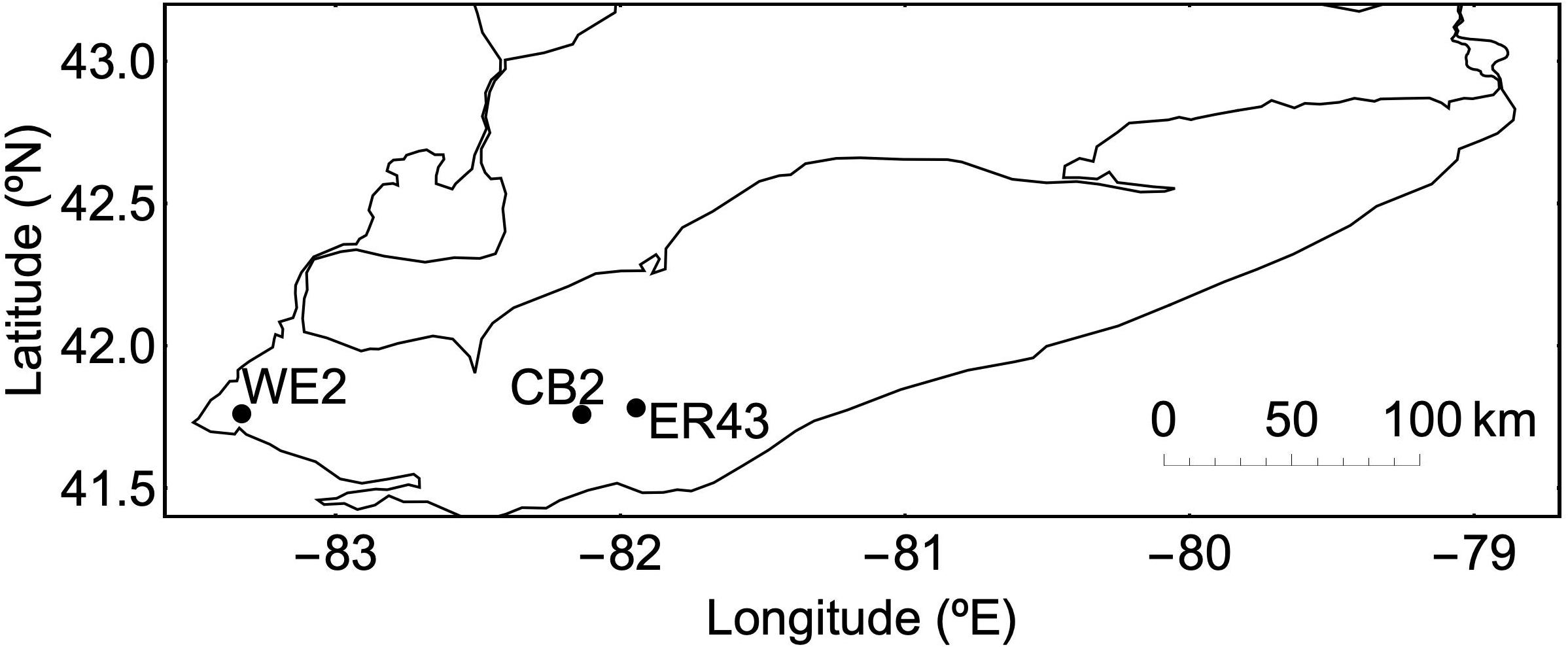
Figure 1. Map of Lake Erie showing the three sampling locations in the central (CB2 and ER43) and western basins (WE2) of the lake.
Experimental Design
Each experiment followed the same experimental design, with six primary treatments in complete factorial with incubation in either the light or dark. All treatments received 5 μmoles L−1 Mn as MnCl2. The control treatment consisted of whole lake water. For the filtered treatment, we removed particles larger than 0.2 μm using a Whatman GD/X filter. For the killed treatment, we added formaldehyde to a final concentration of 0.1% (w/v). Preliminary experiments showed that higher concentrations of formaldehyde caused reduction of Mn(VII) oxides and interfered with the leucoberbelin blue method. We did not observe significant increases in absorbance at 624 nm in any of the experimental units treated with formaldehyde. The enzyme superoxide dismutase (SOD) has been used previously to inhibit the activity of superoxide as an oxidant of Mn(II) (Learman et al., 2011a, 2013; Andeer et al., 2015). In the SOD treatment, we added 12.5 KU of superoxide dismutase (from bovine erythrocytes, Sigma-Aldrich item 574594) to unfiltered lake water. This concentration was equivalent to 5% of the amount used by Learman et al. (2011a) and reflects our expectation that concentrations of superoxide would be lower in lake water than in a dense laboratory culture. For the catalase treatment, we added 4 KU of catalase (from bovine liver, Sigma-Aldrich item C40) to unfiltered lake water in order to scavenge hydrogen peroxide. This concentration was also scaled to 5% of the amount used by Learman et al. (2011a) in cell culture, similar to the SOD treatment. Because catalase is unstable, we added 4 KU 1 h prior to each of the timepoints on the first day of the experiments and thereafter at 1 h prior to sampling. For the SOD/catalase treatment, we applied both the SOD and catalase amendments to unfiltered lake water, including periodic additions of catalase at the same timing and concentration as in the catalase only treatment.
Each treatment was incubated under two different light levels: complete darkness (wrapped in aluminum foil) or high light (300 μmoles m–2 s−1 photosynthetically active radiation, PAR). We selected this intensity because it corresponds to the mean daytime PAR intensity experienced in the surface 2 m of Lake Erie (Weiskerger et al., 2018). Continuous illumination was provided by an array of light emitting diodes (Waveform Lighting) that illuminated all experimental units at the same intensity (±5%) and broad PAR spectrum from 400 to 800 nm. The LEDs were upward-facing beneath a glass platform on which the experimental units were arranged. Experimental units had a volume of 100 mL and were contained in 125 mL borosilicate glass Erlenmeyer flasks that had previously been soaked in 3.7% hydrochloric acid and rinsed with type II water prior to use. We performed each experiment in an environmental chamber that maintained the units at the same temperature as the lake water (Percival Scientific). Flasks were randomly arranged in the environmental chamber and incubated over the course of 7 days.
Sampling Procedures
We measured the production of Mn oxides over the course of each experiment using the dye leucoberbelin blue (LBB), which produces an intense blue color and absorbance peak at 624 nm when reacted with Mn oxides (Krumbein and Altmann, 1973; Lee and Tebo, 1994; Learman et al., 2011a; Oldham et al., 2017). We made two modifications to this method to suit our experiment. First, we decreased the concentration of LBB (Sigma-Aldrich 432199) in order to minimize background absorbance from unreacted dye. Second, we corrected for the background absorbance in the sample and buffer. In our modified method, we mixed 2 mL of sample with 0.67 mL of either 136 mM acetic acid buffer (in water) or acetic acid buffer with 294 μmoles L−1 LBB. Samples were incubated in the dark for 15 min to allow for reaction with the reagent and buffer. Then, absorbance was measured at 624 nm using a Perkin Elmer Lambda 40 spectrophotometer and a 1 cm pathlength. For each sample, we quantified the difference in absorbance between the sample with buffer only and the sample with buffer and LBB. We made standard curves using 0–2 μmoles L−1 potassium permanganate. Since each molecule of permanganate oxidizes five molecules of LBB, whereas one molecule of MnO2 would oxidize two molecules of LBB, 2 μmoles L−1 as permanganate should produce the same absorbance as 5 μmoles L−1 as manganese (IV) dioxide. Because both Mn(III) and Mn(IV) oxides are known to react with LBB, and a given sample may contain a mix of Mn(III) and Mn(IV) oxides (Oldham et al., 2017), we chose to convert the absorbance produced by reaction with LBB to Mn(IV) equivalents.
We sampled each experimental unit at the start of the experiment and twice more during the first day of the experiment. Flasks were consequently sampled once per day for the remaining 6 days of the experiment. Prior to sampling, the flasks were manually mixed for 3 s to homogenize the plankton. At the final sampling in each experiment, we measured Mn oxide concentration in both the raw and filtered (<0.2 μm) water from each treatment.
Statistical Analyses
The treatments exhibited peak Mn oxide concentrations at different times during the experiments (Supplementary Material), thus we summarized the measurements from each experimental unit in terms of the maximum Mn oxide concentration and maximum Mn oxide production rate. We analyzed the maximum Mn oxide concentrations in each experiment using a two-way analysis of variance (ANOVA) with fixed effects of treatment and light. We quantified the maximum rate of Mn oxide formation observed during each time series from the increase in Mn oxide equivalent concentration vs. time. For each flask, we applied a moving window of four consecutive timepoints and used linear regression to estimate the rate across each window. We report the highest rate from each flask as the maximum Mn oxide production rate. Changing the window size from four timepoints to three timepoints caused little change in terms of the mean rate estimates, but did affect the variance. We evaluated pairwise differences among treatments using Tukey’s method. All statistical analyses were performed in R version 3.4.2 using the function “lm” in the “stats” package. The R code and complete dataset are provided as Supplementary Material.
Electron Microscopy
We performed transmission electron microscopy (TEM) and energy-dispersive X-ray spectroscopy (EDS) on Mn oxide particles produced in both lake water from Lake Erie and in the catalase treatment of our experiment. The lake water was sampled from the anoxic hypolimnion at station CB2 (central basin) on September 19, 2019, aerated, and stored at 4°C for 7 days prior to centrifugation at 1000 g for 20 min to form a pellet. The catalase treatment samples (sampled on July 8, 2019 in the western basin) were stored at −20°C and then were thawed, sonicated, and centrifuged at 14,000 g for 15 min. The pellets from the catalase treatment and the Lake Erie water were resuspended in 2 mL of ultrapure water, sonicated, and the centrifuged at 14,000 g for 15 min. The water was decanted and then the pellet was resuspended in 0.2 mL of ultrapure water and sonicated, and then the concentrated samples from each condition was loaded onto a copper TEM grid with a lacy carbon coating (Ted Pella) and then placed in a desiccator to allow water to evaporate. Imaging was performed at Central Michigan University on a Hitachi HT7700 TEM and EDS data was collected on a Thermo Scientific NSS Pathfinder to confirm the presence of Mn.
Results and Discussion
Homogenous Oxidation by Dissolved Oxygen
We hypothesized that homogenous oxidation by dissolved oxygen would not result in appreciable formation of Mn oxides (H1, Table 1). Our results support this hypothesis: Figure 2 shows that in the absence of particles, Mn oxide formation was undetectable in both the western and central basins (<2% of added Mn), even after 1 week in well-oxygenated dark incubations. This result shows that reduced Mn is not rapidly oxidized by dissolved oxygen under the conditions present in Lake Erie and that homogenous oxidation is not a primary means of Mn oxide formation and removal from the surface mixed layer. This finding is consistent with previous studies reporting that it can take several years for Mn(II) to be oxidized by oxygen in the absence of microbes or mineral particles (Diem and Stumm, 1984; Tipping, 1984). Although homogenous oxidation by dissolved oxygen is not responsible for appreciable Mn oxidation in Lake Erie, we did find support for several of the other proposed mechanisms of Mn oxidation.
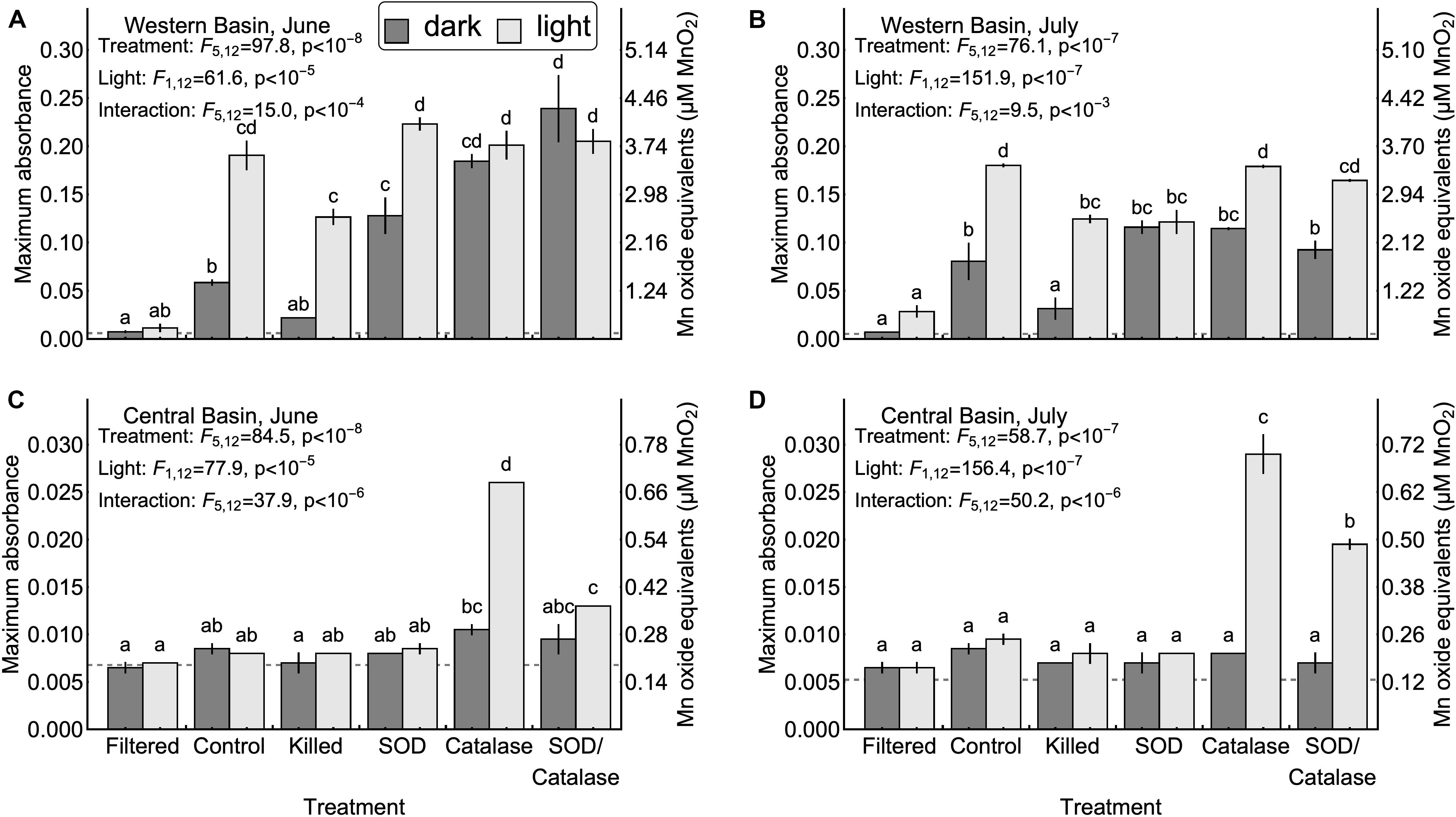
Figure 2. Maximum Mn oxide concentrations observed in each experiment. Error bars represent the values measured in two experimental replicates. Upper confidence intervals for the mean of 10 method blanks are denoted by dash gray lines. Note that the vertical axis ranges differ between the central basin (A,B) and western basin (C,D). Inset letters denote groups of treatments that were not significantly different based on post-hoc comparisons (p < 0.05). Any two treatments that do not share one letter in common are interpreted as different by this criterion.
Abiotic Mn Oxidation
Next, we tested the hypothesis that mineral surfaces can facilitate the oxidation of Mn(II) (H2, Table 1). We did not observe measurable production of Mn oxides in the central basin. In the western basin, the killed experimental treatment (containing only mineral particles and dead biological particles) had higher concentrations of Mn oxides than the filtered treatment (no particles), although this difference was only significant in the light (Figure 2). Similarly, the killed treatment applied to the western basin showed significantly higher rates of Mn oxide production compared to the filtered treatment (except June dark, Figure 3). Higher production of Mn oxides in the presence of particles (minerals, detritus, and killed cells) is consistent with Hypothesis 2 and suggests that minerals are catalyzing oxidation reactions (Table 1). When those particles were removed by filtration we did not observe any production of Mn oxides. Although oxidation by molecular oxidation is kinetically limited at pH below 10, Mn oxides and other minerals can catalyze the oxidation of Mn(II) by molecular oxygen (Davies and Morgan, 1989; Tebo et al., 2005; Learman et al., 2011b; Hansel and Learman, 2015). Suspended sediments in Lake Erie contain both Mn and Fe oxides (Burns and Nriagu, 1976; Mudroch, 1984) and the predominant inputs of suspended sediments to the surface mixed layer is through river inputs and wind-driven resuspension in the shallow western basin (Binding et al., 2012; Shuchman et al., 2013). Concentrations of non-volatile suspended solids were higher in the western basin experiments (33.7 mg L−1 in June 5.25 mg L−1 in July) compared to the central basin (<1 mg L−1), which potentially explains why we did not observe production of Mn oxides from the central basin under the killed treatment. This finding is also consistent with other studies in marine systems showing that Mn(II) oxidation rates are faster in nearshore waters where Mn oxide particles are present (Tebo and Emerson, 1985; Sunda and Huntsman, 1987; Tebo, 1991). Moreover, previous experiments have shown that rates of Mn oxidation catalyzed by mineral surfaces are faster in the light than in the dark (Nico et al., 2002; Learman et al., 2011b, 2013). Although there is no consensus explanation for this phenomenon, it could be attributable to production of ROS through reactions involving DOM or mineral particles (Learman et al., 2011b). Nonetheless, the effects of visible light on abiotic oxidation by mineral particles is consistent with the effects that we observed in the western basin (Figure 3). While particle-associated reactions made up the majority of the abiotic oxidation in the light, we did see limited production of Mn oxides in the filtered treatment (western basin, July), which suggests that photochemical reactions with dissolved organic matter may play a small role in Mn oxide formation in Lake Erie (Painter et al., 2001; Hansel, 2017), or that nanoparticles smaller than 0.2 microns help catalyze oxidation of Mn (Madden and Hochella, 2005).
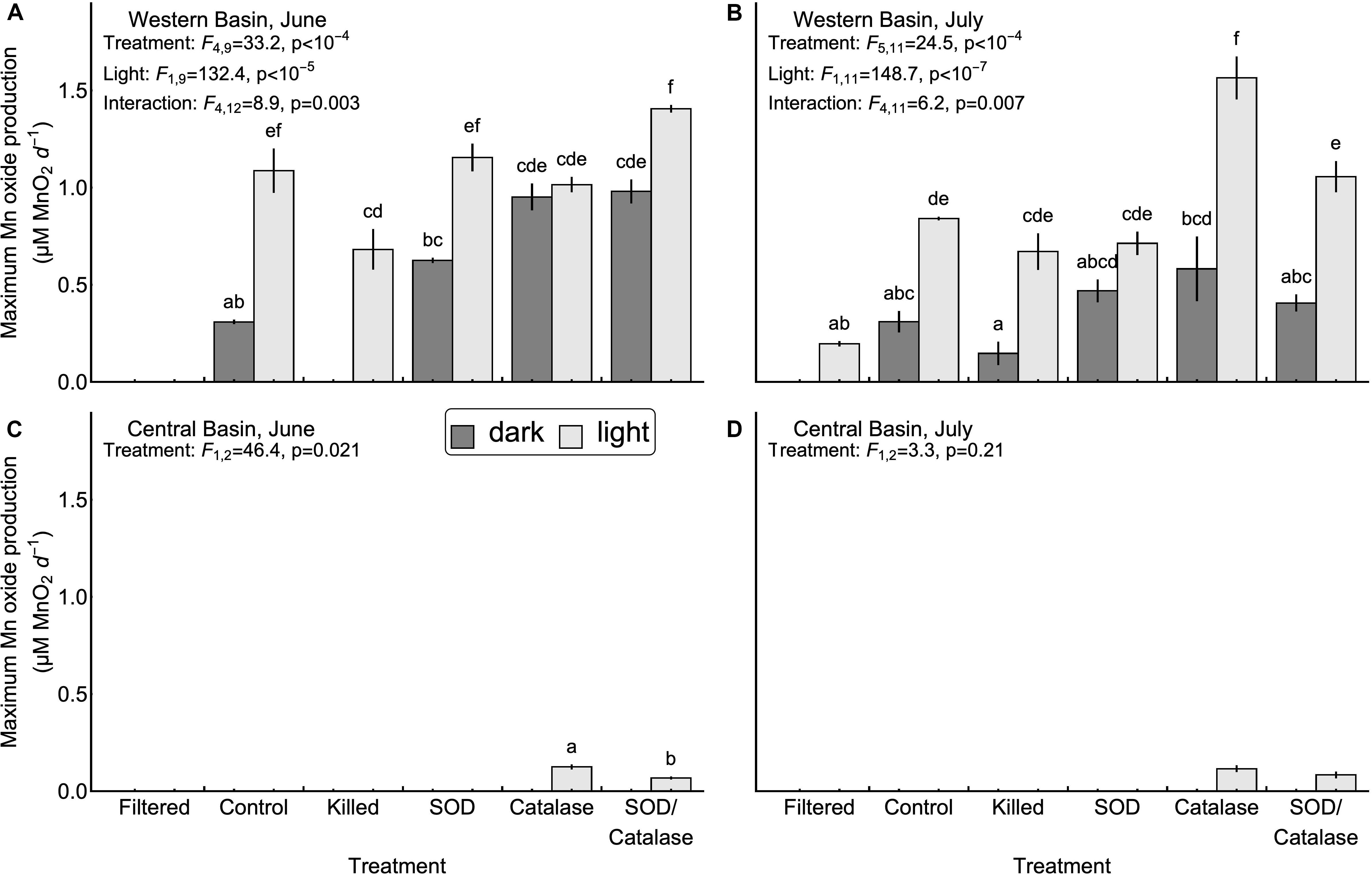
Figure 3. Maximum Mn oxide production rates observed in the western (A,B) and central basins (C,D). Error bars represent the values measures in two experimental replicates. No bars were drawn for treatments where significant Mn oxide production was not observed over the course of the experiment, or for the July experiment in the central basin due to a lack of significant differences among treatments. Inset letters denote groups of treatments that were not significantly different based on post-hoc comparisons (p < 0.05). Any two treatments that do not share one letter in common are interpreted as different by this criterion.
Biological Mn Oxidation
Our third hypothesis (H3, Table 1), that heterotrophic biological activity causes Mn oxidation, was supported by significant differences in Mn oxide concentrations (Figure 2B, July p < 0.05) and Mn oxide production rates (Figure 3A, June p < 0.05) between the killed + dark and control + dark treatments applied to the western basin. We did not observe detectable rates of Mn oxidation in the control treatments from the central basin. These findings show that biological processes played a critical role in Mn oxidation, which is consistent with both experimental (Tebo et al., 2005) and observational studies (Chapnick et al., 1982; Tipping et al., 1984; Hsiung and Tisue, 1994).
We also hypothesized that photosynthetic biological activity causes Mn oxidation (H4, Table 1). In our experiments from the western basin, the control treatments produced significantly higher Mn oxide concentration in the light than the dark, which initially suggests that photosynthetic organisms might play a role in Mn oxide formation (Figure 2A). If this were true, then the addition of formaldehyde should obliterate much of the positive effect of light on Mn oxide production. However, the absolute decrease in Mn oxide concentration (or Mn oxide formation rate) with addition of formaldehyde was similar between the light and dark treatments (Figure 2A), suggesting that the biocide’s effect may have been to reduce oxidation by non-photosynthetic biological processes only (e.g., heterotrophic microorganisms). This suggests that photosynthetic activity does not represent the sole mechanism of Mn oxidation in Lake Erie and the majority of biological Mn oxidation may be due to heterotrophic microorganisms. An alternative explanation for this pattern may be that photosynthetic microbes inside colonies or other aggregates were not killed by the addition of formaldehyde in the experiments. Although we did not observe detectable increases in absorbance (without LBB) in the killed treatments, we cannot rule out some remaining contribution from metabolism. Further studies with different biocide treatments (Tebo, 1991) and the addition of mineral particles could help to determine the importance of light for mineral surface interactions.
Role of Reactive Oxygen Species
We hypothesized that superoxide is an important oxidant of Mn (II) in Lake Erie (H5, Table 1). We tested this hypothesis by adding superoxide dismutase to remove the effect of the free radical. We observed no effect of SOD on Mn oxidation in the central basin. Addition of SOD led to inconsistent effects on Mn oxide concentration and Mn oxide production rates in the western basin (Figures 2, 3). In particular, the July experiment from the western basin did show a decrease in Mn oxide concentration with SOD (in the light only). As a result, our experiments provide little support for superoxide as a primary mechanism of Mn oxidation. This finding was surprising given previous laboratory experiments showing that biologically produced superoxide is a potent oxidant of Mn(II) and Mn(III) (Learman et al., 2011a; Hansel et al., 2012). While addition of SOD did not significantly decrease production of Mn oxides in any of the experiments, the effect of formaldehyde addition shows that some of the Mn oxide production was driven by biological processes. This suggests that other biological mechanisms, besides superoxide, could play an important role. Such biological mechanisms could include Mn specific oxidases (Miyata et al., 2006; Dick et al., 2008; Anderson et al., 2009; Butterfield et al., 2013; Nakama et al., 2014) or exudation of DOM (Duckworth and Sposito, 2005; Hansel, 2017), both of which can catalyze the oxidation of Mn(II) by dissolved oxygen.
Although hydrogen peroxide is not an oxidant of Mn(II) under the conditions in Lake Erie, we hypothesized that hydrogen peroxide has an inhibitory effect of Mn oxide accumulation and production rates by acting as a reductant for Mn(III, IV) oxides (H6, Table 1). We tested this hypothesis by adding catalase to remove the effect of hydrogen peroxide as a reductant. Our experiments in the central basin showed significantly higher Mn oxide concentrations and Mn oxide production rates in the catalase treatment compared to the control, although only in the light (Figures 2, 3). In the western basin there was no difference in maximum Mn oxide concentration between the control and catalase treatments, through there were significant increases in Mn oxide production rate in June (dark treatment) and July (light treatment). However, photosynthetic activity is also known to produce hydrogen peroxide (Painter et al., 2001) and may explain why the inhibitory effect of hydrogen peroxide was greater in the light than in the dark.
Our final hypothesis was that superoxide and hydrogen peroxide acts as competing redox reactions with Mn (H7, Table 1). Compared to the SOD only treatment, addition of SOD/catalase in the western basin significantly increased Mn oxide concentration (June, dark) but had no significant effect on maximum Mn oxide production rate. In the central basin the addition of SOD/catalase led to higher Mn oxide concentrations and Mn oxide production rates compared to the SOD only treatment. In the June experiment the SOD/catalase treatment lowered Mn oxide production rates by 45% compared to catalase only treatment. These results suggest that superoxide was responsible for approximately half of the gross Mn oxidation under those conditions, but the net effect is not detectable due to the effect of hydrogen peroxide. Consistent with Hypothesis 7, our experiments show that both hydrogen peroxide acting as a reductant of Mn oxides can potentially negate the effect of superoxide acting as an oxidant, which has been documented in other studies (Archibald and Fridovich, 1982; Nico et al., 2002; Hansard et al., 2011; Learman et al., 2011a, 2013; Hansel et al., 2012). In several of our experiments the SOD/catalase treatment exhibited higher Mn oxide concentrations and Mn oxide formation rates than the control treatment (Figures 2, 3). If superoxide and hydrogen peroxide were the only mechanisms at play, we would expect the SOD/catalase treatment to produce no more Mn oxides than the control. In contrast, our results suggest that not all of the Mn oxidation was attributable to superoxide and that the other mechanisms of oxidation are normally overwhelmed by the effect of hydrogen peroxide acting as a reductant.
These results suggest that reactive oxygen species could lead to rapid cycling among different redox states within the surface mixed layer, similar to what has been observed in marine and estuarine systems (Sunda and Huntsman, 1988, 1990). The presence of both oxidizing and reducing mechanisms under the conditions in the surface mixed layer has two important implications. First, the presence of reducing mechanisms in the surface mixed layer could serve to slow advection of Mn from the surface mixed layer, similar to what has been proposed in marine systems (Sunda and Huntsman, 1988, 1990). Second, because Mn(III, IV) oxides are among the strongest oxidants in aquatic systems (Yakushev et al., 2009; Oldham et al., 2019), cycling of Mn oxides in the surface mixed layer could have important consequences for organic matter (Allard et al., 2017).
Light-Mediated Effects on Mn Oxide Production
We found that light treatment had a significant and overall positive effect on Mn oxide concentration in all four experiments (Figure 2). This is consistent with other recent work showing that light can enhance Mn oxide production via both photosynthetic microbes (Richardson et al., 1988; Chaput et al., 2019) and/or abiotic mechanisms (Nico et al., 2002; Learman et al., 2011b, 2013). As discussed above, our experiment does not suggest that photosynthesis was solely responsible for the positive effect of light on Mn oxide formation. Instead, our results are consistent with an abiotic photochemical process catalyzed by mineral particles. This phenomenon has been reported previously (Learman et al., 2011b, 2013), but remains poorly understood. We did not find evidence for direct photoreduction of Mn oxides or photo-mediated reduction of Mn oxides by DOM, both of which are known to occur (Sunda and Huntsman, 1988, 1990; Bertino and Zepp, 1991). It is possible that photoreduction did occur but was slower than oxidation, which would have masked this reaction.
Despite these dramatic effects of light on Mn oxidation, our experiment did not completely mimic the natural intensity and spectrum of light in the surface mixed layer of the lake, which could impact interpretation of the experiment in two distinct ways. First, although the lights that we used for our experiment provide the complete PAR spectrum at an intensity representative of daytime PAR conditions in the surface 2 m of Lake Erie, it is possible that some photosynthetic microbes were under-saturated or, more likely, over-saturated under these conditions. If phytoplankton were undersaturated for light, it is possible that our estimates of light-mediated Mn oxidation underestimate the true net effect of light that may occur at the surface of the lake. Second, although the light intensities were high throughout the visible spectrum, our light source did not extend into the ultraviolet portion of the spectrum. Light-mediated reduction of Mn, particularly by ultraviolet wavelengths, is known to occur directly through photo-reduction of Mn(III, IV) (Sunda and Huntsman, 1988, 1990) and indirectly through reaction with DOM or mineral oxides (Sunda et al., 1983; Bertino and Zepp, 1991).
Lake Erie is similar to other freshwater lakes in that UV light is more rapidly attenuated with depth than visible wavelengths or PAR (Morris et al., 1995), and wavelengths corresponding to UV-B and lower do not penetrate beyond the first few meters at the surface. Smith et al. (1999) measured extinction coefficients for PAR, UV-A, and UV-B in the western and central basins of Lake Erie. Based on their median extinction coefficients for each basin, PAR is attenuated to 1% of its surface intensity by 5.3 and 6.8 m (western and central basins, respectively), UV-A by 2.0 and 2.7 m, and UV-B by 1.1 and 1.5 m below the surface. Because photo-reduction of Mn oxides is known to occur even in the absence of UV wavelengths (Sunda et al., 1983), our estimates of net Mn oxidation do not reflect rapid cycling of Mn redox states that may occur in the lake as a result of photo-reduction (Sunda and Huntsman, 1994). Moreover, photo-reduction of Mn oxides in Lake Erie could allow the persistence of dissolved Mn even under oxic conditions and regions of the lake where hypoxia does not typically occur (e.g., the western basin). Determining the effect of photo reduction on Mn cycling and distribution in the lake will ultimately require further experiments that explicitly test the effects of UV at intensities expected in the water of Lake Erie.
Relative Contribution of Biotic and Abiotic Mechanisms
Previous work in freshwater has suggested that the majority of Mn(II) oxidation is attributable to biological processes (Chapnick et al., 1982; Tipping, 1984; Aguilar and Nealson, 1998). While we did find evidence for biological oxidation of Mn (Hypothesis 3), our experiments suggest that abiotic mechanisms involving mineral surfaces also play an important role. In the western basin experiments, biological activity was responsible for 64% of the Mn oxides produced in the dark and 32% of the Mn oxides produced in the light, with the remainder being attributable to mineral surface interactions [(control – killed)/(control – filtered)]. It is important to note, however, that these proportions of biotic and abiotic contribution are based on net Mn oxide formation. Because the effect of hydrogen peroxide acting as a reductant of Mn oxides was evident in all four experiments, this means that gross Mn oxide formation rates were potentially much higher and thus the contribution of biological processes to gross Mn oxidation could be different.
Effect of Lake Basin on Mn Oxidation
Mn oxide concentrations and Mn oxide production rates from the western basin were much higher than those measured in the central basin. Maximum Mn oxide concentration after 7 days in the control + light treatment was 0.13 μmoles L−1 in the central basin but 3.4 μmoles L−1 in the western basin. Rates of Mn oxidation in the central basin were undetectable over 7 days, except for the light treatments including catalase (Figures 2A,B). Our results suggest that under the fastest rates in the western basin, about 90% of the added Mn(II) was converted to Mn(IV) in a week, but previous work has shown that the oxide minerals produced are a mix of Mn(II,III,IV) and thus the 90% value is likely an underestimate of the total removal of reduced Mn (Tipping, 1984). The dramatic differences in Mn oxidation between the western and central basins could reflect differences in suspended sediment and biological productivity between these two areas of the lake (Shuchman et al., 2013). Temperature can also have an effect on rates of Mn oxidation (Hem, 1981; Tipping, 1984), but the difference in temperature between the central (14–16°C) and western basins (19–25°C) is inadequate to explain the lack of measurable Mn oxidation in the central basin.
Products of Mn Oxidation
The Mn oxide minerals produced in our experiments were analyzed for morphology via transmission electron microscopy (TEM). TEM images collected from both natural and experimental samples show particles that contain Mn (confirmed with Energy-dispersive X-ray spectroscopy, EDS, Supplementary Figure S1) with fiber like morphology (Figure 4). Samples without Mn(II) amendments or relatively high concentration of Mn(III,VI) (measured with LBB) did not contain particles with this morphology. The morphology documented here is similar to biogenic phylomanganate mineral birnessite, which has been seen with previous laboratory studies (Villalobos et al., 2003; Tebo et al., 2004; Learman et al., 2011b). Further, birnessite is known for its autocatalytic properties (Coughlin and Matsui, 1976; Bargar et al., 2005; Learman et al., 2011b), thus provides possible support for mineral surface oxidation of Mn(II) (H2) in Lake Erie. The characteristic form of this mineral suggests that it should be readily removed by filtration. At the end of each experiment, we measured the Mn oxide concentration in the unfiltered and filtered (<0.2 μm) fractions from each treatment. In every case, filtration of the water resulted in undetectable concentration of Mn oxides. For those experimental units with detectable Mn oxide production in whole water (n = 55), the median removal by filtration was 99.3%.
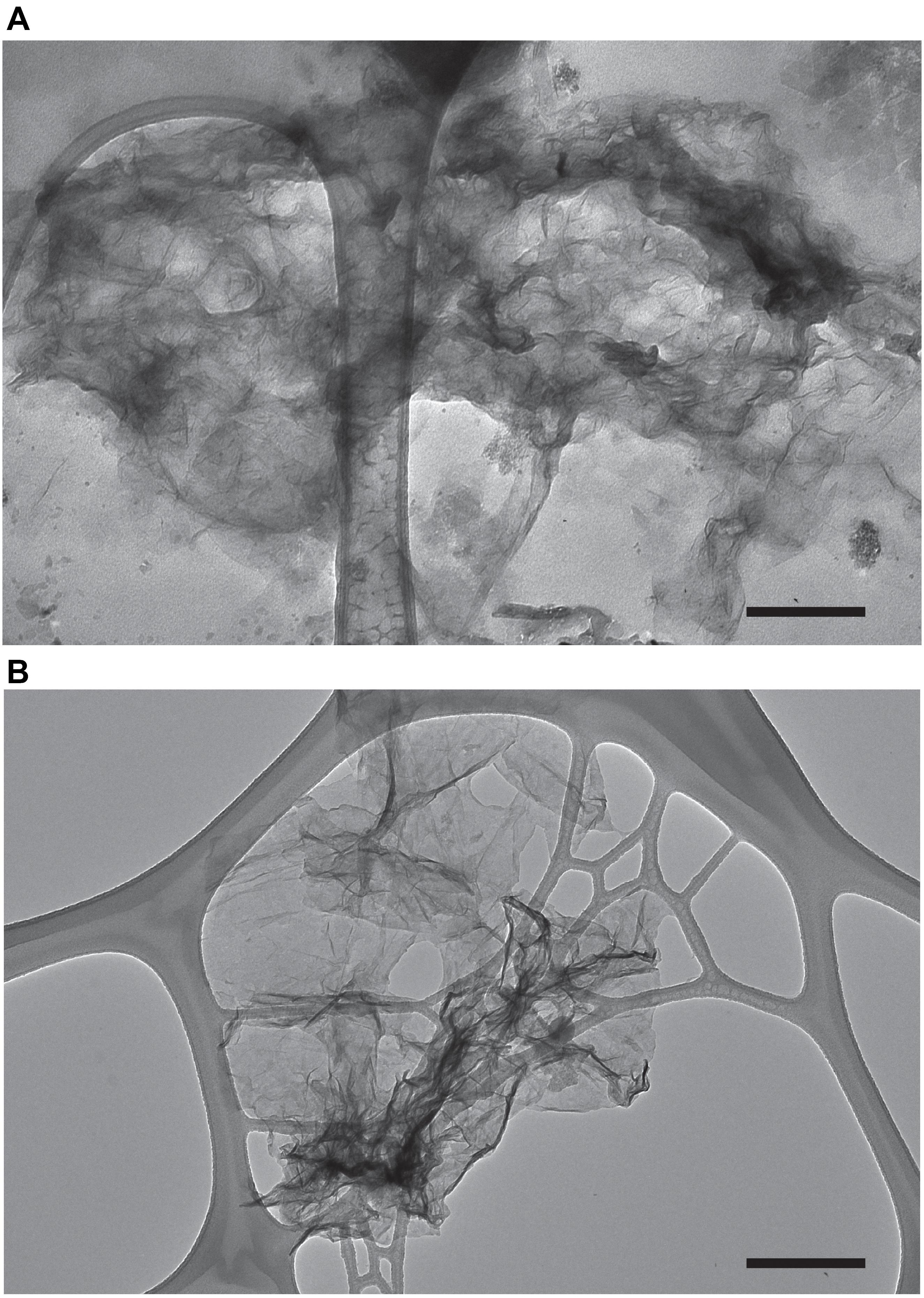
Figure 4. Transmission electron microscopy images of Mn oxides produced under experimental conditions (water from the western basin amended with Mn and catalase) (A) and water from the hypoxic hypolimnion of Lake Erie (central basin) (B). The scale bar (black bar) is 250 nm.
Dynamics of Mn Cycling in Lake Erie
Taken together, this study shows that the cycling of Mn in the surface mixed layer is impacted by both biotic and abiotic mechanisms and the importance of these reactions varies substantially between contrasting basins of the lake. Our study suggests that Mn(II) in Lake Erie will not be oxidized by dissolved oxygen unless this reaction is catalyzed by a biotic or abiotic catalyst, particularly mineral surfaces and heterotrophic plankton. Light also has a strong effect on oxidation, and this is not solely due to the production of ROS or photosynthetic organisms. Our study showed that both superoxide and hydrogen peroxide impact cycling of Mn in Lake Erie, but their combined net effect was highly variable and warrants further study. Lastly, the difference in Mn oxidation rate between the western and eastern basin helps underscore the importance of biological processes and suspended sediments as controls on the fate of Mn once it is released during hypoxia in the central basin. Additional studies examining the effects of UV exposure, in situ metabolism, and hydrodynamic mixing are required to determine the relative impact of these mechanisms in the lake.
Implications for Lake Erie Water Quality
Seasonal hypoxia is largely a phenomenon of the central basin, which is deep enough to stably stratify during the summer but typically has a thin hypolimnion, which becomes anoxic by mid-summer due to sediment oxygen demand (Rowe et al., 2019). Recently, it has been documented that Mn(II) is being released from the sediments in the central basin during these hypoxic events (Ruberg et al., 2008). Thus, the region of the lake experiencing accumulation of Mn(II) in the water (central basin) is also the region with slowest rates of removal (via natural oxidation). The low rates of Mn oxidation in the central basin are important because they mean that when Mn-laden hypoxic water mixes with the surface layer (via diffusion or upwelling), Mn(II) can persist for weeks or longer. While there are likely seasonal patterns in Mn oxidation rate in the central basin, our experiments suggest that co-location of Mn(II) release due to hypoxia and low Mn oxidation rates make the central basin particularly susceptible to accumulation of Mn during stratification.
While a lake-wide survey or budget will be required to quantify patterns of Mn accumulation in relation to hypoxia and removal by oxidation, our results have at least three implications for drinking water intakes. First, our experiment shows that biogeochemical processes leading to net oxidation of Mn are an important control on its fate in Lake Erie. When Mn is eventually oxidized to form birnessite, it will either sink to the sediments or be readily removed by filtration during water treatment. While the problem of elevated dissolved Mn in Lake Erie is ultimately attributable to release of Mn from sediments under hypoxia (∼50 μmoles m–2 d−1, Adams et al., 1982), the low rates of Mn oxidation even under oxic conditions may serve to exacerbate this problem. Second, the low rates of Mn oxidation under oxic conditions present in the surface mixed layer of the central basin mean that dissolved oxygen concentration is an imperfect proxy for whether dissolved Mn(II) will be present. Based on our experiments, a more reliable prediction for potential Mn oxidation and removal from the water would consider suspended sediments, plankton biomass, and light. Third, although homogenous oxidation by dissolved oxygen is not among the mechanisms supported by our experiments, several of those other mechanisms do require dissolved oxygen as a reactant. Indeed, other studies have shown that Mn oxidation rates are sensitive to dissolved oxygen concentration (Tebo and Emerson, 1985; Tebo, 1991; Hsiung and Tisue, 1994). The dependence of these mechanisms on dissolved oxygen concentration means that oxidation rates in the hypoxic (i.e., <2 mg L−1 dissolved oxygen) areas of central basin are likely even lower than we measured. As a result, the accumulation of Mn(II) in the hypolimnion may potentially begin even before the onset of anoxia (Munger et al., 2019). These findings underscores the importance of understanding the mechanisms of Mn oxidation in order to predict the fate of Mn in freshwater systems.
Data Availability Statement
The complete dataset is provided in the Supplementary Material.
Author Contributions
JZ executed the experiments. DL performed the electron microscopy. CG wrote the computer code and drafted the figures. All authors contributed to the experimental design, analysis of data, and writing the manuscript.
Funding
This work was supported by the National Oceanic and Atmospheric Administration’s National Centers for Coastal Ocean Science Competitive Research Program under award NA16NOS4780209 to the University of Michigan and through the NOAA Cooperative Agreement with the Cooperative Institute for Great Lakes Research (CIGLR) at the University of Michigan (NA17OAR4320152).
Conflict of Interest
The authors declare that the research was conducted in the absence of any commercial or financial relationships that could be construed as a potential conflict of interest.
Acknowledgments
JZ was supported through the Cooperative Institute for Great Lakes Research Summer Fellows Program. This is CIGLR contribution #1159, CHRP contribution #245, and contribution #141 of the Central Michigan University Institute for Great Lakes Research.
Supplementary Material
The Supplementary Material for this article can be found online at: https://www.frontiersin.org/articles/10.3389/fenvs.2020.00057/full#supplementary-material
FIGURE S1 | EDS spectra collected on fiber like material produced under experimental conditions using water from the western basin amended with Mn and catalase (left) and water from the hypoxic hypolimnion of Lake Erie’s central basin (right). High Cu peaks are due to the copper grids used to prepare the samples for TEM.
TABLE S1 | Summary data for each experimental unit.
TABLE S2 | The complete experimental dataset.
References
Adams, D. D., Matisoff, G., and Snodgrass, W. J. (1982). Flux of reduced chemical constituents (Fe 2+, Mn 2+, NH4 + and CH4) and sediment oxygen demand in Lake Erie. Hydrobiologia 91, 405–414.
Aguilar, C., and Nealson, K. H. (1998). Biogeochemical cycling of manganese in Oneida Lake, New York: whole lake studies of manganese. J. Great Lakes Res. 24, 93–104. doi: 10.1016/s0380-1330(98)70802-0
Allard, S., Gutierrez, L., Fontaine, C., Croué, J.-P., and Gallard, H. (2017). Organic matter interactions with natural manganese oxide and synthetic birnessite. Sci. Total Environ. 583, 487–495. doi: 10.1016/j.scitotenv.2017.01.120
Andeer, P. F., Learman, D. R., Mcilvin, M., Dunn, J. A., and Hansel, C. M. (2015). Extracellular haem peroxidases mediate Mn(II) oxidation in a marine Roseobacter bacterium via superoxide production. Environ. Microbiol. 17, 3925–3936. doi: 10.1111/1462-2920.12893
Anderson, C., Davis, R., Bandolin, N., Baptista, A., and Tebo, B. (2011). Analysis of in situ manganese (II) oxidation in the Columbia river and offshore plume: linking Aurantimonas and the associated microbial community to an active biogeochemical cycle. Environ. Microbiol. 13, 1561–1576. doi: 10.1111/j.1462-2920.2011.02462.x
Anderson, C., Johnson, H., Caputo, N., Davis, R., Torpey, J., and Tebo, B. M. (2009). Mn (II) oxidation is catalyzed by heme peroxidases in “Aurantimonas manganoxydans” strain SI85-9A1 and Erythrobacter sp. strain SD-21. Appl. Environ. Microbiol. 75, 4130–4138. doi: 10.1128/AEM.02890-08
Archibald, F. S., and Fridovich, I. (1982). The scavenging of superoxide radical by manganous complexes: in vitro. Arch. Biochem. Biophys. 214, 452–463. doi: 10.1016/0003-9861(82)90049-2
Bargar, J. R., Tebo, B. M., Bergmann, U., Webb, S. M., Glatzel, P., Chiu, V. Q., et al. (2005). Biotic and abiotic products of Mn(II) oxidation by spores of the marine Bacillus sp. strain SG-1. Am. Mineral. 90, 143–154. doi: 10.2138/am.2005.1557
Beletsky, D., Hawley, N., Rao, Y. R., Vanderploeg, H. A., Beletsky, R., Schwab, D. J., et al. (2012). Summer thermal structure and anticyclonic circulation of Lake Erie. Geophys. Res. Lett. 39:6605. doi: 10.1029/2012gl051002
Bertani, I., Obenour, D. R., Steger, C. E., Stow, C. A., Gronewold, A. D., and Scavia, D. (2016). Probabilistically assessing the role of nutrient loading in harmful algal bloom formation in western Lake Erie. J. Great Lakes Res. 42, 1184–1192. doi: 10.1016/j.jglr.2016.04.002
Bertino, D. J., and Zepp, R. G. (1991). Effects of solar radiation on manganese oxide reactions with selected organic compounds. Environ. Sci. Technol. 25, 1267–1273.
Binding, C., Greenberg, T., and Bukata, R. (2012). An analysis of MODIS-derived algal and mineral turbidity in Lake Erie. J. Great Lakes Res. 38, 107–116.
Boogerd, F., and De Vrind, J. (1987). Manganese oxidation by Leptothrix discophora. J. Bacteriol. 169, 489–494.
Bouchard, M. F., Sauve, S., Barbeau, B., Legrand, M., Brodeur, M. E., Bouffard, T., et al. (2011). Intellectual impairment in school-age children exposed to manganese from drinking water. Environ. Health Perspect. 119, 138–143. doi: 10.1289/ehp.1002321
Burns, N., and Nriagu, J. (1976). Forms of iron and manganese in Lake Erie waters. J. Fish. Board Canada 33, 463–470. doi: 10.1007/BF00401331
Butterfield, C. N., Soldatova, A. V., Lee, S.-W., Spiro, T. G., and Tebo, B. M. (2013). Mn (II, III) oxidation and MnO2 mineralization by an expressed bacterial multicopper oxidase. Proc. Natl. Acad. Sci. U.S.A. 110, 11731–11735. doi: 10.1073/pnas.1303677110
Carmichael, M. J., Carmichael, S. K., Santelli, C. M., Strom, A., and Bräuer, S. L. (2013). Mn (II)-oxidizing bacteria are abundant and environmentally relevant members of ferromanganese deposits in caves of the upper Tennessee river basin. Geomicrobiol. J. 30, 779–800.
Chapnick, S. D., Moore, W. S., and Nealson, K. H. (1982). Microbially mediated manganese oxidation in a freshwater lake. Limnol. Oceanogr. 27, 1004–1014.
Chaput, D. L., Fowler, A. J., Seo, O., Duhn, K., Hansel, C. M., and Santelli, C. M. (2019). Mn oxide formation by phototrophs: spatial and temporal patterns, with evidence of an enzymatic superoxide-mediated pathway. Sci. Rep. 9:18244. doi: 10.1038/s41598-019-54403-8
Cory, R. M., Davis, T. W., Dick, G. J., Johengen, T., Denef, V. J., Berry, M. A., et al. (2016). Seasonal dynamics in dissolved organic matter, hydrogen peroxide, and cyanobacterial blooms in Lake Erie. Front. Mar. Sci. 3:54. doi: 10.3389/fmars.2016.00054
Coughlin, R. W., and Matsui, I. (1976). Catalytic oxidation of aqueous Mn (II). J. Catal. 41, 108–123. doi: 10.1016/j.watres.2017.10.045
Daly, M. J., Gaidamakova, E. K., Matrosova, V., Vasilenko, A., Zhai, M., Venkateswaran, A., et al. (2004). Accumulation of Mn (II) in Deinococcus radiodurans facilitates gamma-radiation resistance. Science 306, 1025–1028. doi: 10.1126/science.1103185
Davies, S. H., and Morgan, J. J. (1989). Manganese (II) oxidation kinetics on metal oxide surfaces. J. Colloid Interface Sci. 129, 63–77.
Delfino, J. J., and Lee, G. F. (1968). Chemistry of manganese in Lake Mendota, Wisconsin. Environ. Sci. Technol. 2, 1094–1100.
Dick, G. J., Torpey, J. W., Beveridge, T. J., and Tebo, B. M. (2008). Direct identification of a bacterial manganese(II) oxidase, the multicopper oxidase MnxG, from spores of several different marine Bacillus species. Appl. Environ. Microbiol. 74, 1527–1534. doi: 10.1128/AEM.01240-07
Diem, D., and Stumm, W. (1984). Is dissolved Mn2+ being oxidized by O2 in absence of Mn-bacteria or surface catalysts? Geochim. Cosmochim. Acta 48, 1571–1573. doi: 10.3390/s18093144
Dixon, T. C., Vermilyea, A. W., Scott, D. T., and Voelker, B. M. (2013). Hydrogen peroxide dynamics in an agricultural headwater stream: evidence for significant nonphotochemical production. Limnol. Oceanogr. 58, 2133–2144.
Duckworth, O. W., and Sposito, G. (2005). Siderophore- manganese (III) interactions. I. Air-oxidation of manganese (II) promoted by desferrioxamine B. Environ. Sci. Technol. 39, 6037–6044. doi: 10.1021/es050275k
Gantzer, P. A., Bryant, L. D., and Little, J. C. (2009). Controlling soluble iron and manganese in a water-supply reservoir using hypolimnetic oxygenation. Water Res. 43, 1285–1294. doi: 10.1016/j.watres.2008.12.019
Gerling, A. B., Browne, R. G., Gantzer, P. A., Mobley, M. H., Little, J. C., and Carey, C. C. (2014). First report of the successful operation of a side stream supersaturation hypolimnetic oxygenation system in a eutrophic, shallow reservoir. Water Res. 67, 129–143. doi: 10.1016/j.watres.2014.09.002
Geszvain, K., Yamaguchi, A., Maybee, J., and Tebo, B. M. (2011). Mn (II) oxidation in Pseudomonas putida GB-1 is influenced by flagella synthesis and surface substrate. Arch. Microbiol. 193, 605–614. doi: 10.1007/s00203-011-0702-0
Hansard, S. P., Easter, H. D., and Voelker, B. M. (2011). Rapid reaction of nanomolar Mn(II) with superoxide radical in seawater and simulated freshwater. Environ. Sci. Technol. 45, 2811–2817. doi: 10.1021/es104014s
Hansel, C. M. (2017). Manganese in marine microbiology. Adv. Microb. Physiol. 70, 37–83. doi: 10.1016/bs.ampbs.2017.01.005
Hansel, C. M., and Learman, D. R. (2015). “Geomicrobiology of manganese,” in Ehrlich’s Geomicrobiology, eds H. L. Ehrlich, D. K. Newman, and A. Kappler (New York, NY: CRC Press), 401–452.
Hansel, C. M., Zeiner, C. A., Santelli, C. M., and Webb, S. M. (2012). Mn(II) oxidation by an ascomycete fungus is linked to superoxide production during asexual reproduction. Proc. Natl. Acad. Sci. U.S.A. 109, 12621–12625. doi: 10.1073/pnas.1203885109
Hem, J. D. (1981). Rates of manganese oxidation in aqueous systems. Geochim. Cosmochim. Acta 45, 1369–1374.
Hongve, D. (1997). Cycling of iron, manganese, and phosphate in a meromictic lake. Limnol. Oceanogr. 42, 635–647.
Hsiung, T.-M., and Tisue, T. (1994). “Manganese dynamics in Lake Richard B. Russell,” in Environmental Chemistry of Lakes and Reservoirs, ed. L. A. Baker (Washington, DC: American Chemical Society), 499–524.
Junta, J. L., and Hochella, M. F. (1994). Manganese (II) oxidation at mineral surfaces: a microscopic and spectroscopic study. Geochim. Cosmochim. Acta 58, 4985–4999.
Khan, K., Wasserman, G. A., Liu, X., Ahmed, E., Parvez, F., Slavkovich, V., et al. (2012). Manganese exposure from drinking water and children’s academic achievement. Neurotoxicology 33, 91–97. doi: 10.1016/j.neuro.2011.12.002
Krumbein, W., and Altmann, H. (1973). A new method for the detection and enumeration of manganese oxidizing and reducing microorganisms. Helgoländer Wiss. Meeresunters. 25, 347–356.
Learman, D. R., Voelker, B. M., Madden, A. S., and Hansel, C. M. (2013). Constraints on superoxide mediated formation of manganese oxides. Front. Microbiol. 4:262. doi: 10.3389/fmicb.2013.00262
Learman, D. R., Voelker, B. M., Vazquez-Rodriguez, A. I., and Hansel, C. M. (2011a). Formation of manganese oxides by bacterially generated superoxide. Nat. Geosci. 4, 95–98. doi: 10.1038/ngeo1055
Learman, D. R., Wankel, S. D., Webb, S. M., Martinez, N., Madden, A. S., and Hansel, C. M. (2011b). Coupled biotic–abiotic Mn(II) oxidation pathway mediates the formation and structural evolution of biogenic Mn oxides. Geochim. Cosmochim. Acta 75, 6048–6063. doi: 10.1016/j.gca.2011.07.026
Lee, Y., and Tebo, B. M. (1994). Cobalt (II) oxidation by the marine manganese (II)-oxidizing Bacillus sp. strain SG-1. Appl. Environ. Microbiol. 60, 2949–2957.
Lum, K. R., and Leslie, J. K. (1983). Dissolved and particulate metal chemistry of the central and eastern basins of Lake Erie. Sci. Total Environ. 30, 99–109.
Madden, A. S., and Hochella, M. F. (2005). A test of geochemical reactivity as a function of mineral size: manganese oxidation promoted by hematite nanoparticles. Geochim. Cosmochim. Acta 69, 389–398.
Madison, A. S., Tebo, B. M., Mucci, A., Sundby, B., and Luther, G. W. (2013). Abundant porewater Mn(III) is a major component of the sedimentary redox system. Science 341, 875–878. doi: 10.1126/science.1241396
Marsico, R. M., Schneider, R. J., Voelker, B. M., Zhang, T., Diaz, J. M., Hansel, C. M., et al. (2015). Spatial and temporal variability of widespread dark production and decay of hydrogen peroxide in freshwater. Aquat. Sci. 77, 523–533.
Miyata, N., Tani, Y., Maruo, K., Tsuno, H., Sakata, M., and Iwahori, K. (2006). Manganese (IV) oxide production by Acremonium sp. strain KR21-2 and extracellular Mn (II) oxidase activity. Appl. Environ. Microbiol. 72, 6467–6473. doi: 10.1128/AEM.00417-06
Morris, D. P., Zagarese, H., Williamson, C. E., Balseiro, E. G., Hargreaves, B. R., Modenutti, B., et al. (1995). The attenuation of solar UV radiation in lakes and the role of dissolved organic carbon. Limnol. Oceanogr. 40, 1381–1391.
Mortimer, C. H. (1971). Chemical exchanges between sediments and water in the great lakes-speculations on probable regulatory mechanisms 1. Limnol. Oceanogr. 16, 387–404.
Mudroch, A. (1984). Chemistry, mineralogy, and morphology of Lake Erie suspended matter. J. Great Lakes Res. 10, 286–298.
Munger, Z. W., Carey, C. C., Gerling, A. B., Doubek, J. P., Hamre, K. D., Mcclure, R. P., et al. (2019). Oxygenation and hydrologic controls on iron and manganese mass budgets in a drinking-water reservoir. Lake Reserv. Manag. 35, 277–291.
Munger, Z. W., Carey, C. C., Gerling, A. B., Hamre, K. D., Doubek, J. P., Klepatzki, S. D., et al. (2016). Effectiveness of hypolimnetic oxygenation for preventing accumulation of Fe and Mn in a drinking water reservoir. Water Res. 106, 1–14. doi: 10.1016/j.watres.2016.09.038
Munger, Z. W., Shahady, T. D., and Schreiber, M. E. (2017). Effects of reservoir stratification and watershed hydrology on manganese and iron in a dam-regulated river. Hydrol. Process. 31, 1622–1635.
Nakama, K., Medina, M., Lien, A., Ruggieri, J., Collins, K., and Johnson, H. A. (2014). Heterologous expression and characterization of the manganese-oxidizing protein from Erythrobacter sp. strain SD21. Appl. Environ. Microbiol. 80, 6837–6842. doi: 10.1128/AEM.01873-14
Nealson, K. H., and Saffarini, D. (1994). Iron and manganese in anaerobic respiration: environmental significance, physiology, and regulation. Annu. Rev. Microbiol. 48, 311–343. doi: 10.1146/annurev.mi.48.100194.001523
Nealson, K. H., Tebo, B. M., and Rosson, R. A. (1988). Occurrence and mechanisms of microbial oxidation of manganese. Adv. Appl. Microbiol. 33, 279–318. doi: 10.1111/j.1751-7915.2011.00323.x
Nico, P. S., Anastasio, C., and Zasoski, R. J. (2002). Rapid photo-oxidation of Mn (II) mediated by humic substances. Geochim. Cosmochim. Acta 66, 4047–4056.
Oldham, V. E., Mucci, A., Tebo, B. M., and Luther, G. W. (2017). Soluble Mn(III)–L complexes are abundant in oxygenated waters and stabilized by humic ligands. Geochim. Cosmochim. Acta 199, 238–246. doi: 10.1016/j.gca.2016.11.043
Oldham, V. E., Siebecker, M. G., Jones, M. R., Mucci, A., Tebo, B. M., and Luther, G. W. (2019). The speciation and mobility of Mn and Fe in estuarine sediments. Aquat. Geochem. 25, 3–26. doi: 10.1016/j.jhazmat.2010.12.081
Ossa Ossa, F., Hofmann, A., Wille, M., Spangenberg, J. E., Bekker, A., Poulton, S. W., et al. (2018). Aerobic iron and manganese cycling in a redox-stratified Mesoarchean epicontinental sea. Earth Planet. Sci. Lett. 500, 28–40. doi: 10.1016/j.epsl.2018.07.044
Painter, S., Marvin, C., Rosa, F., Reynoldson, T. B., Charlton, M. N., Fox, M., et al. (2001). Sediment contamination in Lake Erie: a 25-year retrospective analysis. J. Great Lakes Res. 27, 434–448.
Post, J. E. (1999). Manganese oxide minerals: crystal structures and economic and environmental significance. Proc. Natl. Acad. Sci. U.S.A. 96, 3447–3454. doi: 10.1073/pnas.96.7.3447
Prater, C., Frost, P. C., Howell, E. T., Watson, S. B., Zastepa, A., King, S. S. E., et al. (2017). Variation in particulate C:N:P stoichiometry across the Lake Erie watershed from tributaries to its outflow. Limnol. Oceanogr. 62, S194–S206. doi: 10.1002/lno.10628
Richardson, L. L., Aguilar, C., and Nealson, K. H. (1988). Manganese oxidation in pH and O2 microenvironments produced by phytoplankton. Limnol. Oceanogr. 33, 352–363. doi: 10.4319/lo.1988.33.3.0352
Rowe, M. D., Anderson, E. J., Beletsky, D., Stow, C. A., Moegling, S. D., Chaffin, J. D., et al. (2019). Coastal upwelling influences hypoxia spatial patterns and nearshore dynamics in Lake Erie. J. Geophys. Res. Oceans 124, 6154–6175. doi: 10.1029/2019jc015192
Ruberg, S. A., Guasp, E., Hawley, N., Muzzi, R. W., Brandt, S. B., Vanderploeg, H. A., et al. (2008). Societal benefits of the real-time coastal observation network (RECON): implications for municipal drinking water quality. Mar. Technol. Soc. J. 42, 103–109.
Scavia, D., David Allan, J., Arend, K. K., Bartell, S., Beletsky, D., Bosch, N. S., et al. (2014). Assessing and addressing the re-eutrophication of Lake Erie: central basin hypoxia. J. Great Lakes Res. 40, 226–246. doi: 10.1016/j.jglr.2014.02.004
Scavia, D., Depinto, J. V., and Bertani, I. (2016). A multi-model approach to evaluating target phosphorus loads for Lake Erie. J. Great Lakes Res. 42, 1139–1150. doi: 10.1016/j.jglr.2016.09.007
Sherman, D. M. (2005). Electronic structures of iron (III) and manganese (IV)(hydr) oxide minerals: thermodynamics of photochemical reductive dissolution in aquatic environments. Geochim. Cosmochim. Acta 69, 3249–3255.
Shuchman, R. A., Leshkevich, G., Sayers, M. J., Johengen, T. H., Brooks, C. N., and Pozdnyakov, D. (2013). An algorithm to retrieve chlorophyll, dissolved organic carbon, and suspended minerals from Great Lakes satellite data. J. Great Lakes Res. 39, 14–33. doi: 10.1016/j.jglr.2013.06.017
Smith, R., Furgal, J., Charlton, M., Greenberg, B., Hiriart, V., and Marwood, C. (1999). Attenuation of ultraviolet radiation in a large lake with low dissolved organic matter concentrations. Can. J. Fish. Aquat. Sci. 56, 1351–1361.
Spiro, T. G., Bargar, J. R., Sposito, G., and Tebo, B. M. (2009). Bacteriogenic manganese oxides. Acc. Chem. Res. 43, 2–9.
Stumm, W., and Morgan, J. J. (1996). Aquatic Chemistry: Chemical Equilibrium and Rates in Natural Waters. New York, NY: Wiley.
Sunda, W., Huntsman, S., and Harvey, G. (1983). Photoreduction of manganese oxides in seawater and its geochemical and biological implications. Nature 301, 234–236.
Sunda, W. G., and Huntsman, S. A. (1987). Microbial oxidation of manganese in a North Carolina estuary. Limnol. Oceanogr. 32, 552–564.
Sunda, W. G., and Huntsman, S. A. (1988). Effect of sunlight on redox cycles of manganese in the southwestern Sargasso Sea. Deep Sea Res. Part A Oceanogr. Res. Pap. 35, 1297–1317.
Sunda, W. G., and Huntsman, S. A. (1990). Diel cycles in microbial manganese oxidation and manganese redox speciation in coastal waters of the Bahama Islands. Limnol. Oceanogr. 35, 325–338.
Sunda, W. G., and Huntsman, S. A. (1994). Photoreduction of manganese oxides in seawater. Mar. Chem. 46, 133–152.
Tebo, B. M. (1991). Manganese (II) oxidation in the suboxic zone of the Black Sea. Deep Sea Res. Part A Oceanogr. Res. Pap. 38, S883–S905.
Tebo, B. M., Bargar, J. R., Clement, B. G., Dick, G. J., Murray, K. J., Parker, D., et al. (2004). Biogenic manganese oxides: properties and mechanisms of formation. Annu. Rev. Earth Planet. Sci. 32, 287–328. doi: 10.1146/annurev.earth.32.101802.120213
Tebo, B. M., and Emerson, S. (1985). Effect of oxygen tension, Mn (II) concentration, and temperature on the microbially catalyzed Mn (II) oxidation rate in a marine fjord. Appl. Environ. Microbiol. 50, 1268–1273.
Tebo, B. M., Johnson, H. A., Mccarthy, J. K., and Templeton, A. S. (2005). Geomicrobiology of manganese(II) oxidation. Trends Microbiol. 13, 421–428. doi: 10.1016/j.tim.2005.07.009
Tipping, E. (1984). Temperature dependence of Mn (II) oxidation in lakewaters: a test of biological involvement. Geochim. Cosmochim. Acta 48, 1353–1356.
Tipping, E., Thompson, D., and Davison, W. (1984). Oxidation products of Mn (II) in lake waters. Chem. Geol. 44, 359–383.
United States Environmental Protection Agency (1996). National Secondary Drinking Water Regulations. Washington, DC: United States Environmental Protection Agency.
Villalobos, M., Toner, B., Bargar, J., and Sposito, G. (2003). Characterization of the manganese oxide produced by Pseudomonas putida strain MnB1. Geochim. Cosmochim. Acta 67, 2649–2662.
Weiskerger, C. J., Rowe, M. D., Stow, C. A., Stuart, D., and Johengen, T. (2018). Application of the Beer–Lambert model to attenuation of photosynthetically active radiation in a shallow, eutrophic lake. Water Resour. Res. 54, 8952–8962. doi: 10.1029/2018wr023024
Wuttig, K., Heller, M. I., and Croot, P. L. (2013). Pathways of superoxide (O2-) decay in the eastern tropical north Atlantic. Environ. Sci. Technol. 47, 10249–10256. doi: 10.1021/es401658t
Yakushev, E., Pakhomova, S., Sørenson, K., and Skei, J. (2009). Importance of the different manganese species in the formation of water column redox zones: observations and modeling. Mar. Chem. 117, 59–70.
Yao, W., and Millero, F. J. (1996). Adsorption of phosphate on manganese dioxide in seawater. Environ. Sci. Technol. 30, 536–541.
Zaw, M., and Chiswell, B. (1999). Iron and manganese dynamics in lake water. Water Res. 33, 1900–1910.
Zhou, Y., Michalak, A. M., Beletsky, D., Rao, Y. R., and Richards, R. P. (2015). Record-breaking Lake Erie hypoxia during 2012 drought. Environ. Sci. Technol. 49, 800–807. doi: 10.1021/es503981n
Keywords: manganese cycling, Mn(II), reactive oxygen species, Lake Erie, manganese oxidation
Citation: Godwin CM, Zehnpfennig JR and Learman DR (2020) Biotic and Abiotic Mechanisms of Manganese (II) Oxidation in Lake Erie. Front. Environ. Sci. 8:57. doi: 10.3389/fenvs.2020.00057
Received: 04 February 2020; Accepted: 27 April 2020;
Published: 21 May 2020.
Edited by:
Laodong Guo, University of Wisconsin–Milwaukee, United StatesReviewed by:
Frantz Ossa Ossa, University of Tübingen, GermanyShingo Kato, RIKEN BioResource Research Center (BRC), Japan
Copyright © 2020 Godwin, Zehnpfennig and Learman. This is an open-access article distributed under the terms of the Creative Commons Attribution License (CC BY). The use, distribution or reproduction in other forums is permitted, provided the original author(s) and the copyright owner(s) are credited and that the original publication in this journal is cited, in accordance with accepted academic practice. No use, distribution or reproduction is permitted which does not comply with these terms.
*Correspondence: Casey Michael Godwin, Y2dvZHdpbkB1bWljaC5lZHU=