- Department of Geology and Geography, West Virginia University, Morgantown, WV, United States
Abundant resources and efforts have been employed in the search for life on Mars. Satellites, landers, and rovers have tested atmospheric gases, general sediment and rock compositions, and images of Mars surface in an effort to detect biosignatures left by any possible modern or ancient life. Chloride and sulfate minerals suggestive of past acid saline lakes have been found on Mars. In terrestrial acid brine environments, these minerals trap microorganisms and organic compounds and preserve them within fluid inclusions and as solid inclusions for long geologic time periods. Some cells remain viable, especially in the isolated, microscopic aqueous environments of fluid inclusions. Fluid inclusions in these same saline minerals on Mars have yet to be examined. This paper describes petrographic and geochemical methods that have been used recently to detect and make general identifications of microorganisms and organic compounds preserved in modern and Permian Mars-analog acid saline lake halite and gypsum. It then makes recommendations for how Martian chemical sediments could be examined for these biosignatures, both by rovers and in returned samples. This new protocol for the examination of Martian chemical sediments and sedimentary rocks may provide the next step for detection of any preserved biosignatures on Mars.
Introduction
Primary fluid inclusions in chemical sediments, such as bedded halite and gypsum, are remnants of the parent surface waters that precipitated these minerals (Goldstein and Reynolds, 1994; Goldstein, 2001). As such, they preserve past hydrosphere (e.g., Benison et al., 1998; Lowenstein et al., 2001), past lithosphere (e.g., Lowenstein and Spencer, 1990), past atmosphere (e.g., Blamey et al., 2016), and past biosphere (e.g., Mormile et al., 2003; Schubert et al., 2010a; Lowenstein et al., 2011; Sankaranarayanan et al., 2014). In particular, rapid growth of halite and gypsum from surface brines makes their primary fluid inclusions excellent “snapshot” repositories for these various components of the environment. Halite, in particular, has been observed (by the author) growing at rates of up to ~5 mm/h, in shallow brines in Western Australia (Figure 1). As long as water is saturated with respect to Na+ and Cl− and evaporation rates are high, halite can precipitate fast enough at the brine-water interface that flying insects that land on the salt crystals get trapped because their legs are encased in newly-precipitated salt in minutes (Figure 1E). As halite grows, microscopic “step-like,” cleavage-controlled irregularities exist on the crystal faces, where the crystal is in contact with parent water. Slow precipitation of halite fills the irregularities, resulting in clear halite. In contrast, rapid growth of halite covers the irregularities, effectively enclosing the surface brine and any other tiny matter from the environment as fluid inclusions. Differing rates of growth, due primarily to changes in evaporation rate caused by diurnal temperature-influenced humidity fluctuations, form aligned primary fluid inclusions (or growth bands) parallel to crystal faces. Alternating fluid inclusion-rich and fluid-inclusion-poor growth bands in halite have been interpreted as representative of diurnal halite growth (i.e., Dellwig, 1955; Roberts and Spencer, 1995; Benison and Goldstein, 1999). Because halite chevron and cumulate crystals can grow in shallow surface brines, commonly at the water-air interface, they are highly efficient at trapping surface water, air, and solids and microorganisms in the waters or blown in by wind. Gypsum likely grows slower than halite, yet little data exists in the literature about rate of growth of gypsum in nature. Regardless, gypsum grows rapidly compared to most minerals; bottom-growth gypsum formed at the brine-sediment interface contains alternating inclusion-rich and inclusion-poor growth bands (Benison et al., 2007). In addition to fluid inclusions, growth bands in halite and gypsum commonly contain solid inclusions of other minerals and biological material. Unaltered primary fluid inclusions and solid inclusions in chemical sediments such as halite and gypsum serve as high-resolution and high-fidelity proxies for environmental conditions, including life.
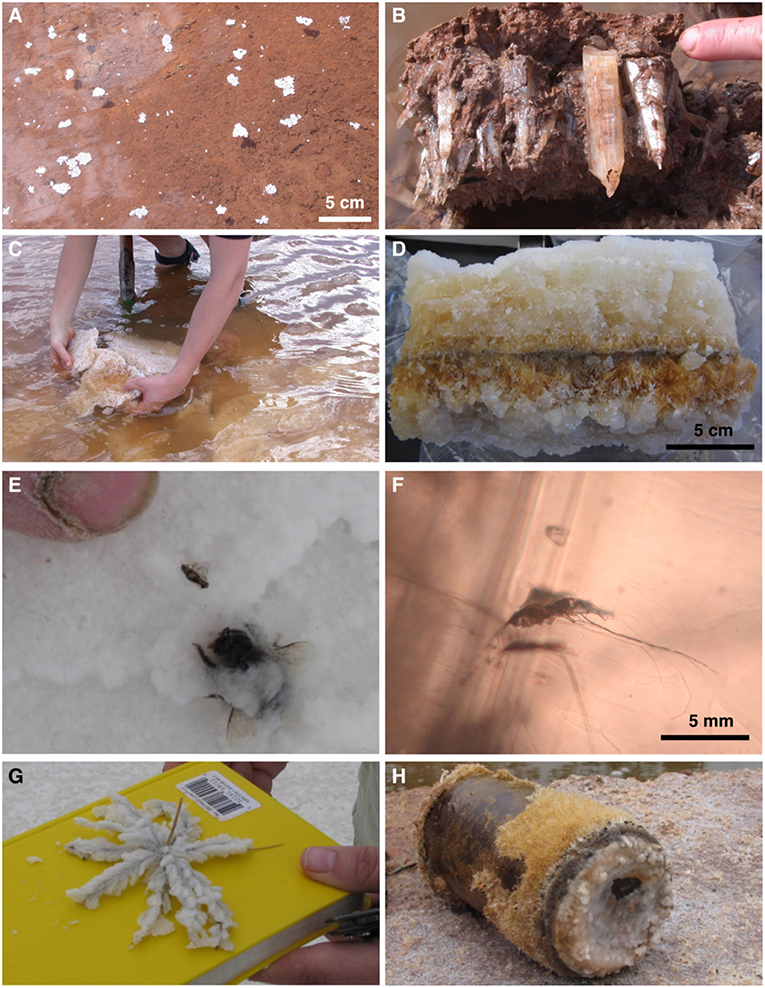
Figure 1. Photographs of halite and gypsum at acid (pH 1.6–2.7) saline (28–32% TDS) lakes in Western Australia, associated hematite, jarosite, and alunite, and its ability to coat objects rapidly. (A) White halite rafts of cumulate crystals in ~10 cm deep water at Lake Brown. White rafts with shadows are floating on air-water interface; white rafts without shadows have sunk to hematite mud lake bottom. (B) Gypsum bottom-growth crystals from Lake Aerodrome; red much is composed of hematite, jarosite, and kaolinite. (C) Halite chevron crystal crust from bottom of Lake Aerodrome. (D) Cross-sectional view of salt crust from Lake Gounter. Pale orange and white blocky crystals in top and bottom beds, respectively, are halite. Needle-like crystals in middle orange strata are gypsum. Orange color is due to iron oxide and jarosite. (E) Beetle trapped in halite at Twin Lake West; author observed beetle land on halite crust and get encased in halite as it grew rapidly. (F) Mosquito trapped in halite from Lake Aerodrome. (G) Spinifex grass coated with halite, Twin Lake West. (H) Beer can coated with halite and gypsum crystals, Lake Brown.
On Earth, modern acid brines lakes in Western Australia and Chile have pH as low as 1.4 and salinities as high as 32% total dissolved solids (TDS). In Western Australia, individual lakes have varying water chemistries, mainly in response to evaporation and flooding conditions over days, weeks, and months (Benison et al., 2007; Bowen and Benison, 2009). Ephemeral lake waters fluctuate within the 1.4 to ~4 pH range and ~280 to ~32% TDS salinity range. In Permian halite from acid saline lake deposits, −1 pH in primary fluid inclusions has been measured (Benison et al., 1998). The combined low pH and high salinity makes terrestrial acid saline lakes amongst the most chemically extreme environments on Earth.
Terrestrial acid brine lakes host diverse communities of extremophiles (e.g., Mormile et al., 2009; Johnson et al., 2015; Escudero et al., 2018; Zaikova et al., 2018). The same modern acid brine lakes in Western Australia and Chile that contain microorganisms and organic compounds in their waters also precipitate halite and gypsum that contain microorganisms and organic compounds in fluid inclusions and as solid inclusions (i.e., Conner and Benison, 2013; Benison and Karmanocky, 2014). Limited previous work has suggested ancient acid saline lake halite hosts microorganisms in fluid inclusions (Benison et al., 2008a; Benison, 2013).
Halite is an excellent host for microfossils. As long as terrestrial halite remains at low-humidity and low-moderate temperatures and pressures, such as at subsurface depths of ~300–900 m, it can remain unaltered for hundreds of millions of years (i.e., Benison et al., 1998; Benison and Goldstein, 1999; Lowenstein et al., 2001; Mormile et al., 2003; Blamey et al., 2016). In contrast, terrestrial gypsum succumbs to dehydration to become anhydrite. On Earth, unaltered gypsum older than Miocene is rare.
Acid brine lakes have been interpreted as the depositional environments for some strata on Mars (e.g., Benison and LaClair, 2003; Squyres et al., 2004; Benison and Bowen, 2006). Chemical sediments, including chlorides such as halite and hydrated sulfates such as gypsum, have been detected on Mars (e.g., Clark and Van Hart, 1981; Gendrin et al., 2005; Osterloo et al., 2008; Glotch et al., 2010). In both terrestrial acid lake systems and in some Mars strata, the mineral assemblage consists of halite, gypsum, other sulfate minerals, iron oxides, jarosite, alunite, clay minerals, and some opalline silica (i.e., Burns, 1987, 1994; Benison and LaClair, 2003). Sedimentary structures and diagenetic features on Mars also are comparable to those in terrestrial acid saline lakes (i.e., Benison, 2006; Benison and Bowen, 2006). Yet, to date, solid inclusion and fluid inclusion studies have not been attempted in Martian chemical sediments.
This paper builds on preliminary data of microfossils in modern acid lake halite and gypsum. The goals of this paper are to: (1) present new observations and summarize new and previously-described microorganisms and organic compounds in modern and Permian acid halite and modern and Pleistocene gypsum; (2) review and evaluate the geological and geochemical methods that can be used to detect microorganisms and organic compounds in fluid inclusions and as solid inclusions in halite and gypsum; (3) distinguish between some biotic and abiotic signatures; and (4) make a case for, and offer recommendations for, the use of these methods on Martian chemical sediments and sedimentary rocks to expand the search for life on Mars.
The petrographic and laser Raman spectroscopic methods described in this paper could prove to be easier to conduct remotely on Martian samples than traditional microbiological methods such as culturing and sequencing. Most of the instruments needed to conduct this work are currently available in rovers on Mars, or are planned for the future Mars 2020 mission. Therefore, these petrographic and spectroscopic methods may allow for a screening test to non-destructively evaluate chemical sediments and chemical sedimentary rocks on Mars for suspect biological matter prior to their return to Earth and subsequent traditional microbiological analyses.
Overview of Search for Life on Mars
Although no evidence of life has been found on Mars to date, several observations have suggested that past life may have been possible. Minerals that form on Earth from water, such as chlorides, iron oxides, and sulfates, including hydrated forms of such minerals, such as gypsum, have been found on Mars (e.g., Clark and Van Hart, 1981; Burns, 1987; Klingelhöfer et al., 2004; Clark et al., 2005; Osterloo et al., 2008; Farrand et al., 2009; Glotch et al., 2010; Ehlmann et al., 2016). Terrestrial life is found in the same places as terrestrial water; even the most extreme aqueous environments on Earth, such as acid brine lakes, hot springs, subglacial lakes, and deep ocean trenches, host life (i.e., Mikucki et al., 2010; Zaikova et al., 2018). Therefore, the presence of water-precipitated minerals on Mars suggests the possible habitability of this planet (Kargel, 2004).
Biological and chemical clues for life on Mars are more elusive. Polycyclic aromatic compounds and a chain of carbonate globules in meteorite ALH84001 were speculated to be fossil remains of past microorganisms (McKay et al., 1996). However, contamination of the meteorite on the Earth surface was never discounted. Recently, two stronger lines of evidence for possible biological activity were reported from Mars. Eigenbrode et al. (2018) reported organic sulfur molecules, including aromatic C4H4S compound thiophene, in ancient mudstones in Gale Crater. In addition, seasonal fluctuations in methane in the Martian atmosphere have been detected (Webster et al., 2018), suggesting a biological cycling process. These recent discoveries encourage continued work to search for current or past life on Mars, especially that work targeted at preservation and recognition of extremophilic microorganisms.
A challenge to preservation of any organic material on Mars is the high UV-radiation at the planet's surface. UV radiation on modern Martian surface is ~3.5 times higher than it was on early Mars, and similar to UV radiation on the surface of early Earth (Cockell et al., 2000). Because UV radiation breaks down organic compounds, Mars' high UV radiation has led many researchers to consider it an inhospitable environment for life and its preservation. However, microorganisms trapped within chemical sediments are subjected to less radiation than a microorganism exposed at the planet's surface (Phoenix et al., 2006). Furthermore, experiments have shown that, in Mars simulated environment, 2–5 mm thick coatings of iron oxides and/or iron hydroxides, including jarosite, and alunite. block UV radiation (Gomez et al., 2010). Therefore, it is possible that any biological material trapped in halite or gypsum that is covered by iron oxides or iron hydroxides could remain preserved for long periods of geological time.
The Case for Acid Brines on Mars
Terrestrial acid brines, such as modern acid saline lakes on the Yilgarn Craton in Western Australia and volcaniclastic-hosted acid salars in northern Chile, precipitate an unusual suite of minerals, including halite, gypsum and other sulfate salts, iron oxides, jarosite, alunite, kaolinite and other clay minerals, and opalline silica (e.g., Risacher et al., 2002; Benison et al., 2007; Story et al., 2010; Karmanocky and Benison, 2016). These same minerals are found in several places on Mars, including Meridiani Planum, Gusev Crater, and Gale Crater (e.g., Burns, 1987; Klingelhöfer et al., 2004; Madden et al., 2004; Clark et al., 2005; Bibring et al., 2006; Osterloo et al., 2008; Farrand et al., 2009; Glotch et al., 2010; Ehlmann et al., 2016). For these reasons, as well as for their thermochemical stability in Martian conditions, acid brines have been proposed as a likely past liquid on Mars (Burns, 1994; Clark, 1994; Benison and LaClair, 2003; Squyres et al., 2004; Benison and Bowen, 2006; Benison et al., 2008b).
Sedimentological evidence on Mars suggests past shallow lake, mudflat/sandflat, and eolian environments and past shallow acid saline groundwaters (e.g., Squyres et al., 2004; Grotzinger et al., 2005, 2015; Benison, 2006; Benison and Bowen, 2006; Metz et al., 2009). Sedimentary structures in some lithified strata include wave ripple cross-stratification, suggesting shallow ephemeral surface waters. Sandstones with various types of cross-bedding indicate eolian and fluvial sediment transportation. Diagenetic features found on Mars, including pseudomorphs after displacive sulfate crystals and hematite concretions, are characteristic of past environments with shallow acid saline groundwaters. Such diagenetic features have been documented in modern and Permian acid saline lake systems (Benison and Goldstein, 2001; Benison, 2006; Benison and Bowen, 2006; Benison et al., 2007; Bowen et al., 2008, 2012). Modern acid salars in northern Chile may have the most in common with some Martian strata; they contain the acid saline mineral suite, similar depositional and diagenetic features, and are also hosted by volcaniclastic sediments (Benison and Karmanocky, 2014; Karmanocky and Benison, 2016) and experience strong winds (Benison, 2017). In both Western Australia and Chile, halite and gypsum are closely associated with, and commonly coated with hematite, jarosite, and/or alunite. The mineralogical, geochemical, sedimentological, and diagenetic characteristics of Martian sediments and sedimentary rocks are consistent with acid saline systems.
Microbiology of Terrestrial Acid Brines
A limited number of recent studies have focused on identification of microorganisms in acid brines. DGGE and clone libraries documented distinct microbial communities among 11 lakes with pH as low as 2.7 on the Yilgarn Craton of southern Western Australia (Mormile et al., 2009). Wood and Kelly (1991) observed growth of uncharacterized, halophilic, heterotrophic bacteria in enrichments of acid and neutral (pH 2.8–5.5) saline water from two lakes in Western Australia. Jones et al. (2006) and Podell et al. (2014) reported two separate microbiological studies of Lake Tyrrell in Victoria in moderately acid (pH 4.7–5.5), moderately saline (~3.5% total dissolved solids, or TDS) lake waters. Lu et al. (2016), using 16S rRNA analyses, identified Acidophillim and Acidocella bacteria and archaea Aplasma in moderately acid, moderately saline river sediments in southernmost Western Australia. The first metagenomic study of Western Australian acid saline lakes showed a diversity of acidophilic and acidotolerant microorganisms in pH 3.6 Lake Gneiss water (Johnson et al., 2015). Zaikova et al. (2018) found that pH 1.6, 32% TDS Lake Magic in southern Western Australia contains a diverse community of archaea, bacteria, algae, and fungi, many of which are novel. Recent microbiological surveys have also been conducted in acid brines at Salar Gorbea in northern Chile and have detected diverse community of prokaryotic extremophiles, including Acidithiobacillus thiooxidans (Demergasso et al., 2010; Davis-Belmar et al., 2013; Escudero et al., 2013, 2018; Quatrini et al., 2017). These studies found that acid brines host microbiologically diverse communities with many novel genera and species related to the complex chemistry in these extreme environments.
A number of environmental extremes besides low pH and high salinity exist at acid saline lakes. These settings also tend to have high UV radiation, large diurnal temperature ranges, and strong winds. In addition, in Western Australia, water salinity, pH, and chemical composition fluctuate due to flooding, evapoconcentration, and desiccation that occur on daily—decadal scales (Benison et al., 2007; Bowen and Benison, 2009; Benison and Bowen, 2015). Furthermore, the most extreme Western Australian acid brines have low water activity measurements which approach those traditionally accepted as the lower limit of life on Earth (Grant, 2004; Williams and Hallsworth, 2009; J. Hallsworth, pers. comm.). These low water activities are likely due to their low pH, high salinity, and complex chemistry. Therefore, microorganisms in ephemeral acid brine lakes must be considered polyextremophiles, microorganisms adapted to multiple extreme conditions (Bowers et al., 2009). That is, the microorganisms in these acid saline lakes in Western Australia, Chile, and the Permian of Pangea must be adapted to live in low pH (<2), high salinities (> ~32% TDS), and high UV radiation, as well as great fluctuations in diurnal and seasonal temperatures, water chemistry, and water availability (including desiccation).
Microorganisms and Organic Compounds Within Halite and Gypsum
Microfossils have been described previously, both as solid inclusions and in fluid inclusions, within neutral—alkaline halite and gypsum. Tasch (1963) provided an overview of fossils trapped in modern and ancient halite, including bacteria, fungi, diatoms, brine shrimp, pollen, and wood. Gypsum-hosted microfossils, including filamentous bacteria and diatoms, have been discovered in modern, Miocene, and Permian deposits (Schopf et al., 2012). Schluter et al. (2003) documented dragonflies and Dela Pierre et al. (2015) found diatoms, algae, and bacteria preserved in Miocene gypsum crystals from northern Italy.
Microorganisms entrapped within fluid inclusions in natural and laboratory-grown halite from neutral–alkaline brines have been documented (e.g., Fendrihan and Stan-Lotter, 2004; Adamski et al., 2006; Fendrihan et al., 2006). Mormile et al. (2003) directly isolated Halobacterium salinarum from a fluid inclusion in 97-kyr halite from Death Valley, California. Prokaryotes and the halophilic alga Dunaliella were found co-existing in primary fluid inclusions in halite as old as 30 kyr from Death and Saline Valleys in California (Schubert et al., 2009a,b, 2010a,b). Lowenstein et al. (2011) suggested organic compounds, such as glycerol derived from Dunaliella cells, may be used by prokaryotes for long-term survival within fluid inclusions in halite.
Microorganisms have also been documented in fluid inclusions in ancient neutral-alkaline halite (e.g., Reiser and Tasch, 1960; Dombrowski, 1963, 1966; Norton and Grant, 1988; Grant et al., 1998; Stan-Lotter et al., 1999; McGenity et al., 2000). Bacteria from a fluid inclusion in Permian halite and halophilic archaea in fluid inclusions in Cretaceous halite have been reported (Vreeland et al., 2000, 2007). Fish et al. (2002) extracted ancient bacterial and archaeal DNA from 11-425 myr halites. Recently, the genome was reported for a Halobacterium noricense-related archaeum isolated from 123-million year-old halite (Jaakkola et al., 2016).
Fluid inclusions have been previously considered as a potential source of paleoenvironmental and biological data for Mars. Bodnar (1999) described how fluid inclusions in Martian meteorites might yield information about past liquid and gas compositions and pressures on Mars. Zolensky et al. (1998) and Chan et al. (2018) identified fluid inclusions in halite and sylvite in two Martian meteorites. However, limited petrographic descriptions suggest that these fluid inclusions are large, isolated, and likely altered, so they may not be considered proxies for past Martian surface conditions. Parnell and Baron (2004) suggested that a wide range of chemical precipitate minerals on Mars should be examined for past waters that may contain biomolecules.
To date, no isolation, enrichment, and culturing from fluid inclusions in acid-precipitated halite or gypsum has been conducted. However, improved optics using long-working distance microscope objectives have allowed detection of objects as small as 1 micron within fluid inclusions in clear minerals such as halite and gypsum. As a result, microorganisms and organic compounds have been imaged in primary fluid inclusions in halite from acid saline Lake Magic in Western Australia and in gypsum from two acid salars in northern Chile (Conner and Benison, 2013; Benison and Karmanocky, 2014). In addition, UV-vis fluorescence and laser Raman spectroscopy has been used to chemically characterize biotic and abiotic objects in these acid fluid inclusions (Conner and Benison, 2013; Karmanocky and Benison, 2016).
Primary fluid inclusions in chevron halite, sampled at pH 1.7–2.3, 28–32% TDS salinity, cm-deep lake water from Lake Magic, Western Australia, contain 1–3 μm cocci that fluoresce green, 5–7 μm dimpled yellow spherules that fluoresce blue, and yellow-orange globules that fluoresce orange-red (Conner and Benison, 2013). The cocci are likely bacteria or archea, the dimpled yellow spherules resemble Dunaliella algae (J. Polle, pers. comm.), and the yellow-orange globules were preliminarily identified as organic compounds. Laser Raman spectroscopic analyses of suspect Dunaliella cells yielded spectra characteristic of disordered graphite. Yellow-orange globules have Raman spectra matching beta-carotene (Conner and Benison, 2013; Winters et al., 2013).
Preliminary investigation of primary fluid inclusions in bottom-growth gypsum at acid Salars Gorbea and Ignorado (northern Chile), sampled at pH 1.8–4.6, 5–28% TDS salinity, showed 4–5 μm rod-shaped and 1–2 μm cocci-shaped prokaryotes, 3–10 μm dimpled yellow spherules, ~5 μm clear spherulitic solids, and ~30 μm reworked pennate diatoms (Benison and Karmanocky, 2014). The dimpled yellow spherules may be an unknown species of Dunaliella algae (J. Polle, pers. comm.; Oren, 2005). Laser Raman spectroscopic analyses yielded spectra characteristic of disordered graphite for algal cells in fluid inclusions, and Raman spectra confirming that the clear spherulitic solids are long-chain hydrocarbons (Karmanocky and Benison, 2016).
Transmitted and UV-vis petrography, laser Raman spectroscopy, and limited scanning electron microscopy (SEM) have also been used to characterize unusual biomasses (“hairy blobs”) trapped both in fluid inclusions and as solid inclusions in halite and gypsum from modern extremely acid lakes and their ancient deposits (Benison et al., 2008a; Jagniecki and Benison, 2010). These masses are composed of both microbial remains and sulfate crystals and seem unique to acid-precipitated minerals (Benison, 2013).
The preservation potential of biosignatures in acid halite and gypsum is enhanced by co-precipitation of less soluble minerals, such as hematite and clay minerals. Bottom-growth gypsum in Western Australia contains growth bands lined with hematite mud and clay minerals, which alternate with fluid inclusion growth bands. Observed precipitation events (“reddings” and “whitings”) and water chemistry at modern acid saline Lake Aerodrome in Western Australia shows that the hematite and clay precipitated directly from the same lake waters that formed the gypsum (Benison et al., 2007; Bowen and Benison, 2009). Hematite concretions and early hematite, jarosite, alunite, and clay cements indicate that shallow groundwaters precipitate these minerals (Bowen et al., 2008, 2012). Blue-fluorescing Dunaliella algal cells have been found in fluid inclusions in hematite-coated gypsum sand grains from dunes near acid saline lakes in Western Australia (Benison et al., 2016). Hematite concretions in Western Australia contain reworked halite and gypsum grains, which host some biosignatures in the form of cocci prokaryotes and “hairy blob” organic masses (Farmer et al., 2009). Coatings of iron oxides in concretions protect the halite and gypsum biosignature hosts from dissolution from dilute waters and from degradation by UV radiation (Gomez et al., 2010). These same occurrences of chemical sediments as reworked grains and as clasts within concretions may also preserve biosignatures in halite and gypsum on Mars.
Methods for Detecting Microorganisms and Organic Compounds in Fluid Inclusions
Sample Selection and Preparation
All samples used for this study were halite or gypsum precipitated in acid brine lake waters. Fieldwork, core descriptions, and petrography were used to identify crystals that precipitated in shallow (cm-scale) acid saline lakes (see Benison and Goldstein, 2000, 2001; Benison et al., 2007; Karmanocky and Benison, 2016 for foundational work for the samples used in this study). Analyses focused on bottom-growth and cumulate halite and bottom-growth gypsum because depositional environment could be demonstrated. Petrographic observations were used to evaluate for any alteration features and to select only unaltered primary fluid inclusions for further analyses (Goldstein and Reynolds, 1994). Fluid and solid inclusions along growth bands were chosen for their high potential to yield biotic material trapped in the depositional environment.
Modern samples were collected by the author at acid saline lakes in southern Western Australia and northern Chile (Benison et al., 2007; Benison, 2019). Two Pleistocene (?) gypsum samples from LA2-09 core from below Lake Aerodrome in Western Australia were examined (Benison et al., 2011). Permian samples were taken from cores of the Nippewalla Group (including the Salt Plain, Cedar Hills, Flowerpot, and Blaine Formations) from the subsurface of Kansas and the Opeche Shale from the subsurface of North Dakota (Benison and Goldstein, 2000, 2001; Benison et al., 2013). Table 1 provides details about samples and methods used for each.
Crystals used for fluid inclusion studies need to be thin enough for light to penetrate well, but thick enough for growth bands composed of primary fluid inclusions to maintain their integrity. For clear minerals with cleavage, such as halite and gypsum, manually cleaving the crystals with a razor blade to a thickness of 0.5–2 mm provides a flat crystal surface and appropriate thickness for the best optical examination of fluid inclusions. Cleaving samples by razor also eliminates the need to polish crystals, which can introduce abundant scratches to soft minerals such as halite and gypsum. Cleaved crystal chips are stored in glass or plastic vials. Beads of desiccant are added to vials containing halite so the halite crystal surfaces do not develop pits due to dissolution by atmospheric moisture. However, storage of prepared crystals with desiccant is only necessary if analyses will be delayed for more than ~1 week.
Transmitted Light Microscopy
Finding and documenting microorganisms and organic compounds in fluid inclusions in halite and gypsum and other chemical sediments requires a high-quality microscope with transmitted light, long-working distance microscope objectives, and a high-resolution digital imaging system attached to the microscope. The ability to center and focus the light source is crucial; centering and focusing should be done each time a new mineral chip is used and each time a the microscope objective is changed (Goldstein and Reynolds, 1994). Long-working distance objectives of 60x and 100x magnification, paired with a microscope with additional magnification through eyepieces and magnification dial, allow total magnification in the 1,200x−2,000x range (e.g., Conner and Benison, 2013).
UV-vis Microscopy
Mormile and Storrie-Lombardi (2005) described the use of UV microscopy to identify biomarkers in halite, based on fluorescent response. We use a combined 330 nm and 385 nm illumination at the same magnification range and with the same microscope as we use for transmitted light microscopy. Using the UV-vis 330 and 385 nm light alone detects only features with a fluorescent response; host halite, gypsum, most inclusion waters, and any air bubbles in fluid inclusions remain dark. Carbon-bonded and hydrogen-carbon-bonded substances fluoresce and their colors can be seen best when transmitted light is blocked and only UV light is used. However, using both transmitted light and UV-vis light at the same time allows for the fluorescing features to be placed in context.
Laser Raman Spectroscopy
Laser Raman spectroscopy measures the shift in wavelength resulting from the excitation of electrons in covalent bonds by a focused laser. This method can be used, through a microscope, to detect covalently-bonded solids, liquids, and gases as small as 1 micron in diameter. Peaks at characteristic wavenumbers differing from the incoming laser wavenumber are used to identify the chemical composition of the targeted substance.
Because the mineral halite contains no covalent bonds, it yields no Raman peaks and, therefore, makes an ideal host for the laser Raman spectroscopic analyses of fluid inclusions and solid inclusions. In contrast, gypsum has a Raman spectra composed of several narrow, sharp peaks. Any laser Raman spectroscopic analyses of fluid inclusions or solid inclusions in gypsum will result in spectra that include Raman peaks attributable to both the inclusion material and the host gypsum. For this reason, some compounds, such as aqueous sulfate in an inclusion fluid, cannot be easily distinguished from the Raman peak for sulfate in gypsum. But other Raman peaks that do not coincide with those for the host gypsum can be distinguished. Thus, many organic compounds can be identified in gypsum with laser Raman spectroscopy (i.e., Karmanocky and Benison, 2016).
We have used four different laser Raman microprobe models: (1) a Kaiser Optical Holoprobe at Central Michigan University, (2) a S-3000Jobin Yvon at Washington University, (3) a JY/Horiba LabRam HR at the U.S. Geological Survey-Reston, and (4) a Renishaw InVia microprobe at West Virginia University. We employed two lasers at each facility, including either a 514 nm or a 532 nm green argon laser with a spectral range of ~100–~4,275 cm−1) and a 785 nm near IR red laser with a spectral range of ~200–~3,200 cm−1. Optimal power settings depend upon the magnification used for analyses. For 100x magnification, 1.4–1.52 mW worked best; 0.84–0.94 mW and 0.5–0.64 mW were optimal power settings for magnifications of 500x and 1,000x, respectively.
Results
Results shown here are compiled examples of transmitted and UV-vis petrography and laser Raman spectroscopy conducted on bedded halite and gypsum from various acid saline lake deposits. Petrographic observations were made using transmitted, UV-vis, and combined transmitted and UV-vis light at magnifications up to 2,000x (Figure 2). A variety of microorganisms and organic compounds were observed and described in fluid inclusions in acid halite and gypsum. In addition, some microfossils were also found as solid inclusions within the halite and gypsum.
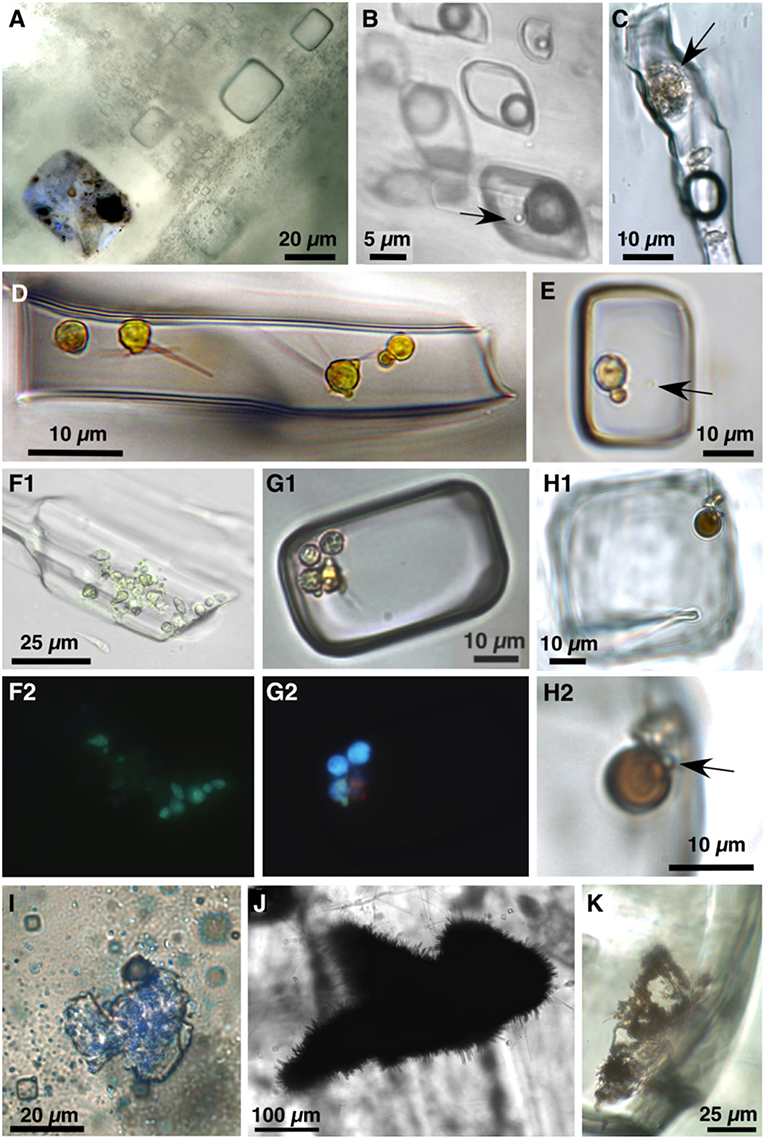
Figure 2. Photomicrographs of biological material preserved within primary fluid inclusions and as solid inclusions in modern (A–E,F1/F2,G1/G2) and Permian (H1/H2,I–K) acid lake-precipitated halite and gypsum. All photos in plane transmitted light unless otherwise indicated. (A) Primary fluid inclusions in halite precipitated from pH 1.4 32% TDS salinity Gneiss Lake in Western Australia. Inclusion in the lower left contains liquid, solid dark organic matter, and cells, including cocci bacteria and algae; combined plane transmitted and UV-vis light. (B) Primary fluid inclusions in gypsum from acid Salar Gorbea in northern Chile. Inclusion in lower right contains liquid, H2S gas, and cocci (arrow); modified from Benison and Karmanocky (2014). (C) Algal cell (arrow) in fluid inclusion in Salar Gorbea gypsum; modified from Benison and Karmanocky (2014). (D) Yellow algal cells with flagella in fluid inclusion in halite from Lake Gounter, Western Australia. Photograph courtesy of Tim Lowenstein and Michael Timofeeff. (E) Two yellow algal cells and yellow prokaryote cocci (arrow) in fluid inclusion in halite from Twin Lake West, Western Australia. (F1,F2). Paired transmitted light (F1) and UV light (F2) view of cells in fluid inclusion in gypsum from Lake Aerodrome, Western Australia. (G1,G2) Paired transmitted light (G1) and UV-vis light (G2) views of fluid inclusion in halite from pH 1.8 Lake Magic, Western Australia, with three Dunaliella algal cells (blue fluorescence), two prokaryotic cocci (green fluorescence), and beta-carotene (pink fluorescence); modified from Conner and Benison (2013). (H) Suspect spherical brown algal cell and adjacent yellow organic suspects in fluid inclusion in bedded halite from Permian Salt Plain Formation, Kansas. (H1) View of entire inclusion. (H2) Close-up view of microbial suspects. Arrow points to pale green cocci prokaryote. (I) Solid inclusion of thin, transparent organic matter in bedded halite of Permian Salt Plain Formation, Kansas; combined plane transmitted and UV light. (J) “Hairy blob” solid inclusion in bedded halite from the Permian Blaine Formation, Kansas. (K) “Hairy blobs” within primary fluid inclusion in Permian Salt Plain Formation, Kansas.
Suspect microorganisms were distinguished by their size, shape, and appearance (Figure 2). Cocci are 1–2 μm diameter, clear, pale yellow, or pale green spheres of high relief that are suspected to be bacteria or archea. A second, less common, type of suspect prokaryote are 4–5 μm long rods. Dimpled, 3–10 μm diameter spheres, typically yellow, are considered to be algae; many share morphological appearance and size with the halophilic alga genus Dunaliella (Oren, 2005). Orange-brown, ~8–10 μm diameter spheres may be a different type of alga. Uncommon algal cells have flagella. These suspect microorganisms were observed within fluid inclusions, as well as solid inclusions.
Microorganisms and organic compounds were not detected in every fluid inclusion growth band observed with petrography in modern and ancient halite and gypsum. Some growth bands contained suspect microorganisms and organic compounds in an estimated 20% of fluid inclusions; this represents the most abundant organics detected, perhaps trapped as a microbial “bloom” occurred in the depositional environment. In addition, no relationship between fluid inclusion size and presence of organic material was noted.
In Permian halite, primary fluid inclusions were well-preserved and unaltered. Microorganisms and organic compounds were approximately as common as in Permian halite as in modern halite and gypsum. In contrast, Permian bedded gypsum has been altered, most likely by repeated dehydration to anhydrite and re-hydration back to gypsum; thus, no primary fluid inclusions have been seen in the Permian gypsum in this study.
Organic suspects in fluid inclusions as seen in transmitted light also had some fluorescent response to UV-vis light. Organic suspects in modern halite and gypsum typically had stronger, brighter fluorescence than did Permian suspects. The duller fluorescence on the Permian organic suspects may be due to decay over time since entrapment. Figure 2 shows representative images of microorganisms and organic compounds in modern and ancient acid-precipitated halite and gypsum.
Some growth bands in acid-precipitated halite and gypsum were not composed solely of fluid inclusions. Some growth bands are composed of solid inclusions, including minerals (most commonly gypsum in halite and hematite and kaolinite in halite and gypsum), as well as some suspect organic matter. In other growth bands, some solid organic inclusions are associated with primary fluid inclusions. Solid inclusions observed included diatoms in Chilean gypsum (Benison and Karmanocky, 2014) and a scorpion, and whole insects and insect fragments in halite from Western Australia. Solid organic inclusions also included thin, transparent sheets (Figure 2I) and “hairy blobs,” clumps of organic matter and sulfate crystals (Figures 2J,K, 3C,D), as well as 1–2-micron-sized yellow and orange cocci, and 3–10 μm diameter dimpled yellow spheres. UV-vis fluorescent response seems to depend upon thickness and transparency of sample. For example, larger hairy blobs do not typically fluoresce, but smaller hairy blobs and thin, transparent sheets do. Fluorescence seems slightly duller in Permian solid organic inclusions than in comparable modern inclusions; however, this has not been quantified. The strength of the detected fluorescent responses to UV-vis light is likely due to depth within crystal of fluid or solid inclusion, size and density of targeted material, and focus of the microscope at high magnification, and age and/or amount of decay of targeted material.
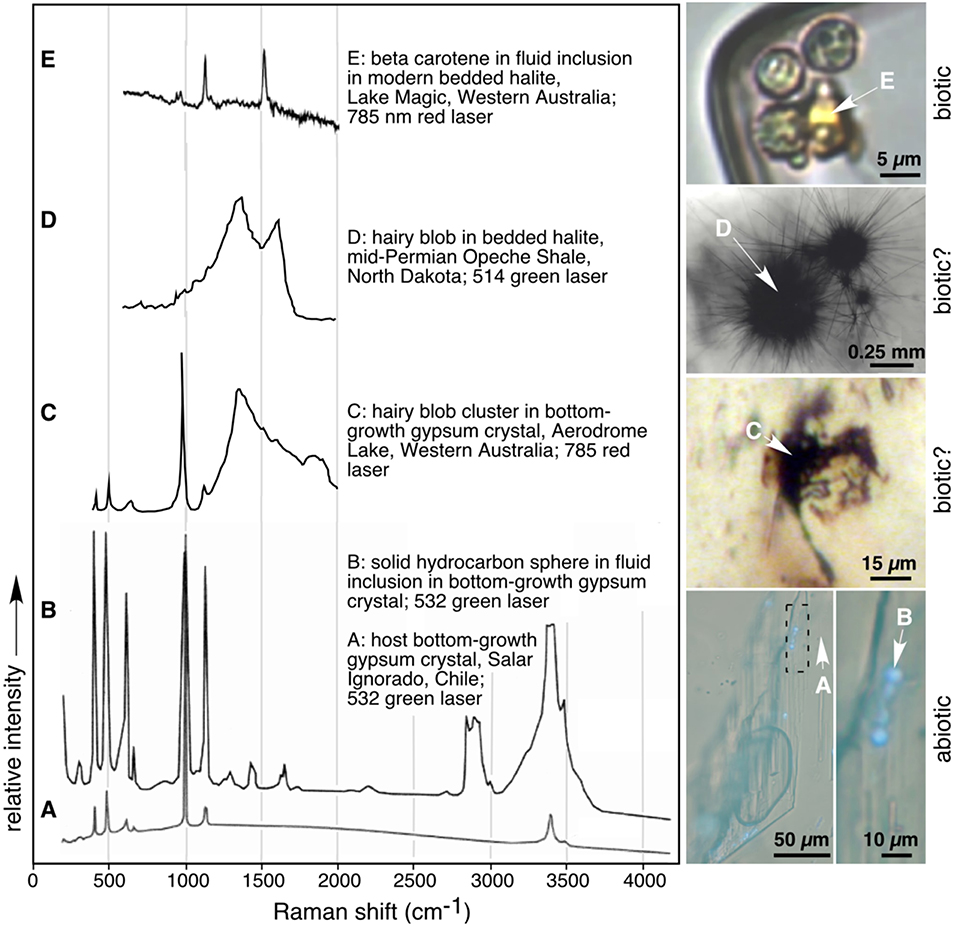
Figure 3. Stacked Raman spectra and corresponding images of modern and Permian abiotic (A,B), likely biotic (C,D), and biotic (E) features in Mars-analog acid halite and gypsum. White arrows in photomicrographs point to spot of analyses. (A) Modern solid bottom-growth gypsum host crystal and (B) solid sphere of long-chain hydrocarbons within fluid inclusion in (A); Salar Ignorado, Chile; modified from Karmanocky and Benison, 2016. (C) Biotic hairy blob cluster in bottom-growth gypsum crystal from modern Lake Aerodrome, Western Australia. Note sharp peaks characteristic of covalent bonds in gypsum and wide peak suggestive of disordered graphite from decayed organismal remains. (D) Biotic signature from hairy blob in bedded halite from Permian Opeche Shale, North Dakota; modified from Benison et al. (2008a). (E) Beta carotene in fluid inclusion in bedded halite from Lake Magic, Western Australia; modified from Conner and Benison (2013).
Laser Raman spectroscopy was conducted on organic suspects observed during petrographic analyses (Figure 3). When exposed to the laser, some suspect microorganisms were highly fluorescent and, as a result, their Raman spectra were swamped by the fluorescence. Other suspect prokaryotes, eukaryotes, transparent sheets, and hairy blobs yielded Raman peaks characteristic for disordered graphite (Figure 3D). Some pink and orange fluorescent blobs within fluid inclusions have Raman peaks matching carotenoid compounds, most commonly beta-carotene (Figure 3E). Other suspect organics had peaks characteristic of long-chain hydrocarbon waxes (Figure 3B; Karmanocky and Benison, 2016).
Discussion
Modern and Ancient Halite and Gypsum as Microfossil Hosts
Results show that halite and gypsum both trap microorganisms and organic compounds as solid inclusions and within fluid inclusions. Location of organic material along growth bands and within primary fluid inclusions in halite chevron and cumulate crystals and in gypsum bottom-growth crystals indicates that organics existed in lake waters during deposition. Organics trapped as solid inclusions and within isolated fluid inclusions in displacive halite suggests organics existed within shallow saline groundwaters. For this study, only acid-precipitated halite and gypsum samples were studied. For this reason, the microorganisms and organic compounds either lived in or were transported into acid brine waters.
Microorganisms and organic compounds were found in both modern and ancient samples. In halite, the same abundances were found, regardless of the age of the sample. Appearances of these organics under plane transmitted light were comparable between modern and Permian halite, as were laser Raman spectra. However, fluorescent response to UV-vis light was somewhat fainter in most Permian samples, perhaps indicating that these older organics have decayed. Bedded halite itself can remain unaltered for billions of years, as long as it has remained in a subsurface depth range that keeps it free from dissolution or high heat and pressure (Benison et al., 2015). Therefore, halite makes an excellent material for trapping and preserving organic material over long periods of geologic time.
There are differences in gypsum samples as microfossil hosts, depending upon its age. Modern gypsum contains similar petrographic and Raman spectroscopic characteristics to modern and Permian halite. Permian gypsum, in contrast, provides challenging in examination for preserved microorganisms and organic compounds. The Permian gypsum observed in this study is highly altered. Permian bedded gypsum can be recognized as having formed as bottom-growth crystals or cumulate crystals. However, no internal structures, such as growth bands, remain. Some Permian gypsum crystals have undergone partial dissolution and filling with anhydrite and halite cement. Other Permian bedded gypsum crystals exist as replacement anhydrite and gypsum that likely resulted from repeated dehydration and hydration through time. Because no original primary fluid inclusions in the Permian bottom-growth gypsum remains, we did not further investigate Permian gypsum.
Biotic vs. Abiotic Signatures
Salt minerals trap both biotic and abiotic materials. Here, “biotic” is assigned to microorganisms, their remains, or organic compounds produced by microorganisms, such as beta-carotene, and “abiotic” refers to inorganic hydrocarbons and minerals. To distinguish between biotic and abiotic materials, critical consideration must be given to suspect organic material. Plane transmitted light microscopy can be used to document appearance, including size (down to ~ 1 micron), morphology, color, and texture, of suspect microorganisms and organic compounds. Appearances of unknowns in halite and gypsum by transmitted light microscopy can rule out most inorganic crystals by shape and can support identification of some prokaryotic and eukaryotic cells. However, some abiotic solids, such as some minerals and some hydrocarbons, may look similar to some biotic solids when viewed with transmitted light microscopy. Under polarized light, some inorganic crystals can be distinguished by their birefringence; but solids smaller than ~10 microns may have too weak an optical response to crossed polarized light. Comparison of unknown solids in halite and gypsum to high-resolution images of known microorganisms should be considered a necessary step in the evaluation of plane transmitted light images of suspect microorganisms at various life stages and under various environmental conditions, such as starvation-survival miniaturization at times of desiccation (e.g., Morita, 1982; Oren, 2005; Schubert et al., 2009b). For example, size, shape, color, and dimpled texture of ~5–10 micron yellow spheres in fluid inclusions in halite and gypsum from this study appear similar to images of spherical cells of the alga Dunaliella from various modern ephemeral saline lakes (J. Polle, personal communication).
UV-vis microscopy detects fluorescent response, seen by brightness and colors, due to carbon-carbon or hydrogen-carbon bonds (e.g., Mormile and Storrie-Lombardi, 2005). In this study, both biotic and some abiotic materials fluoresced. Color aided identification of suspect microorganisms and organic compounds. Prokaryotes fluoresced pale green and alga fluoresced pale blue. Carotinoids and long-chain hydrocarbons fluoresced pink, orange, yellow, or white (Conner and Benison, 2013; Winters et al., 2013). Simple liquid hydrocarbons, mostly observed in secondary fluid inclusions in halite from the Permian Opeche Shale at depths > ~2,255 m (7,400 feet), fluoresced bright blue. Some suspect organic material, such as the larger hairy blobs, were black and opaque in thin chips of halite and gypsum, and, thus, did not show a fluorescent response to UV-vis light. In summary, fluorescence to UV-vis light was only seen in materials that were considered by plane light microscopy to be microorganisms or organic compounds. But, as shown in the case of hairy blobs, not all organic suspects responded to UV-vis light. The abiotic compounds that fluoresced, such as solid long-chain hydrocarbons and liquid hydrocarbons, had different fluorescent colors and brightnesses than the cellular material.
Laser Raman spectroscopy is an excellent method for chemically distinguishing between biotic and abiotic materials at the microscopic scale. Raman peaks related to covalent bonds, so it can be used to describe chemical composition of solids, liquids, and gases as small as ~1 micron in size. In this study, a Raman spectra for disordered graphite was observed for biotic suspects, including prokaryotic cells, alga, and hairy blobs (e.g., Ferrari and Robertson, 2000, 2001; Pasteris and Wopenka, 2003). Organic compounds, such as beta-carotene, and minerals, such as gypsum, were confirmed by matching them to known Raman spectra (e.g., Winters et al., 2013; Karmanocky and Benison, 2016).
Teaming transmitted light petrography, UV-vis petrography, and laser Raman spectroscopy, at high resolution (micron scale) is an optimal approach for recognition of biosignatures in chemical sediments. These methods do not result in unambiguous identification of microorganisms at genus and species level. However, the combination of these methods results in sufficient evidence to assign biotic vs. abiotic origin to unknown materials.
Implications and Recommendations for Mars
This study has direct implications for the search for life on Mars because it focuses on minerals found on Mars from similar ephemeral acid saline systems. The halite and gypsum investigated here is closely associated with iron oxides, jarosite, alunite, clay minerals, and opalline silica, making the mineralogical context similar to that of some Mars strata. Due to their rapid growth in saline surface waters, halite, and gypsum have been shown to be good repositories for environmental components, including microorganisms (e.g., Norton and Grant, 1988; McGenity et al., 2000; Adamski et al., 2006; Schubert et al., 2009a,b, 2010a,b; Lowenstein et al., 2011). The use of petrography and laser Raman spectroscopy on the same samples should serve as a foundational approach for the search for any remnant biotic material in chemical sediments on Mars. These methods could be conducted remotely in situ by future Mars rovers. In addition, these methods could be easily conducted on Martian rock and sediment samples returned to Earth. Besides recognition of any Martian biotic materials, combined high-resolution petrography and laser Raman spectroscopy would be invaluable as a way to target materials for further biological study to describe and identify specific biomarkers, such as lipids, fatty acids, and DNA.
No modifications to the methods described in this study would be necessary to conduct petrography and laser Raman spectroscopy on returned Martian samples. However, some relatively modest engineering modifications of these analyses on Mars would be warranted. Table 2 describes these requirements. The Mars 2020 rover will be equipped with high-resolution, high magnification cameras and laser Raman spectrometers. The greatest rover modifications needed for analyses of the interior of halite and gypsum crystals would involve preparation of the samples. Sample holders would need to grab and hold steady mm-cm-scale crystals. A precision scalpel (or a diamond wire cutting mechanism) would need to be designed to slice the crystals thin enough to allow transmitted light. In addition, transmitted plane light and UV-vis light at magnifications up to 2,000x would be optimal.
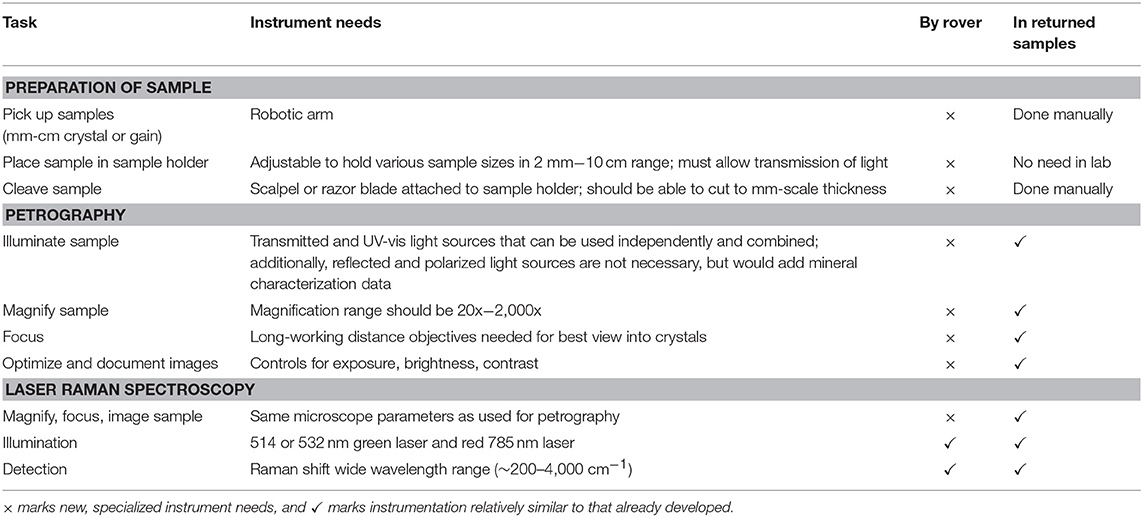
Table 2. Anticipated requirements for searching for biogenic signatures in Martian halite, gypsum, and most other chemical sediments, by rover and in returned samples.
An investigation of the interior of Martian chemical sediments is a necessary part of the search for life on Mars. As a first step, careful selection of samples should be made by evaporite sedimentologists. These samples would include bedded halite, gypsum, and any other minerals interpreted to have been formed by chemical precipitation, as well as detrital rocks and concretions that may contain reworked chemical sediments. Petrography of these samples at high-resolution and high-magnification by transmitted and UV-vis light is needed to target suspect microorganisms and organic compounds. Laser Raman spectroscopy of these targets can confirm chemical signatures to further distinguish true fossils, organic compounds, and minerals. Until such petrographic and chemical investigations are made on the interiors of Martian chemical sediments, the search for life on Mars will remain incomplete.
Conclusions
Study of Mars-analog halite and gypsum from extremely acid, saline, and chemically complex lakes has shown that microorganisms and organic compounds are entrapped within fluid inclusions and as solid inclusions during growth of these chemical sediments in the depositional environment. Similar features documented in modern, Pleistocene, and Permian samples show that biosignatures can be preserved well for long periods of geologic time; unaltered microfossils have been found in fluid inclusions in gypsum that is >10,000 years old; in halite, microfossils can remain unchanged within fluid inclusions and as solid inclusions for hundreds of millions of years. Microscopy at high magnification can reveal physical appearance and UV fluorescent response that allows for characterization of biological material and organic compounds. Laser Raman spectroscopy is an effective tool for chemical identification of organic materials as small as 1 micron in size in both halite and gypsum.
Observations of the interior of Martian chemical sediments has not yet been conducted. Guidelines for planning such a study in situ on Mars by rovers and in returned Martian samples focuses on paired plane transmitted light microscopy, UV-vis microscopy, and laser Raman spectroscopy. The search for life on Mars will not be complete until these methods have been attempted on Martian chemical sediments.
Author Contributions
KB was responsible for doing all the tasks concerning the development of the work, conceptualized the idea and goals of the study, performed literature search and revision, and wrote the manuscript.
Funding
Various parts of this work were funded by NASA Exobiology research grant 80NSSC18K1286, National Science Foundation grants EAR-0902250, EAR-1317138, and MRI-0821152, a National Geographic Committee on Research Grant, and West Virginia University.
Conflict of Interest Statement
The author declares that the research was conducted in the absence of any commercial or financial relationships that could be construed as a potential conflict of interest.
Acknowledgments
Anna Sofia Andeskie, Robert C. Burruss, Amber Conner, John Hallsworth, Elliot Jagniecki, Sarah S. Johnson, Francis Karmanocky, James Lamsdell, Tim K. Lowenstein, Melanie R. Mormile, Juergen Polle, Brian Schubert, Michael Storrie-Lombardi, and Reed Wicander are thanked for discussions about extremophiles and methods for their recognition in halite and gypsum and their fluid inclusions. I-Ming Chou, Jill Pasteris, Mary Teklenburg, and Bridget Wopenka are acknowledged for their assistance with Raman spectroscopy. T. James Reynolds is thanked for many years of logistical support with microscopy.
References
Adamski, J. C., Roberts, J. A., and Goldstein, R. H. (2006). Entrapment of bacteria in fluid inclusions in laboratory-grown halite. Astrobiology 6, 552–562. doi: 10.1089/ast.2006.6.552
Benison, K. C. (2006). A Martian analog in Kansas: comparing Martian strata with permian acid saline lake deposits. Geology 34, 385–388. doi: 10.1130/G22176.1
Benison, K. C. (2013). Acid saline fluid inclusions: examples from modern and permian extreme lake systems. Geofluids 13, 579–593. doi: 10.1111/gfl.12053
Benison, K. C. (2017). Gypsum gravel devils in Chile: movement of largest natural gains by wind? Geology 45, 423–426. doi: 10.1130/G38901.1
Benison, K. C. (2019). The physical and chemical sedimentology of two high-altitude acid salars in Chile: sedimentary processes in an extreme environment. J. Sediment. Res. 89, 147–167. doi: 10.2110/jsr.2019.9
Benison, K. C., and Bowen, B. B. (2006). Acid saline lake systems give clues about past environments and the search for life on Mars. Icarus 183, 225–229. doi: 10.1016/j.icarus.2006.02.018
Benison, K. C., and Bowen, B. B. (2015). The evolution of end-member continental waters: the origin of acidity in southern Western Australia. GSA Today 25, 4–10. doi: 10.1130/GSATG231A.1
Benison, K. C., Bowen, B. B., Haagsma, A. J., Oboh-Ikuenobe, F. E., Sanchez Botero, C., and Story, S. (2011). “Multiple paleoclimate proxies from eocene – holocene lake, eolian, and paleosol facies,” in Western Australian Cores: Preliminary Results: Abstracts with Programs, Geological Society of America Annual Meeting (Minneapolis, MN).
Benison, K. C., Bowen, B. B., Oboh-Ikuenobe, F. E., Jagniecki, E. A., LaClair, D. A., Story, S. L., et al. (2007). Sedimentology of acid saline lakes in southern Western Australia: newly described processes and products of an extreme environment. J. Sediment. Res. 77, 366–388. doi: 10.2110/jsr.2007.038
Benison, K. C., and Goldstein, R. H. (1999). Permian paleoclimate data from fluid inclusions in halite. Chem. Geol. 154, 113–132. doi: 10.1016/S0009-2541(98)00127-2
Benison, K. C., and Goldstein, R. H. (2000). Sedimentology of ancient saline pans: an example from the permian opeche shale, Williston basin, north Dakota. J. Sediment. Res. 70, 159–169. doi: 10.1306/2DC40907-0E47-11D7-8643000102C1865D
Benison, K. C., and Goldstein, R. H. (2001). Evaporites and siliciclastics of the permian nippewalla group of Kansas, U.S.A: a case for non-marine deposition of saline lakes and saline pans. Sedimentology 48, 165–188. doi: 10.1046/j.1365-3091.2001.00362.x
Benison, K. C., Goldstein, R. H., Wopenka, B., Burruss, R. C., and Pasteris, J. D. (1998). Extremely acid permian lakes and groundwaters in North America. Nature 392, 911–914. doi: 10.1038/31917
Benison, K. C., Jagniecki, E. A., Edwards, T. B., Mormile, M. C., and Storrie-Lombardi, M. C. (2008a). “Hairy blobs”: microbial suspects from modern and ancient ephemeral acid saline evaporites. Astrobiology 8, 807–821. doi: 10.1089/ast.2006.0034
Benison, K. C., Johnson, S. S., Mormile, M. R., and Karmanocky, F. J. III (2016). Preservation of Biosignatures in Halite and Gypsum From Mars-analog Acid-brine Lakes and Associated Eolian Sediments. Denver, CO: Abstracts with Program, Geological Society of America Annual Meeting.
Benison, K. C., and Karmanocky, F. J. III (2014). Could microorganisms be preserved in Mars gypsum? insights from terrestrial examples. Geology 42, 615–618. doi: 10.1130/G35542.1
Benison, K. C., LaClair, D., and Walker, J. (2008b). Physical sedimentology experiments with sulfuric acid solutions: implications for Mars? Earth Planet Sci. Lett. 270, 330–337. doi: 10.1016/j.epsl.2008.03.036
Benison, K. C., and LaClair, D. A. (2003). Modern and ancient extremely acid saline deposits: terrestrial analogs for Martian environments? Astrobiology 3, 609–618. doi: 10.1089/153110703322610690
Benison, K. C., Zambito, J. J., and Knapp, J. P. (2015). Contrasting siliciclastic-evaporite strata in subsurface and outcrop: an example from the Permian Nippewalla Group of Kansas, U.S.A: J. Sediment. Res. 85, 626–645. doi: 10.2110/jsr.2015.43
Benison, K. C., Zambito, J. J., Soreghan, G. S., Soreghan, M. J., Foster, T. M., and Kane, M. J. (2013). “Permian red beds and evaporites of the Amoco Rebecca K. Bounds core, Greeley County, Kansas: implications for science and industry,” in Mid-Continent Core Workshop: From Source to Reservoir to Seal, Mid-Continent Section American Association of Petroleum Geologists, Kansas Geological Survey and Kansas Geological Society, eds M. K. Dubois, W. L. Watney, and Tollefson (Wichita), 9–14.
Bibring, J.-P., Langevin, Y., Mustard, J. F., Poulet, F., Arvidson, R., Gendrin, A., et al. (2006). Global mineralogical and aqueous mars history derived from OMEGA/Mars express data. Science 312, 400–404. doi: 10.1126/science.1122659
Blamey, N. J. F., Brand, U., Parnell, J., Spear, N., Lecuyer, C., Benison, K. C., et al. (2016). Paradigm shift in determining neoproterozoic atmospheric oxygen. Geology 44, 651–654. doi: 10.1130/G37937.1
Bodnar, R. J. (1999). “Fluid inclusions in ALH84001 and other Martian meteorites: evidence for volatiles on Mars,” 30th Annual Lunar and Planetary Science Conference (Houston, TX), 1222.
Bowen, B. B., and Benison, K. C. (2009). Geochemical characteristics of naturally acid and alkaline saline lakes in southern Western Australia. Appl. Geochem. 24, 268–284. doi: 10.1016/j.apgeochem.2008.11.013
Bowen, B. B., Benison, K. C., Oboh-Ikuenobe, F. E., Story, S., and Mormile, M. R. (2008). Active hematite concretion formation in modern acid saline lake sediments, Lake Brown, Western Australia. Earth Planet. Sci. Lett. 288, 52–63. doi: 10.1016/j.epsl.2007.12.023
Bowen, B. B., Benison, K. C., and Story, S. (2012). “Early diagenesis by modern acid brines in Western Australia and implications for the history of sedimentary modification on Mars,” in SEPM Special Publication: Sedimentary Geology of Mars, eds J. Grotzinger, and R. Milliken (Boulder, CO, 229–252.
Bowers, K. J., Mesbah, N. M., and Wiegel, J. (2009). Biodiversity of poly-extremophilic bacteria: does combining the extremes of high salt, alkaline pH and elevated temperature approach a physic-chemical boundary for life? Saline Syst. 5:9. doi: 10.1186/1746-1448-5-9
Burns, R. G. (1987). Ferric sulfates on mars. J. Geophys. Res. 92, E570–E574. doi: 10.1029/JB092iB04p0E570
Burns, R. G. (1994). “Schwertmannite on mars: deposition of this ferric oxyhydroxysulfate mineral in acidic saline meltwaters,” Abstracts, Lunar and Planetary Science Conference (Houston, TX), 203.
Chan, Q. H. S., Zolensky, M. E., Kebukawa, Y., Fries, M., Ito, M., Steele, A., et al. (2018). Organic matter in extraterrestrial water-bearing salt crystals. Science 4:3521. doi: 10.1126/sciadv.aao3521
Clark, B. C. (1994). Acid waters as agents of change on a cold early Mars. Proceedings of the Lunar and Planetary Science Conference XXV (Houston, TX), 263–264.
Clark, B. C., Morris, R. V., McLennan, S. M., Gellert, R., Jolliff, B. J., Knoll, A. H., et al. (2005). Chemistry and mineralogy of outcrops at Meridiani Planum. Earth Planet. Sci. Lett. 240, 73–94. doi: 10.1016/j.epsl.2005.09.040
Clark, B. C., and Van Hart, D. C. (1981). The salts of mars. Icarus 45, 370–378. doi: 10.1016/0019-1035(81)90041-5
Cockell, C. S., Catling, D. C., Davis, W. L., Snook, K., Kepner, R. L., Le, P., et al. (2000). The ultraviolet environment of mars: biological implications past, present, future. Icarus 146, 343–359. doi: 10.1006/icar.2000.6393
Conner, A. J., and Benison, K. C. (2013). Acidophilic, halophilic microorganisms in fluid inclusions in halite from Lake Magic, Western Australia. Astrobiology 9, 850–860. doi: 10.1089/ast.2012.0956
Davis-Belmar, C. S., Pinto, E., Demergasso, C., and Rautenbach, G. (2013). Proteo and actinobacteria diversity at a sulfide, salt and acid-rich lake in the north of Chile. Adv. Mater. Res. 825, 37–41. doi: 10.4028/www.scientific.net/AMR.825.37
Dela Pierre, F., Natalicchio, M., Ferrando, S., Giusttto, R., Birgel, D., Carnevale, G., et al. (2015). Are the large filamentous microfossils preserved in Messinian gypsum colorless sulfide-oxidizing bacteria? Geology 43, 855–858. doi: 10.1130/G37018.1
Dellwig, L. F. (1955). Origin of the salina salt of Michigan. J. Sediment. Petrol. 25, 83–110. doi: 10.1306/D4269819-2B26-11D7-8648000102C1865D
Demergasso, C., Dorador, C., Meneses, D., Blamey, J., Cabrol, N., Escudero, L., et al. (2010). Prokaryotic diversity pattern in high-altitude ecosystems of the Chilean altiplano. J. Geophys. Res. Biogeosci. 115, 2156–2202. doi: 10.1029/2008JG000836
Dombrowski, H. (1963). Bacteria from Paleozoic salt deposits. Ann. N. Y. Acad. Sci. 108, 453–460. doi: 10.1111/j.1749-6632.1963.tb13400.x
Dombrowski, H. J. (1966). “Geological problems in the question of living bacteria in Paleozoic salt deposits,” in Second Symposium on Salt. Northern Ohio Geol. Soc., ed J. Rau (Cleveland, OH), 215–220.
Ehlmann, B. L., Swayze, G. A., Milliken, R. E., Wray, J. J., Mustard, J. F., Breit, G. N., et al. (2016). Discovery of alunite in cross crater, terra sirenum, mars: evidence for precipitation from acidic, sulfurous waters. Am. Mineralogist 101, 1527–1542. doi: 10.2138/am-2016-5574
Eigenbrode, J. L., Summons, R. E., Steele, A., Freissinet, C., Millan, M., Navarro-González, R., et al. (2018). Organic matter preserved in 3-billion-year-old mudstones at Gale crater, Mars. Science 360, 1096–1101. doi: 10.1126/science.aas9185
Escudero, L. V., Bijman, J., Chong, G., Pueyo, J. J., and Demergasso, C. (2013). Geochemistry and microbiology in an acidic, high altitude (4,000 m) salt flat, high Andes, northern Chile. Adv. Mater. Res. 825, 28–32. doi: 10.4028/www.scientific.net/AMR.825.28
Escudero, L. V., Oetiker, N., Gallardo, K., Tebes-Cayo, M., Guajardo, M., Nunez, C., et al. (2018). A thiotrophic microbial community in an acidic brine lake in Northern Chile. Antoine van Leeuwenhoek 111, 1572–1589. doi: 10.1007/s10482-018-1149-y
Farmer, J. D., Bell, J. F. III., Benison, K. C., Boynton, W. V., Cady, S. L., Ferris, F. G., MacPherson, D., et al. (2009). Assessment of Planetary Protection Requirements for Mars Sample Return Missions, Space Studies Board, National Research Council. Washington, DC: National Academy Press.
Farrand, W. H., Glotch, T. D., Rice, J. W. Jr., Hurowitz, J. A., and Swayze, G.A. (2009). Discovery of jarosite within the Mawrth Hills region of MarsL Implications for the geologic history of the region. Icarus 204, 478–488. doi: 10.1016/j.icarus.2009.07.014
Fendrihan, S., Legat, A., Pfaffenhuemer, M., Gruber, C., Weidler, G., Gerbl, F., et al. (2006). Extremely halophilic archaea and the issue of long-term microbial survival. Rev Environ Sci Biotechnol. 5, 203–218. doi: 10.1007/s11157-006-0007-y
Fendrihan, S., and Stan-Lotter, H. (2004). “Survival of halobacteria in fluid inclusions as a model of possible biotic survival in Martian halite,” in Mars and Planetary Science and Technology, eds H. Teodorescu, and H. Griebel (Iasi: Performantica Press, 9–18.
Ferrari, A. C., and Robertson, J. (2000). Interpretation of Raman spectra of disordered and amorphous carbon. Phys. Rev. B. 61, 14095–14107. doi: 10.1103/PhysRevB.61.14095
Ferrari, S. C., and Robertson, J. (2001). Resonant Raman spectroscopy of disordered, amorphous, and diamondlike carbon. Phys. Rev. B. 64, 75414-1–75414-13. doi: 10.1103/PhysRevB.64.075414
Fish, S. A., Shepherd, T. J., McGenity, T. J., and Grant, W. D. (2002). Recovery of 16S ribosomal RNA gene fragments from ancient halite. Nature 417, 432–436. doi: 10.1038/417432a
Gendrin, A., Mangold, N., Bibring, J.-P., Langevin, Y., Gondet, B., Poulet, F., et al. (2005). Sulfates in martin layered terrains: the OMEGA/Mars express view. Sci. Express 307:5715. doi: 10.1126/science.1109087
Glotch, T. D., Bandfield, J. L., Tornabene, L. L., et al. (2010). Distribution and formation of chlorides and phyllosilicates in Terra Sirenum, Mars. Geophys. Res. Lett. 37:L16202. doi: 10.1029/2010GL044557
Goldstein, R. H. (2001). Clues from fluid inclusions. Science 294, 1009–1011. doi: 10.1126/science.1066322
Goldstein, R. H., and Reynolds, T. J. (1994). Systematics of Fluid Inclusions in Diagenetic Minerals: SEPM Short Course Notes (Tulsa, OK: SEPM Society for Sedimentary Geology).
Gomez, F., Mateo-Marti, E., Prieto-Ballesteros, O., Martin-Gago, J., and Amils, R. (2010). Protection of chemolithoautotrophic bacteria exposed to simulated Mars environmental conditions. Icarus 209, 482–487. doi: 10.1016/j.icarus.2010.05.027
Grant, W. D. (2004). Life at low water activity: Philosophical Transactions of the Royal Society of London, B. Biol. Sci. 359, 1249–1267. doi: 10.1098/rstb.2004.1502
Grant, W. D., Gemmell, R. T., and McGenity, T. J. (1998). Halobacteria: the evidence for longevity. Extremophiles 2, 279–287. doi: 10.1007/s007920050070
Grotzinger, J. P., Arvidson, R. E., Bell, J. F. III., Calvin, W., Clark, B. C., Fike, D. A., et al. (2005). Stratigraphy and sedimentology of a dry to wet eolian depositional system, burns formation, meridiani planum, mars. Earth Planet. Sci. Lett. 240, 11–72. doi: 10.1016/j.epsl.2005.09.039
Grotzinger, J. P., Gupta, S., Malin, M. C., Rubin, D. M., Schieber, J., Siebach, K., et al. (2015). Deposition, exhumation, and paleoclimate of an ancient lake deposit, gale crater, mars. Science 350, 7575-1–7575-12. doi: 10.1126/science.aac7575
Jaakkola, S. T., Pfeiffer, F., Ravantti, J. J., Guo, Q., Liu, Y., Chen, X., et al. (2016). The complete genome of a viable archaeum isolated from 123-million-year-old rock salt. Environ. Microbiol. 18, 565–579. doi: 10.1111/1462-2920.13130
Jagniecki, E. A., and Benison, K. C. (2010). Criteria for the recognition of acid-precipitated halite. Sedimentology 57, 273–292. doi: 10.1111/j.1365-3091.2009.01112.x
Johnson, S. S., Chevrette, M. G., Ehlmann, B. L., and Benison, K. C. (2015). Insights from the metagenome of an acid salt lake: the role of biology in an extreme depositional environment. PLoS ONE 10:e0122869. doi: 10.1371/journal.pone.0122869
Jones, C. M., Allen, E. E., Giska, J. R., Welch, S. A., Kirste, D., and Banfield, J. F. (2006). Iron formations at lake Tyrrell, Victoria, Australia: microbially-mediated redox chemistry. goldschmidt conference abstracts. Geochimica et Cosmochimica Acta 70:A297. doi: 10.1016/j.gca.2006.06.603
Kargel, J. S. (2004). Proof for water, hints of life? Science 306, 1689–1691. doi: 10.1126/science.1105533
Karmanocky, F. J. III., and Benison, K.C. (2016). A fluid inclusion record of hydrothermal pulses in acid Salar Ignorado gypsum, northern Chile. Geofluids 16, 490–506. doi: 10.1111/gfl.12171
Klingelhöfer, G., Morris, R. V., Bernhardt, B., Schröder, C., Rodionov, D. S., de Souza, P. A. Jr., Yen, A., et al. (2004). Jarosite and hematite at meridiani planum from opportunity's Mossbauer spectrometer. Science 306, 1740–1745. doi: 10.1126/science.1104653
Lowenstein, T. K., Schubert, B. A., and Timofeef, M. N. (2011). Microbial communities in fluid inclusions and long-term survival in halite. GSA Today 21, 4–9. doi: 10.1130/GSATG81A.1
Lowenstein, T. K., and Spencer, R. J. (1990). Syndepositional origin of potash evaporites: petrographic and fluid inclusion evidence. Am. J. Sci. 290, 1–42. doi: 10.2475/ajs.290.1.1
Lowenstein, T. K., Timofeeff, M. N., Brennan, S. T., Hardie, L. A., and Demicco, R. V. (2001). Oscillations in phanerozoic seawater chemistry: evidence from fluid inclusions. Science 294, 1086–1088. doi: 10.1126/science.1064280
Lu, S., Peiffer, S., Lazar, C. S., Oldham, C., Neu, T. R., Ciobota, V., et al. (2016). Extremophile microbiomes in acidic and hypersaline river sediments of Western Australia. Environ. Microbiol. Rep. 8, 56–67. doi: 10.1111/1758-2229.12351
Madden, M. E. E., Bodnar, R. J., and Rimstidt, J. D. (2004). Jarosite as geochemical indicator of water-limited chemical weathering on Mars. Nature 431, 821–823. doi: 10.1038/nature02971
McGenity, T. J., Gemmell, R. T., Grant, W. D., and Stan-Lotter, H. (2000). Origins of halophilic microorganisms in ancient salt deposits. Environ. Microbiol. 2, 243–250. doi: 10.1046/j.1462-2920.2000.00105.x
McKay, D. S., Gibson, E. K., Thomas-Kerpta, K. L., Vali, H., Romanek, C. S., Clemett, S. J., et al. (1996). Search for past life on mars: possible relic biogenic activity in Martian meteorite ALH84001. Science 273, 924–930. doi: 10.1126/science.273.5277.924
Metz, J. M., Grotzinger, J. P., Rubin, D. M., Lewis, K. W., Squyres, S. W., and Bell, J. F. III (2009). Sulfate-rich eolian and wet interdune deposits, erebus crater, meridiani planum, mars. J. Sediment. Res. 79, 247–264. doi: 10.2110/jsr.2009.033
Mikucki, J. A., Lyons, W. B., Hawes, I., Lanoil, B. D., and Doran, P. T. (2010). “Saline lakes and ponds in the McMurdo Dry Valleys: ecological analogs to Martian paleolake environments,” in Life in Antarctic Deserts and Other Cold Dry Environments: Astrobiological Analogues, eds P. T. Doran, W. B. Lyons, and D. McKnight (Cambridge, UK: Cambridge University Press, 160–194.
Morita, R. Y. (1982). Starvation-survival of heterotrophs in the marine environment. Adv. Microbial Ecol. 6, 171–198. doi: 10.1007/978-1-4615-8318-9_5
Mormile, M. R., Biesen, M. A., Gutierrez, M. C., Ventosa, A., Pavlovich, J. B., Onstott, T. C., et al. (2003). Isolation of halobacterium salinarum retrieved directly from halite brine inclusions. Environ. Microbiol. 5, 1094–1102. doi: 10.1046/j.1462-2920.2003.00509.x
Mormile, M. R., Hong, B.-Y., and Benison, K. C. (2009). Molecular analysis of the microbial communities of Mars-analog lakes in Western Australia. Astrobiology 9, 919–930. doi: 10.1089/ast.2008.0293
Mormile, M. R., and Storrie-Lombardi, M. C. (2005). “The use of ultraviolet excitation of native fluorescence for identifying biomarkers in halite crystals,” in Astrobiology and Planetary Missions, Proceedings of SPIE (The International Society of Optical Engineering), eds R. B. Hoover, G. V. Levin, A. Y. Rozanov, and G. R. Gladstone (Bellingham, WA), 5906.
Norton, C. F., and Grant, W. D. (1988). Survival of halobacteria within fluid inclusions in salt crystals. J. General Microbiol. 134, 1365–1373. doi: 10.1099/00221287-134-5-1365
Oren, A. (2005). A hundred years of Dunaliella research, 1905-2005. Saline Syst. 1:2. doi: 10.1186/1746-1448-1-2
Osterloo, M. M., Hamilton, V. E., Bandfield, J. L., Glotch, T. D., Baldridge, A. M., Christensen, P. R., et al. (2008). Chloride-bearing materials in the Southern highlands of mars. Science 319, 1651–1654. doi: 10.1126/science.1150690
Parnell, J., and Baron, M. (2004). The preservation of fluid inclusions in diverse surface precipitates: the potential for sampling palaeo-water from surface deposits on Mars. Int. J. Astrobiol. 3, 21–30. doi: 10.1017/S1473550404001867
Pasteris, J. D., and Wopenka, B. (2003). Necessary, but not sufficient: Raman identification of disordered carbon as a signature of ancient life. Astrobiology 3, 727–738. doi: 10.1089/153110703322736051
Phoenix, V. R., Bennet, P. C., Engel, A. S., Tyler, S. W., and Ferris, F. G. (2006). Chilean high-altitude hot spring sinters: a model system for UV screening mechanism by early Precambrian cyanobacteria. Geobology 4, 15–28. doi: 10.1111/j.1472-4669.2006.00063.x
Podell, S., Emerson, J. B., Jones, C. M., Ugalde, J. A., Welch, S., Heidelberg, K. B., et al. (2014). Seasonal fluctuations in ionic concentrations drive microbial succession in a hypersaline lake community. ISME J. 8, 979–990. doi: 10.1038/ismej.2013.221
Quatrini, R., Escudero, L. V., Moya-Beltrán, A., Galleguillos, P. A., Issotta, F., Acosta, M., et al. (2017). Draft genome sequence of Acidithiobacillus thiooxidans CLST isolated from the acidic hypersaline Gorbea salt flat in northern Chile. Stand. Genomic Sci. 12, 1–8. doi: 10.1186/s40793-017-0305-8
Reiser, R., and Tasch, P. (1960). Investigation of the viability of osmophilic bacteria of great age. Trans. Kans. Acad. Sci. 63, 31–34. doi: 10.2307/3626919
Risacher, F., Alonso, H., and Salazar, C. (2002). Hydrochemistry of two adjacent acid saline lakes in the Andes of northern Chile. Chem. Geol. 187, 39–57. doi: 10.1016/S0009-2541(02)00021-9
Roberts, S. M., and Spencer, R. J. (1995). Paleotemperatures preserved in fluid inclusions in halite. Geochim. Cosmchim. Acta 59, 3929–3942. doi: 10.1016/0016-7037(95)00253-V
Sankaranarayanan, K., Lowenstein, T. K., Timofeeff, M. N., Schubert, B. A., and Lum, J. K. (2014). Characterization of ancient DNA supports long-term survival of haloarchaea. Astrobiology 4, 553–560. doi: 10.1089/ast.2014.1173
Schluter, T., Kohring, R., and Gregor, H.-J. (2003). Dragonflies preserved in transparent gypsum crystals from the Messinian (Upper Miocene) of Alba, northern Italy. Acta Zoologica Cracoviensia 46, 373–379.
Schopf, J. W., Farmer, J. D., Foster, I. S., Kudryavtsev, A. B., Gallardo, V. A., and Espinoza, C. (2012). Gypsum-permineralized microfossils and their relevance to the search for life on mars. Astrobiology 12, 1–15. doi: 10.1089/ast.2012.0827
Schubert, B. A., Lowenstein, T. K., and Timofeeff, M. N. (2009b). Microscopic identification of prokaryotes in modern and ancient halite, Saline Valley and Death Valley, California. Astrobiology 9, 467–482. doi: 10.1089/ast.2008.0282
Schubert, B. A., Lowenstein, T. K., Timofeeff, M. N., and Parker, M. A. (2009a). How can prokaryotes survive in fluid inclusions in halite for 30,000 years? Geology 37, 1059–1062. doi: 10.1130/G30448A.1
Schubert, B. A., Lowenstein, T. K., Timofeeff, M. N., and Parker, M. A. (2010b). Halophilic archaea cultured from ancient halite, death valley, California. Environ. Microbiol. 12, 440–454. doi: 10.1111/j.1462-2920.2009.02086.x
Schubert, B. A., Timofeeff, M. N., Polle, J. E. W., and Lowenstein, T. K. (2010a). Dunaliella cells in fluid inclusions in halite: significance for long-term survival of prokaryotes. Geomicrobiol. J. 27, 61–75. doi: 10.1080/01490450903232207
Squyres, S. W., Grotzinger, J. P., Arvidson, R. E., Bell, J. F., Calvin, W., Cristensen, P. R., et al. (2004). In situ evidence for an ancient aqueous environment at meridiani planum, mars. Science 306, 1709–1714. doi: 10.1126/science.1104559
Stan-Lotter, H., McGenity, T. J., Legat, A., Denner, E. B., Glaser, K., Stetter, K. O., et al. (1999). Very similar strains of Halococcus salifodinae are found in geographically separated permo-triassic salt deposits. Microbiology 145, 3565–3574. doi: 10.1099/00221287-145-12-3565
Story, S. L., Bowen, B. B., Benison, K. C., and Schulze, D. G. (2010). Authigenic phyllosilicates in modern acid saline lake sediments and implications for mars. J. Geophys. Res. Planets 115:E12012. doi: 10.1029/2010JE003687
Tasch, P. (1963). “Fossil content of salt and association evaporites,” Symposium on Salt, 1: 96-102, Northern Ohio Geological Society (Cleveland, OH).
Vreeland, R. H., Jones, J., Monson, A., Rosenzweig, W. D., Lowenstein, T. K., Timofeeff, M., et al. (2007). Isolation of live cretaceous (121-112 million years old) halophilic archaea from primary salt crystals. Geomicrobiol. J. 24, 275–282. doi: 10.1080/01490450701456917
Vreeland, R. H., Rosenzweig, W. D., and Powers, D. W. (2000). Isolation of a 250 million-year-old halotolerant bacterium from a primary salt crystal. Nature 407, 897–900. doi: 10.1038/35038060
Webster, C. R., Mahaffy, P. R., Atreya, S. K., Moores, J. E., Flesch, G. J., Malespin, C., et al. (2018). Background levels of methane in Mars' atmosphere show strong seasonal variations. Science 360, 1093–1096. doi: 10.1126/science.aaq0131
Williams, J. P., and Hallsworth, J. E. (2009). Limits of life in hostile environments: no barriers to biosphere function? Environ. Microbiol. 11, 3292–3308. doi: 10.1111/j.1462-2920.2009.02079.x
Winters, Y. D., Lowenstein, T. K., and Timofeeff, M. N. (2013). Identification of carotenoids in ancient salt from death valley, saline valley, and searles lake, California, using laser Raman spectroscopy. Astrobiology 13, 1065–1080. doi: 10.1089/ast.2012.0952
Wood, A. P., and Kelly, D. P. (1991). Isolation and characterisation of Thiobacillus halophilus sp. nov., a sulphur-oxidising autotrophic eubacterium from a Western Australian hypersaline lake. Arch. Microbiol. 156, 277–280. doi: 10.1007/BF00262998
Zaikova, E., Benison, K. C., Mormile, M. R., and Johnson, S. S. (2018). Microbial communities and their predicted metabolic functions in a desiccating acid salt lake. Extremophiles 22, 367–379. doi: 10.1007/s00792-018-1000-4
Keywords: mars, fluid inclusions, gypsum, halite, extremophiles, acid saline lakes, astrobiology
Citation: Benison KC (2019) How to Search for Life in Martian Chemical Sediments and Their Fluid and Solid Inclusions Using Petrographic and Spectroscopic Methods. Front. Environ. Sci. 7:108. doi: 10.3389/fenvs.2019.00108
Received: 14 October 2018; Accepted: 21 June 2019;
Published: 16 July 2019.
Edited by:
Marjorie A. Chan, The University of Utah, United StatesReviewed by:
Kai Waldemar Finster, Aarhus University, DenmarkJeffrey James Marlow, Harvard University, United States
Copyright © 2019 Benison. This is an open-access article distributed under the terms of the Creative Commons Attribution License (CC BY). The use, distribution or reproduction in other forums is permitted, provided the original author(s) and the copyright owner(s) are credited and that the original publication in this journal is cited, in accordance with accepted academic practice. No use, distribution or reproduction is permitted which does not comply with these terms.
*Correspondence: Kathleen C. Benison, a2NiZW5pc29uQG1haWwud3Z1LmVkdQ==