- 1Division of Biological and Environmental Science and Engineering (BESE), Red Sea Research Center (RSRC), King Abdullah University of Science and Technology (KAUST), Thuwal, Saudi Arabia
- 2School of Environmental Science and Engineering, Guangzhou University, Guangzhou, China
We recently demonstrated the rapid adaptation of Red Sea phytoplankton to ocean warming, with associated constraints on physiological performance. However, the possible tradeoff between thermal adaptation and the organism's tolerance to other environmental drivers in a warmer future remains understudied. Here, we designed an evolutionary selection environment where the Red Sea diatom Chaetoceros tenuissimus was adapted to ambient (26°C) and warming (30°C) temperature scenarios for over 2,000 generations. These strains were subsequently exposed to a range of copper (Cu) dose over three assay temperatures (26, 30, and 35°C), to assess whether adaptation to experimental warming is accompanied by a reformed tolerance to toxic pollutants. Most previous studies on Cu toxicity in marine phytoplankton were conducted within a smaller range of temperature (20–25°C), indicating the need for further assessments to reveal the potential complex interactive effects between pollutants and more significant warming in the future. The acute Cu toxicity was estimated in terms of reduction in cell abundance (cells mL−1), growth rate (μ) and PSII photosynthetic efficiency (Fv/Fm), with 48 h median effective concentration values (EC50) ranging from 2.22 to 20.19 μg L−1. We found a statistically significant interaction between assay temperature, selection temperature, and Cu doses in all the criteria tested. However, under the extreme warming scenario (35°C), the Cu sensitivity was significantly reduced, indicating cumulative antagonistic effects between these factors. Adaptation of phytoplankton to higher temperatures may help maintain their heavy metal tolerance, although a shift in temperature during the tests clearly altered their sensitivities. We conclude that selection for warming had made cells more resistant to Cu at the selection temperature in comparison to ambient-adapted population tested at 26°C. However, in warming-adapted cells, this response was traded off against cupper resistance at 26°C.
Highlights
- Chaetoceros tenuissimus, isolated from the Red Sea, adapted rapidly to experimental warming.
- Adaptation to warming significantly increased the subsequent copper tolerance.
- Significant interaction was observed among selection temperature, copper dose, and assay temperature.
- High copper sensitivity of Red Sea taxa than reported elsewhere.
- Highlights the multi-stressor scenario of ecological impacts of persistent pollutants in the Red Sea.
Introduction
Global climate change and the subsequent increase in the surface temperature of the ocean are among the greatest concerns for marine ecosystems worldwide. The Intergovernmental Panel on Climate Change (IPCC) predicts a further rise in surface temperature, between 0.6 and 2.0°C, by the end of the century (Stocker et al., 2013), which is expected to have an unpredictable impact. Warming not only alters the physicochemical characteristics of the seawater but also triggers rapid evolutionary physiological responses in marine biota (Collins, 2011; Padfield et al., 2016). Among the complex issues of ocean warming is its interaction with chemical pollutants, and their environmental distribution and toxicity in particular (Noyes et al., 2009). For instance, experimental evidence shows that rising temperatures increase the toxicity of heavy metals, due to enhanced uptake and accumulation in tissues (Wang and Wang, 2008; Manciocco et al., 2014). For those species living at the edge of their physiological tolerance range, the complex interactions between ocean warming and pollutants may be particularly harmful due to their limited capacity to acclimate (Noyes et al., 2009).
The Red Sea hosts some of the most diverse marine habitats in the global oceans; however, recent evidence highlights its high susceptibility to rising temperatures as part of the widespread warming trend observed worldwide (Raitsos et al., 2011). The overall surface temperature of the Red Sea is increasing at a rate of 0.17 ± 0.07°C decade−1, and the northern Red Sea is warming even more quickly at a rate of 0.40–0.45°C per decade, which is approximately four times faster than the global average (Chaidez et al., 2017; Gittings et al., 2018). The impact of warming on pristine Red Sea habitats has been reported (Cantin et al., 2010).
Prolonged warming induces ecological modifications in phytoplankton, such as physiological acclimation and evolutionary adaptation to gradual temperature shifts (Irwin et al., 2015; Listmann et al., 2016; Padfield et al., 2016; Schaum et al., 2017). Evolutionary experiments have shown the capacity of phytoplankton to evolve over thousands of generations in response to variations in temperature and increasing pollution levels, aided by their rapid generation times and high population densities (Huertas et al., 2011). Recent reports have documented the development of marine phytoplankton tolerance to anthropogenic pollutants such as heavy metals (Marvá et al., 2014; Duarte et al., 2018), herbicides (Huertas et al., 2010) and petroleum products (Romero-Lopez et al., 2012) through adaptation. Consequently, these physiological and genetic adaptation mechanisms bring significant changes to the natural community structure when, after being exposed to pollutants, sensitive species are replaced by more tolerant ones (Rouco et al., 2014).
Persistent toxic substances (PTS) are an ongoing threat to marine ecosystems on both local and global scales. Heavy metals are one of the most persistent pollutants in coastal areas, harbors, and marinas, and they mainly originate from antifouling paints, industrial effluents and fuel combustion (Deheyn and Latz, 2006). In phytoplankton, heavy metals inhibit the electron transport chain and synthesis of photosynthetic pigments, and enzymes during photosynthesis, leading to a decline in photosynthetic efficiency, metabolism and growth (Pfeiffer et al., 2018). Complex interplays between heavy metal ions and the physicochemical characteristics of seawater collectively determine their availability for biological uptake. Several studies have addressed the interactive effects of temperature and heavy metals and found both antagonistic and synergistic effects in phytoplankton (Oukarroum et al., 2012; Morin et al., 2017). The toxicity of pollutants can be influenced by a changing climate (Schiedek et al., 2007), with a warming environment causing additional stress to phytoplankton. We still lack a holistic understanding on how ocean warming and toxic metals interact simultaneously in a multi-stressor scenario, however, the former is likely to alter the metal toxicity through changes in biological uptake rates and metal binding to organic ligands in marine organisms (Hoffmann et al., 2012). Prolonged warming can also result in phytoplankton adaptation at population or community levels (Huertas et al., 2011; Thomas et al., 2012). The cost of thermal adaptation, such as tradeoffs and physiological or metabolic modification (Padfield et al., 2016), is likely to alter the organism's response to other environmental stressors including the potential to acquire tolerance to toxicants. Since the majority of the reported studies in this line have examined the simultaneous effects of warming and toxicants, the consequences of thermal adaptation on subsequent exposure to pollutants remains unexplored.
Phytoplankton in the Red Sea, the warmest ocean in the world, showed rapid adaptation to ongoing warming, beyond the current thermal extremes, with tradeoffs in photosynthetic rates (Jin and Agustí, 2018). In the planktonic diatom Chaetoceros tenuissimus, growth was significantly promoted by the selection temperature throughout the selection period, resulting in higher maximum growth rate at its optimal temperature, however in a narrower thermal breadth in warming-adapted strains (Jin and Agustí, 2018). C. tenuissimus adopted a “hotter is better” strategy to adapt to warming, with maximal performance at a higher optimal temperature. However, the possible costs of physiological modification during adaptation to warming in a rapidly changing environment remain unclear. In this context, the goal of the present study is to elucidate how the adaptation of C. tenuissimus in the Red Sea to thermal changes interplays with its sensitivity to the toxic heavy metal, Cu. Although dealing these two drivers in different temporal contexts constitutes an oversimplification of reality, our approach hypothesizes phytoplankton thermally adapted for thousands of generations shall confront their metal tolerance levels, considering long term thermal selection as the dominant driver. Cu is an essential trace metal nutrient for phytoplankton, however, excess Cu inhibits their growth and photosystem II (PSII) photochemistry by hindering the water-splitting system, electron transport chain and chlorophyll biosynthesis (Lombardi and Maldonado, 2011). Due to the extensive use of Cu-based antifouling agents in aquaculture facilities, Cu is one of the most abundant metal pollutants in the coastal waters (Saad, 1996) and sediments (Badr et al., 2009) of the Red Sea. Phytoplankton represent a relevant functional group that sustains critical marine ecosystem functions. Therefore, insights on the response of heavy metal sensitivity of phytoplankton to experimental warming conditions and the likely tradeoff between these stressors would help us understand the complex interactions of future warming and pollution scenarios in the fragile ecosystems of the Red Sea.
Materials and Methods
Phytoplankton Cultures
Chaetoceros tenuissimus (Bacillariophyceae) was isolated from coastal surface water, at 2–3 m depth, near the Al Fahal Reef in the Red Sea (22.2528°N, 38.9612°E), after filtration through a 45 μm filter. Approximately ten clonal cultures were established using the precise single-cell isolation technique under the microscope (Axioscope A1, Carl Zeiss, Oberkochen, Germany). These isolates were maintained in batch cultures using filtered, sterilized and enriched (f/4 and silicate) seawater obtained from the same location. The cultures were incubated at 24°C in a temperature-controlled incubator (Percival Scientific Inc., USA) with a light:dark cycle of 12:12 h under an irradiance of 50 μmol photons m−2 s−1.
After the monospecific cultures of C. tenuissimus were established, experimental cultures were grown in 200 mL Erlenmeyer flasks at 26 ± 0.1°C (ambient-adaptation) and 30 ± 0.1°C (warming-adaptation) under 150 μmol photons m−2 s−1 with a light:dark cycle of 12:12 h. Independent cultures (n = 4) were replicated semi-continuously for about two years under both selection temperatures. The long-term nature of these experiments allowed the species to adapt to the selection temperatures over ~2,000 generations (Jin and Agustí, 2018). The initial cell concentration was set to 1000 cells mL−1, and the medium was partially renewed every three days to restore the cell density to the initial values measured at the beginning of the growth batch cycle. Nutrients in the medium were not limiting as the cell abundances achieved at the end of the batch cycles were lower than those expected at the stationary phase. The cell abundance was quantified concurrently every three days by examining the samples under an optical microscope (Leica DMI 3000B-Germany) with a hemocytometer.
Cu Toxicity Assays
After two years of allowing the C. tenuissimus strains to adapt to the ambient and warming temperatures, we tested their differential responses to Cu toxicity. Acute toxicity of Cu to the ambient- and warming-adapted strains of C. tenuissimus was determined by 48 h static, non-renewal assays at three different temperatures; ambient (26°C), warming (30°C), and extreme warming (35°C). All the glassware was soaked in 10% HCl for 2 h before use, to remove any trace metals, followed by repeated rinsing with Milli-Q water and oven drying. All tests were conducted in f/2-enriched filtered seawater medium free of EDTA (ethylenediaminetetraacetic acid). The media were equilibrated in the incubator chamber for one day under the same conditions as the toxicity tests. A Cu stock solution at 1000 μg L−1 was prepared from CuSO4.5H2O and refrigerated until use. From the stock solution, 50 mL of the Cu treatments (0.25, 0.5, 1, 5, 10, and 25 μg L−1) and the controls (no Cu added) were prepared in triplicate 125 mL Erlenmeyer flasks. Then, the algae were inoculated with cultures in log phase to obtain an approximate initial abundance of 5 × 104 cells mL−1. All the replicates in the experiment were originated from the same clonal ancestor culture. The test flasks were incubated under similar conditions of irradiance and photoperiod as the adaptation period. The experimental flasks were manually shaken at least three times per day.
Flow Cytometry Analysis
After metal exposure, the cell abundances were directly estimated using a high performance, true volumetric absolute counting (TVAC), bench-top flow cytometer equipped with a 488-nm blue solid-state laser (CyFlow Space, Sysmex Partec GmbH, Germany). Data were acquired by triggering the chlorophyll autofluorescence (FL3, 675 nm) in log mode, and the C. tenuissimus population was enumerated following the standard protocols (Franklin et al., 2001). The population was identified based on the characteristic signals in a cytogram of FL3 versus side scatter (SSC). Non-algal particles and dead cells were excluded from the analysis by gating on the SSC/FL3 dot plot. The algal abundance was expressed as cells mL−1.
PhytoPAM Fluorescence Measurement
The chlorophyll fluorescence parameters were measured using a noninvasive pulse-amplitude modulated fluorometer (PhytoPAM II, Heinz Walz GmbH, Effeltrich, Germany). The PhytoPAM II measures fluorescence at five excitation wavelengths (440, 480, 540, 590, and 625 nm), and the algal group values were deconvoluted from these signals using linear unmixing based on the selected reference spectra. Samples were adapted to the dark for 15 min before measurement of the dark fluorescence, F0. The maximum PSII efficiency of the population, which indicates the potential photochemical efficiency of PSII and is related to the fraction of absorbed energy that is transferred to photosynthesis by the PSII reaction centers, was estimated as Fv/Fm, where Fv is the variable fluorescence (Fm – F0).
Statistical Analysis
The specific growth rate (μ, d−1) after the various treatments was calculated using the following formula:
where N1 and N2 are the cell numbers at time t1 (initial, 0 h) and t2 (final, 48 h), respectively (Levasseur et al., 1993).
The effective concentrations at which a 50% decline in population abundance occurred (EC50) were estimated using the JMP software (JMP® Pro version 13.1, SAS Institute, USA) and the following equation, described previously (Kottuparambil and Agusti, 2018):
where Ω is the slope of the relationship between the ln of the cell abundance versus the Cu concentrations tested at the end of the experiment.
The EC50 values for growth rate and Fv/Fm and their respective confidence intervals (95%) were calculated using a log-normal model in the Excel macro REGTOX (Vindimian, 2012). A mixed-effects modeling analysis of covariance was used to quantify the variance in all measured variables at the replicate levels within two selection temperatures, where biological replicates within treatments were the random effect, and selection temperature, assay temperature, and Cu doses were the fixed effects, using the JMP software. Tukey's post-hoc multiple comparison test was conducted for the comparison of means across treatments. The significance of the differences observed among the treatments was analyzed using a Student's t-test or one-way analysis of variance (ANOVA).
Literature Survey
A survey of the literature from the last decade was conducted regarding the influence of temperature on Cu toxicity in marine phytoplankton. A summary of the relevant data from previous studies and a comparison with the toxicity values obtained in the present study are provided in Table 1.
Results
Cu Toxicity to Chaetoceros tenuissimus
Our results showed a significant acute toxic effect of Cu on various criteria of C. tenuissimus at ambient and elevated temperatures, over an acclimation time scale of a few generations. 48 h Cu EC50 values for various test criteria under different experiments are summarized in Table 2. The 48 h abundance was assumed to be the critical criterion for gauging the toxic impact of Cu. Cell density (mL−1) of the ambient-adapted strain was not significantly affected at Cu concentrations up to 0.5 μg L−1 in 26°C tests (ANOVA, df = 2, p > 0.05), and up to 1 μg L−1 (ANOVA, df = 3, p > 0.05) when tested at 30°C (Figure 1). However, in the case of the warming-adapted strain, significant reductions in abundance was recorded above 0.5 μg L−1 and 0.25 μg L−1 Cu under ambient (26°C) and warming (30°C) test conditions, respectively (ANOVA, df = 6, p < 0.05).
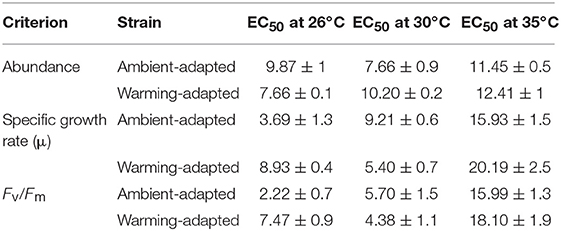
Table 2. 48 h Cu EC50 values (± SD) of ambient-adapted and warming-adapted Chaetoceros tenuissimus assayed at 26, 30, and 35°C.
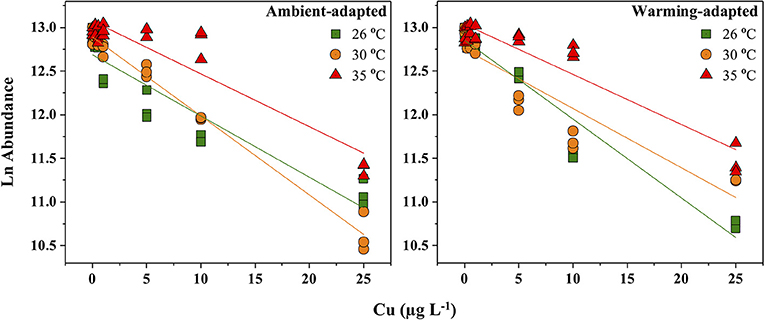
Figure 1. Response of Ln cell abundance of ambient-adapted and warming-adapted Chaetoceros tenuissimus to Cu exposure at ambient (26°C; green), warming (30°C; orange), and extreme warming (35°C; red) temperatures. Each individual shapes denotes individual replicates and the lines indicate linear regression.
Our literature survey revealed that reduction in cellular growth rate provides sensitive estimations of Cu toxicity in marine phytoplankton (Table 1). Cu caused significant dose-dependent effects on the specific growth rate of C. tenuissimus, the magnitude of which varied between various experiments (Figure 2; Table 2). The growth rate was more sensitive to Cu than cell abundance for the ambient-adapted strain at 26°C and the warming-adapted strain at 30°C (Table 2). The EC50 value for the growth rate of the ambient-adapted strain was significantly higher (t-test, df = 3.86, p < 0.05) at 30°C than that at 26°C (9.21 μg L−1and 3.69 μg L−1, respectively, Table 2). Similarly, a significantly higher EC50 value was obtained for the warming-adapted strain tested at 26°C than that at 30oC (8.93 μg L−1and 5.40 μg L−1, respectively; t-test, df = 3.37, p < 0.05). These values revealed an antagonistic effect of the abrupt shift in temperature on Cu toxicity to the cellular growth rate.
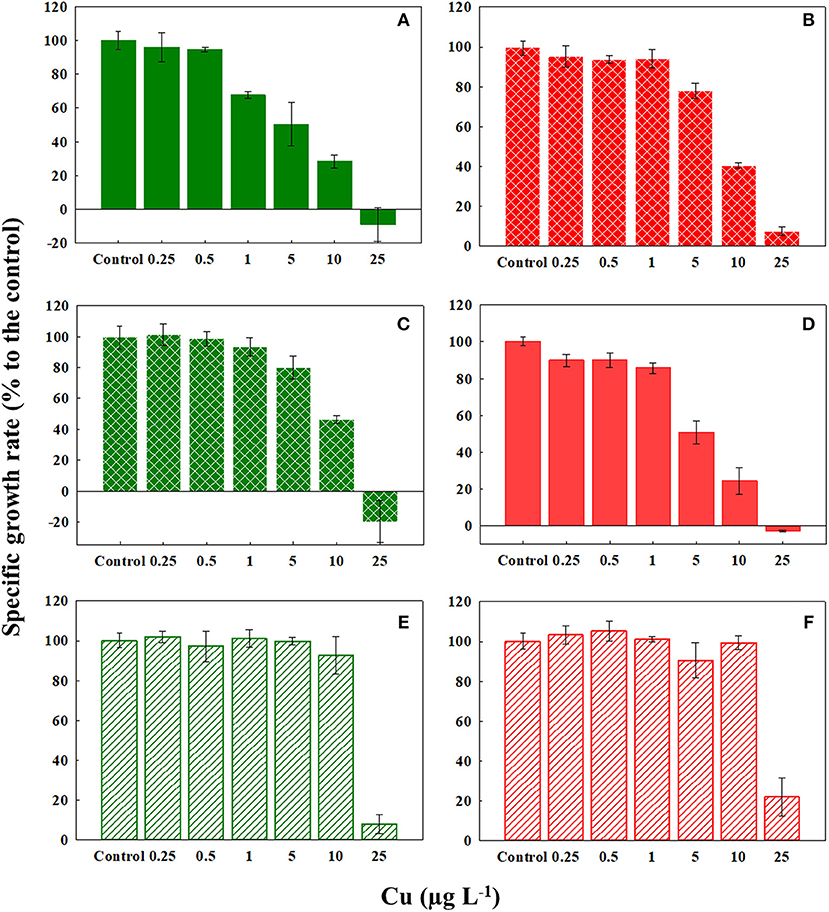
Figure 2. Response of the specific growth rate (μ) of ambient-adapted (green) and warming-adapted (red) strains of Chaetoceros tenuissimus to Cu exposure at various temperatures: (A) ambient-adapted tested at 26°C, (B) warming-adapted tested at 26°C, (C) ambient-adapted tested at 30°C, (D) warming-adapted tested at 30°C, (E) ambient-adapted tested at 35°C, and (F) warming-adapted tested at 35°C. Solid fills: Cu test at selection temperatures, textured fills: Cu test at reciprocal transplants and line fills: Cu test at 35°C. Data are plotted as treatment means with error bars ± SD.
We observed a drastic effect on the photosynthetic efficiency of PSII, measured as Fv/Fm, of C. tenuissimus upon Cu exposure (Figure 3). When tested in selection temperatures, the average EC50 value for Fv/Fm was significantly lower (t-test, df = 6.32, p < 0.05) than that of abundance, scoring 4.94 ± 1.1 μg L−1 and 8.85 ± 0.6 μg L−1 Cu (mean ± SD), respectively. Fv/Fm indicates the potential photochemical efficiency of PSII and related to the fraction of absorbed energy transferred to photosynthesis by the PSII reaction centers. Fv/Fm was more Cu sensitive than cellular growth at the ambient temperature, 26°C (Figures 2, 3). Moreover, when tested at 26°C, Fv/Fm in the ambient-adapted strain was drastically reduced with respect to the controls (Figure 3A), with an EC50 value of 2.22 μg L−1. Contrarily, for the warming-adapted strain tested at 26°C, no significant difference (ANOVA, df = 3, p > 0.05) was observed up to 1 μg L−1 of Cu (Figure 3B), with the higher EC50 value of 7.47 μg L−1. Whereas at 35°C, no significant effect on Fv/Fm was observed except at the highest Cu concentration tested, for both the strains (Figures 3E,F). These disparities in the Fv/Fm response reveal that adaptation of the photosynthetic apparatus to warming does influence the sensitivity of phytoplankton photosynthesis to subsequent Cu exposure.
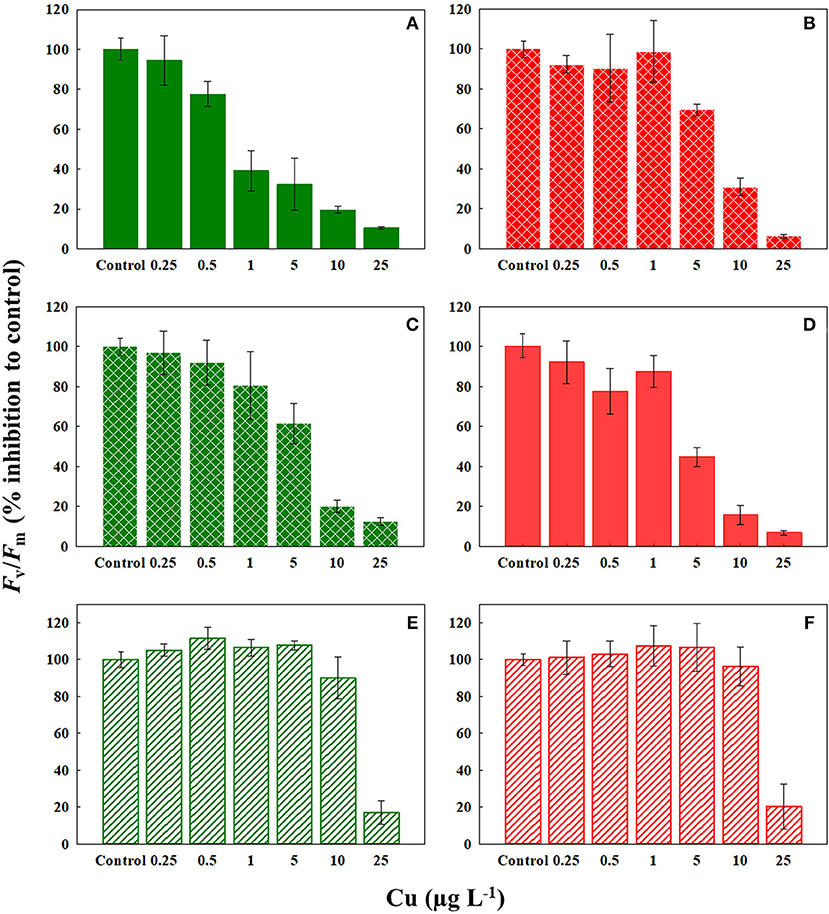
Figure 3. Response of Fv/Fm of ambient-adapted (green) and warming-adapted (red) strains of Chaetoceros tenuissimus to Cu exposure at various temperatures: (A) ambient-adapted tested at 26°C, (B) warming-adapted tested at 26°C, (C) ambient-adapted tested at 30°C, (D) warming-adapted tested at 30°C, (E) ambient-adapted tested at 35°C, and (F) warming-adapted tested at 35°C. Solid fills: Cu test at selection temperatures, textured fills: Cu test at reciprocal transplants and line fills: Cu test at 35°C. Data are plotted as treatment means with error bars ± SD.
Interaction Between Selection Temperature, Assay Temperature, and Cu Toxicity
We hypothesized that adaptation to selection temperatures and variation in assay temperature interact with the organism's response to subsequent Cu exposure, particularly in the warming-adapted C. tenuissimus. A mixed-effects model analysis revealed significant interactive effects of Cu dose, assay temperature, and selection temperature on parameter estimates of the growth rate, cell abundance, and Fv/Fm of the model diatom (Table 3). The results also showed a strong influence of assay temperature on the overall interaction among the variables, which was antagonistic to the Cu toxicity response. Mixed effects model analysis revealed that adaptation to selection temperatures helped the strains to maintain their response to Cu while strong interactions between assay temperature, adaptation, and Cu doses modified the toxicity responses in all tested criteria.
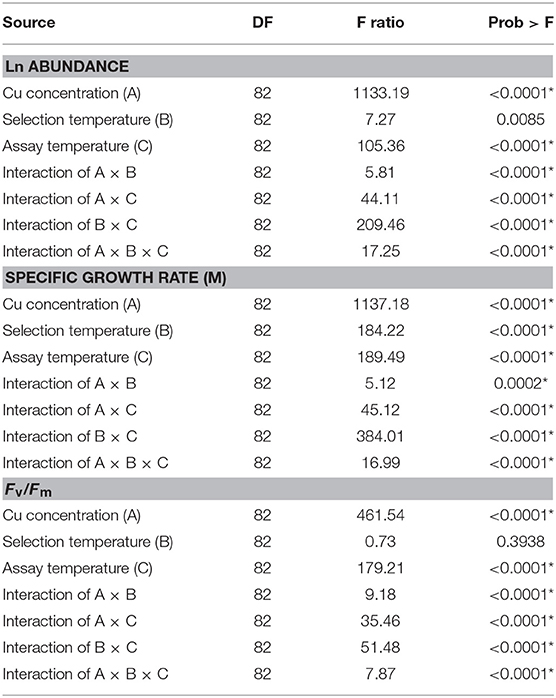
Table 3. Statistical results of linear mixed-effects modeling analysis of covariance with Ln abundance, specific growth rate (μ), and Fv/Fm of Chaetoceros tenuissimus as dependent variables, selection temperatures (26 and 30°C), Cu concentrations, and assay temperatures (26, 30, and 35°C) as fixed effects, and the biological replicates within treatments as a random effect on intercepts (DF: Degrees of freedom, F ratio: the model mean square divided by the error mean square, Prob > F: The p-value for the test).
Influence of Thermal Adaptation on Cu Sensitivity
We further examined how adaptation to warming influenced the metal sensitivity of C. tenuissimus under three assay temperatures. The lethal 48 h EC50 values (for decay in abundance) were statistically similar (t-test, df = 2.15, p > 0.05) between the ambient-adapted strain tested at 26°C (9.87 μg L−1) and the warming-adapted strain tested at 30°C (10.20 μg L−1). Similarly, a lower value of 7.66 μg L−1 was obtained from both the ambient-adapted and warming-adapted strains tested at 30 and 26°C, respectively. At the extreme warming assay condition, however, there was no significantly different Cu sensitivity between the two strains (t-test, df = 2.79, p > 0.05, Table 2).
The EC50 values for reduced growth rates demonstrated a similar influence of temperature adaptation. The EC50 values for the ambient-adapted strain tested at 26°C and the warming-adapted strain tested at 30°C were not significantly different (t-test, df = 2.91, p > 0.05, Table 2). Likewise, the EC50 values for the ambient-adapted strain in the 30°C assay and the warming-adapted strain in the 26°C assay were statistically similar (t-test, df = 2.55, p > 0.05). However, a significant difference was noted in the EC50 values for the warming-adapted strain tested at 26 and 30°C (t-test, df = 3.37, p < 0.05). These different EC50s at two test temperatures indicated that previous adaptation to a warming environment alleviated or nullified the impact of abrupt shifts in temperature on Cu toxicity. However, at 35°C, a significant decrease in Cu sensitivity was observed in the warming-adapted strains (t-test, df = 3.27, p < 0.05, Table 2).
The contrasting effects of adaptation to ambient or warming temperatures were reflected by the photosynthetic criterion, Fv/Fm, in the tested diatom. The ambient-adapted strain responded to warming with a significant drop in the sensitivity of Fv/Fm to Cu (Table 2), with the EC50 value becoming statistically similar to that of the warming-adapted strain tested at 30°C. In the 26°C exposure, the adaptation to warming reduced the toxicity of Cu. Similarly, when tested at 30°C, adaptation to ambient temperature resulted in slightly reduced Cu toxicity. Moreover, EC50 values for Fv/Fm showed that crossing temperatures during the tests caused significant reductions in Cu sensitivity of both strains (Table 2). A similar trend was observed in the 35°C assay, where the EC50 values were 15.99 μg L−1 and 18.10 μg L−1, for ambient- and warming-adapted strains, respectively. The Tukey's test for comparison of the means clearly showed the significantly higher EC50 values at 35°C, indicating an antagonistic effect of elevated temperature.
Discussion
Environmental factors such as increased temperature can interact with persistent pollutants like heavy metals, resulting in increased mortality and reduced reproduction of aquatic organisms (Gama-Flores et al., 2005). Although rising temperatures can significantly affect the metabolism of marine phytoplankton (Toseland et al., 2013), their sensitivity to toxicants is exacerbated, primarily due to increased rates of uptake and accumulation (Wang and Wang, 2008; Yung et al., 2017). The model diatom used in this study showed rapid adaption to warming, over hundreds of generations, although tradeoff on photosynthetic performance could alter their competitive fitness to other environmental drivers such as high irradiation (Jin and Agustí, 2018). As a consequence of tradeoffs, the “hotter is better” strategy was achieved by narrowing the thermal breadth of growth in Chaetoceros tenuissimus (Jin and Agustí, 2018). More ecological consequences of thermal adaptation have been recently addressed in phytoplankton communities (Yvon-Durocher et al., 2017). However, our results achieved with two strains of the diatom C. tenuissimus, adapted to either the ambient temperature of the Red Sea or an experimental warming temperature, indicate that selective adaptation to warming could help mitigate the expected synergistic interaction between warming and Cu toxicity.
Most of the reported Cu toxicity studies on microalgae in the last decade were conducted in the laboratory within a narrow temperature range of 20–25°C over an acclimation timescale of a few generations (Table 1). Several recent studies have addressed the interactive effect of rising temperatures and heavy metal toxicity in marine phytoplankton. For instance, elevated temperatures caused increased Cu toxicity and reduced community tolerance in periphytic diatoms in an acclimation timescale of 6 weeks (Morin et al., 2017), while significantly higher Cu toxicity for the green microalgae Raphidocelis subcapitata resulted at temperatures higher than the ambient levels (Silva et al., 2018). High temperatures enhanced the accumulation of metals by diatoms (Yung et al., 2017), either by the increased cellular intake of metal ions or by their weakened detoxification ability at higher temperatures (Wang and Wang, 2008). Moreover, disruption of the normal physiological functions, such as photosynthetic performance, results in higher susceptibility to toxic metals (Yung et al., 2017).
The response of abundance (Figure 1) shows that adaptation of C. tenuissimus to warming, prior to Cu exposure, caused a decrease in the lower toxicity threshold of Cu. However, we found an increased tolerance at higher temperature, but no significant difference between the toxicity thresholds required to decrease the abundance of ambient- and warming-adapted strains at their selection temperatures. The EC50 value at 30°C for the warming-adapted strain was statistically identical to that for the ambient-adapted strain at 26°C (Table 2). Nevertheless, the lowest observed effect concentration (LOEC) values recorded from the warming-adapted strain were ecologically significant, since these values are close to the current marine water quality guideline for Cu, i.e., 1.3 μg L−1 (USEPA, 2016). Moreover, Table 1 clarifies that the Red Sea species C. tenuissimus tested here was more sensitive to Cu than most phytoplankton reported previously. This disparity in interspecific sensitivity can be influenced by the habitat, the physiological status of the organism, and the background Cu concentrations (Debelius et al., 2010). In the Red Sea, the mean Cu concentrations were as high as 5.23 ± 0.98 μg L−1 in the northern region (Ali et al., 2011), 4.71 ± 0.87 μg L−1 along the Egyptian coast (Abouhend and El-Moselhy, 2015) and 1.69 μg L−1 at an industrial area along the Saudi Arabian coast (Saad, 1996). These heavy metal levels in the Red Sea are already above the critical threshold values for the optimal growth of some phytoplankton species and more aggravated impacts are expected in the warmer future.
Our results indicate that thermally adapted C. tenuissimus strains were more resistant to Cu at selection temperatures to which they were previously adapted, and the Cu sensitivity increased slightly but significantly when tested as reciprocal transplants during the Cu tests. As shown by the respective EC50 values (Table 2), crossing the temperatures during Cu exposure resulted in a significant increase in Cu sensitivity of both the ambient- (t-test, df = 3.96, p < 0.05) and warming-adapted (t-test, df = 3.36, p < 0.05) strains of C. tenuissimus. In general, the interactive effect of elevated temperature and metal toxicity largely depends on the organism's ability to acclimate to higher temperatures (Pereira et al., 2017). Therefore, the inherent capacity of the tested diatom to adapt to warming must have resulted in the higher EC50 values obtained at 35°C. Our results indicated an antagonistic effect of warming, and the joint effect of Cu exposure and increasing temperature was statistically significant. The observed Cu toxicity profile at elevated temperature is more likely justified by the effect of temperature on Cu speciation rather than by an effect of temperature on the biological interaction of free Cu2+ ions at the cellular boundaries of the phytoplankton.
While earlier experiments clearly demonstrated the successful adaptation of C. tenuissimus to experimental warming conditions after 500 generations (Jin and Agustí, 2018), adaptation to a warmer temperature increases the energetic cost of optimizing metabolic machinery of the cells adapted to a particular temperature, such as photosynthesis, that could help the organism achieve increased carbon use efficiency (Padfield et al., 2016) or greater photosynthetic capacity and reduced susceptibility to photoinhibition (Schaum et al., 2017). In C. tenuissimus, adaptation to warming resulted in higher growth rates and widening of the critical thermal limits with associated costs, since adaptations of enzymes and membranes to high temperatures may result in maladaptations to low temperatures (Jin and Agustí, 2018). The cellular metabolism imposes constraints that are usually reflected in the growth, photosynthetic yield and light utilization efficiencies. However, a complete calculation of the absolute cost of temperature adaptation must account for the multiple processes of the organismal existence (Clarke, 2003). In this context, our hypothesis prompts an important question about the significance of thermal adaptation at the cellular level to processes at the population level, such as sensitivity to pollutants. Our results (Table 3), showing significant antagonistic interaction between Cu doses, assay temperature (26, 30, or 35°C), and selection temperature (26 or 30°C) suggest that adaptation to environmental temperatures could help maintain a similar metal tolerance. However, a change in the assay temperature (e.g., we tested a 4°C increase or decrease from selection temperature) may significantly increase the Cu sensitivity at lower temperatures. Low levels of ambient Cu can accelerate the growth of marine phytoplankton (Wang et al., 2017). Temperature, within the thermal limits of individual organisms, also has strong stimulating effects on the cellular growth and physiological fitness of phytoplankton (Jutterström et al., 2014; Yvon-Durocher et al., 2015). However, the interaction between Cu and rising temperature in natural phytoplankton communities is a complex phenomenon and its full-scale impacts are hard to predict. Our laboratory experiments provided significant evidence for a pronounced interaction between warmer temperatures and Cu in the Red Sea model diatom (Table 3). The distribution of EC50 values for tested criteria (Figure 4) showed negligible variations in Cu toxicity between ambient adapted samples tested at 26°C and warming adapted samples tested at 30°C. However, adaptation to warming did cause an improved Cu tolerance, particularly at a higher temperature, which would have wide-ranging impacts on the ecological performance of phytoplankton in the warming Red Sea. Climate change in the Red Sea is intense (Chaidez et al., 2017), and even more extreme thermal events that may modify physiological robustness of phytoplankton against pollutants are likely to occur in the future.
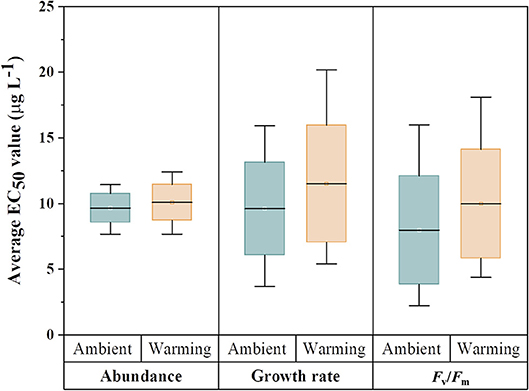
Figure 4. Comparison of the distribution of EC50 values for various criteria tested across three temperatures for ambient-adapted and warming-adapted Chaetoceros tenuissimus. Tops and bottoms of box plots represent the 75th and 25th percentiles, respectively, and black horizontal lines the medians.
Global climate change phenomena, such as ocean warming and acidification, will alter the toxicity of waterborne metals by influencing their speciation in seawater (Pascal et al., 2010). The behavior of heavy metals in seawater is complex and determined by several parameters, such as pH and the concentrations of organic matter, minerals and redox potentials. To predict the joint effect of these critical factors on marine systems at the individual, population and community levels is a great challenge for ecotoxicologists. Apparently, the response of phytoplankton to warming and heavy metal toxicity would be influenced by the pollution-induced tolerance of the community, in line with the capacity of phytoplankton to adapt to warming. In general, stress due to warming may reduce the organismal potential for tolerance and recovery after toxicant exposure (Moe et al., 2013), however, our data shows that adaptation of phytoplankton to warming in the Red Sea could induce a certain degree of tolerance to subsequent heavy metal exposure. This conclusion is based on acute Cu exposure, and further studies are recommended to reveal the impact of long-term exposure. Although sensitive toxicity threshold values were obtained, our approach of laboratory tests based on a single species do not represent real environmental conditions. Moreover, considering the fact that warming may alter the composition of phytoplankton communities (Thomas et al., 2012), the response of the whole community to metal pollution could be altered in a future scenario. We emphasize the need to consider these multiple factors in risk assessments of heavy metal pollution in warming marine ecosystems.
Conclusion
Increased concentrations of Cu adversely affected the growth and photosynthesis of the marine phytoplankton tested here. Our model organism, C. tenuissimus, is capable of rapid adaptation to experimental warming, up to 4°C increase in its ambient temperature, with clear benefits on growth rates (Jin and Agustí, 2018). Temperature is a pivotal factor in toxicological models for phytoplankton since variations in temperature cause significant changes in their physiological and biochemical processes. The present study confirms the positive interaction between Cu toxicity and warming, highlighting the significance of combined anthropogenic stressors on oceanic phytoplankton. Our data confirm that the thermal adaptation of phytoplankton to the warm tropical Red Sea increases the species tolerance to subsequent toxic metal exposure. Adaptation to selection temperatures (ambient and warming) helped maintain their Cu tolerances, although reciprocal transplant of warming-adapted cells resulted in an increase in sensitivity at the lower test temperature. A change in the biosynthesis of cellular metabolites in C. tenuissimus as a result of a shift in the culture temperature (Jin and Agusti, in prep.) is likely to weaken the metal complexation and detoxification mechanisms under different temperature treatments. The population did suffer in terms of photosynthetic carbon fixation, implying a tradeoff between the metabolic cost of Cu detoxification and photosynthetic energy fixation. The enhanced Cu tolerance at a warmer temperature, corresponding to the summer sea surface temperature (SST) of the Red Sea, also highlights the likely fluctuation in the toxicity of persistent contaminants. In addition, our results raise concerns on the impact of Cu contamination of pristine Red Sea habitats. The sensitivity of Red Sea model diatom to Cu was relatively higher than other species reported in previous studies (Table 1). Overall, our study emphasizes that the complex interactions among pollutants and vital abiotic factors should be a priority for further research to fully comprehend the influence of future climate conditions on the biota of the Red Sea.
Data Availability
The raw data supporting the conclusions of this manuscript will be made available by the authors, without undue reservation, to any qualified researcher.
Author Contributions
SK, PJ, and SA conceived and designed the experiments. PJ provided the experimental cultures. SK and SA analyzed and interpreted the data. SK performed the experiments and wrote the paper. All the authors contributed to the revisions.
Conflict of Interest Statement
The authors declare that the research was conducted in the absence of any commercial or financial relationships that could be construed as a potential conflict of interest.
Acknowledgments
The research reported in this publication was supported by funding from King Abdullah University of Science and Technology (KAUST), under award numbers BAS/1/1072-01-01 and BAS/1/1072-01-03 to SA. We thank Juan D. Martinez Ayala and Dr. Fran Aparicio at the Red Sea Research Center, KAUST, for their assistance in isolation and culture of the strains used in this study.
References
Abouhend, A. S., and El-Moselhy, K. M. (2015). Spatial and seasonal variations of heavy metals in water and sediments at the northern Red Sea coast. Am. J. Water Resour. 3, 73–85. doi: 10.12691/ajwr-3-3-2
Ali, A.-H. A. M., Hamed, M. A., and El-Azim, H.A. (2011). Heavy metals distribution in the coral reef ecosystems of the Northern Red Sea. Helgol. Mar. Res. 65, 67–80. doi: 10.1007/s10152-010-0202-7
Angel, B. M., Simpson, S. L., Chariton, A. A., Stauber, J. L., and Jolley, D. F. (2015). Time-averaged copper concentrations from continuous exposures predicts pulsed exposure toxicity to the marine diatom, Phaeodactylum tricornutum: importance of uptake and elimination. Aquat. Toxicol. 164, 1–9. doi: 10.1016/j.aquatox.2015.04.008
Anu, P., Nandan, S. B., Jayachandran, P., and Xavier, N. D. (2016). Toxicity effects of copper on the marine diatom, Chaetoceros calcitrans. Reg. Stud. Mar. Sci. 8, 498–504. doi: 10.1016/j.rsma.2016.07.001
Badr, N. B., El-Fiky, A. A., Mostafa, A. R., and Al-Mur, B. A. (2009). Metal pollution records in core sediments of some Red Sea coastal areas, Kingdom of Saudi Arabia. Environ. Monit. Assess. 155, 509–526. doi: 10.1007/s10661-008-0452-x
Bao, V. W., Leung, K. M., Kwok, K. W., Zhang, A. Q., and Lui, G. C. (2008). Synergistic toxic effects of zinc pyrithione and copper to three marine species: implications on setting appropriate water quality criteria. Mar. Pollut. Bull. 57, 616–623. doi: 10.1016/j.marpolbul.2008.03.041
Bielmyer-Fraser, G. K., Jarvis, T. A., Lenihan, H. S., and Miller, R. J. (2014). Cellular partitioning of nanoparticulate versus dissolved metals in marine phytoplankton. Environ. Sci. Technol. 48, 13443–13450. doi: 10.1021/es501187g
Cantin, N. E., Cohen, A. L., Karnauskas, K. B., Tarrant, A. M., and McCorkle, D. C. (2010). Ocean warming slows coral growth in the central Red Sea. Science 329, 322–325. doi: 10.1126/science.1190182
Chaidez, V., Dreano, D., Agusti, S., Duarte, C. M., and Hoteit, I. (2017). Decadal trends in Red Sea maximum surface temperature. Sci. Rep. 7:8144. doi: 10.1038/s41598-017-08146-z
Clarke, A. (2003). Costs and consequences of evolutionary temperature adaptation. Trends Ecol. Evol. 18, 573–581. doi: 10.1016/j.tree.2003.08.007
Collins, S. (2011). Many possible worlds: expanding the ecological scenarios in experimental evolution. Evol. Biol. 38, 3–14. doi: 10.1007/s11692-010-9106-3
Couet, D., Pringault, O., Bancon-Montigny, C., Briant, N., Poulichet, F. E., Delpoux, S., et al. (2018). Effects of copper and butyltin compounds on the growth, photosynthetic activity and toxin production of two HAB dinoflagellates: the planktonic Alexandrium catenella and the benthic Ostreopsis cf. ovata. Aquat. Toxicol. 196, 154–167. doi: 10.1016/j.aquatox.2018.01.005
Debelius, B., Forja, J. M., DelValls, Á., and Lubián, L. M. (2009). Toxicity and bioaccumulation of copper and lead in five marine microalgae. Ecotoxicol. Environ. Saf. 72, 1503–1513. doi: 10.1016/j.ecoenv.2009.04.006
Debelius, B., Forja, J. M., DelValls, Á., and Lubián, L. M. (2010). Toxic effect of copper on marine picophytoplankton populations isolated from different geographic locations. Sci. Mar. 74, 133–141. doi: 10.3989/scimar.2010.74s1133
Deheyn, D. D., and Latz, M. I. (2006). Bioavailability of metals along a contamination gradient in San Diego Bay (California, USA). Chemosphere 63, 818–834. doi: 10.1016/j.chemosphere.2005.07.066
Duarte, B., Cabrita, M. T., Vidal, T., Pereira, J. L., Pacheco, M., Pereira, P., et al. (2018). Phytoplankton community-level bio-optical assessment in a naturally mercury contaminated Antarctic ecosystem (Deception Island). Mar. Environ. Res. 140, 412–421. doi: 10.1016/j.marenvres.2018.07.014
Dupraz, V., Stachowski-Haberkorn, S., Mènard, D., Limon, G., Akcha, F., Budzinski, H., et al. (2018). Combined effects of antifouling biocides on the growth of three marine microalgal species. Chemosphere 209, 801–814. doi: 10.1016/j.chemosphere.2018.06.139
Franklin, N. M., Stauber, J. L., and Lim, R. P. (2001). Development of flow cytometry-based algal bioassays for assessing toxicity of copper in natural waters. Environ. Toxicol. Chem. 20, 160–170. doi: 10.1002/etc.5620200118
Gama-Flores, J. L., Sarma, S., and Nandini, S. (2005). Interaction among copper toxicity, temperature and salinity on the population dynamics of Brachionus rotundiformis (Rotifera). Hydrobiologia 546, 559–568. doi: 10.1007/s10750-005-4300-5
Gittings, J. A., Raitsos, D. E., Krokos, G., and Hoteit, I. (2018). Impacts of warming on phytoplankton abundance and phenology in a typical tropical marine ecosystem. Sci. Rep. 8:2240. doi: 10.1038/s41598-018-20560-5
Guo, R., Ebenezer, V., and Ki, J.-S. (2012). Transcriptional responses of heat shock protein 70 (Hsp70) to thermal, bisphenol A, and copper stresses in the dinoflagellate Prorocentrum minimum. Chemosphere 89, 512–520. doi: 10.1016/j.chemosphere.2012.05.014
Guo, R., Lee, M.-A., and Ki, J.-S. (2013). Different transcriptional responses of heat shock protein 70/90 in the marine diatom Ditylum brightwellii exposed to metal compounds and endocrine-disrupting chemicals. Chemosphere 92, 535–543. doi: 10.1016/j.chemosphere.2013.03.052
Hoffmann, L. J., Breitbarth, E., Boyd, P. W., and Hunter, K. A. (2012). Influence of ocean warming and acidification on trace metal biogeochemistry. Mar. Ecol. Prog. Ser. 470, 191–205. doi: 10.3354/meps10082
Huertas, I. E., Rouco, M., López-Rodas, V., and Costas, E. (2010). Estimating the capability of different phytoplankton groups to adapt to contamination: herbicides will affect phytoplankton species differently. New Phytol. 188, 478–487. doi: 10.1111/j.1469-8137.2010.03370.x
Huertas, I. E., Rouco, M., López-Rodas, V., and Costas, E. (2011). Warming will affect phytoplankton differently: evidence through a mechanistic approach. Proc. R. Soc. B 278, 3534–3543. doi: 10.1098/rspb.2011.0160
Irwin, A. J., Finkel, Z. V., Müller-Karger, F. E., and Ghinaglia, L. T. (2015). Phytoplankton adapt to changing ocean environments. Proc. Natl. Acad. Sci. U.S.A. 112, 5762–5766. doi: 10.1073/pnas.1414752112
Jin, P., and Agustí, S. (2018). Fast adaptation of tropical diatoms to increased warming with trade-offs. Sci. Rep. 8:17771. doi: 10.1038/s41598-018-36091-y
Johnson, H. L., Stauber, J. L., Adams, M. S., and Jolley, D. F. (2007). Copper and zinc tolerance of two tropical microalgae after copper acclimation. Environ. Toxicol. 22, 234–244. doi: 10.1002/tox.20265
Jutterström, S., Andersson, H., Omstedt, A., and Malmaeus, J. (2014). Multiple stressors threatening the future of the Baltic Sea–Kattegat marine ecosystem: implications for policy and management actions. Mar. Pollut. Bull. 86, 468–480. doi: 10.1016/j.marpolbul.2014.06.027
Koppel, D., Adams, M. S., King, C. K., and Jolley, D. F. (2018). Chronic toxicity of an environmentally relevant and equitoxic ratio of five metals to two Antarctic marine microalgae shows complex mixture interactivity. Environ. Pollut. 242(Pt. B), 1319–1330. doi: 10.1016/j.envpol.2018.07.110
Koppel, D., Gissi, F., Adams, M. S., King, C. K., and Jolley, D. F. (2017). Chronic toxicity of five metals to the polar marine microalga Cryothecomonas armigera – Application of a new bioassay. Environ. Pollut. 228, 211–221. doi: 10.1016/j.envpol.2017.05.034
Kottuparambil, S., and Agusti, S. (2018). PAHs sensitivity of picophytoplankton populations in the Red Sea. Environ. Pollut. 239, 607–616. doi: 10.1016/j.envpol.2018.04.079
Lelong, A., Jolley, D. F., Soudant, P., and Hégaret, H. (2012). Impact of copper exposure on Pseudo-nit-zschia spp. physiology and domoic acid production. Aquat. Toxicol. 118, 37–47. doi: 10.1016/j.aquatox.2012.03.010
Leung, P. T., Yi, A. X., Ip, J. C., Mak, S. S., and Leung, K. M. (2017). Photosynthetic and transcriptional responses of the marine diatom Thalassiosira pseudonana to the combined effect of temperature stress and copper exposure. Mar. Pollut. Bull. 124, 938–945. doi: 10.1016/j.marpolbul.2017.03.038
Levasseur, M., Thompson, P. A., and Harrison, P. J. (1993). Physiological acclimation of marine-phytoplankton to different nitrogen-sources. J. Phycol. 29, 587–595. doi: 10.1111/j.0022-3646.1993.00587.x
Levy, J. L., Stauber, J. L., and Jolley, D. F. (2007). Sensitivity of marine microalgae to copper: the effect of biotic factors on copper adsorption and toxicity. Sci. Total Environ. 387, 141–154. doi: 10.1016/j.scitotenv.2007.07.016
Levy, J. L., Stauber, J. L., Wakelin, S. A., and Jolley, D. F. (2009). The effect of bacteria on the sensitivity of microalgae to copper in laboratory bioassays. Chemosphere 74, 1266–1274. doi: 10.1016/j.chemosphere.2008.10.049
Listmann, L., LeRoch, M., Schlüter, L., Thomas, M. K., and Reusch, T. B. (2016). Swift thermal reaction norm evolution in a key marine phytoplankton species. Evol. Appl. 9, 1156–1164. doi: 10.1111/eva.12362
Liu, G., Chai, X., Shao, Y., Hu, L., Xie, Q., and Wu, H. (2011). Toxicity of copper, lead, and cadmium on the motility of two marine microalgae Isochrysis galbana and Tetraselmis chui. J. Environ. Sci. 23, 330–335. doi: 10.1016/S1001-0742(10)60410-X
Lombardi, A. T., and Maldonado, M. T. (2011). The effects of copper on the photosynthetic response of Phaeocystis cordata. Photosyn. Res. 108, 77–87. doi: 10.1007/s11120-011-9655-z
Manciocco, A., Calamandrei, G., and Alleva, E. (2014). Global warming and environmental contaminants in aquatic organisms: the need of the etho-toxicology approach. Chemosphere 100, 1–7. doi: 10.1016/j.chemosphere.2013.12.072
Manimaran, K., Karthikeyan, P., Ashokkumar, S., Prabu, V. A., and Sampathkumar, P. (2012). Effect of copper on growth and enzyme activities of marine diatom, Odontella mobiliensis. Bull. Environ. Contam. Toxicol. 88, 30–37. doi: 10.1007/s00128-011-0427-4
Marvá, F., García-Balboa, C., Baselga-Cervera, B., and Costas, E. (2014). Rapid adaptation of some phytoplankton species to osmium as a result of spontaneous mutations. Ecotoxicology 23, 213–220. doi: 10.1007/s10646-013-1164-8
Mella-Flores, D., Machon, J., Contreras-Porcia, L., Mesa-Campbell, S., and Dassow, P. (2018). Differential responses of Emiliania huxleyi (Haptophyta) strains to copper excess. Cryptog. Algol. 39, 481–509. doi: 10.7872/crya/v39.iss4.2018.481
Moe, S. J., De Schamphelaere, K., Clements, W. H., Sorensen, M. T., Van den Brink, P. J., and Liess, M. (2013). Combined and interactive effects of global climate change and toxicants on populations and communities. Environ. Toxicol. Chem. 32, 49–61. doi: 10.1002/etc.2045
Morin, S., Lambert, A. S., Rodriguez, E. P., Dabrin, A., Coquery, M., and Pesce, S. (2017). Changes in copper toxicity towards diatom communities with experimental warming. J. Hazard. Mater. 334, 223–232. doi: 10.1016/j.jhazmat.2017.04.016
Noyes, P. D., McElwee, M. K., Miller, H. D., Clark, B. W., Van Tiem, L. A., Walcott, K. C., et al. (2009). The toxicology of climate change: environmental contaminants in a warming world. Environ. Int. 35, 971–986. doi: 10.1016/j.envint.2009.02.006
Oukarroum, A., Perreault, F., and Popovic, R. (2012). Interactive effects of temperature and copper on photosystem II photochemistry in Chlorella vulgaris. J. Photochem. Photobiol. B Biol. 110, 9–14. doi: 10.1016/j.jphotobiol.2012.02.003
Padfield, D., Yvon-Durocher, G., Buckling, A., Jennings, S., and Yvon-Durocher, G. (2016). Rapid evolution of metabolic traits explains thermal adaptation in phytoplankton. Ecol. Lett. 19, 133–142. doi: 10.1111/ele.12545
Pascal, P. Y., Fleeger, J. W., Galvez, F., and Carman, K. R. (2010). The toxicological interaction between ocean acidity and metals in coastal meiobenthic copepods. Mar. Pollut. Bull. 60, 2201–2208. doi: 10.1016/j.marpolbul.2010.08.018
Pereira, C. M. S., Deruytter, D., Blust, R., and De Schamphelaere, K. A. C. (2017). Effect of temperature on chronic toxicity of copper, zinc, and nickel to Daphnia magna. Environ. Toxicol. Chem. 36, 1909–1916. doi: 10.1002/etc.3714
Pérez, P., Beiras, R., and Fernández, E. (2010). Monitoring copper toxicity in natural phytoplankton assemblages: application of Fast Repetition Rate fluorometry. Ecotoxicol. Environ. Saf. 73, 1292–1303. doi: 10.1016/j.ecoenv.2010.06.008
Pfeiffer, T. Ž., čamagajevac, I. Š., Maronić, D. Š., and Maksimović, I. (2018). “Regulation of photosynthesis in algae under metal stress.” in: Environment and Photosynthesis: A Future Prospect, eds V. Singh, S. Singh, R. Singh, and S. Prasad (New Delhi: Stadium Press), 261–286.
Puspitasari, R., Purbonegoro, T., and Agustin, A. (2018). “Cu toxicity on growth and chlorophyll-a of Chaetoceros sp.,” in IOP Conference Series: Earth and Environmental Science (Bandung: IOP Publishing), 012061.
Raitsos, D. E., Hoteit, I., Prihartato, P. K., Chronis, T., Triantafyllou, G., and Abualnaja, Y. (2011). Abrupt warming of the Red Sea. Geophys. Res. Lett. 38, 1–5. doi: 10.1029/2011GL047984
Rico, M., López, A., Santana-Casiano, J. M., Gonzàlez, A. G., and Gonzàlez-Dàvila, M. (2013). Variability of the phenolic profile in the diatom Phaeodactylum tricornutum growing under copper and iron stress. Limnol. Oceanogr. 58, 144–152. doi: 10.4319/lo.2013.58.1.0144
Romero-Lopez, J., Lopez-Rodas, V., and Costas, E. (2012). Estimating the capability of microalgae to physiological acclimatization and genetic adaptation to petroleum and diesel oil contamination. Aquat. Toxicol. 124, 227–237. doi: 10.1016/j.aquatox.2012.08.001
Rouco, M., López-Rodas, V., González, R., Huertas, I. E., García-Sánchez, M. J., Flores-Moya, A., et al. (2014). The limit of the genetic adaptation to copper in freshwater phytoplankton. Oecologia 175, 1179–1188. doi: 10.1007/s00442-014-2963-1
Saad, M. A. S. (1996). Heavy metal pollution in coastal Red Sea waters, Jeddah. Mar. Sci. 17, 1–2. doi: 10.4197/mar.7-1.7
Schaum, C. E., Barton, S., Bestion, E., Buckling, A., Garcia-Carreras, B., Lopez, P., et al. (2017). Adaptation of phytoplankton to a decade of experimental warming linked to increased photosynthesis. Nat. Ecol. Evol. 1:0094. doi: 10.1038/s41559-017-0094
Schiedek, D., Sundelin, B., Readman, J. W., and Macdonald, R. W. (2007). Interactions between climate change and contaminants. Mar. Pollut. Bull. 54, 1845–1856. doi: 10.1016/j.marpolbul.2007.09.020
Silva, V., Marques, C. R., Campos, I., Vidal, T., Keizer, J. J., Gonçalves, F., et al. (2018). Combined effect of copper sulfate and water temperature on key freshwater trophic levels–approaching potential climatic change scenarios. Ecotoxicol. Environ. Saf. 148, 384–392. doi: 10.1016/j.ecoenv.2017.10.035
Stauber, J. L., Binet, M. T., Bao, V. W., Boge, J., Zhang, A. Q., Leung, K. M., et al. (2008). Comparison of the Qwiklite™ algal bioluminescence test with marine algal growth rate inhibition bioassays. Environ. Toxicol. 23, 617–625. doi: 10.1002/tox.20400
Stocker, T. F., Qin, D., Plattner, G., Tignor, M., Allen, S., Boschung, J., et al. (2013). “Climate change 2013: the physical science basis,” in Intergovernmental Panel on Climate Change, Working Group I Contribution to the IPCC Fifth Assessment Report (AR5) (New York, NY).
Suratno, S., Puspitasari, R., Purbonegoro, T., and Mansur, D. (2015). Copper and cadmium toxicity to marine phytoplankton, Chaetoceros gracilis and Isochrysis sp. Indones. J. Chem. 15, 172–178. doi: 10.22146/ijc.21211
Thomas, M. K., Kremer, C. T., Klausmeier, C. A., and Litchman, E. (2012). A global pattern of thermal adaptation in marine phytoplankton. Science 338, 1085–1088. doi: 10.1126/science.1224836
Toseland, A., Daines, S. J., Clark, J. R., Kirkham, A., Strauss, J., Uhlig, C., et al. (2013). The impact of temperature on marine phytoplankton resource allocation and metabolism. Nat. Clim. Change 3, 979–984. doi: 10.1038/nclimate1989
USEPA (2016). Draft Aquatic Life Ambient Estuarine/Marine Water Quality Criteria for Copper - 2016. Available online at: https://www.epa.gov/sites/production/files/2017-02/documents/copper-estuarine-marine-draft-document.pdf (accessed March 10, 2019).
Vindimian, E. (2012). MSExcel Macro Regtox 7.06 Freely Available From Eric Vindimian. Montpellier: IRSTEA.
Wang, F., Chen, Y., Guo, Z., Gao, H., Mackey, K., Yao, X., et al. (2017). Combined effects of iron and copper from atmospheric dry deposition on ocean productivity. Geophys. Res. Lett. 44, 2546–2555. doi: 10.1002/2016GL072349
Wang, M.-J., and Wang, W. X. (2008). Temperature-dependent sensitivity of a marine diatom to cadmium stress explained by subcelluar distribution and thiol synthesis. Environ. Sci. Technol. 42, 8603–8608. doi: 10.1021/es801470w
Yi, X., Bao, V. W., and Leung, K. M. (2017). Binary mixture toxicities of triphenyltin with tributyltin or copper to five marine organisms: Implications on environmental risk assessment. Mar. Pollut. Bull. 124, 839–846. doi: 10.1016/j.marpolbul.2017.02.031
Yung, M. M., Kwok, K. W., Djurišić, A. B., Giesy, J. P., and Leung, K. M. (2017). Influences of temperature and salinity on physicochemical properties and toxicity of zinc oxide nanoparticles to the marine diatom Thalassiosira pseudonana. Sci. Rep. 7:3662. doi: 10.1038/s41598-017-03889-1
Yvon-Durocher, G., Allen, A. P., Cellamare, M., Dossena, M., Gaston, K. J., Leitao, M., et al. (2015). Five years of experimental warming increases the biodiversity and productivity of phytoplankton. PLoS Biol. 13:e1002324. doi: 10.1371/journal.pbio.1002324
Keywords: Red Sea, Chaetoceros, warming, adaptation, copper toxicity, Fv/Fm
Citation: Kottuparambil S, Jin P and Agusti S (2019) Adaptation of Red Sea Phytoplankton to Experimental Warming Increases Their Tolerance to Toxic Metal Exposure. Front. Environ. Sci. 7:125. doi: 10.3389/fenvs.2019.00125
Received: 06 May 2019; Accepted: 16 August 2019;
Published: 29 August 2019.
Edited by:
Andrew Hursthouse, University of the West of Scotland, United KingdomReviewed by:
C. Elisa Schaum, Universität Hamburg, GermanyVictoria Lopez-Rodas, Complutense University of Madrid, Spain
Copyright © 2019 Kottuparambil, Jin and Agusti. This is an open-access article distributed under the terms of the Creative Commons Attribution License (CC BY). The use, distribution or reproduction in other forums is permitted, provided the original author(s) and the copyright owner(s) are credited and that the original publication in this journal is cited, in accordance with accepted academic practice. No use, distribution or reproduction is permitted which does not comply with these terms.
*Correspondence: Sreejith Kottuparambil, c3JlZWppdGgua290dHVwYXJhbWJpbEBrYXVzdC5lZHUuc2E=