- 1Cetacean Logic Foundation, Inc., New Smyrna Beach, FL, United States
- 2Florence Bascom Geoscience Center, United States Geological Survey, Reston, VA, United States
Determining hydrologic conditions prior to instrumental records is a challenge for restoration of freshwater ecosystems worldwide. Paleoecologic data provide this information on past conditions and when these data are used to adjust hydrologic models, allow conditions to be hindcast that may not be directly estimated from the paleo-data alone. In this context, the paleo-data provide real-world estimates as input to the models. Restoration of the Greater Everglades Ecosystem requires this understanding of the hydrology of the natural system prior to significant alterations due to water management and land use. Large scale models such as the Natural Systems Model (NSM 4.6.2) have been used by the South Florida Water Management District and other agencies responsible for restoration to estimate past hydrologic conditions; however, these models typically portray a drier natural system for the beginning of the 20th century than what is indicated by paleoecologic analyses and historical data. The purpose of this study is to estimate pre-20th century water levels, hydroperiods and flow in the freshwater wetlands of the Everglades by using pollen assemblage data in three sediment cores to adjust the Natural Systems Model. This study is designed to further test estimates of flow through the Everglades derived from analysis of sediment cores collected in Florida Bay. The results demonstrate that the NSM 4.6.2 underestimates water levels and hydroperiods in the Everglades compared to the paleo-adjusted NSM 4.6.2 model outputs. Flow models that use the paleo-adjusted water levels as input indicate flow through Shark River Slough in the late 19th century was approximately two times flow between 1990 and 2000, and flow through Taylor Slough was approximately three times flow between 1990 and 2000. The flow estimates derived from this study agree with the estimates derived from earlier studies using estuarine cores. This integration of paleoecologic information and hydrologic models provides resource managers with the best available estimates of past conditions and allows them to set realistic targets for restoration of freshwater ecosystems.
Introduction
The Greater Everglades Ecosystem in southern Florida, United States, is one of the largest freshwater wetlands in the continental United States (McPherson and Halley, 1996) and has been recognized as a Wetland of International Importance, an International Biosphere Reserve, and a World Heritage Site. The ecosystem includes the Kissimmee River basin in central Florida, Lake Okeechobee, the Everglades, and the estuaries surrounding southern Florida (Figure 1). Beginning in the early 20th century, the construction of water control features (canals, levees, etc.) profoundly altered the natural water delivery to the southern part of the ecosystem (referred to herein as “pre-drainage”), reducing the volume of freshwater flowing through the Everglades via Shark River Slough (SRS) and Taylor Slough into Florida Bay and the southwest coastal region (Van Lent et al., 1993; Light and Dineen, 1994; McPherson and Halley, 1996; Renken et al., 2005; Lodge, 2010; Figure 2). The reduced flow volumes impacted the downstream coastal system, raising salinities along the southwest coast of Florida and in Florida Bay. Recent estuarine studies linking paleoecologic data to hydrologic models (Marshall et al., 2009, 2011, 2014; Marshall and Wingard, 2012) estimated that the average SRS flows have been reduced to approximately one-half of the historic volumes and the average Taylor Slough flows to approximately one-third to one-fourth. The reduced flow volumes translate into reduced water depths and shortened hydroperiods (the number of days water is above ground each calendar year) in the freshwater wetlands compared to pre-water management conditions.
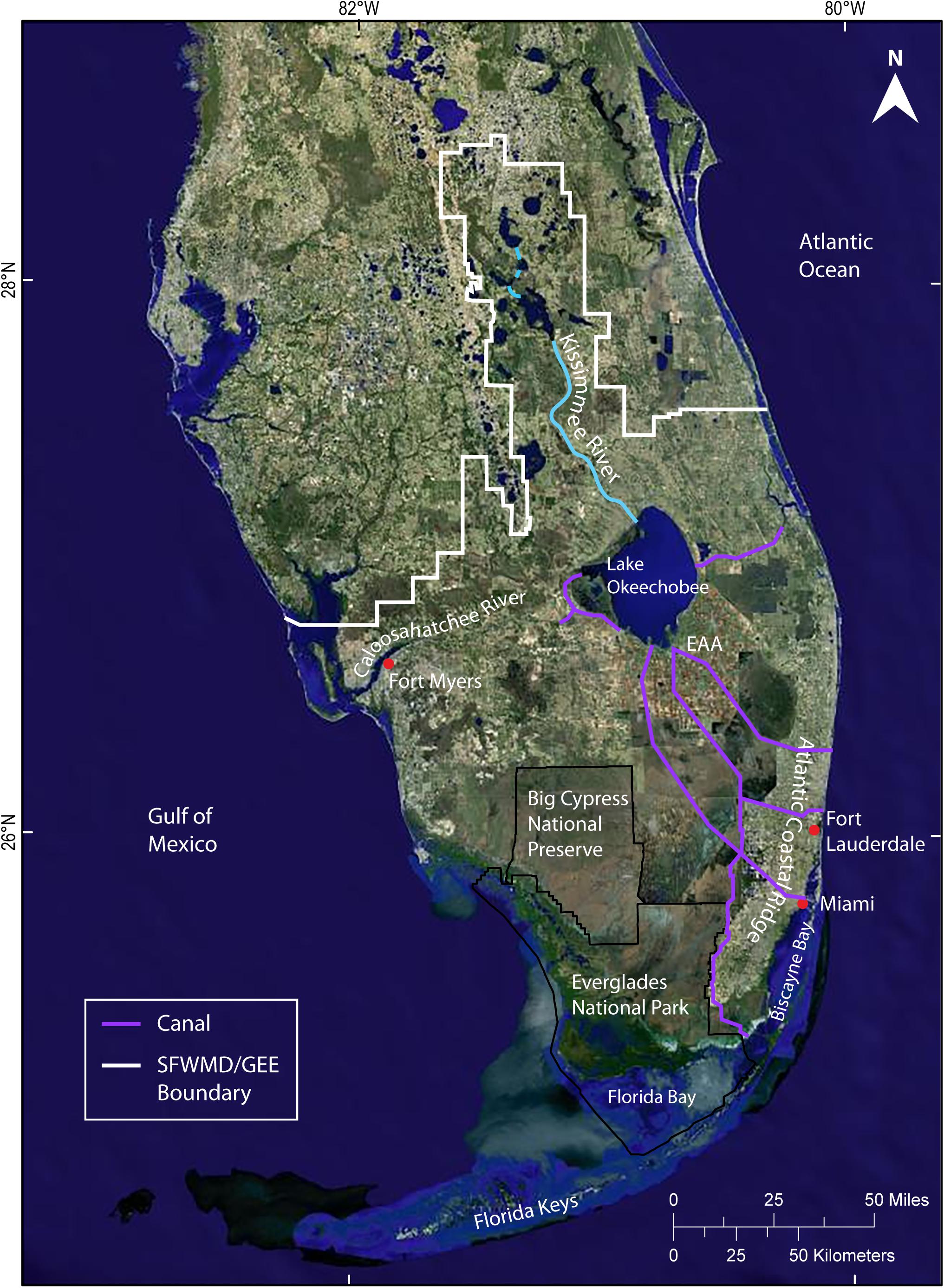
Figure 1. Satellite image showing key features of south Florida and the Greater Everglades Ecosystem (GEE). The extent of the natural Everglades Ecosystem closely corresponds to the current boundaries of the South Florida Water Management District (SFWMD). Location of sites and features within Everglades National Park are shown in Figure 2. EAA is the Everglades Agricultural Area, located south of Lake Okeechobee. Image from NaturalVue 15 m satellite imagery created by MDA Information Systems Inc. from Landsat-7 images.
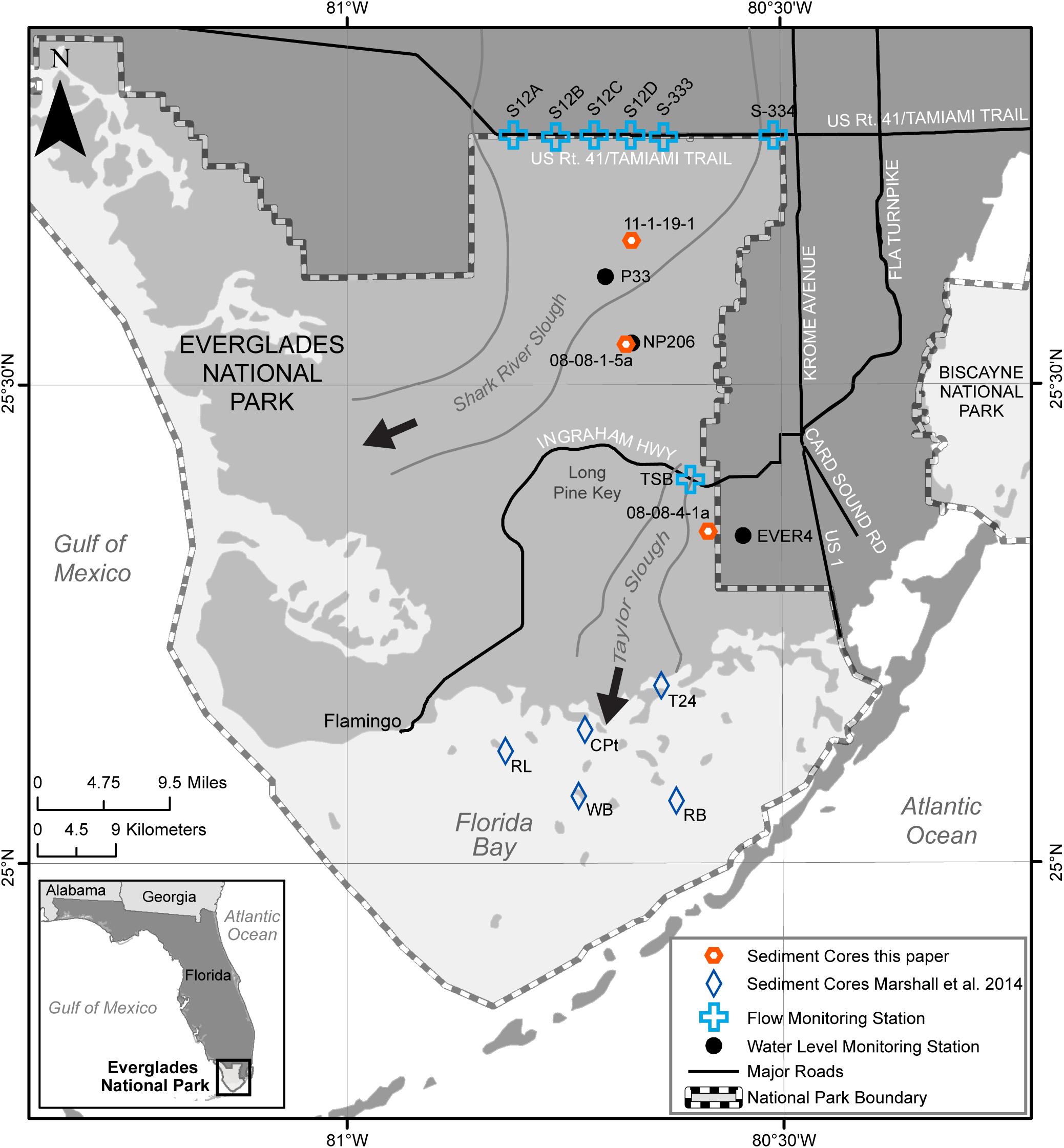
Figure 2. Map showing location of cores, water level and flow monitoring stations, and other relevant features in and around Everglades National Park. Geographic coordinates for cores and stations are given in Table 1.
Currently, much of the remnant natural freshwater wetlands and estuarine areas are located within ENP. In 2000, the US Congress adopted the CERP, a program that is designed to protect the remaining ecological integrity of the Everglades by restoring more natural hydrologic patterns. Restoration is considered a high priority for local, state and Federal government in part because of the national and international legal protections but also because the ecosystem services provided by the Everglades are an important part of south Florida’s economy (Johns et al., 2014). Everglades restoration is guided by the CERP and based on scientifically derived targets and goals for individual components of the ecosystem, but basic questions and uncertainties about the natural hydrologic conditions remain.
Previous authors have addressed the impacts of twentieth century changes to the hydrologic patterns and ecology of the Greater Everglades Ecosystem (for example, Light and Dineen, 1994; Davis et al., 2005; Sklar et al., 2005; McVoy et al., 2011), and conducted focused research on past hydrologic conditions for components of the ecosystem (for example, Smith et al., 1989; Willard et al., 2007). However, little work has been done to quantify past changes in hydrology across the ecosystem or to incorporate pre-twentieth century information into models. In this study we utilize pre-twentieth century paleoecologic information (pollen assemblages) from sediment cores in the freshwater wetlands of the Everglades to estimate water level for the circa 1900 CE time period. This information is incorporated into existing hydrologic models to provide simulated time-series and to estimate past flow conditions. The current research builds on previous work that estimated pre-water management flows and water levels in the freshwater wetlands based on paleoecologic salinity estimates in Florida Bay (Marshall et al., 2009, 2014; Marshall and Wingard, 2012; core locations shown in Figure 2) and it provides a means to further test the estuarine-derived estimates of upstream freshwater levels and flows. The results impart an increased understanding of the interconnected natural hydrologic conditions in the southern Everglades and a validation of previous results that can be used by resource managers for setting targets and realistic performance measures as directed by CERP for restoration of the remnant Everglades. The methodology outlined here can be used to adjust large scale hydrologic models to pre-alteration levels in any drainage system with the necessary data sets.
Materials and Methods
Regional Setting and Site Locations
The Natural and Altered Everglades Ecosystem
The natural Everglades ecosystem was primarily formed by a combination of long-term geologic and climatic factors including extremely low topographic relief, limestone bedrock underlying much of the system, climate, and seasonal hydrology (DeAngelis and White, 1994; McPherson and Halley, 1996; Lodge, 2010). Changes in sea-level over the last 5000 years, interacting with the low topographic relief and porous bedrock, have played an important role in determining the regional distribution of the diverse ecotones of the Everglades.
South Florida is in a climate transition zone from sub-tropical in the north to tropical in the south (Koppen classification). Average annual rainfall totals about 134.5 cm with about 75% occurring between May and October (Ali and Abtew, 1999). The wet season generally corresponds to the hurricane season, and periodic tropical storms can contribute large volumes of rainfall to the Everglades over a short period of time. Evaporation in south Florida is high in the summer months, but total annual rainfall exceeds annual evaporation. In addition to discrete storm events, multi-decadal oscillations such as El Niño Southern Oscillation (ENSO), Atlantic Multidecadal Oscillation (AMO), and North Atlantic Oscillation (NAO), combined with millennial scale forcing like the mean position of the Intertropical Convergence Zone (ITCZ), have a significant role in shaping the south Florida climate (Bernhardt, 2011; Cronin et al., 2012; Wachnicka et al., 2013b). These climatic oscillations are a factor in periodic flooding and droughts in the Everglades ecosystem, and affect water level, flow and hydroperiod (DeAngelis and White, 1994).
In a region of relatively low-lying topography, subtle differences in the terrain have a significant impact on the hydrology. The primary drainage pathway through the center of the Everglades (SRS; Figure 2) is confined on the east by the Atlantic Coastal Ridge and on the west by the Western Flatwoods (McVoy et al., 2011) and the slightly higher Big Cypress area. In the natural ecosystem, the source of water in the Everglades was primarily direct rainfall (Obeysekera et al., 1999). Other sources of freshwater to the wetlands included intermittent overflows from Lake Okeechobee to SRS and seasonal groundwater seepage from the Atlantic Coastal ridge onto the marl prairie (Parker et al., 1955; McVoy et al., 2011). Freshwater has a relatively long residence time in the shallow-slope landscape and the low north-to-south topographic relief creates slow, mostly laminar (sheet) flow (Kushlan, 1990; Lodge, 2010). The slow-movement of water created lag-times in water delivery to the southern part of the system, which continued past the end of the wet season (Kushlan, 1990). In addition, the presence of peat contributed to the retention of groundwater after the end of the wet season, but, conversely, retarded groundwater rising to the surface from the porous limestone below (Kushlan, 1990; Lodge, 2010; McVoy et al., 2011).
The naturally occurring seasonal wet and dry periods, in conjunction with microtopographic, topographic, and climatic variations, create varying water depths and hydroperiods throughout the freshwater wetlands. East of SRS in the southern part of the marl prairie, water can overflow into Taylor Slough (Figure 2) when water levels are high enough. Prior to the 20th century, Taylor Slough freshwater flows were augmented by rainfall and local groundwater contributions from Long Pine Key (the western extension of the Atlantic Coastal Ridge in ENP). In the natural ecosystem, Taylor Slough flows reduced salinity in Florida Bay by as much as 7–9 ppt compared to the late 20th century, particularly in the near-shore central and eastern Bay waters (Marshall et al., 2014) and likewise, the temporally variable inputs of freshwater from SRS were an important determinant of salinity in the southwest Gulf Coast mangrove zone.
Variations in water depth and hydroperiod allow for diverse and productive wetland plant communities (Lodge, 2010; McVoy et al., 2011; Harvey et al., 2017), which are preserved in the sediments as pollen and macroplant fossils. Recognition of these distinctive water-defined communities in sediment cores provides the means to interpret past hydrologic environments (Bernhardt and Willard, 2009). For example, plants such as water lilies (Nymphaea) prefer deeper water and can be found in SRS and Taylor Slough, whereas plants such as sawgrass (Cladium) are better suited to the adjacent, shallower-water wet prairies and marshes (Long, 1984; Lodge, 2010; McVoy et al., 2011).
Alteration of the natural hydrology began around the start of the 20th century in an attempt to drain the wetlands to create agricultural land. Larger-scale changes followed as canals, levees, pumps and storage structures were built in attempts to control flooding, increase available dry land, and provide water for agriculture and the growing population (Light and Dineen, 1994; McVoy et al., 2011). The largest-scale effort was the Central and Southern Florida Project for Flood Control, authorized by Congress in 1948. By the late 20th century, the reduction of freshwater entering the southern part of the ecosystem from Lake Okeechobee and the diversions through the Atlantic Coastal Ridge into the estuaries, resulted in ENP being almost entirely dependent on precipitation for freshwater inputs. These hydrologic alterations negatively affected the freshwater flora and fauna (Kushlan et al., 1975; Davis et al., 1994, 2005; Ogden et al., 2005; Renken et al., 2005; Lodge, 2010). Harvey et al. (2017) found that alterations in the natural seasonal fluctuations of water depth were a primary cause of degradation in ecosystem function over the last century. In addition, the more frequent intermittent exposures of the freshwater wetland soil surfaces led to oxidation of the peat deposits in many places, thereby altering the microtopography and further affecting hydroperiods (Lodge, 2010; McVoy et al., 2011; Harvey et al., 2017). Downstream, the reduced freshwater contributions altered the natural salinity regimes in the mangrove transition zone and estuaries causing negative impacts to the estuarine biota (Olmsted and Armentano, 1997; Fourqurean and Robblee, 1999; Lorenz, 1999; Sklar et al., 2005; Yarbro and Carlson, 2008; Wachnicka et al., 2013a; Wachnicka and Wingard, 2015; Wingard et al., 2017).
The net result of these hydrologic alterations combined with urban and agricultural development was to reduce the Everglades wetlands ecosystem to approximately 50 percent of its original size. In the late 1980s efforts began to reverse the effects of almost a century of hydrologic alteration with the passage of the Everglades National Park Protection and Expansion Act of 1989 (Public Law 101-229) and the Everglades Forever Act by the Florida Legislature in 1994. The CERP was formulated in the 1990s and authorized by Congress in 2000 (Public Law 106-451; U. S. Army Corps of Engineers and South Florida Water Management District, 1999). Restoration of the remaining Everglades wetlands is envisioned as a 30 to 50-year effort and the National Research Council (2003) has identified the importance of using science to guide restoration.
Study Sites and Observed Conditions
Three sediment cores were selected for paleoecological analysis from cores that had previously been collected by the U.S. Geological Survey (USGS) in the freshwater wetlands of ENP (Figure 2 and Table 1). Cores were selected for this study based on their proximity to long-term ENP water level monitoring stations with each core coming from a different hydrologic and ecologic setting.

Table 1. Locations of the three sediment cores and the adjacent monitoring stations within Everglades National Park.
The P33 core (USGS core 11-1-19-1) is located in SRS about 5.2 km from the P33 water level monitoring station. Water level data have been continuously collected at P33 since October 1952, longer than any other water level monitoring station in ENP (hydrologic station data from South Florida Water Management District; Supplementary Table S1A). For this study, the station data from January 1, 1965 to December 31, 2000 were included to coincide with the period covered by the NSM 4.6.2 water model discussed below. SRS is the primary flow path through the Everglades and the P33 site is the deepest-water environment of the three sites and has the longest observed hydroperiod (Table 2). The SRS flow used in this study is the sum of flows measured at the six monitoring stations located north of the P33 station along the Tamiami Trail (Figure 2) and span the main flow path of the slough.
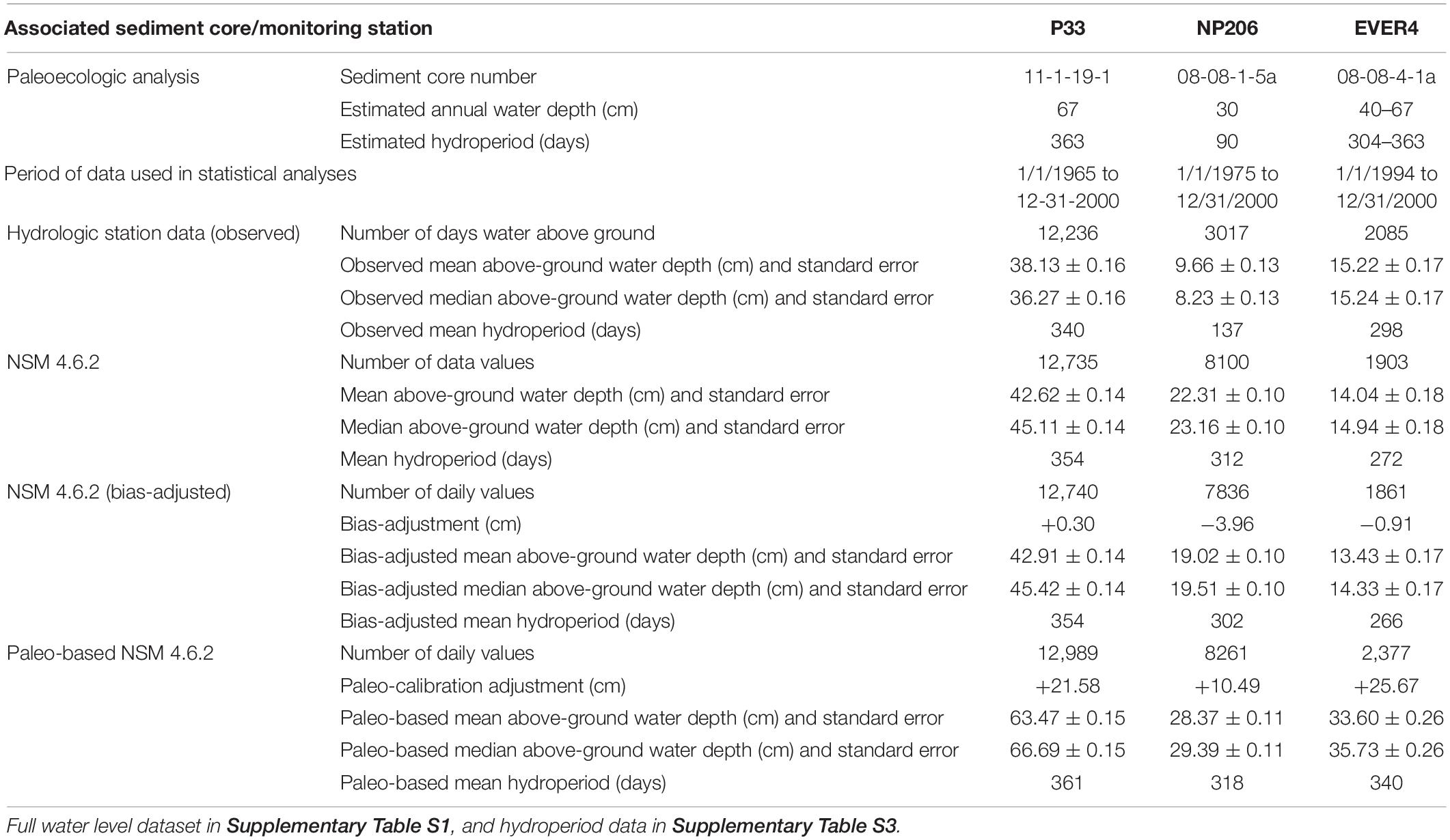
Table 2. Water level and hydroperiod data from paleoecologic analyses, observed, NSM 4.6.2, NSM 4.6.2 bias-adjusted, and NSM 4.6.2 paleo-adjusted.
The NP206 sediment core (USGS core 08-08-1-5a) is located east of the main flow path of SRS approximately 0.7 km from the associated ENP NP206 water level monitoring station in an area that has been characterized as both Rockland marl marsh and as wet prairie/sawgrass marsh for the pre-20th century time period (Lodge, 2010; McVoy et al., 2011). The collection of water level data started at NP206 in October 1974; for this study the data start on January 1, 1975 and run through December 31, 2000 (Supplementary Table S1B), the date the NSM 4.6.2 water model ends. The average observed water levels at NP206 monitoring station over the period of data analyzed are the shallowest of the three locations and the average observed hydroperiod is the shortest (Table 2). In addition to rainfall and groundwater contributions to the water levels in the vicinity of NP206, when water levels are high enough in SRS the slightly higher wet prairie around NP206 also can be inundated by the overflow. When this area is inundated, water may flow south into Taylor Slough through an existing low area in the westward extension of the Atlantic Coastal Ridge. The Taylor Slough Bridge (TSB) flow monitoring station is located near this low area (Figure 2).
The EVER4 sediment core (USGS core 08-08-4-1a) is in the southeastern region of ENP and may be influenced by tides and salt water intrusion into the ground water. This area has been characterized as a Perrine marl marsh (McVoy et al., 2011), a coastal wet prairie (Lodge, 2010), and a marl prairie. The EVER4 core is about 2.5 km from the associated EVER4 water level monitoring station. Collection of water level data began at the site in November 1993 and the data used for this study start January 1, 1994 and run through December 31, 2000 (Supplementary Table S1C), the date the NSM 4.6.2 water model ends. Observed water levels and hydroperiods are intermediate between the other two sites (Table 2). Water levels at the EVER 4 station are relatively well correlated to Taylor Slough flows even though EVER4 is approximately 12 km east of the slough and water levels in the surrounding coastal wet prairie are supplemented by rainfall and groundwater contributions.
Paleoecologic Analysis
In order to reconstruct past vegetation assemblages to use as a proxy for hydrologic conditions, pollen was isolated from sediment using standard palynological methods as described in Bernhardt and Willard (2015; Figure 3, step 1). Cores were subsampled in 1-cm increments and approximately 0.5 g of dry sediment was used for palynological analysis. Samples were first acetolyzed (9 parts acetic anhydride: 1 part sulfuric acid) in a hot-water bath (100°C) for 10 min, then neutralized and treated with 10% KOH in a hot-water bath for 15 min. Neutralized samples were sieved with 5 and 200 μm sieves, and the 5–200 μm fraction was stained with Bismarck Brown, mixed with warm glycerin jelly, and mounted on microscope slides. Palynomorph identification was conducted using a light microscope at 400x magnification. Taxonomic identifications were based on reference collections of the United States Geological Survey (Reston, VA, United States) and Willard et al. (2004).
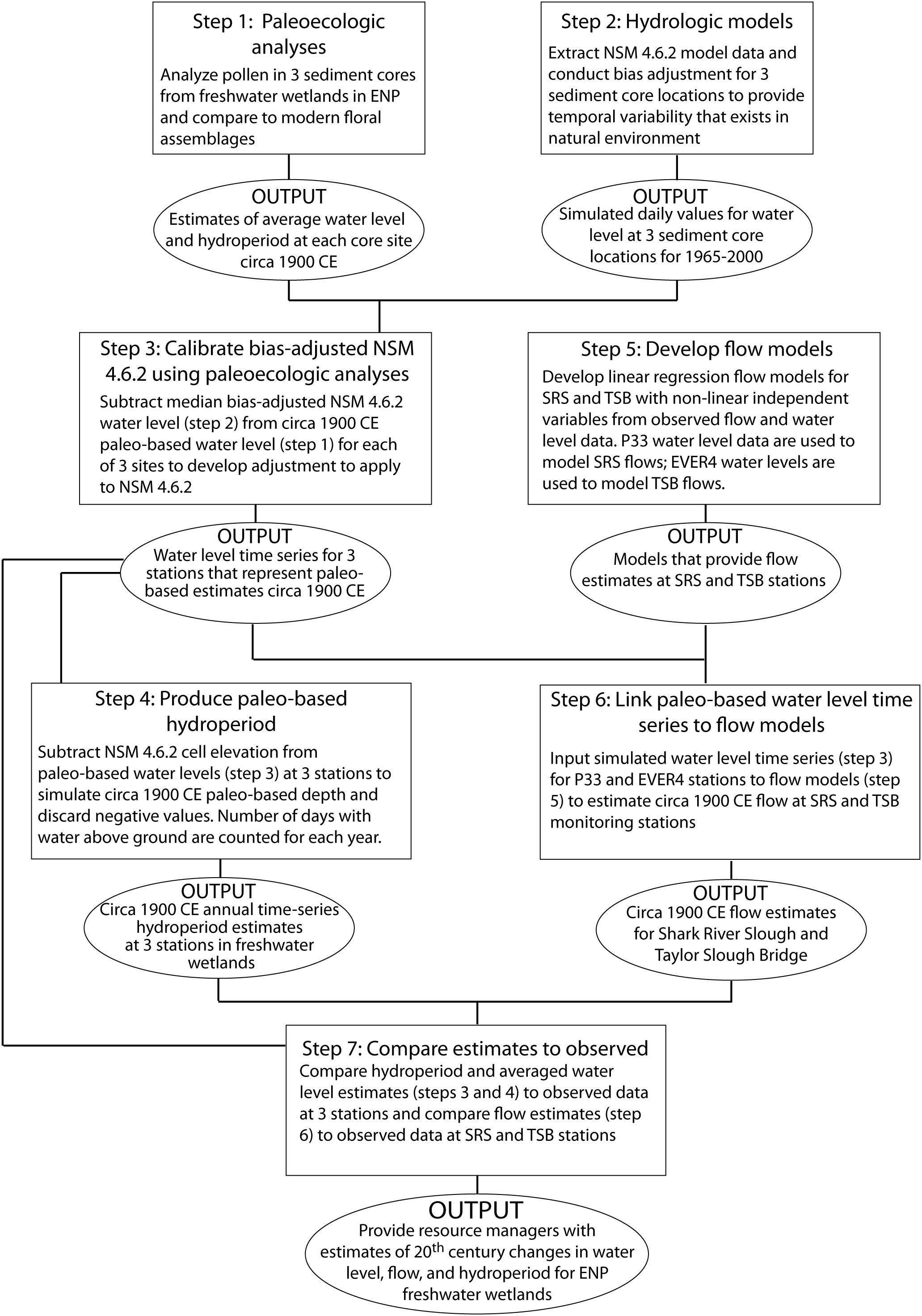
Figure 3. Flow chart illustrating sequence of steps to produce estimates of circa 1900 CE water levels, flow, and hydroperiods using paleoecologic data, and comparing these estimates to modern observed records. The three stations referred to in the diagram are the Everglades National Park (ENP) water level monitoring stations P33, NP206, and EVER4 that are in proximity to the three sediment cores selected for this study. SRS is Shark River Slough; flow for SRS is a composite of data from 6 stations spread horizontally across US Rt. 41/Tamiami Trail. TSB is a flow monitoring station at Taylor Slough Bridge. See Figure 2 for location of cores and monitoring stations.
Percent abundance of major wetland taxa can be used to accurately discern plant communities only meters apart in the Everglades (Willard et al., 2001; Bernhardt and Willard, 2009). Because hydrologic conditions are one of the primary controls on the distribution of plant communities in the Everglades, we can infer water depth and hydroperiod of past intervals based on documented hydrologic controls observed and measured for the modern plant communities. This method of using present knowledge of plant hydrologic requirements to determine past hydrologic conditions has been used successfully to relate changing plant communities to changes in climate and land use (Willard et al., 2001, 2007; Bernhardt and Willard, 2009; Bernhardt, 2011).
In order to determine past plant communities (and therefore hydrologic conditions) based on pollen assemblages, we applied the Modern Analog Technique (Overpeck et al., 1985) to downcore pollen assemblages at the P33, NP206 and EVER4 core sites (Figure 3, step 1). We used square-chord distance between pollen assemblages from the cores and pollen assemblages from a surface sample data set (where hydrologic conditions are documented for each sample/vegetation type). Square-chord distance was used because it is not influenced by rare taxa. The hydrologic environments (average seasonal water levels and hydroperiod) for the identified plant communities in each segment of each core were characterized using field measurements and the published research on freshwater wetland plants in the Everglades and similar environments (for example see Willard et al., 2001; Givnish et al., 2008; Lodge, 2010).
Downcore pollen assemblages were divided into plant community zones by using stratigraphically constrained cluster analysis (CONISS) using the Tilia software package (Grimm, 1992)1. In this method of zonation, clusters are constrained by incremental sum of squares method. The data are not transformed, and we use the percent abundance pollen data including all taxa in the pollen sum for each sample. This method visually groups segments in the core with similar pollen assemblage types and therefore similar plant communities (i.e., prairie, marsh, slough, or ridge).
For the P33 and EVER4 sediment cores, the first increase (greater than one pollen grain) of Casuarina equisetifolia (Australian pine) was used to estimate the depth in the core that corresponded to the circa 1900 CE period. Casuarina equisetifolia was introduced to south Florida from Australia around the beginning of the 20th century, and thus serves as an important biostratigraphic marker (Duever et al., 1986; Langeland, 1990; Wingard et al., 2007). For the NP206 core, the circa 1900 CE horizon was identified by the abrupt increase of Ambrosia (ragweed) and by comparison to the regional pollen stratigraphy. Abrupt increases in percent abundance of Ambrosia can be indicative of intensification of regional land-clearance in south Florida, which occurred around the beginning of the 20th century.
For this study, the plant communities just before and directly following the circa 1900 CE period were the primary focus. The products of this paleoecological analysis are average water level and hydroperiod values for the segments of the cores before-and-after circa 1900 CE, for each sediment core (Figures 4–6; pollen data in Supplementary Table S2). These sediment core-based data at the P33, NP206, and EVER4 sediment core locations are considered the best available information on the historic condition and are the primary data used in this paper to estimate average hydrologic conditions for the pre- and post-circa 1900 CE time frame.
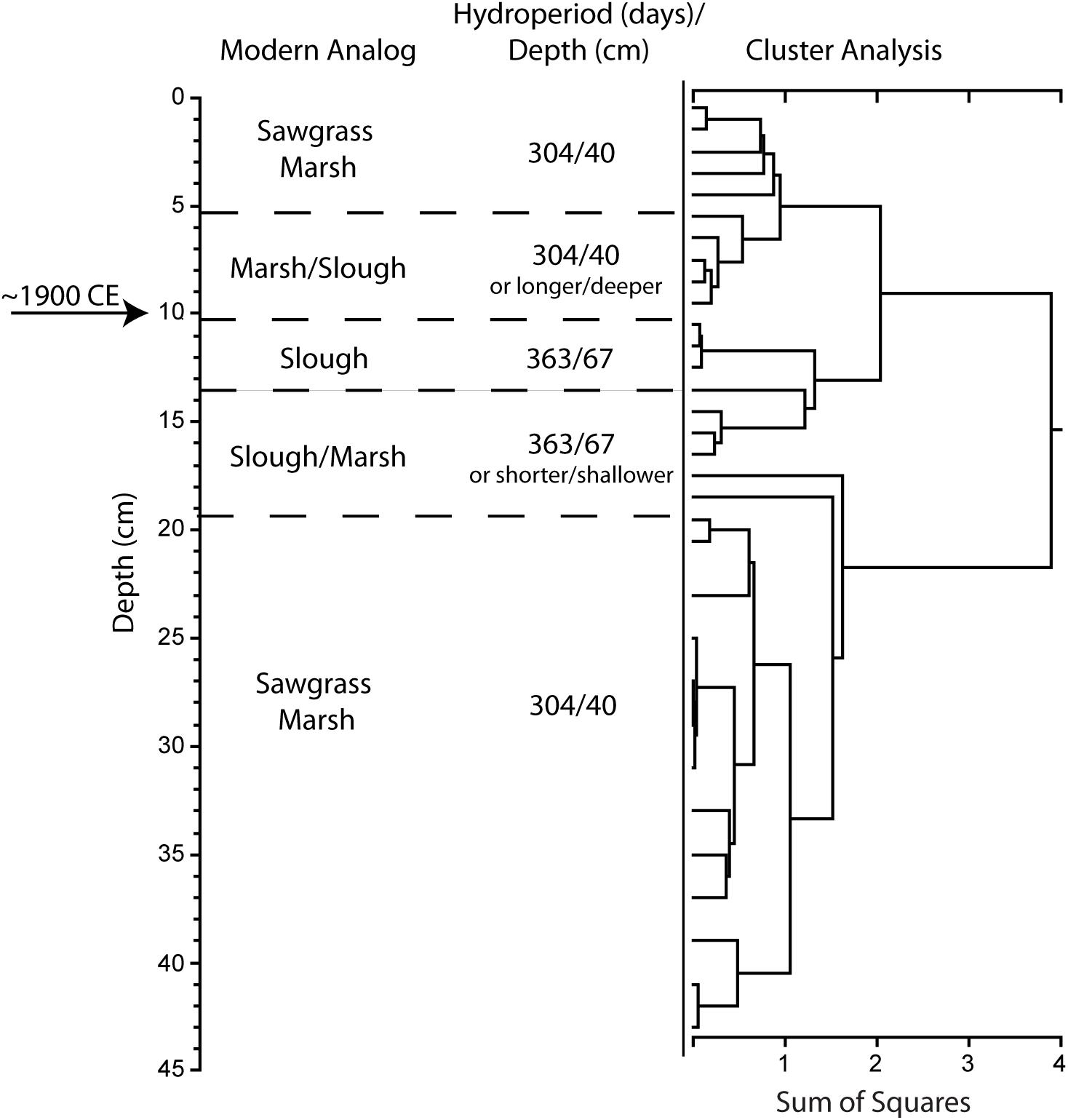
Figure 4. Results of stratigraphically constrained, correlation coefficient (Pearson product moment) cluster analysis of pollen assemblage from P33 sediment core (USGS core 11-1-19-1). Pollen count data are presented in Supplementary Table S2. Circa 1900 CE horizon is based on the first occurrence of Casuarina equisetifolia (Australian pine).
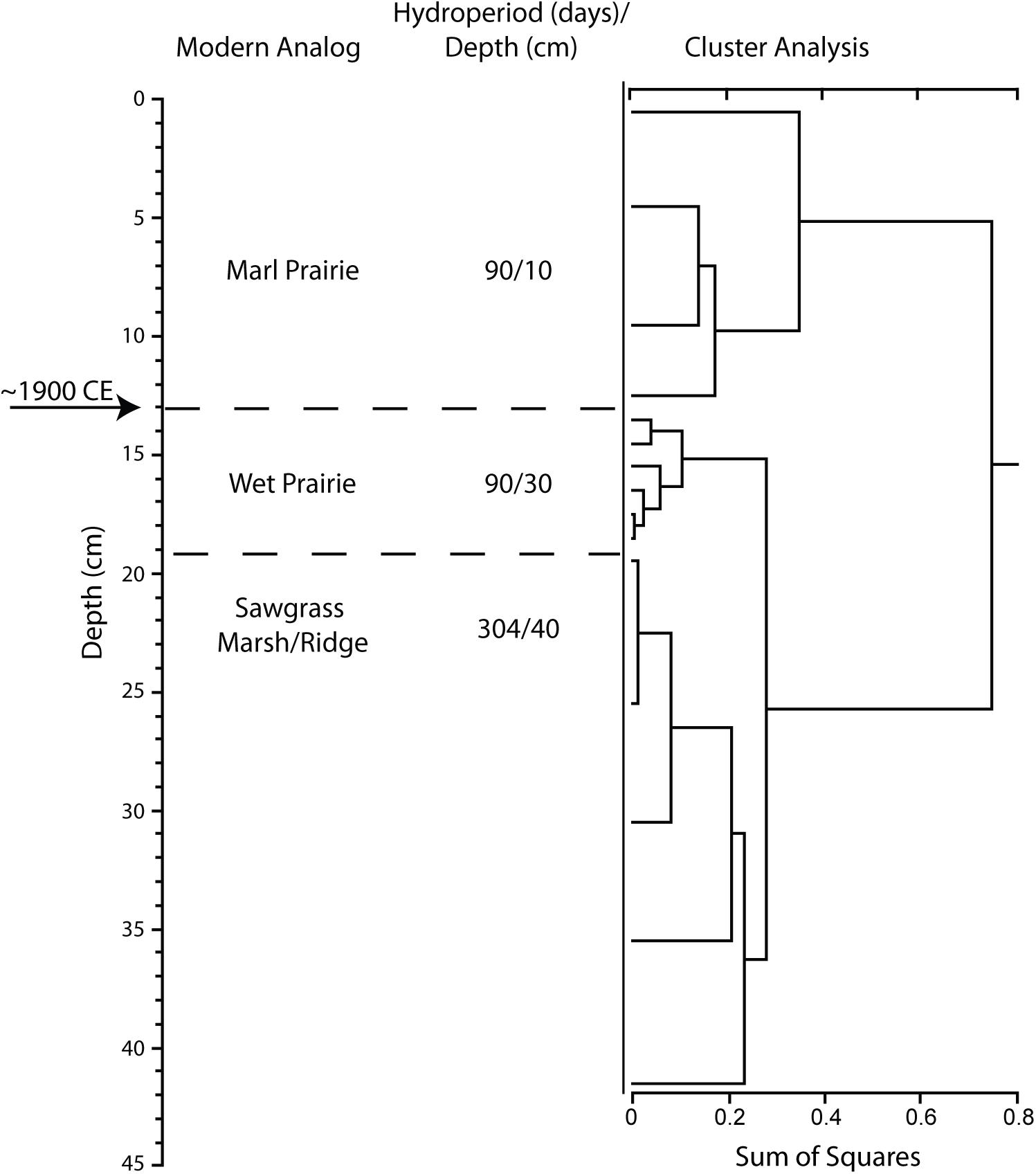
Figure 5. Results of stratigraphically constrained, correlation coefficient (Pearson product moment) cluster analysis of pollen assemblage from NP206 sediment core (USGS core 08-08-1-5a). Pollen count data are presented in Supplementary Table S2. Circa 1900 CE horizon was identified by the presence of Ambrosia (ragweed) and by comparison to the regional pollen stratigraphy.
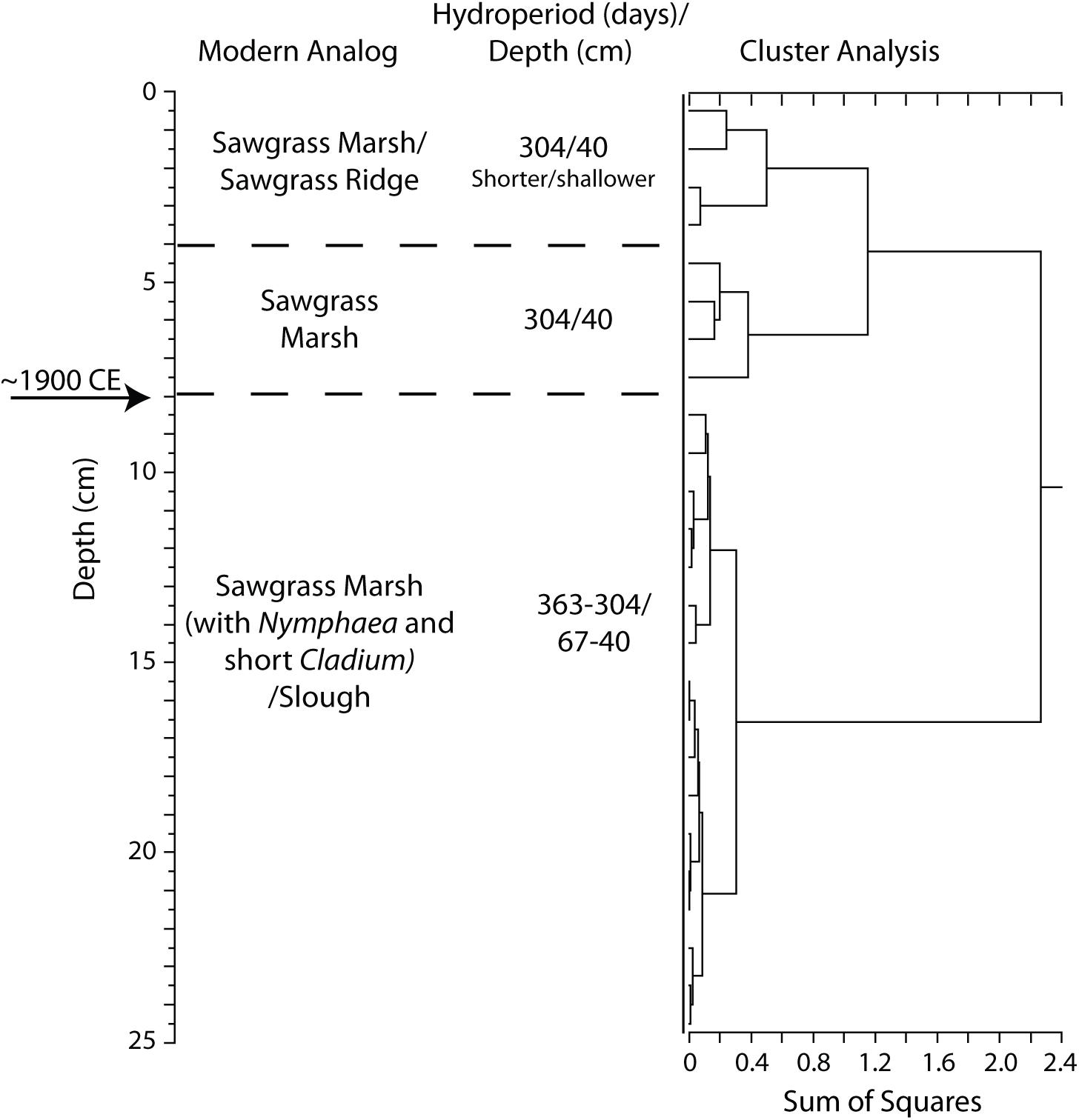
Figure 6. Results of stratigraphically constrained, correlation coefficient (Pearson product moment) cluster analysis of pollen assemblage from EVER4 sediment core (USGS core 08-08-4-1a). Pollen count data are presented in Supplementary Table S2. Circa 1900 CE horizon is based on the first occurrence of Casuarina equisetifolia (Australian pine).
NSM 4.6.2 Hydrologic Model
The basis for the simulated temporal variability for the pre-circa 1900 CE average water levels and hydroperiods at the P33, NP206, and EVER4 sediment core locations was provided by the outputs from an existing model of the historic Everglades developed by the South Florida Water Management District – the Natural System Model2 (NSM version 4.6.2; Figure 3, step 2; Table 2; full dataset in Supplementary Table S1). This temporal hydrologic variability provided by the NSM 4.6.2 is necessary to reconstruct water levels, flows and hydroperiods prior to the beginning of water monitoring; this seasonal and annual variability cannot be derived from the paleo-record. The NSM 4.6.2 is a large-scale numeric model (grid cell size is 3.2 km by 3.2 km) that simulates the pre-circa 1900 water level conditions of south Florida and produces timeseries outputs for specific locations. The model utilizes 1965-2000 climate records because no continuous observed data exist within the Everglades prior to construction of the drainage features. The NSM 4.6.2 also uses available information on elevation of the Everglades landscape from maps, surveys, and anecdotal records from the early 1900s.
The NSM 4.6.2, and our use of the model in this study, assumes that seasonal patterns of rainfall for the beginning of the 20th century were similar to the 1965–2000 period. As explained in Marshall et al. (2009), this is a reasonable assumption because patterns of rainfall for the region are similar for 1895–1950 time period and the 1960–2000 time period (Enfield et al., 2001; Basso and Schultz, 2003). Large scale climate drivers such as the AMO also were similar for the beginning of the 20th century and the 1965–2000 time period (Enfield et al., 2001). For these reasons, the output from NSM 4.6.2 is considered to be a ‘best professional judgment’ estimate of the variability of the pre-1900 CE hydrology at the sediment core locations.
To use the NSM 4.6.2 for our paleo-analyses, several adjustments to the raw model output were made (Supplementary Table S1 and Supplementary Documentation). NSM model output for each model cell is water level elevation in feet above the 1929 National Geodetic Vertical Datum (NGVD29). We subtracted the elevation data at each water monitoring station from the NSM output for the associated model cell which converted the resulting water levels (elevation) to water depth (cm). The NSM 4.6.2 is a derivative of the South Florida Water Management Model (SFWMM), a regional hydrologic model for south Florida on a 2 by 2-mile grid; however, the SFWMM output contains a bias (MacVicar et al., 1984; South Florida Water Management District, 2005; also described in unpublished reports, F. E. Marshall, 2014, 2015, 2016). Model bias is the difference between the model output and the measured value of the parameter being estimated. Therefore, we removed the bias by making a temporally constant adjustment to the NSM data (Table 2; complete dataset in Supplementary Table S1) for each station (P33, NP206, and EVER4). Only the above ground values were used to determine mean and median water levels, and hydroperiods.
Calibration of NSM 4.6.2 Using Paleoecologic Analyses
The results of the paleoecologic analyses were used to calibrate the bias-adjusted NSM 4.6.2 output to reflect late 19th century conditions (Figure 3, step 3) using the following method. First, the median bias-adjusted NSM 4.6.2 above-ground water depth at each of the three primary monitoring stations (P33, NP206, and EVER4) was subtracted from the paleo-estimated water level for the circa 1900 time-frame at the corresponding sediment core sites. For EVER4, the paleo-estimated water level produced a range of 40–67 cm. Based on our knowledge of the EVER4 core site and the EVER4 monitoring station site, we used the low end of the paleo-based range (40 cm) as the starting point for subtracting the bias-adjusted NSM median. The differences calculated are the paleo-based calibration adjustments for each station (Table 2). Next, these paleo-based calibration adjustments were added to each value in the P33, NP206, and EVER4 bias-adjusted NSM 4.6.2 time series to create the paleo-based NSM 4.6.2 water levels (Supplementary Table S1 and Supplementary Documentation). Paleo-based NSM 4.6.2 hydroperiods for each station were calculated by (1) subtracting the ground elevation (NGVD 29) from the paleo-based NSM 4.6.2 water levels for each day of each year in the model; (2) removing any water level values less than or equal to zero; and (3) counting the number of days water level was above-ground each year (Supplementary Table S3). All years in the model were averaged to produce a paleo-based mean hydroperiod for each station (step 4).
The goal in calibrating the NSM 4.6.2 to the paleoecological analyses was to produce average water levels and hydroperiods from the bias-adjusted NSM 4.6.2 time series that simulate the longer-term average values derived from the sediment cores for comparison to the observed data. The period included in the analyses for the water level monitoring data at each of the three monitoring stations have different starting dates, but the same ending date (December 31, 2000). Even though the periods of analysis are different, these data were considered to be the best available information and the number of values is sufficient for producing statistically significant differences when comparing the observed data to the model output. The adjusted NSM 4.6.2 water levels and hydroperiods for each of the three sediment core sites are herein referred to as paleo-based/paleo-adjusted. The outputs from steps 3 and 4 are paleo-based NSM 4.6.2 water level time series and hydroperiods for the P33, NP206, and EVER4 sites that, for this study, are assumed to estimate the variability of the late 19th century hydrologic conditions near the three sediment core locations.
Development of Flow Models
In order to simulate the paleo-based flow at SRS and TSB, linear flow models with non-linear independent variables were developed from daily average observed flow data3 (Table 3) and daily average observed water level data (Supplementary Table S1) using the SAS© PROC REG stepwise regression routine with a 99% level of confidence as the criterion for including any independent variable in a linear regression flow model (Figure 3, step 5 and Supplementary Documentation).
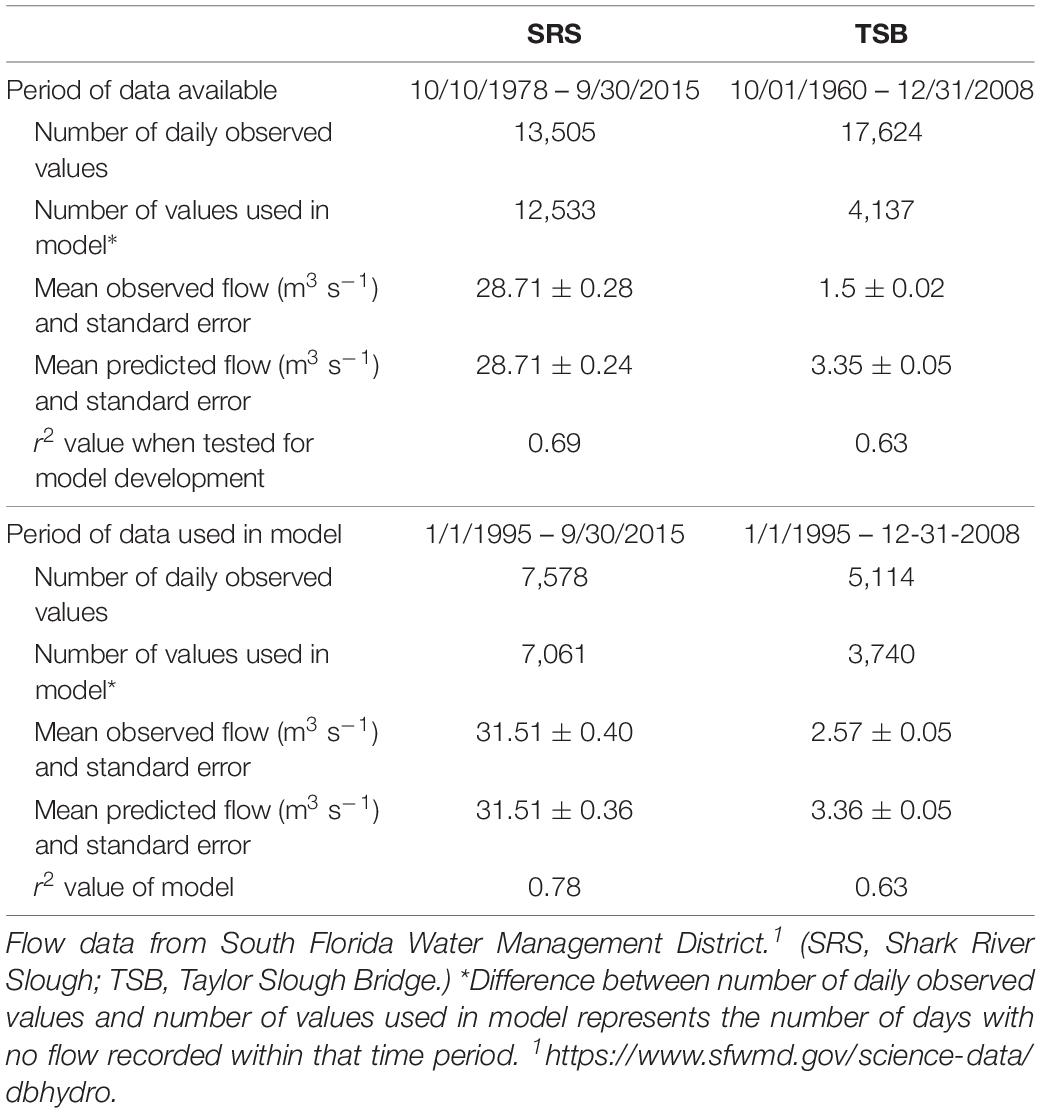
Table 3. Summary of observed data from flow monitoring station(s); comparison of model development results using full period of available data, and period beginning in 1995.
To develop the models, we experimented with the following: (1) which periods of observed data to include in model development; (2) whether to include below ground water levels and zero values; (3) whether to use squared or cubed water level as flow model inputs to account for peak flow periods; and (4) which variable (flow or water level) should be the dependent and which the independent. With all other components being equal, when the models using flow as dependent and water level as independent were compared to the inverse, the two versions of the models produced similar results. However, water level was the logical choice for the independent variable because stage (converted to water level in the models) is the output variable provided by the NSM (and other hydrologic models), and it is the variable estimated by the paleoecologic analyses. In addition to experimenting with model configurations, we also tested which water level monitoring stations best explained the variability seen in flow for SRS and TSB. The models selected for use in these analyses, and described in the next paragraphs, are the iterations that had the highest r2 values and that came closest to replicating the observed flow (mean and pattern of highs and lows).
The observed daily SRS flow4 used in this study is a water balance based on the measured flows into SRS through six water control structures along Tamiami Trail: SRS = S12A + S12B + S12C + S12D + S333 - S334 (note, S334 is a subtraction, because its location on the eastern side of the park diverts water away from SRS). The P33 monitoring station is located within the main channel of SRS and a model with the P33 water level data as the independent variable explains more of the variability in SRS flow compared to the water depth data from NP206 and EVER4 (r2 = 0.78 vs. r2 = 0.29 and r2 = 0.31, respectively).
The selected SRS flow model used daily average water depth at P33 as the independent variable and the observed daily flow across the Tamiami Trail as the dependent variable. For the model, below ground water levels were set to zero, the observed data from January 1, 1995 to September 30, 2015 were included in model development, and water level was cubed. Although a much longer period of record was available to use for the model (Table 3), the data from 1978 through the end of the 1980s represented a period when the effects of water management created a highly altered hydrologic system (mean observed for 1978–2015 = 28.7 m3s–1, compared to 31.5 m3s–1 for 1995–2015). In the early 1990s concern emerged that water management was negatively affecting the water-based ecosystem (Light and Dineen, 1994), and increased flows began to be provided to ENP resulting in flow regimes that were considerably closer to natural conditions. Therefore, the data from January 1, 1995 to September 30, 2015 were used for SRS flow model development.
The selected Taylor Slough flow model used daily average water depth at EVER4 as the independent variable and the observed daily flow at TSB5 as the dependent variable. For the model, below ground water levels were set to missing and the observed data from January 1, 1995 to December 31, 2008 were included in model development. As described above for the SRS model, a longer record was available for use in model development (Table 3), but the period from 1995 forward provided a better model of the natural system. Although the r2 values are the same for the two periods for TSB, the observed and predicted mean flows are much closer for the 1995–2008 period and using the shorter time period makes comparison to the SRS model more viable. The EVER4 water depth data were used because flow for TSB is more highly correlated with water depths at EVER4 than at NP206 (r2 = 0.63 vs. r2 = 0.48, respectively). When a stepwise-selection process was used to select parameters for a multivariate linear regression (MLR) model, the addition of NP206 as an independent variable to a model that had already been fitted with EVER4 independent variables provided very little improvement to goodness-of-fit statistics as measured by partial r2 values.
Paleo-Based Estimates and Comparison to Observed Data
To produce the paleo-based flow estimates for circa 1900 CE, the paleo-based NSM 4.6.2 water level time series data for P33 and EVER4 (Figure 3, step 3) were input to the SRS and TSB flow models (step 5) to simulate paleo-based flows at SRS and TSB, respectively (step 6; Supplementary Documentation). As the final step, the paleo-based water levels and hydroperiods at P33, NP206, and EVER4 and the paleo-based flows at SRS and TSB were compared to the observed data for the period of the data (Tables 4, 5) for each monitoring station (step 7). The results from these comparisons provide an approximation of the differences between the late 19th century water levels, hydroperiods, and flows and the late 20th century conditions. The paleo-based water level and flow estimates from this study also were compared to the estimates of wetland hydrologic conditions inferred from Florida Bay paleoecology/modeling studies (Marshall et al., 2014).
Results
Observed Hydrologic Conditions
Observed hydrologic data (water depth and hydroperiod) from the monitoring stations associated with each sediment core serve as a reference point for the current status and conditions in the three Everglades wetland areas (Table 2 and Supplementary Table S1). Although longer records exist at the three monitoring stations, the observed data summarized here coincide with the data used for the NSM 4.6.2 model. The P33 water level monitoring station in the main flow path of SRS, has the highest average water depth over the period of analysis (38.1 cm, averaged over 12,236 days) and the longest hydroperiod (340 days, averaged over 36 years) of the three study sites (Table 2). At NP206, in the marsh east of the SRS, the average water depth (9.7 cm, averaged over 3017 days) and hydroperiod (137 days, averaged over 26 years) are the lowest of the three sites. The EVER4 water monitoring station, located in the southeastern coastal wet prairie, is intermediate to the other two sites with an average water depth of 15.2 cm (averaged over 2085 days) and a hydroperiod of 298 days (averaged over 7 years). Observed flow across the Tamiami Trail in SRS over a 22-year period is 31.3 m3 s–1 and observed flow at TSB over a 36-year period is 1.3 m3 s–1; for the decade of the 1990s flow was slightly higher – 40.6 m3 s–1 for SRS and 2.3 m3 s–1 for TSB (Table 5).
Paleoecologic Analyses
Paleoecological analysis of the P33 sediment core from SRS (Figure 3, step 1) identified the circa 1900 CE horizon at a depth of 10 cm below the top of the core based on the first occurrence of Casuarina (Figure 4). The P33 sediment core pollen assemblage for the segments directly below the identified circa 1900 CE horizon (i.e., prior to the first major water management projects) is analogous to freshwater slough assemblages and indicates that the average seasonal water depth at that time was about 67 cm and the average hydroperiod was about 363 days (Table 2). The immediate post-circa 1900 CE period shows the beginning of a transition at the core site to a marsh environment, with relatively shorter hydroperiods and shallower water levels than the pre-1900 segment as indicated by the increased abundance of Morella and Amaranthaceae pollen.
The NP206 sediment core was collected from the freshwater wetland area east of SRS. The abrupt increase in the abundance of Ambrosia pollen in the NP206 sediment core was found at 13 cm below the present ground surface and provided an estimate of the circa 1900 CE horizon (Figure 5). The pollen assemblages for the segments prior to circa 1900 CE describe an environment that was characteristic of a wet prairie with a hydroperiod of about 90 days and an average seasonal water depth of about 30 cm (Table 2). Similar to the P33 sediment core analysis, the core segment just prior to the circa 1900 CE horizon was wetter than the observed data at the NP206 sediment core location. These wetter conditions are indicated by a reduced abundance of Amaranthaceae and Asteraceae pollen. The early post-circa 1900 CE pollen assemblage indicates a transition at the NP206 core location to a marl prairie with an average hydroperiod of about 90 days, similar to pre-circa 1900 CE, but with a reduced average seasonal water depth of approximately 10 cm, similar to the observed data.
The pollen analysis for the EVER4 core (Figure 6) identified the circa 1900 CE horizon at a depth of 8 cm below the ground surface based on the first occurrence of Casuarina. Modern analog analysis of the pollen assemblage in the core segment just below the circa 1900 CE horizon provides evidence of a late 19th century plant community surrounding the EVER4 site that was characteristic of a sawgrass marsh with a 304 to 363-day hydroperiod and annual average water depths of about 40 to 67 cm (Table 2). The pollen analysis for the EVER4 sediment core segments just above the circa 1900 CE horizon indicates a transition in the early 20th century to a generally drier site, indicated by the increase in Poaceae and Asteraceae pollen. Throughout the core there is a relatively consistent amount of Cladium pollen. In addition, there is evidence of two distinct plant communities post-circa 1900 CE at this sediment core site (Figure 6). The pollen analyses indicate that, for the earlier 20th century conditions, the average hydroperiod was about 304 days and the average seasonal water depth was about 40 cm. The pollen assemblages from the uppermost portion of the core (i.e., later in the 20th century) provide evidence of a further-reduced hydroperiod of less than 304 days with an average seasonal water depth of approximately 40 cm, more characteristic of a sawgrass marsh or sawgrass ridge environment as interpreted by an abrupt increase in Cladium pollen.
Collectively, the sediment cores examined provide evidence for a wetter pre-drainage environment at the three locations within the Everglades. The shifts in pollen assemblages occurred at approximately the same time as the first large-scale water management projects were completed in south Florida, circa 1900 CE.
Calibration of the NSM 4.6.2 Hydrologic Model
A comparison of the NSM 4.6.2 data for each of the three primary monitoring stations (P33, NP206, and EVER4) to the paleo-estimated circa 1900 CE average water levels (Figure 3, step 3) indicates that the unadjusted NSM 4.6.2 water levels (step 2) were less than the pollen-based paleo-estimates (step 1). When the paleo-based water depth adjustments were applied to the bias-adjusted NSM 4.6.2 water level data for the P33, NP206, and EVER4 stations, the resulting time series outputs portray water levels that are generally similar to the hydrologic environment indicated by the circa 1900 CE paleoecologic analyses (Table 2 and Figure 7). The paleo-based NSM 4.6.2 water levels for P33 and EVER4 provide the input for the flow models.
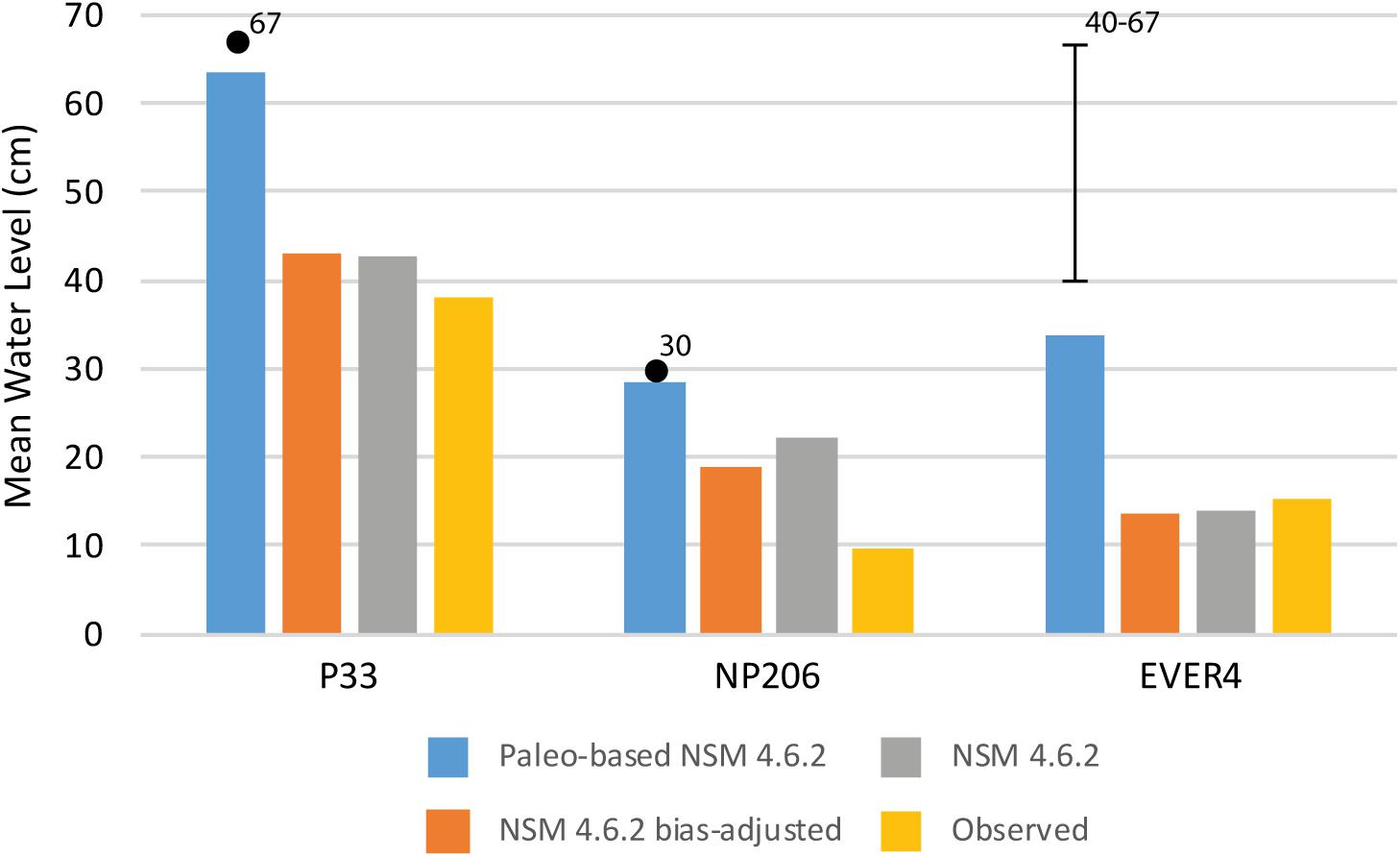
Figure 7. Histogram comparing paleo-adjusted, NSM 4.6.2 bias-adjusted, NSM 4.6.2, and observed mean water levels (in cm) over the period of data used in the analyses for each of the three locations. Numbered dots and range are estimates of water level from paleoecologic analysis for reference. Data are in Table 2.
Flow Models
To estimate paleo-based flows at SRS, the following linear regression flow model (based on observed data from 1995 to 2015, Table 3) was developed using SAS© Proc Reg procedures:
SRS flow is the dependent variable and water level at P33 is the independent variable. The r2 value for this SRS flow model is 0.78 and the standard error of prediction is 1.4 m3 s–1 (N = 7,061).
To estimate paleo-based flows at TSB, the following linear regression flow model (based on observed data from 1995 to 2008, Table 3) was developed using SAS© Proc Reg procedures:
TSB flow is the dependent variable and water level at EVER4 is the independent variable. The r2 value for this TSB flow model is 0.63 and the standard error of prediction is 0.5 m3 s–1 (N = 3740).
Comparison of Paleoecologic, Observed, and Model Results
The observed mean water depth and hydroperiod and the model-produced mean water depth and hydroperiod for each of the model runs (NSM 4.6.2, bias-adjusted NSM 4.6.2, and paleo-based NSM 4.6.2) are presented in Table 2 for each of the three stations associated with a sediment core analysis (full dataset in Supplementary Table S1). Even though the full period for the model-produced NSM 4.6.2 data ranges from January 1, 1965 to December 31, 2000, the period of the data presented in Table 2 represents the period of overlap between the observed data at each station (truncated as described in Methods) and the NSM 4.6.2 data. Consequently, results from the P33 analyses are based on 36 years of observed data and model input, NP206 on 26 years, and EVER4 on only 7 years.
Figure 7 and Table 2 show that the paleo-based NSM 4.6.2 water depths, which represent circa-1900 values, are deeper than the observed or than the other simulated values for the period of analysis at all three stations. At P33 and NP206, the observed mean and median water levels represent the lowest values for the period of analysis compared to the output from the model runs. At EVER4, the NSM 4.6.2 and bias-adjusted 4.6.2 represent the lowest mean and median water level values. Comparing the observed and paleo-based NSM 4.6.2 results (Table 4), the paleo-based mean water depth exceeds the observed by 25.3 cm for P33, 18.7 cm for NP206, and 18.4 cm for EVER4.
A comparison of the paleo-based NSM 4.6.2 mean hydroperiod to the observed, the NSM 4.6.2, and the bias-adjusted NSM 4.6.2 hydroperiods for each of the three stations indicates that the paleo-based NSM 4.6.2 hydroperiods are the longest for the period of analysis (Table 2 and Figure 8). For P33 and EVER4, the paleo-adjusted NSM comes the closest to producing the paleoecologic estimates of circa 1900 CE hydroperiods. In contrast, for NP206, the NSM 4.6.2 model outputs (unadjusted, bias-adjusted, and paleo-adjusted) produce a significantly longer hydroperiod than that indicated by the paleoecologic analysis, and even the observed hydroperiod of 137 days exceeds the paleoecologic estimate of 90 days. Comparing the observed and paleo-based NSM 4.6.2 results (Table 4), the paleo-based mean hydroperiod exceeds the observed by 21 days for P33, 181 days for NP206, and 42 days for EVER4.
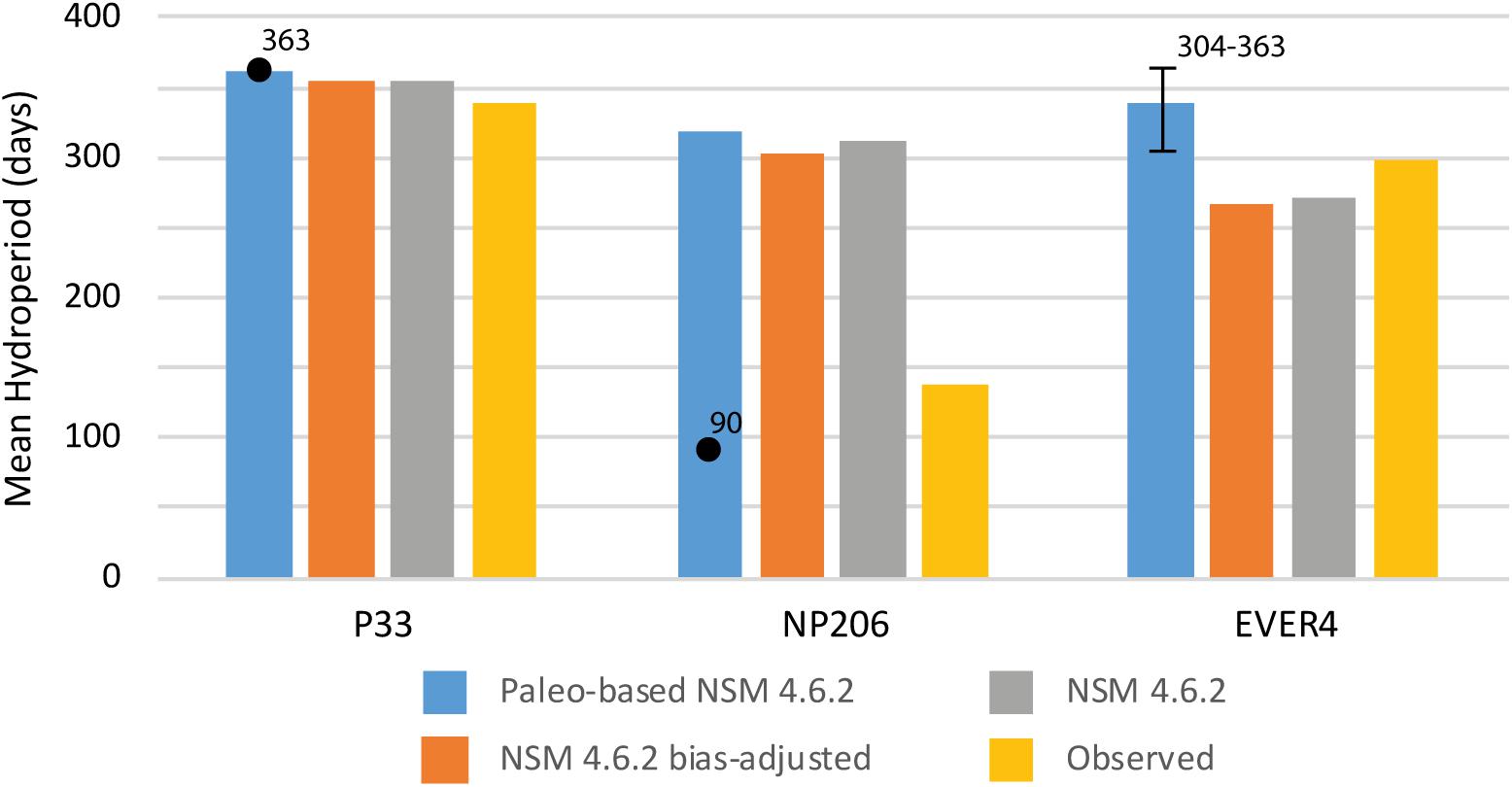
Figure 8. Histogram comparing paleo-adjusted, NSM 4.6.2 bias-adjusted, NSM 4.6.2, and observed mean hydroperiods (in days) over the period of data used in the analyses for each of the three locations. Numbered dots and range are estimates of water level from paleoecologic analysis for reference. Data are in Table 2.
Pre-circa 1900 CE paleo-based flows into SRS were simulated using the P33 paleo-based water levels as inputs to the SRS flow model for two time periods: (1) October 12, 1978 to December 31, 2000, the full period of overlap between the observed data and the NSM 4.6.2 model data, and (2) January 1, 1990 through December 31, 2000. The 1990s time period incorporates the documented increase in flows due to water management (Van Lent et al., 1993; Kotun and Renshaw, 2013) and also is the time period used in Marshall et al. (2014). The model outputs for the full period of overlap (Table 5 and Figure 9) estimate that the average paleo-based SRS flow was 71.5 m3 s–1. The observed SRS flow for the same time period was 31.3 m3 s–1, a difference between observed flows and paleo-based flows of 40.2 m3 s–1 and a ratio of paleo-based SRS flows to observed flows of 2.3. For the truncated time period (1990–2000), the model outputs estimate that the average paleo-based SRS flow was about 82.9 m3 s–1 and the average observed SRS flow for the same time period was 40.6 m3 s–1. The difference between the observed flows and paleo-based flows for the 1990s is 42.3 m3 s–1 and a ratio of paleo-based SRS flows to observed flows of 2.0.
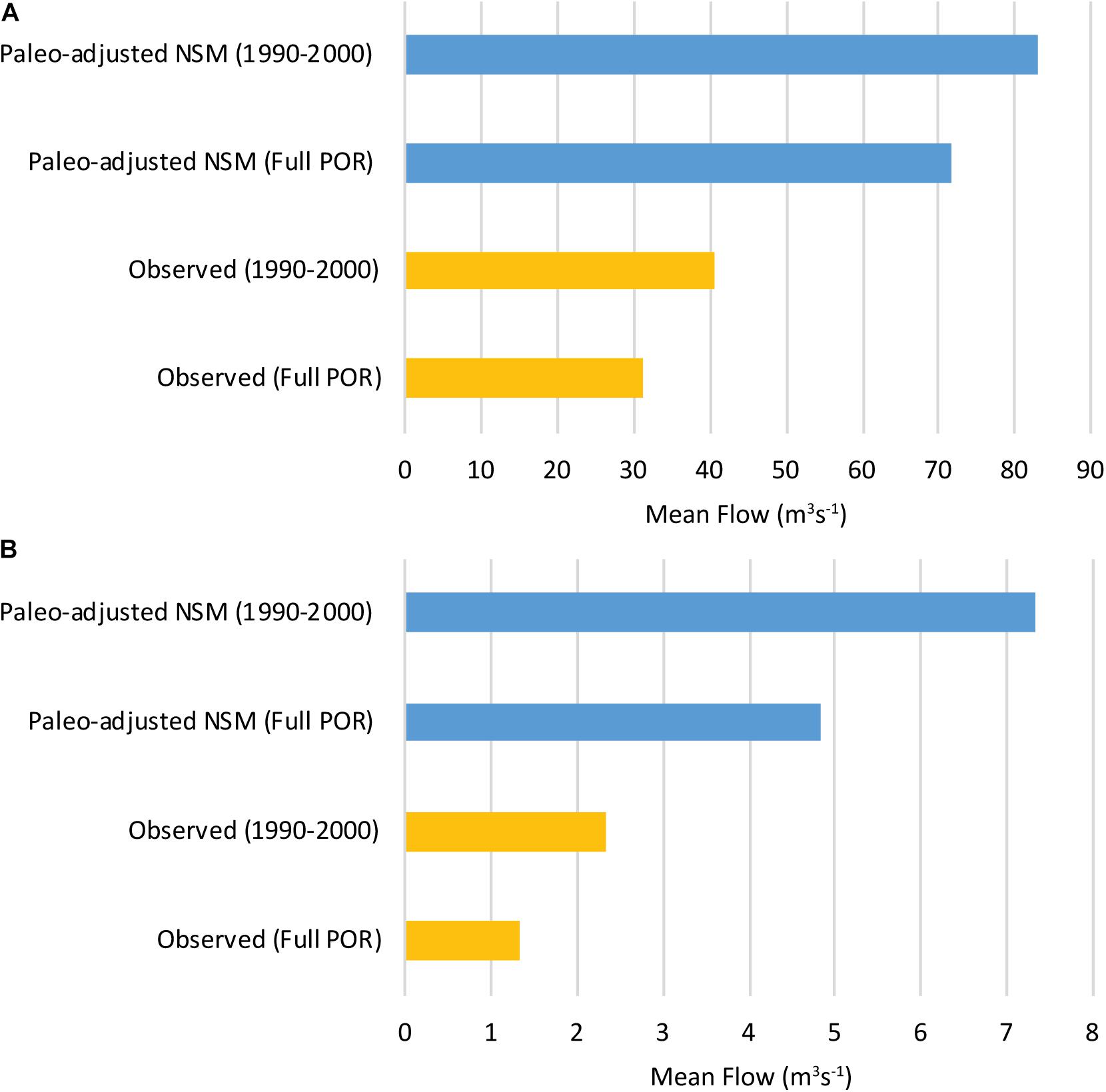
Figure 9. Bar chart comparing mean observed flow to the mean paleo-adjusted NSM 4.6.2 flow for the full period of record (POR) and the 1990–2000 period, in cubic meters per second (m3s–1). (A) Shark River Slough (SRS) and (B) Taylor Slough Bridge (TSB) (data in Table 5; note different x-axis scales).
Pre-circa 1900 CE paleo-based flows into Taylor Slough were simulated using the EVER4 paleo-based water levels as inputs to the TSB flow model for two time periods: (1) January 1, 1965 to December 31, 2000, the full period of overlap between the observed data and the NSM 4.6.2 model data, and (2) January 1, 1990 through December 31, 2000, for comparison to SRS and Marshall et al. (2014). The model outputs for the full period of overlap (Table 5) estimate that the average paleo-based TSB flow was about 4.8 m3 s–1. The observed TSB flow for the same time period was 1.3 m3 s–1, a difference between observed flows and paleo-based flows of 3.5 m3 s–1 and a ratio of paleo-based TSB flows to observed flows of 3.6. For the truncated time period (1990–2000), the model outputs estimate that the average paleo-based TSB flow was about 7.3 m3 s–1. The average observed TSB flow for the same time period was 2.3 m3 s–1, which is a difference of 5.0 m3 s–1 and the ratio of paleo-based flows to observed flows is 3.2.
Discussion
A primary goal of Everglades restoration is “to get the water right” (Sklar et al., 2005) – returning the quantity, quality, and timing of freshwater flow into and through ENP and into its downstream estuaries to an approximation of natural, pre-drainage hydrology. The National Research Council Committee on Independent Scientific Review of Everglades Restoration Progress stated in 2010 that it was “essential” for restoration planning to understand freshwater flow in the pre-drainage system (National Research Council, 2010, p. 118). To assist restoration managers in identifying the quantity of pre-drainage flow, a project was implemented in 2006 to link paleoecologic data on salinity in Florida Bay with linear regression model outputs based on observed water levels in the freshwater wetlands and flows to the estuaries. The goal was to estimate the freshwater input to the downstream estuarine ecosystem that was necessary to produce the circa 1900 CE salinities in Florida Bay (Marshall et al., 2009). The initial effort, which used paleosalinity estimates from one core in the central portion of Florida Bay, was followed with additional work incorporating data from five Florida Bay sediment cores into the linear regression models and synthesizing the model outputs (Marshall and Wingard, 2012; Marshall et al., 2014). The paleosalinity results indicated that flow through SRS was 2.1 times the observed, and flow through Taylor Slough at the TSB was 3.7 times the observed, over the period of analysis (1990–2000; Marshall et al., 2014). In addition, the water level at P33 was estimated to be 23 cm higher circa 1900 CE than the observed (period of analysis 1990–2000; Marshall et al., 2014).
The study described here was designed to better understand the late 19th century freshwater hydrology of the Everglades and to determine if paleoecologic data from the freshwater wetlands, and the models developed using these data, would support the earlier (Marshall et al., 2014) estimates of flow based on paleosalinity in Florida Bay. Few anecdotal records of the pre-drainage Everglades ecosystem exist, so it is essential to corroborate any estimates of the historic conditions prior to implementation of targets and performance measures. To date, paleoecologic analyses have provided needed insights into past hydrologic conditions and habitat characterizations in the Everglades freshwater wetlands (for example, Saunders et al., 2006; Willard et al., 2006; Bernhardt and Willard, 2009; Willard and Bernhardt, 2011; Bernhardt et al., 2013), but these assessments are generally site specific and have highlighted the spatial heterogeneity of the system (Bernhardt et al., 2013). Willard and Bernhardt (2011) proposed using paleoecologic records from the freshwater wetlands to validate model estimates of pre-drainage hydrology. This is the approach we have used for this study – paleoecologic evaluations at three sites to assess and adjust as necessary the NSM 4.6.2 hydrologic model. The NSM 4.6.2 is considered a “best professional judgment” for simulating the pre-drainage hydrology and is used for restoration goal setting for the CERP (U.S. Army Corps of Engineers and U.S. Department of the Interior, 2015). However, as demonstrated here, when the outputs from the NSM 4.6.2 model are compared to paleoecologic studies and to outputs from other models of pre-drainage Everglades hydrology (such as NSRSM; F.E. Marshall, unpublished report, 2016), the NSM 4.6.2 model portrays a generally drier natural system for the circa 1900 time period than the results of other analyses (National Research Council, 2010).
The pollen records from the three core sites indicate a wetter pre-drainage environment and responses to changing conditions around the beginning of the 20th century. Such rapid responses of vegetation to hydrologic changes were noted by Harvey et al. (2017) and they believe reductions in average water depth caused the rapid initial responses in the plant communities. When comparing our results to historical reconstructions, the paleo-based NSM 4.6.2 outputs indicate that the pre-1900 CE seasonal average water depths may have been slightly higher for the P33 and NP206 sediment core locations than what is described in McVoy et al. (2011). At the location of the EVER4 sediment core, the results of this study and the water level estimate of McVoy et al. (2011) are similar.
Hydroperiod results are not as consistent as water level. For P33 and EVER4, the paleo-based NSM 4.6.2 hydroperiods (Table 2 and Figure 8) are close to approximating the hydroperiod derived from the paleoecologic analyses and exceed the model-based (unadjusted and bias-adjusted NSM 4.6.2) hydroperiod estimates. However, for NP206 the paleoecological data indicate a hydroperiod significantly less than any of the model estimates (unadjusted, bias-adjusted, and paleo-adjusted NSM 4.6.2), and less than the observed, despite the close approximation of the paleo-adjusted NSM to the paleo-estimated water level (28.4 cm and 30 cm, respectively). This inconsistency is due in part to the NSM 4.6.2 overestimating hydroperiod for this site (312 days compared to 137 observed; Table 2). In the observed data, the water level at NP206 has the shallowest water depth and shortest hydroperiods. The station is above ground only 3017 days in 26 years. During this period, four years have zero flow, 10 years out of 26 have values >0 but <100 days, only 7 years have values >200 days, and only 1 year (1995) exceeds 300 days. The ratios comparing NSM 4.6.2 to observed hydroperiods are 1.04 for P33, 2.78 for NP206, and 0.91 for EVER4, so despite changes in water level over time, the NSM 4.6.2 comes close to approximating the number of days above ground water levels are observed at P33 and EVER4 over the periods analyzed. However, the number of days NSM 4.6.2 estimates above ground water levels at NP206 is almost three times greater than observed. When the paleo-adjustments in water level are added to the NSM 4.6.2, it converts more below ground levels to above ground, increasing the discrepancy even further.
This discrepancy between the hydroperiod estimated from the paleoecologic analysis at NP206 and the modeled NP206 hydroperiod illustrates the difficulty of modeling a spatially heterogeneous system. The pollen records contained in the sediment cores are the best available information on the pre-1900 CE hydrology at each core site. In contrast, the NSM 4.6.2 is a landscape-scale model and each cell in the model covers over 10 km2 and assumes that there is a constant elevation within each cell. Lodge (2010) describes the marl prairie where the NP206 monitoring station and the NP206 sediment core are located as containing ‘pinnacle rock’ – an irregular limestone surface with projections that may rise 0.3 ± meters above the surrounding terrain and solution holes forming in low areas. Assuming constant elevation in an area with this type of microtopography could explain the discrepancy between the NSM 4.6.2 estimates of hydroperiod and therefore the paleo-adjusted model output. Peat fires might be another explanation for the discrepancy at NP206 because they can cause subsidence through oxidation (Lodge, 2010; McVoy et al., 2011); however, no charcoal analyses were done on the core so this idea cannot be tested. In contrast, the P33 monitoring station and its associated core are located in the main flow channel of SRS, a relatively large area that is typically underwater, so the effects of micro-topography and/or model-cell size do not cause significant variations in the hydroperiod results. P33 also is farther from any drainage canals than the other two sites and has the longest period of analysis. The results at P33 portray a general lowering of the average water level over the last century and a relatively small change in the hydroperiod with an observed hydroperiod at P33 of 340 days per year and model-based hydroperiods ranging from 354 to 360. These minor shifts in the P33 hydroperiod are significant, however, because with P33’s location in the main flow channel it would be expected to be underwater most of the time. In addition, the P33 paleo-adjustment used in this study (+21.58 cm) is very close to the +23 cm above the P33 paleo-estimated water level for the circa 1900 system in Marshall et al. (2014). At EVER4, which is closest to drainage canals, the paleo-adjusted NSM 4.6.2 average water level does not quite reach the low-end of the paleoecologically estimated range for water level but is still significantly higher than the observed and the unadjusted and bias-adjusted NSM 4.6.2 output. The observed hydroperiod at EVER4 is higher than the unadjusted and bias-adjusted NSM 4.6.2, again highlighting the problems with using NSM 4.6.2 model cells that do not take into account the local variations in topography.
The goal of this and previous paleoecologic and modeling exercises has been to estimate the hydrologic conditions that existed in the Greater Everglades Ecosystem prior to construction of drainage features. The output from the SRS flow model presented here indicates that restoration of the pre-water management levels in the SRS freshwater wetlands within ENP will require an increase in average SRS flow of 2.0 times the 1990s observed average flow or 2.3 times the average flow from 1978 to 2000 (Table 5 and Figure 9). These findings are consistent with the previous paleosalinity-based flow estimates for SRS (Marshall et al., 2014) of 2.1 times the 1990s observed SRS flow. The long period of water level data at the P33 station, the position of the core and the station in the main flow channel of SRS, the high r2 value for the model (r2 = 0.78), and the close correspondence of the estimates of paleo-adjusted flow in this study to our previous results using cores from Florida Bay, give us confidence in our estimate that late 19th century flow in SRS was 2.0 to 2.3 times the late 20th century flows.
The outputs of the TSB flow model presented here indicate that restoration of pre-drainage water levels would require an increase in TSB flows of 3.2 times the 1990s observed average flow or 3.7 times the averaged flow from 1965 to 2000. Like SRS, these findings are consistent with the Marshall et al. (2014) estimate that TSB flow for the late 19th and early 20th century was 3.7 times TSB flow in the 1990s. Despite these consistent results, we are not as confident in the TSB flow estimates compared to the SRS estimates for several reasons. First, the TSB flow model is based on EVER4 water depth data and the EVER4 station has only been in place since 1994, therefore it documents hydrologic conditions during a period when water management had already begun to increase flow into Taylor Slough (Van Lent et al., 1993; Kotun and Renshaw, 2013) and the r2 value (0.63) is lower than for SRS (0.78). Second, the EVER4 station is located outside the main flow path for Taylor Slough and therefore EVER4 may not be as reliable a predictor of flow at TSB as P33 is for SRS. Third, EVER4 is located close to the ENP boundary and is more impacted by water management in the adjacent non-regulated park lands; this is also a region where ground water and surface water interactions affect water levels and flow (Harvey et al., 2000). Finally, the area outside of the main sloughs can have variable microtopography. We selected a paleo-adjustment for EVER4 of +25.7 cm based on the paleo-based water level data at the core site, but a different adjustment may have been appropriate for the EVER4 monitoring station, given the existing variations in topography.
These results support previous observations and model results that indicate Taylor Slough and SRS carried significantly more water prior to the implementation of water control. The paleo-based flows also estimate the quantity of water needed for restoration. The close agreement of the estimates of flow for SRS and TSB from this study and the previous estimates based on paleosalinity in Florida Bay is a significant finding, because two completely different proxies from two different regions of the Everglades (pollen from Everglades freshwater wetlands and mollusks from Florida Bay) have provided very similar results. The results also are consistent with Smith et al. (1989) estimates of a 59% decrease in flow from SRS in the 20th century based on analyses of fluorescent banding in a coral from Florida Bay.
Estimates of pre-drainage hydrology are intended as a guide for restoration managers in determining project feasibility and in setting performance measures and targets for restoration. In practice, it will be difficult to increase water levels in the ENP beyond a certain point due to the extensive urban and agricultural development that has occurred in south Florida over the last century and because the CERP mandate that restoration cannot negatively impact flood control or water supply. Additionally, restoration of more natural hydrology alone will not be equally effective in all regions of the Everglades; success will depend on a number of factors including retention of the pre-drainage microtopography (Harvey et al., 2017). The existing SRS flow data indicate that water levels at P33 have a relatively strong correlation to flows, and water levels at the different monitoring stations within the freshwater wetlands of ENP are relatively highly correlated to one another (Probst and Rosendahl, 1981; Marshall et al., 2011). These correlations suggest that the most direct and effective way of increasing water levels in SRS is by increasing flows across Tamiami Trail into ENP. Attempts to do so thus far have been hampered by a number of factors, including seepage into the existing network of canals6. In addition, any water used to increase SRS water levels must be of suitable quality such that the benefits of increased flows outweigh any potential harm; thus, treatment may be required before delivery to SRS. Because of the direct hydrologic connection between SRS and the ENP estuaries along the Gulf of Mexico, increases in water levels and flows in SRS should result in reduced salinities downstream. Lower salinities would benefit the ecologically important salinity transition zone (“inshore marine habitat,” Livingston, 1990) and is a desired CERP objective. Engineering options for restoration of pre-water management flows to Taylor Slough are somewhat limited, although some projects have already been implemented (for example, widening of the TSB; South Florida Water Management District, 2008). The lack of options for Taylor Slough increases the importance of restoring freshwater levels and flows in SRS and the eastern wet prairie, where NP206 is located, in order to maximize the overflow from SRS into Taylor Slough. In addition, improving the hydrologic conditions of Taylor Slough has the benefit of improving the dependent freshwater wetland biota in Taylor Slough as well as the salinity conditions in the downstream nearshore estuarine embayments of Florida Bay within ENP.
It has been demonstrated through long-term paleoecologic studies (Saunders et al., 2006; Willard et al., 2006; Bernhardt and Willard, 2009; Willard and Bernhardt, 2011; Wingard and Hudley, 2012; Bernhardt et al., 2013; Wachnicka et al., 2013b; Wachnicka and Wingard, 2015; Wingard et al., 2017) that over the last 5000 years changes in climate patterns have altered the hydrology and the habitats of the Greater Everglades Ecosystem in different ways, depending on location within the ecosystem. Virtually all environmental proxies that have been measured in sediment cores indicate nearly synchronous changes in the early to mid-20th century, most of which are believed to be associated with the construction of water management structures and the subsequent land-use changes that have altered the natural landscape. The most important driving factor in the Greater Everglades Ecosystem is the availability of freshwater. Everglades restoration is driven by the primary goal to “get the water right,” therefore, efforts have been focused on restoring more natural hydrologic conditions. Responses of the Everglades biota to past natural perturbations seen in paleoecologic analyses indicate that the ecosystem will respond positively to an increase in flow, and the logical target is restoration to the circa 1900 CE levels indicated in this and previous studies. The question is whether such an increase is defensible and feasible. A mass balance check on estimated SRS flow (Marshall et al., 2014) found that the volumes of freshwater needed to restore the SRS flows were roughly comparable to the estimates of the average total volumes of freshwater that are presently being discharged to the east and west coasts through water management activities. This implies there is enough water being diverted from flowing south into the Everglades to achieve historic flows if it could be redirected. Restoration of more natural flow would benefit not only the habitats and biota of the freshwater wetlands of ENP, but also the downstream estuaries and in addition, it would reduce or eliminate the ecologically harmful discharges to the Atlantic and Gulf coasts from the canals draining Lake Okeechobee.
Summary
The results of this study agree with previous paleoecologic and modeling efforts. The results support the concept that the pre-drainage Everglades ecosystem of the late 19th century was significantly wetter than the post-drainage conditions of the wetlands within ENP. We have quantified the pre- and post-drainage differences in water level and hydroperiod for three sites and flow estimates at two stations within the freshwater wetlands. In addition, we have demonstrated a method for adjusting individual cells within the NSM 4.6.2 hydrologic model to circa 1900 CE conditions that can then be used to estimate historic flow. The differences noted between the three core locations highlight the heterogeneity of the Everglades wetlands and the need to further calibrate the NSM 4.6.2 and other models of the pre-circa 1900 CE conditions (i.e., the Natural System Regional Simulation Model, or NSRSM) to better reflect the pre-drainage conditions. Paleoecologic characterizations in previous studies also have shown this spatial heterogeneity of the freshwater wetlands in ENP and the long-term responses to both natural and anthropogenic disturbances; however, past studies have not been directly incorporated into models that can quantify the needed restoration flows into ENP. Paleoecologic data allow us to hindcast conditions prior to anthropogenic disturbance. By incorporating these data into existing models, the models are calibrated to the past conditions instead of relying solely on hydrologic modeling to recreate those conditions. We propose that paleoecologic studies be used similarly at additional locations in the freshwater wetlands and the estuaries to ground-truth the models to the greatest extent possible using the available data.
The results from this study and a previous study (Marshall et al., 2014) indicate that flow through the SRS would need to increase approximately two times current levels to simulate late 19th century conditions and flow through Taylor Slough would need to increase approximately three times. The existing hydrologic conditions indicate that an increase in flow would achieve the higher water levels and typically longer hydroperiods that have been estimated by the paleoecologic analyses. We recognize that restoring flows to these levels may be difficult given the land-use changes that have occurred over the last century and potential loss of microtopography. However, it is essential for restoration planners and resource managers to understand the magnitude of the flows needed and the relationships between flow, water level and downstream salinity in the estuaries needed to simulate the natural hydrology. By basing performance measures and targets on models that have been calibrated to reflect pre-drainage conditions, CERP managers will have defensible targets and a better chance of achieving the restoration goal to “get the water right” for the benefit of the ecology of the freshwater wetlands of the Everglades and the downstream receiving estuaries.
Data Availability Statement
All datasets generated for this study are included in the article/Supplementary Material.
Author Contributions
FM and GW collaborated on the original concept of using paleoecologic data to adjust hydrologic models. CB determined how to utilize pollen data in the current models and conducted all palynological analyses. FM, GW, and CB developed the paleo-adjustment to the freshwater models. FM ran the models. GW cross-checked input and output, and documented methodology. FM wrote the original drafts. GW revised text for the final submission and designed all tables and most figures.
Funding
This research was entirely funded by the U.S. Geological Survey, Greater Everglades Priority Ecosystems Science (GEPES) Program. Cetacean Logic Foundation received grants from the USGS to conduct the modeling work through the Cooperative Ecosystem Studies Units (CESU) Network (cooperative agreement numbers G13AC00248 and G18AC00347).
Conflict of Interest
The authors declare that the research was conducted in the absence of any commercial or financial relationships that could be construed as a potential conflict of interest.
Acknowledgments
The research described in this article was entirely funded by the USGS Greater Everglades Priority Ecosystems Science (GEPES) program. Any use of trade, firm, or product names is for descriptive purposes only and does not imply endorsement by the U.S. Government. We would like to thank Patrick Pitts, U.S. Fish and Wildlife Service (retired) for reviewing several early versions of this manuscript; his comments and questions significantly improved the final product. Lucy Edwards, U.S. Geological Survey (emeritus) also provided helpful comments at an early stage. We would like to thank our two reviewers for their thorough reading of the manuscript and their helpful suggestions. Bethany Stackhouse and Sarah Bergstressor, USGS, prepared Figures 1, 2, and B. Stackhouse helped with preparation of the reference list. Bryan Landacre, USGS, assisted with the pollen analyses. We would also like to thank Everglades National Park for providing access to the core sites; the work described here is covered in part by NPS Study Number EVER-00141.
Supplementary Material
The Supplementary Material for this article can be found online at: https://www.frontiersin.org/articles/10.3389/fenvs.2020.00003/full#supplementary-material
Abbreviations
CERP, Comprehensive Everglades Restoration Plan; ENP, Everglades National Park; NSM, Natural Systems Model; SRS, Shark River Slough; TSB, Taylor Slough Bridge; USGS, U.S. Geological Survey.
Footnotes
- ^ https://www.tiliait.com/
- ^ South Florida Water Management District, https://www.sfwmd.gov/science-data/nsm-model
- ^ Flow data from South Florida Water Management District; https://www.sfwmd.gov/science-data/dbhydro
- ^ Flow data from South Florida Water Management District; https://www.sfwmd.gov/science-data/dbhydro
- ^ Flow data from South Florida Water Management District; https://www.sfwmd.gov/science-data/dbhydro
- ^ http://www.l31nseepage.org/index2.html
References
Ali, A., and Abtew, W. (1999). Regional Rainfall Frequency Analysis for Central and South Florida. Technical Report, Report No. WRE # 380. West Palm Beach, FL: South Florida Water Management District. XSXS
Basso, R., and Schultz, R. (2003). Long-Term Variation in Rainfall and Its Effect on Peace River Flow in West-Central Florida. Hydrologic Evaluation Section. Brooksville, FL: Southwest Florida Water Management District.
Bernhardt, C. E. (2011). Native Americans, regional drought, and tree island evolution in the Florida Everglades. Holocene 21, 967–978. doi: 10.1177/0959683611400204
Bernhardt, C. E., Brandt, L. A., Landacre, B., Marot, M. E., and Willard, D. A. (2013). Reconstructing vegetation response to altered hydrology and its use for restoration, Arthur R. Marshall Loxahatchee national wildlife refuge, Florida. Wetlands 33, 1139–1149. doi: 10.1007/s13157-013-0469-y
Bernhardt, C. E., and Willard, D. A. (2009). Response of the Everglades sawgrass ridge and slough landscape to late Holocene climate variability and 20th century water-management practices. Ecol. Appl. 19, 1723–1738. doi: 10.1890/08-0779.1
Bernhardt, C. E., and Willard, D. A. (2015). “Pollen and spores of terrestrial plants,” in Handbook of Sea Level Research, eds I. Shennan, A. J. Long, and B. P. Horton (West Sussex: John Wiley & Sons), 218–232. doi: 10.1002/9781118452547
Cronin, T. M., Wingard, G. L., Dwyer, G. S., Swart, P. K., Willard, D. A., and Albietz, J. (2012). “Climate variability during the medieval climate anomaly and little ice age based on ostracod faunas and shell geochemistry from Biscayne Bay, Florida,” in Developments in Quaternary Science, Ostracoda as Proxies for Quaternary Climate Change, Vol. 17, eds D. J. Horne, J. A. Holmes, J. Rodriguez-Lazaro, and F. Viehberg (Amsterdam: Elsevier), 241–262. doi: 10.1016/B978-0-444-53636-5.00014-7
Davis, S. M., Gaiser, E. E., Loftus, W. F., and Huffman, A. E. (2005). Southern Marl Prairies conceptual ecological model. Wetlands 254, 821–831.
Davis, S. M., Gunderson, L. H., Park, W. A., Richardson, J. R., and Mattson, J. E. (1994). “Water control in the Everglades: a historical perspective,” in Everglades: The Ecosystem and its Restoration, eds S. M. Davis and J. C. Ogden (Delray Beach, FL: St. Lucie Press), 419–444.
DeAngelis, D. L., and White, P. S. (1994). “Ecosystems as products of spatially and temporally varying driving forces, ecological processes, and landscapes: a theoretical perspective,” in Everglades: The Ecosystem and Its Restoration, eds S. M. Davis and J. C. Ogden (Delray Beach, FL: St. Lucie Press), 9–27.
Duever, M. J., Carlson, J. E., Meeder, J. F., Duever, L. C., Gunderson, L. H., Riopelle, L. A., et al. (1986). The Big Cypress National Preserve. Research Report No. 8. Manhattan, NY: National Audubon Society.
Enfield, D. B., Nunez, A. B., and Trimble, P. J. (2001). The Atlantic multidecadal oscillation and its relation to rainfall and river flows in the continental U.S. Geophys. Res. Lett. 28, 2077–2088.
Fourqurean, J. W., and Robblee, M. B. (1999). Florida Bay: a history of recent ecological changes. Estuaries 22, 345–357.
Givnish, T. J., Volin, J. C., Owen, V. D., Volin, V. C., Muss, J. D., and Glaser, P. H. (2008). Vegetation differentiation in the patterned landscape of the central Everglades: importance of local and landscape drivers. Glob. Ecol. Biogeogr. 17, 384–402. doi: 10.1111/j.1466-8238.2007.00371.x
Grimm, E. C. (1992). CONISS: a Fortran 77 program for stratigraphically constrained cluster analysis by the method of incremental sum of squares. Comput. Geosci. 13, 13–35. doi: 10.1016/0098-3004(87)90022-7
Harvey, J. W., Jackson, J. M., Mooney, R. H., and Choi, J. (2000). Interaction Between Ground Water and Surface Water in Taylor Slough and Vicinity, Everglades National Park, South Florida: Study Methods and Appendixes. Open-File Report No. 00-483. Reston, VA: U.S. Geological Survey. doi: 10.3133/ofr00483
Harvey, J. W., Wetzel, P. R., Lodge, T. E., Engel, V. C., and Ross, M. S. (2017). Role of a naturally varying flow regime in Everglades restoration. Restor. Ecol. 25, S27–S38. doi: 10.1111/rec.12558
Johns, G., Lee, D. J., Leeworthy, V., Boyer, J., and Nuttle, W. (2014). Developing economic indices to assess the human dimensions of the South Florida coastal marine ecosystem services. Ecol. Indic. 44, 69–80. doi: 10.1016/j.ecolind.2014.04.014
Kotun, K., and Renshaw, A. (2013). Taylor Slough Hydrology, fifty years of water management 1961-2010. Wetlands 34, S9–S22. doi: 10.1007/s13157-013-0441-x
Kushlan, J. A. (1990). “Freshwater marshes,” in Ecosystems of Florida, eds R. L. Meyers and J. J. Ewel (Orlando, FL: University of Central Florida Press), 324–363.
Kushlan, J. A., Ogden, J. C., and Higer, A. L. (1975). Relation of Water Level and Fish Availability to Wood Stork Reproduction in the Southern Everglades, Florida. Open File Report No. 75-434. Reston, VA: U.S. Geological Survey, 56. doi: 10.3133/ofr75434
Langeland, K. (1990). Exotic Woody Plant Control Florida. Circular No. 868. Tavares, FL: Florida Cooperative Extension Service.
Light, S. S., and Dineen, J. W. (1994). “Water control in the Everglades: a historical perspective,” in Everglades: The Ecosystem and its Restoration, eds S. M. Davis and J. C. Ogden (Delray Beach, FL: St. Lucie Press), 47–84.
Livingston, R. J. (1990). “Inshore marine habitats,” in Ecosystems of Florida, eds R. L. Meyers and J. J. Ewel (Orlando, FL: University of Central Florida Press), 549–573.
Long, R. W. (1984). “Origin of the vascular flora of Florida,” in Environments of South Florida: Present and Past II, ed. P. J. Gleason (Miami, FL: Geological Society), 118–126.
Lorenz, J. J. (1999). The response of fishes to physiochemical changes in the mangroves of northeast Florida Bay. Estuaries 22, 500–517.
MacVicar, T., Van Lent, T., and Castro, A. (1984). South Florida Water Management Model Documentation Report. Technical Report, Report No. 84-3. Brooksville, FL: South Florida Water Management District.
Marshall, F. E., Smith, D. T., and Nickerson, D. M. (2011). Empirical tools for simulating salinity in the estuaries in Everglades National Park, Florida. Estuar. Coast. Shelf Sci. 95, 379–387. doi: 10.1016/j.ecss.2011.10.001
Marshall, F. E., and Wingard, G. L. (2012). Florida Bay Salinity and Everglades Wetlands Hydrology Circa 1900 CE: A Compilation of Paleoecology-Based Statistical Modeling Analyses. Open-File Report No. 2012–1054. Reston, VA: U.S. Geological Survey. doi: 10.3133/ofr20121054
Marshall, F. E., Wingard, G. L., and Pitts, P. (2009). A simulation of historic hydrology and salinity in Everglades National Park: coupling paleoecologic assemblage data with statistical models. Estuar. Coast. 32, 37–53. doi: 10.1007/s12237-008-9120-1
Marshall, F. E., Wingard, G. L., and Pitts, P. A. (2014). Estimates of natural salinity and hydrology in a subtropical estuarine ecosystem: implications for Greater Everglades restoration. Estuar. Coast. 37, 1449–1466. doi: 10.1007/s12237-014-9783-8
McPherson, B. F., and Halley, R. (1996). The South Florida Environment: A Region Under Stress. Circular No. 1134. Reston, VA: U.S. Geological Survey. doi: 10.3133/cir1134
McVoy, C. W., Said, W. P., Obeysekera, J., VanArman, J. A., and Dreschel, T. W. (2011). Landscapes and Hydrology of the Predrainage Everglades. Gainesville, FL: University Press of Florida.
National Research Council (2003). Science and the Greater Everglades Ecosystem Restoration. Washington, DC: National Academy Press. doi: 10.17226/10589
National Research Council (2010). Progress Toward Restoring the Everglades: The Third Biennial Review. Washington, DC: National Academy Press. doi: 10.17226/12988
Obeysekera, J., Browder, J., Hornung, L., and Harwell, M. A. (1999). The natural South Florida system I: climate, geology, and hydrology. Urban Ecosyst. 3, 223–244. doi: 10.1111/gcb.14904
Ogden, J. C., Davis, S. M., Jacobs, K., Barnes, T., and Fling, H. E. (2005). The use of ecological models to guide ecosystem restoration in South Florida. Wetlands 25, 795–809. doi: 10.1007/s00267-013-0189-3
Olmsted, I., and Armentano, T. (1997). Vegetation of Shark Slough, Everglades National Park. Technical Report, Report No. 97-001. Homestead, FL: South Florida Natural Resources Center.
Overpeck, J. T., Webb, T. III, and Prentice, I. C. (1985). Quantitative interpretation of fossil pollen spectra: dissimilarity coefficients and the method of modern analogs. Quat. Res. 23, 87–108. doi: 10.1016/0033-5894(85)90074-2
Parker, G. G., Ferguson, G. E., and Love, S. K. (1955). Water Resources of Southeastern Florida. Water Supply Paper No. 1255. Reston, VA: U.S. Geological Survey. doi: 10.3133/wsp1255
Probst, R. J., and Rosendahl, P. C. (1981). Shark Slough Water Level Correlation Analysis. Report No. T-618. Miami, FL: South Florida Research Center.
Renken, R. A., Dixon, J., Koehnstedt, J., Lietz, A. C., Ishman, S., Marella, R. L., et al. (2005). Impact of Anthropogenic Development on Coastal Ground-Water Hydrology in Southeastern Florida, 1900-2000. Circular No. 1275. Reston. VA: U.S. Geological Survey. doi: 10.3133/cir1275
Saunders, C. J., Gao, M., Lynch, J., Jaffe, R., and Childers, D. L. (2006). Using soil profiles of seeds and molecular markers as proxies for sawgrass and wet prairie slough vegetation in Shark River Slough, Everglades National Park. Hydobiologia 569, 475–492. doi: 10.1007/s10750-006-0150-z
Sklar, F. H., Chimney, M. J., Newman, S., McCormick, P., Gawlik, D., Miao, S., et al. (2005). The ecological–societal underpinnings of everglades restoration. Front. Ecol. Environ. 33:161–169. doi: 10.1890/1540-9295(2005)003[0161:TEUOER]2.0.CO;2
Smith, T. J., Hudson, J. H., Robblee, M. B., Powell, G. V. N., and Isdale, P. J. (1989). Freshwater flows from the Everglades to Florida Bay: a historical reconstruction based on fluorescent banding in the coral Solenastrea bournoni. Bull. Mar. Sci. 44, 274–282.
South Florida Water Management District (2005). Documentation for the South Florida Water Management Model Version 5.5. West Palm Beach, FL: South Florida Water Management District.
South Florida Water Management District (2008). Quick Facts on Modified Water Deliveries to Everglades National Park. Splash! Factsheet. West Palm Beach, FL: South Florida Water Management District.
U. S. Army Corps of Engineers, and South Florida Water Management District (1999). Central and Southern Florida Project Comprehensive Review Study: Final Integrated Feasibility Report and Programmatic Environmental Impact Statement. West Palm Beach, FL: South Florida Water Management District.
U.S. Army Corps of Engineers and U.S. Department of the Interior (2015). Central & Southern Florida Project: Report to Congress, Comprehensive Everglades Restoration Plan. West Palm Beach, FL: South Florida Water Management District.
Van Lent, T., Johnson, R., and Fennema, R. (1993). Water Management in Taylor Slough and Effects on Florida Bay. Technical Report, Report No. 93-03. Homestead, FL: South Florida Natural Resources Center.
Wachnicka, A., Gaiser, E., and Collins, L. S. (2013a). Correspondence of historic salinity fluctuations in Florida Bay, USA, to atmospheric variability and anthropogenic changes. J. Paleoliminol. 49, 103–115. doi: 10.1007/s10933-011-9534-9
Wachnicka, A., Gaiser, E., Wingard, G. L., Briceño, H., and Harlem, P. (2013b). Impact of the late Holocene climate variability and anthropogenic activities on Biscayne Bay (Florida, U.S.A.) environment: evidence from diatoms. Palaeogeogr. Palaeoclimatol. Palaeoecol. 371, 80–92. doi: 10.1016/j.palaeo.2012.12.020
Wachnicka, A. H., and Wingard, G. L. (2015). “Biological indicators of changes in water quality and habitats of the coastal and estuarine areas of the Greater Everglades Ecosystem,” in Microbiology of the Everglades Ecosystem, eds J. A. Entry, A. D. Gottlieb, K. Jayachandrahan, and A. Ogram (Boca Raton, FL: CRC Press), 218–240. doi: 10.1201/b18253-14
Willard, D. A., and Bernhardt, C. E. (2011). Impacts of past climate and sea level change on Everglades wetlands: placing a century of anthropogenic change into a late-Holocene context. Clim. Change 107, 59–80. doi: 10.1007/s10584-011-0078-9
Willard, D. A., Bernhardt, C. E., Holmes, C. W., Landacre, B., and Marot, M. (2006). Response of Everglades tree islands to environmental change. Ecol. Monogr. 76, 565–583. doi: 10.1890/0012-9615(2006)076%5B0565:roetit%5D2.0.co;2
Willard, D. A., Bernhardt, C. E., Holmes, C. W., Landacre, B., and Marot, M. (2007). Response of Everglades tree islands to environmental change. Ecol. Monogr. 76, 565–583. doi: 10.1890/0012-9615(2006)076%5B0565:roetit%5D2.0.co;2
Willard, D. A., Bernhardt, C. E., Weimer, L., Cooper, S. R., Gamez, D., and Jensen, J. (2004). Atlas of pollen and spores of the Florida Everglades. Palynology 28, 175–227. doi: 10.2113/28.1.175
Willard, D. A., Weimer, L. M., and Riegel, W. L. (2001). Pollen assemblages as paleoenvironmental proxies in the Florida Everglades. Rev. Palaeobot. Palynol. 113, 213–235. doi: 10.1016/s0034-6667(00)00042-7
Wingard, G. L., Bernhardt, C. E., and Wachnicka, A. H. (2017). The role of paleoecology in restoration and resource management – the past as a guide to future decision-making: review and example from the Greater Everglades Ecosystem, U.S.A. Front. Ecol. Evol. 5:11. doi: 10.3389/fevo.2017.00011
Wingard, G. L., and Hudley, J. W. (2012). Application of a weighted-averaging method for determining paleosalinity: a tool for restoration of south Florida’s estuaries. Estuar. Coast. 35, 262–280. doi: 10.1007/s12237-011-9441-3
Wingard, G. L., Hudley, J. W., Holmes, C. W., Willard, D. A., and Marot, M. (2007). Synthesis of Age Data and Chronology for Florida Bay and Biscayne Bay Cores Collected for the Ecosystem History of South Florida’s Estuaries Projects. Open File Report No. 2007-1203. Reston, VA: U.S. Geological Survey, 127.
Keywords: paleoecology, palynology, freshwater wetlands, historic hydrology, Everglades restoration, statistical modeling and simulation, hydrologic modeling
Citation: Marshall FE, Bernhardt CE and Wingard GL (2020) Estimating Late 19th Century Hydrology in the Greater Everglades Ecosystem: An Integration of Paleoecologic Data and Models. Front. Environ. Sci. 8:3. doi: 10.3389/fenvs.2020.00003
Received: 30 July 2019; Accepted: 09 January 2020;
Published: 31 January 2020.
Edited by:
Carmen De Jong, Université de Strasbourg, FranceReviewed by:
Michal Kowalewski, Florida Museum of Natural History, United StatesWilliam Nuttle, University of Maryland Center for Environmental Science (UMCES), United States
Copyright © 2020 Marshall, Bernhardt and Wingard. This is an open-access article distributed under the terms of the Creative Commons Attribution License (CC BY). The use, distribution or reproduction in other forums is permitted, provided the original author(s) and the copyright owner(s) are credited and that the original publication in this journal is cited, in accordance with accepted academic practice. No use, distribution or reproduction is permitted which does not comply with these terms.
*Correspondence: G. Lynn Wingard, bHdpbmdhcmRAdXNncy5nb3Y=