- 1Department of Wildland Resources and the Ecology Center, Utah State University, Logan, UT, United States
- 2Biodiversity Research/Systematic Botany, University of Potsdam, Potsdam, Germany
- 3Berlin-Brandenburg Institute of Advanced Biodiversity Research, Berlin, Germany
Plant-soil feedbacks (PSFs) have become a commonly invoked mechanism of plant coexistence and abundance. Yet, most PSF experiments have been performed in greenhouse conditions. To test whether or not greenhouse-measured PSF values are of similar magnitude and positively correlated with field-measured PSFs, we compared PSF values from five different studies that measured PSF values in both greenhouse and field conditions. For 36 plant species, greenhouse-measured PSF values were larger than and not positively correlated with field-measured PSF values. Similarly, these 36 species produced 269 soil-specific PSF values, and for each site there was no positive correlation between these greenhouse- and field-measured PSF values. While PSFs were observed in both greenhouse and field conditions, results provided no support at the soil, site or species level that a positive correlation exists between greenhouse- and field-measured PSF. Further, greenhouse-measured PSF appear to overestimate field-measured PSF. Although from five studies, results strongly suggest that field experiments are needed to understand the role of PSFs in plant communities in natural settings.
Introduction
Plant-soil feedbacks (PSFs) are increasingly used to explain plant community dynamics including succession, invasion, legacy effects, landscape abundance, coexistence, and biodiversity (Klironomos, 2002; Kardol et al., 2006; van der Putten et al., 2013). However, PSF research continues to rely mostly on greenhouse experiments (Figure 1). Greenhouse PSF studies are useful for developing conceptual models of plant community dynamics (Bever et al., 1997; Bonanomi et al., 2005; Aguilera, 2011), however, it remains largely untested whether or not PSFs measured in the greenhouse are correlated with PSFs measured in the field (Kulmatiski and Kardol, 2008; Schittko et al., 2016).
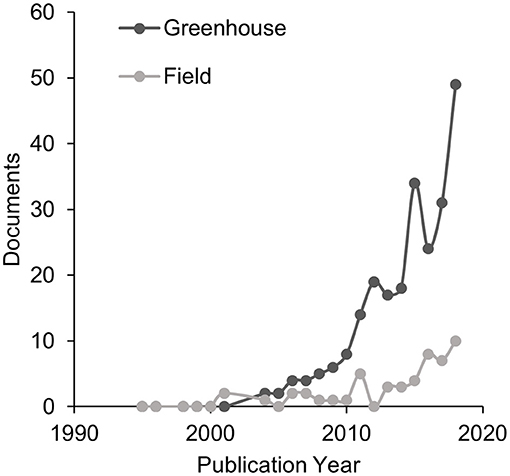
Figure 1. A Scopus search performed on March 18, 2019 for “plant-soil feedback” OR “plant-soil feedbacks” in abstract, title, or keyword of published articles from 1995 to 2018 demonstrated an exponential increase in PSF research over the past 20 years. Dark gray indicates greenhouse studies and gray indicates field studies.
Plants can alter soil biota, and these changes in soil biota may subsequently affect their own growth and the growth of neighboring plants (Reynolds et al., 2003; Ehrenfeld et al., 2005). PSFs are typically investigated by testing a plant's growth response to soils cultivated by different plant species (Bever, 1994). Many approaches have been used to test PSF effects (Kulmatiski and Kardol, 2008) including unsterilized vs. sterilized soils, comparisons among different field soil inoculum into sterilized soils, microbial filtrate inoculations, and two-phase experiments in which soil types are cultivated during an experiment. The two-phase approach remains a standard approach (Bever et al., 1997; van der Putten et al., 2013). In a two-phase experiment, during the conditioning phase of the bioassay (Phase 1), plants are used to create a soil with biota specific to that species. In the response phase (Phase 2), phytometers are planted to test the growth response of a species to the altered soil biota. Growth of the Phase 2 species on soil previously conditioned by the same plant (“home”) is compared to growth on soil previously conditioned by a different plant (“away”). By using soil from Phase 1 to inoculate sterilized soils, this approach can isolate microbial from soil chemical (Ehrenfeld et al., 2005; Morris et al., 2009; Ke et al., 2015) and physical (Kyle, 2005; Kulmatiski et al., 2017) effects.
PSF studies are typically executed in the greenhouse for several reasons. Greenhouse studies allow for many isolated replicates and can be performed throughout the year in rapid growth conditions. Because it is relatively easy to sterilize greenhouse soils, greenhouse studies more easily control legacy effects and separate soil nutrient effects from soil microbial effects, relative to field studies. However, completely isolating microbial from nutrient PSF may be unrealistic (Kulmatiski and Kardol, 2008; Ke et al., 2015). Greenhouse studies also lack microsite variability which can increase the likelihood of detecting PSFs in the greenhouse (Burns et al., 2015; Rinella and Reinhart, 2017).
Abiotic and biotic conditions can be very different between the greenhouse and the field (Heinze et al., 2016; Schittko et al., 2016). Greenhouse soils are typically sterilized and inoculated with small amounts of live soil; this likely creates soil conditions favoring fast-growing microbes and fast-growing plant species (Eno and Popenoe, 1964; De Deyn et al., 2004; Howard et al., 2017). Frequent fertilization and watering can cause arbuscular mycorrhizal fungi to become parasitic as conditions change from low to high fertilization regimes, and dry to wet water regimes (Johnson et al., 2003; Schmidt et al., 2011). This could cause PSF to appear neutral or positive in dry field conditions, and negative or neutral in a greenhouse with a consistent water regime (Mohan et al., 2014). Large soil organisms are typically absent in greenhouses which would affect plant-soil interactions (Kutáková et al., 2018), such as below-ground herbivory (Hol et al., 2010; Bezemer et al., 2013). More broadly, stressful conditions found in field studies may induce greater facilitation and a more positive PSF in the field (Maestre et al., 2009). These differences have led several authors to recommend greater field experimentation (Kulmatiski and Kardol, 2008; Heinze et al., 2016; Schittko et al., 2016).
Here, our goal was to test whether or not greenhouse-measured PSFs are of a similar magnitude and positively correlated with field-measured PSFs. We predicted that greenhouse- and field-measured PSF would be positively correlated because we expected that plants have a dominant effect on soil microbial community composition and subsequent PSF; these effects should be similar in both settings due to similar plant species and soil microbial communities. A negative correlation or a lack of correlation between greenhouse- and field-measured PSF suggests that greenhouse conditions change plant-soil interactions in ways that reverse or change PSF values. To test this prediction, we compared greenhouse- and field-measured PSF values from published studies and publicly available datasets. To assess whether PSF is overestimated in greenhouse or field conditions, we compared the magnitude of PSF values (regardless of sign) by taking the absolute values of greenhouse- and field-measured PSF.
Methods
A Scopus search for PSF studies with the term “plant-soil feedback” or “plant-soil feedbacks” in the title, abstract, or keywords was performed on March 19, 2019. Of the resulting 515 studies, meta-analyses, modeling papers, reviews, and non-English studies were removed. The remaining studies were reviewed to identify studies containing (1) a home/away PSF method (Brinkman et al., 2010), (2) aboveground biomass or cover as the response variable, and (3) grasslands as the study ecosystem (Burns et al., 2017; Teste et al., 2017), which left 297 studies. Species from grassland ecosystems were selected as the focal organisms because most PSF research has been conducted in grassland ecosystems, so sufficient sample sizes from non-grassland ecosystems were unlikely (Kulmatiski et al., 2008; van der Putten et al., 2013). Of these 297 studies, 237 occurred in the greenhouse, 50 in the field, seven in mesocosms, and three included both greenhouse and field approaches. Of these three studies, data was collected from two, but one possible study did not respond to requests for data. An additional three datasets produced by the authors, which are publicly available at the USU Digital Commons, were also included.
The resulting dataset contained paired greenhouse-measured and field-measured PSF values for 36 species derived from 2,975 field observations and 2,907 greenhouse observations at five different study sites. We used the paired dataset to (1) calculate PSF values using a single method for all data, (2) test for correlations between greenhouse- and field-measured PSF, and (3) compare PSF values and PSF magnitudes (absolute values) between greenhouse- and field-measured PSF.
Study Sites
Of the five study sites included, three were from Europe (Berlin, Potsdam, and Jena in Germany) and two were from North America (Winthrop, Washington and Cedar Creek, Minnesota in the United States). At all sites, the focal species selected were abundant in local plant communities. Four species were common among at least two study sites (Supplementary Material).
All five studies compared phytometer growth responses to “home” and “away” conditioned soil (Bever, 1994). When more than two species are used in a PSF experiment, this comparison can be undertaken by mixing all conditioned “away” soils together to create a single “away” treatment. This approach was used in the Berlin study; it eliminates site-by-site variation in soil microbes (Reinhart and Rinella, 2016; Rinella and Reinhart, 2017), and can be useful when the research question is not focused on spatial variability (Cahill et al., 2017; Gundale et al., 2017). Alternately, phytometer responses can be measured on each “away” soil creating a species*soil-level design. This approach was used in the studies at Cedar Creek, Jena, Potsdam, and Winthrop. Data from species*soil-level PSF experiments were converted to species-level PSF values by averaging a species' growth across “away” soil types.
Greenhouse Experiments
The experiments at Cedar Creek, Jena, and Winthrop implemented a cultivated two-phase approach (Rinella and Reinhart, 2018). The experiments at Berlin and Potsdam collected conditioning soils from underneath monotypic stands in the field (Kulmatiski and Kardol, 2008) (Table 1).
For Phase 1, the Cedar Creek greenhouse experiment steam-sterilized a six-to-one mixture of sand and sphagnum peat inoculated with ten percent field soil. The prepared 1-L pots were planted and grown for a 6-months Phase 1. The Jena greenhouse experiment inoculated a three-to-one mixture of compost and sand with ten percent field soil. The prepared 1-L pots were planted and grown for an 8-months Phase 1. The Winthrop greenhouse experiment steam-sterilized a six-to-one mixture of coarse sand and sphagnum peat and inoculated with five percent field soil. The prepared 1-L pots were planted and grown for a 3-months Phase 1. At the end of Phase 1, plants were removed by hand-clipping; 2,282 pots at Cedar Creek, 239 pots at Jena, and 216 pots at Winthrop had growth.
For Phase 1, the greenhouse experiment at Potsdam collected field soil from underneath three different species' monotypic stands and filled 90 0.41-L pots with 100% field soil (Heinze et al., 2016). In Berlin, Schittko et al. (2016) collected field soil from underneath eight different species' monotypic stands. The soil for the “away” treatment was mixed, where the soil for the “home” treatment was not mixed. A steam-sterilized sandy loam soil was inoculated with 23% “home” or “away” soils collected in the field and used to fill 240 pots, 80 of which were retained for the greenhouse experiment.
For the greenhouse experiment at Cedar Creek, the Phase 2 length was 6 months; at Jena 3 months; at Winthrop, 3 months; at Potsdam two and one-third months; and at Berlin 4 months. Pots were clipped and aboveground biomass weighed for all species at the end of Phase 2 (Table 1).
Field Experiments
At Cedar Creek and Jena, the field site area was sprayed with glyphosate and disked. Experimental plots (0.35/0.75 m) were established with 0.75 mm thick HDPE root barrier inserted to 35 cm deep between each plot. For Phase 1 at Cedar Creek, ten grams of pure live seed per m2 was applied to each of the plots. At Jena, 2,000 total pure live seeds per m2 were applied to each of the plots. After a 2-years Phase 1, the area was sprayed with glyphosate and hand-tilled using a garden claw. Non-target species were removed by hand-weeding. At Cedar Creek, plots containing C3 grasses and forbs were hand-tilled using a garden claw, but vigorous root growth in the C4 grasses necessitated tilling using a miniature tiller on plots containing that functional group. Seed was re-applied at the same respective rates. After a 2-years Phase 2, aboveground biomass was clipped, dried and weighed; 2,066 Cedar Creek plots and 345 Jena plots had growth.
At Winthrop, the top 10 cm of vegetation and soil was removed (Kulmatiski, 2019). A one-to-one mix of native soil inoculum and sand was applied to the prepared site, and disked to 15 cm to homogenize. A grid of 1.2 m wide geotextile cloth was laid down to create 315 1.5/1.5 m PSF plots in the area. Ten grams of pure live seed per m2 was applied to each plot, and allowed to grow for a 4-years Phase 1. After 4 years, Phase 1 plants were sprayed with glyphosate. Seed was re-applied for Phase 2 and plots were allowed to grow for 3 years. Growth was estimated using percent cover in June 2013.
At Potsdam, 30 (0.4/0.4 m) plots were prepared by cutting the first 25 cm of roots under three different monotypic stands to create three Phase 1 treatments (Heinze et al., 2016). Three individuals of each species were planted in each plot. Individuals were spaced 10 cm apart and allowed to grow for 10 weeks. After the 10 weeks, aboveground biomass was harvested, and 89 individuals had growth.
At Berlin, at week 14 of the greenhouse experiment, 160 pots were transferred to the field and left to sit on top of the soil for a period of 2 weeks (Schittko et al., 2016). After 2 weeks, the aboveground biomass was harvested. Extended methods for Cedar Creek and Jena are in Supplementary Material; for Potsdam, Winthrop, and Berlin extended methods are in Heinze et al. (2016), Kulmatiski et al. (2011, 2017), and Schittko et al. (2016).
Statistical Analyses
To avoid bias from different calculation methods, original plant growth data on “home” and “away” soils was used to calculate PSF values using a single method for all data (Brinkman et al., 2010). PSFs were calculated as (H-A)/maximum (H,A), where H is the aboveground growth (ground cover or biomass) produced by a species in Phase 2 on “home” soils, and A is the aboveground growth produced by a species in Phase 2 on “away” soils. The denominator refers to the maximum aboveground growth produced by a species regardless of soil type. This calculation has similar mathematical properties to the commonly used ln(H/A) metric (i.e., values that are symmetric around zero and bounded between +1 and −1). In addition, it has the advantage of being easily interpretable as the proportion increase or decrease in growth due to soil type (Brinkman et al., 2010). Plots or pots where the Phase 1 or the Phase 2 realized no growth were removed from the dataset. To prepare the data from species*soil-level PSF studies for a species-level analysis, one PSF value was calculated for each “away” species by taking the mean PSF value for each species across soil types.
To determine if the mean PSF value for each experiment was different from zero, we took the standard error of the mean. For data from species-level PSF studies, one home vs. away PSF was calculated for each species. For species-level PSF values, we used linear models to test for a correlation between greenhouse- and field-measured PSF within each study site and overall. For species*soil-level PSF values, we used linear models to test for a correlation between greenhouse- and field-measured PSF within each study site only, to control for the outsized effect of Cedar Creek's data on the overall dataset. Linear models were performed using the polyfit and fitlm scripts in MATLAB (The MathWorks Inc., 2015). Residuals for the species-level data were checked for normality using the Shapiro-Wilk test.
Comparisons
To compare PSF values and PSF magnitudes (absolute values) among study sites and regions, we performed a one-way analysis of variance (ANOVA) using the script anova1 in MATLAB. Significance was evaluated at α = 0.05. When significant, differences were explored with a Tukey's Honest Significant Difference test in MATLAB using the script multcompare.
Results
From species-level data, 36 paired PSF values were compared. Of these 36 values, eight came from the mixed-soil PSF experiment at Berlin, and the remainder from species*soil-level studies where the mean PSF value across all soil types was calculated to create a single PSF value per plant species: 16 PSF values came from Cedar Creek, five from Jena, three from Potsdam, and four from Winthrop. Greenhouse PSF values were positive in Berlin and Potsdam, and neutral in Jena, Winthrop, and Cedar Creek (Figure 2A). Field PSFs were positive in Berlin and Winthrop, neutral in Jena and Potsdam, and negative in Cedar Creek (Figure 2A). For the species-level greenhouse-measured data the average PSF was 0.046 and the coefficient of variance was 5.14; for the field-measured data the average PSF was −0.008 and the coefficient of variance was 24.01.
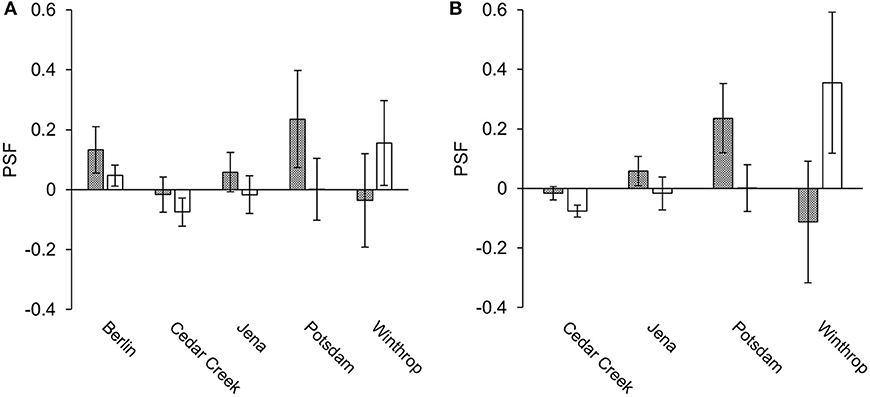
Figure 2. Average greenhouse- and field-measured species-level (A) and species*soil-level (B) PSF values (mean ± SE). Gray indicates greenhouse studies and white indicates field studies.
A total of 269 PSF values from species*soil-level field/greenhouse paired experiments were compared. Of these values, 239 came from the Cedar Creek study, 20 from the Jena study, six from the Potsdam study, and four from the Winthrop study. PSF values for Berlin were excluded from the species*soil-level dataset because the study was not species*soil-level in design. Greenhouse PSF values were positive in Potsdam and Jena, and neutral in Winthrop and Cedar Creek (Figure 2B). Field PSF values were positive in Winthrop, neutral in Jena and Potsdam, and negative in Cedar Creek (Figure 2B). For the species*soil-level greenhouse-measured data the average PSF was −0.007 and the coefficient of variance was 50.59; for the field-measured data the average PSF was −0.064 and the coefficient of variance was 4.81.
We tested for correlations in species-level data both within and among sites. For species*soil-level data we tested within but not among sites because 86% of species-level data was from one site. For species-level data, there was no correlation between greenhouse- and field-measured PSF values across all study sites (F1,34 = 0.179, P = 0.675, Figure 3A). Similarly, there was no correlation between greenhouse- and field-measured PSF values within study sites (P > 0.05, Figure 3A). For the species*soil-level PSFs, there was no correlation between greenhouse- and field-measured PSF values at the Cedar Creek, Jena, and Winthrop sites (F1,237 = 0.001, P = 0.972; F1,18 = 0.003, P = 0.959; and F1,2 = 0.039, P = 0.801; respectively; Figure 3B). There was a negative correlation between greenhouse- and field-measured data from the Potsdam site (F1,4 = 10.129, P = 0.034, R2 = 0.717; Figure 3B).
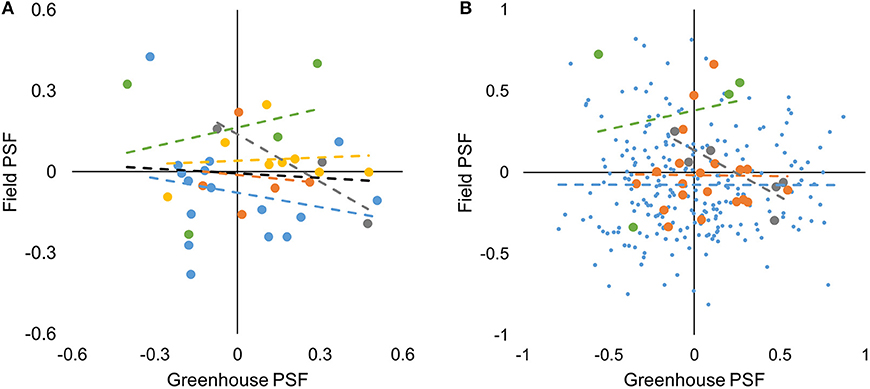
Figure 3. Greenhouse- vs. field-measured species-level (A) and species*soil-level (B) PSF values from five study sites. Yellow, Berlin; blue, Cedar Creek; orange, Jena; gray, Potsdam; green, Winthrop; black is a best-fit line for all study sites. Though only significant for the Potsdam species*soil-level data, best-fit regression lines are shown for each site to demonstrate that slopes were close to zero.
We tested for differences in magnitude (absolute value) for species-level data only because of the strong effects Cedar Creek had on species*soil-level data. While there were few correlations between greenhouse- and field-measured PSF values, there were differences between the magnitude of greenhouse- and field-measured PSF values, indicating that PSF (either positive or negative) were larger in greenhouse than field conditions (F1,70 = 5.056, P = 0.028).
Discussion
Although PSFs are commonly invoked as a mechanism to explain complex plant community dynamics in the field, the majority of PSF experiments take place in controlled greenhouse conditions. We had predicted that greenhouse- and field-measured PSF would be positively correlated due to the dominant effects of plants on their soil microbial communities, but found no evidence to suggest that greenhouse-measured PSF data are positively correlated with field-measured PSF. We also found greenhouse-measured PSF values were exaggerated relative to field-measured PSF values. Together, results suggest that the greenhouse-measured PSFs that predominate in the literature both overestimate and provide little direct inference into PSF effects in the field. Although our dataset is derived from only five sites, our results strongly suggest that PSFs are sensitive to growth conditions (Casper et al., 2008). Consequently, field experiments are likely to be needed to fully understand the role of PSFs in natural systems.
There are several potential reasons that could explain why PSF values were smaller in the field than in the greenhouse. More stressful growing conditions (for example, competition, drought, or herbivory) may minimize PSF effects (van der Putten et al., 2016; Crawford and Knight, 2017; Fry et al., 2018). Although researchers in all five field experiments attempted to decrease competitive effects by hand-weeding, it is likely that competitive pressure was still greater in the field than greenhouse experiments due to the larger seed bank in unsterilized field soils (Lekberg et al., 2018). Similarly, greater aboveground herbivory in the field was likely to decrease PSF values directly by removing aboveground biomass and potentially indirectly by inducing increased belowground growth (Heinze and Joshi, 2018). Drought in the field may also decrease PSF values by decreasing plant growth, microbial growth, and nutrient cycling rates (van der Putten et al., 2016). With only five studies and many potential factors affecting differences between greenhouse and field results, it was not possible to test these hypotheses, but they are consistent with our observation of larger PSF values in the greenhouse.
Methodological differences were likely to explain why there was no positive correlation between field and greenhouse PSF values, though we were unable to isolate any specific methodological difference that would explain our results. Compared to the field, growing space is restricted, experiment length is shorter, and dominant soil microbes differ in the greenhouse. Excepting Berlin, greenhouse pots were smaller than field plots. Yet, we did not observe a qualitatively different relationship between greenhouse and field PSF values at Berlin. The Winthrop site had the largest difference between field plot and greenhouse pot size, yet PSF values were not notably different from other sites.
Differences in temporal scales among sites similarly did not appear to drive our results. PSFs have been suggested to accumulate over time (Kardol et al., 2006; Kulmatiski et al., 2008; Diez et al., 2010; Lepinay et al., 2018), but Potsdam, which had similar greenhouse and field experiment lengths, did not have a positive correlation between greenhouse- and field-measured PSF. Sterilized soils, which were used at three of the five reviewed experiments, often have higher nutrient availability and promote faster plant growth, changing PSF values and soil microbial communities that drive PSF (De Deyn et al., 2004). However, sites using sterilized soils and sites using unsterilized soils both had uncorrelated PSF values. Little can be inferred from the five studies reviewed, but results did not provide strong evidence to suggest that pot size, experiment length, or sterilization technique provided a strong explanation for the difference between greenhouse and field results.
The only correlation observed between greenhouse- and field-measured PSF, was a negative correlation at the Potsdam site. This site was the only site to use 100% field soil in the greenhouse experiment. It is possible that a negative correlation occurred because under decreasing light conditions PSF can be reversed (Smith and Reynolds, 2015), but it is not clear why this effect would only appear when 100% field soils were used. To the contrary, we would have expected that the use of 100% field soil would produce more similar results to the field.
Although our results and results from previous studies suggest that PSF values are very context-dependent (Casper and Castelli, 2007), the PSF concept remains relevant to plant community ecology. Greenhouse-measured PSFs have been found to improve predictions of plant growth in communities in the greenhouse (Kulmatiski et al., 2011, 2017) and field-measured PSFs have been found to improve predictions of plant growth in communities in the field (Klironomos, 2002; Kardol et al., 2006; Mangan et al., 2010; Mariotte et al., 2018; Kulmatiski, 2019). Thus, our results suggest that while greenhouse studies are useful for conceptual model development and predicting plant growth in greenhouse conditions, ecologists who wish to understand the role of PSFs for specific plant species in the field should rely on field studies.
While from five studies, our results suggest that the PSF literature, which is predominantly derived from greenhouse experiments, overestimates PSF effects and while it may provide insight into general patterns of interactions that occur in plant communities in the field, it provides little insight into the specific PSFs that determine the growth and abundance of specific plants in natural communities. Our findings are consistent with results from previous studies (Heinze et al., 2016; Schittko et al., 2016), and suggest that although greenhouse-measured PSFs are important for conceptual models, field experiments will likely be needed to understand the role of PSFs in complex plant community dynamics in the field.
Data Availability Statement
The Potsdam dataset analyzed for this study is available on request from JH. The Cedar Creek, Jena, and Winthrop datasets analyzed for this study can be found at USU Digital Commons (https://doi.org/10.26078/52k0-jr94, https://doi.org/10.15142/T3XM19). The Berlin dataset analyzed for this study can be found in the Dryad Digital Repository (https://doi.org/10.5061/dryad.r7c23).
Author Contributions
LF and AK coordinated the writing. LF coded the papers included in the literature search, and conducted the meta-analyses with input from AK, JG, JH, and CS. LF, JG, and AK performed the studies at Cedar Creek and Jena. AK performed the study at Winthrop. JH performed the study at Potsdam. CS performed the study at Berlin. All co-authors participated in generating the ideas discussed here.
Funding
CS was funded by the German Federal Ministry of Education and Research BMBF within the Collaborative Project Bridging in Biodiversity Science–BIBS (funding number 01LC1501A-H). LF, JG, and AK were supported by the National Science Foundation (funding number 1354129) and the Utah State University Ecology Center.
Conflict of Interest
The authors declare that the research was conducted in the absence of any commercial or financial relationships that could be construed as a potential conflict of interest.
Acknowledgments
Susan Durham provided the statistical assistance. Karen Beard and Peter Adler assisted with the revisions, and Scott Kuehl-Shelby assisted with the proofreading. This research was supported by the Utah Agricultural Experiment Station, Utah State University, and approved as journal paper number 9252.
Supplementary Material
The Supplementary Material for this article can be found online at: https://www.frontiersin.org/articles/10.3389/fenvs.2019.00184/full#supplementary-material
References
Aguilera, A. G. (2011). The influence of soil community density on plant-soil feedbacks: an important unknown in plant invasion. Ecol. Model. 222, 3413–3420. doi: 10.1016/j.ecolmodel.2011.06.018
Bever, J. D. (1994). Feedback between plants and their soil communities in an old field community. Ecology 75, 1965–1977. doi: 10.2307/1941601
Bever, J. D., Westover, K. M., and Antonovics, J. (1997). Incorporating the soil community into plant population dynamics: the utility of the feedback approach. J. Ecol. 85, 561–573. doi: 10.2307/2960528
Bezemer, T. M., van der Putten, W. H., Martens, H., van de Voorde, T. F. J., Mulder, P. P. J., and Kostenko, O. (2013). Above- and below-ground herbivory effects on below-ground plant-fungus interactions and plant-soil feedback responses. J. Ecol. 101, 325–333. doi: 10.1111/1365-2745.12045
Bonanomi, G., Giannino, F., and Mazzoleni, S. (2005). Negative plant–soil feedback and species coexistence. Oikos 111, 311–321. doi: 10.1111/j.0030-1299.2005.13975.x
Brinkman, E. P., Van der Putten, W. H., Bakker, E.-J., and Verhoeven, K. J. F. (2010). Plant–soil feedback: experimental approaches, statistical analyses and ecological interpretations. J. Ecol. 98, 1063–1073. doi: 10.1111/j.1365-2745.2010.01695.x
Burns, J. H., Anacker, B. L., Strauss, S. Y., and Burke, D. J. (2015). Soil microbial community variation correlates most strongly with plant species identity, followed by soil chemistry, spatial location and plant genus. AoB Plants 7:plv030. doi: 10.1093/aobpla/plv030
Burns, J. H., Brandt, A. J., Murphy, J. E., Kaczowka, A. M., and Burke, D. J. (2017). Spatial heterogeneity of plant–soil feedbacks increases per capita reproductive biomass of species at an establishment disadvantage. Oecologia 183, 1077–1086. doi: 10.1007/s00442-017-3828-1
Cahill, J. F., Cale, J. A., Karst, J., Bao, T., Pec, G. J., and Erbilgin, N. (2017). No silver bullet: different soil handling techniques are useful for different research questions, exhibit differential type I and II error rates, and are sensitive to sampling intensity. New Phytol. 216, 11–14. doi: 10.1111/nph.14141
Casper, B. B., Bentivenga, S. P., Ji, B., Doherty, J. H., Edenborn, H. M., and Gustafson, D. J. (2008). Plant–soil feedback: testing the generality with the same grasses in serpentine and prairie soils. Ecology 89, 2154–2164. doi: 10.1890/07-1277.1
Casper, B. B., and Castelli, J. P. (2007). Evaluating plant–soil feedback together with competition in a serpentine grassland. Ecol. Lett. 10, 394–400. doi: 10.1111/j.1461-0248.2007.01030.x
Crawford, K. M., and Knight, T. M. (2017). Competition overwhelms the positive plant–soil feedback generated by an invasive plant. Oecologia 183, 211–220. doi: 10.1007/s00442-016-3759-2
De Deyn, G. B., Raaijmakers, C. E., and Putten, W. H. V. D. (2004). Plant community development is affected by nutrients and soil biota. J. Ecol. 92, 824–834. doi: 10.1111/j.0022-0477.2004.00924.x
Diez, J. M., Dickie, I., Edwards, G., Hulme, P. E., Sullivan, J. J., and Duncan, R. P. (2010). Negative soil feedbacks accumulate over time for non-native plant species. Ecol. Lett. 13, 803–809. doi: 10.1111/j.1461-0248.2010.01474.x
Ehrenfeld, J. G., Ravit, B., and Elgersma, K. (2005). Feedback in the plant-soil system. Annu. Rev. Environ. Resour. 30, 75–115. doi: 10.1146/annurev.energy.30.050504.144212
Eno, C. F., and Popenoe, H. (1964). Gamma radiation compared with steam and methyl bromide as a soil sterilizing agent 1. Soil Sci. Soc. Am. J. 28, 533–535. doi: 10.2136/sssaj1964.03615995002800040024x
Fry, E. L., Johnson, G. N., Hall, A. L., Pritchard, W. J., Bullock, J. M., and Bardgett, R. D. (2018). Drought neutralises plant–soil feedback of two mesic grassland forbs. Oecologia 186, 1113–1125. doi: 10.1007/s00442-018-4082-x
Gundale, M. J., Wardle, D. A., Kardol, P., Van der Putten, W. H., and Lucas, R. W. (2017). Soil handling methods should be selected based on research questions and goals. New Phytol. 216, 18–23. doi: 10.1111/nph.14659
Heinze, J., and Joshi, J. (2018). Plant-soil feedback effects can be masked by aboveground herbivory under natural field conditions. Oecologia 186, 235–246. doi: 10.1007/s00442-017-3997-y
Heinze, J., Sitte, M., Schindhelm, A., Wright, J., and Joshi, J. (2016). Plant-soil feedbacks: a comparative study on the relative importance of soil feedbacks in the greenhouse versus the field. Oecologia, 181, 1–11. doi: 10.1007/s00442-016-3591-8
Hol, W. H. G., Boer, W. D., Termorshuizen, A. J., Meyer, K. M., Schneider, J. H. M., Dam, N. M. V., et al. (2010). Reduction of rare soil microbes modifies plant–herbivore interactions. Ecol. Lett. 13, 292–301. doi: 10.1111/j.1461-0248.2009.01424.x
Howard, M. M., Bell, T. H., and Kao-Kniffin, J. (2017). Soil microbiome transfer method affects microbiome composition, including dominant microorganisms in a novel environment. FEMS Microbiol. Lett. 364:fnx092. doi: 10.1093/femsle/fnx092
Johnson, N. C., Rowland, D. L., Corkidi, L., Egerton-Warburton, L. M., and Allen, E. B. (2003). Nitrogen enrichment alters mycorrhizal allocation at five mesic to semiarid grasslands. Ecology 84, 1895–1908. doi: 10.1890/0012-9658(2003)084[1895:NEAMAA]2.0.CO;2
Kardol, P., Martijn Bezemer, T., and van der Putten, W. H. (2006). Temporal variation in plant–soil feedback controls succession. Ecol. Lett. 9, 1080–1088. doi: 10.1111/j.1461-0248.2006.00953.x
Ke, P.-J., Miki, T., and Ding, T.-S. (2015). The soil microbial community predicts the importance of plant traits in plant–soil feedback. New Phytol. 206, 329–341. doi: 10.1111/nph.13215
Klironomos, J. N. (2002). Feedback with soil biota contributes to plant rarity and invasiveness in communities. Nature 417, 67–70. doi: 10.1038/417067a
Kulmatiski, A. (2019). Plant-soil feedbacks predict native but not non-native plant community composition: a 7-year common-garden experiment. Front. Ecol. Evol. 7:326. doi: 10.3389/fevo.2019.00326
Kulmatiski, A., Beard, K. H., Norton, J. M., Heavilin, J. E., Forero, L. E., and Grenzer, J. (2017). Live long and prosper: plant–soil feedback, lifespan, and landscape abundance covary. Ecology 98, 3063–3073. doi: 10.1002/ecy.2011
Kulmatiski, A., Beard, K. H., Stevens, J. R., and Cobbold, S. M. (2008). Plant–soil feedbacks: a meta-analytical review. Ecol. Lett. 11, 980–992. doi: 10.1111/j.1461-0248.2008.01209.x
Kulmatiski, A., Heavilin, J., and Beard, K. H. (2011). Testing predictions of a three-species plant–soil feedback model. J. Ecol. 99, 542–550. doi: 10.1111/j.1365-2745.2010.01784.x
Kulmatiski, A., and Kardol, P. (2008). “Getting plant—soil feedbacks out of the greenhouse: experimental and conceptual approaches,” in Progress in Botany, eds U. Lüttge, W. Beyschlag, and J. Murata (Berlin; Heidelberg: Springer), 449–472. doi: 10.1007/978-3-540-72954-9_18
Kutáková, E., Cesarz, S., Münzbergová, Z., and Eisenhauer, N. (2018). Soil microarthropods alter the outcome of plant-soil feedback experiments. Sci. Rep. 8:11898. doi: 10.1038/s41598-018-30340-w
Kyle, G. P. (2005). Mechanisms Influencing Invasive Plant Establishment Following Rodent Disturbance in a Shrub-Steppe Ecosystem. Utah State University, Logan, UT, United States.
Lekberg, Y., Bever, J. D., Bunn, R. A., Callaway, R. M., Hart, M. M., Kivlin, S. N., et al. (2018). Relative importance of competition and plant–soil feedback, their synergy, context dependency and implications for coexistence. Ecol. Lett. 21, 1268–1281. doi: 10.1111/ele.13093
Lepinay, C., Vondráková, Z., Dostálek, T., and Münzbergová, Z. (2018). Duration of the conditioning phase affects the results of plant-soil feedback experiments via soil chemical properties. Oecologia 186, 459–470. doi: 10.1007/s00442-017-4033-y
Maestre, F. T., Callaway, R. M., Valladares, F., and Lortie, C. J. (2009). Refining the stress-gradient hypothesis for competition and facilitation in plant communities. J. Ecol. 97, 199–205. doi: 10.1111/j.1365-2745.2008.01476.x
Mangan, S. A., Schnitzer, S. A., Herre, E. A., Mack, K. M. L., Valencia, M. C., Sanchez, E. I., et al. (2010). Negative plant-soil feedback predicts tree-species relative abundance in a tropical forest. Nat. Lond. 466, 752–755. doi: 10.1038/nature09273
Mariotte, P., Mehrabi, Z., Bezemer, T. M., Deyn, G. B. D., Kulmatiski, A., Drigo, B., et al. (2018). Plant–soil feedback: bridging natural and agricultural sciences. Trends Ecol. Evol. 33, 129–142. doi: 10.1016/j.tree.2017.11.005
Mohan, J. E., Cowden, C. C., Baas, P., Dawadi, A., Frankson, P. T., Helmick, K., et al. (2014). Mycorrhizal fungi mediation of terrestrial ecosystem responses to global change: mini-review. Fungal Ecol. 10, 3–19. doi: 10.1016/j.funeco.2014.01.005
Morris, C., Grossl, P. R., and Call, C. A. (2009). Elemental allelopathy: processes, progress, and pitfalls. Plant Ecol. 202, 1–11. doi: 10.1007/s11258-008-9470-6
Reinhart, K. O., and Rinella, M. J. (2016). A common soil handling technique can generate incorrect estimates of soil biota effects on plants. New Phytol. 210, 786–789. doi: 10.1111/nph.13822
Reynolds, H. L., Packer, A., Bever, J. D., and Clay, K. (2003). Grassroots ecology: plant–microbe–soil interactions as drivers of plant community structure and dynamics. Ecology 84, 2281–2291. doi: 10.1890/02-0298
Rinella, M. J., and Reinhart, K. O. (2017). Mixing soil samples across experimental units ignores uncertainty and generates incorrect estimates of soil biota effects on plants: response to Cahill et al. (2017) ‘No silver bullet: different soil handling techniques are useful for different research questions, exhibit differential type i and ii error rates, and are sensitive to sampling intensity'. New Phytol. 216, 15–17. doi: 10.1111/nph.14432
Rinella, M. J., and Reinhart, K. O. (2018). Toward more robust plant-soil feedback research. Ecology 99, 550–556. doi: 10.1002/ecy.2146
Schittko, C., Runge, C., Strupp, M., Wolff, S., Wurst, S., and Austin, A. (2016). No evidence that plant–soil feedback effects of native and invasive plant species under glasshouse conditions are reflected in the field. J. Ecol. 104, 1243–1249. doi: 10.1111/1365-2745.12603
Schmidt, B., Gaşpar, S., Camen, D., Ciobanu, I., and Sumălan, R. (2011). Arbuscular mycorrhizal fungi in terms of symbiosis-parasitism continuum. Commun. Agric. Appl. Biol. Sci. 76, 653–659.
Smith, L. M., and Reynolds, H. L. (2015). Plant–soil feedbacks shift from negative to positive with decreasing light in forest understory species. Ecology 96, 2523–2532. doi: 10.1890/14-2150.1
Teste, F. P., Kardol, P., Turner, B. L., Wardle, D. A., Zemunik, G., Renton, M., et al. (2017). Plant-soil feedback and the maintenance of diversity in Mediterranean-climate shrublands. Science 355, 173–176. doi: 10.1126/science.aai8291
The MathWorks Inc. (2015). MATLAB and Statistics Toolbox Release 2015b. Natick, MA: The MathWorks Inc.
van der Putten, W. H., Bardgett, R. D., Bever, J. D., Bezemer, T. M., Casper, B. B., Fukami, T., et al. (2013). Plant–soil feedbacks: the past, the present and future challenges. J. Ecol. 101, 265–276. doi: 10.1111/1365-2745.12054
Keywords: plant-soil feedback, environmental factors, above-belowground interactions, experimental environment, field experiment, greenhouse experiment
Citation: Forero LE, Grenzer J, Heinze J, Schittko C and Kulmatiski A (2019) Greenhouse- and Field-Measured Plant-Soil Feedbacks Are Not Correlated. Front. Environ. Sci. 7:184. doi: 10.3389/fenvs.2019.00184
Received: 25 July 2019; Accepted: 05 November 2019;
Published: 26 November 2019.
Edited by:
Hannes Schmidt, University of Vienna, AustriaReviewed by:
Jennifer A. Schweitzer, The University of Tennessee, Knoxville, United StatesRobin Heinen, Netherlands Institute of Ecology (NIOO-KNAW), Netherlands
Copyright © 2019 Forero, Grenzer, Heinze, Schittko and Kulmatiski. This is an open-access article distributed under the terms of the Creative Commons Attribution License (CC BY). The use, distribution or reproduction in other forums is permitted, provided the original author(s) and the copyright owner(s) are credited and that the original publication in this journal is cited, in accordance with accepted academic practice. No use, distribution or reproduction is permitted which does not comply with these terms.
*Correspondence: Leslie E. Forero, bGVzbGllZWZvcmVyb0BnbWFpbC5jb20=