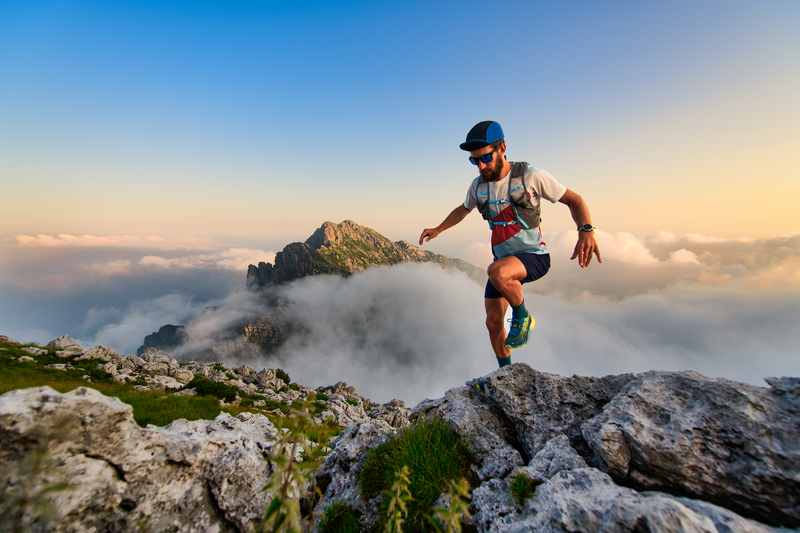
95% of researchers rate our articles as excellent or good
Learn more about the work of our research integrity team to safeguard the quality of each article we publish.
Find out more
ORIGINAL RESEARCH article
Front. Environ. Sci. , 02 July 2019
Sec. Environmental Toxicology
Volume 7 - 2019 | https://doi.org/10.3389/fenvs.2019.00100
Changes in the biochemical composition of primary producers can alter their food quality, influencing their consumers and further propagating through the food web. Gamma (ɤ) radiation is an environmentally important type of ionizing radiation as it can damage macromolecules such as DNA, proteins, and lipids due to its high frequency, short wavelength, and high energy photons. Here, we investigate whether short-term ɤ-radiation changes the biochemical composition of primary producers and if radiation-induced changes affect higher trophic levels. Two phytoplankton species were exposed to two doses of ɤ-radiation and compared to a control. The metabolic profile and total protein content of the algae were measured at five time points within 24 h. Additionally, we measured carbon incorporation rates of Daphnia magna fed with the exposed algae. Gamma radiation had a significant effect on phytoplankton biochemical composition, although these effects were species-specific. The changes in phytoplankton biochemical composition indicate that ɤ-radiation induced the production of reactive oxygen species (ROS). D. magna incorporated more carbon when fed with algae previously exposed to ɤ-radiation; this could be due to radiation-induced changes in nutritional quality, algal anti-grazing defenses, or chemical feeding stimuli.
The biochemical composition, or food quality, of primary producers can affect the productivity of aquatic food webs (Sterner and Hessen, 1994; Vrede et al., 2004; Malzahn et al., 2010). One of the challenges in aquatic ecology is to predict how changes in primary production propagate up the food chain. Food quality can be defined as all attributes of the food that influence edibility as well as nutritional quality (Dickman et al., 2008; Sommer et al., 2012), including the elemental composition, biochemical make-up, particle size, and morphological characteristics of the food item (Sommer et al., 2012). As primary producers synthesize many biochemical compounds, such as essential fatty and amino acids that cannot be synthesized by primary consumers like crustaceous zooplankton, the food quality of primary producers has been shown to be important for reproduction and growth in copepods and cladocerans (Elser and Hassett, 1994).
Exposure to contaminants has been shown to change the biochemical composition of microalgae. In the presence of pesticides, the fatty acid (FA) profile of various microalgae changed to one with fewer polyunsaturated FA (PUFAs), especially eicosapentaenoic acid (EPA) and linoleic acid, but more monounsaturated FA (MUFAs), particularly palmitoleic acid and oleic acid (Filimonova et al., 2016). Exposure to heavy metals resulted in significant biochemical changes in phytoplankton, with a decrease in PUFAs and an increase in saturated fatty acids (SFAs) and MUFAs (Rocchetta et al., 2006; Filimonova et al., 2016). Higher concentrations of PUFAs in phytoplankton have been linked to higher somatic growth and higher fecundity in crustaceous zooplankton (Ahlgren et al., 1990; Lang et al., 2011). Other macromolecules also change when phytoplankton species are exposed to certain contaminants. For instance, exposure to copper and salicylic acid led to the amino acid composition of Scenedesmus quadricauda being altered to include a higher concentration of arginine, histidine, methionine, and proline (Kovacik et al., 2010).
Ecosystems are continuously exposed to ionizing radiation from cosmic rays and naturally occurring radioactive isotopes, which are found in many natural resources, such as igneous rocks and ores (IAEA, 2003). While naturally occurring radionuclides can pose a potential radiological threat locally, the risk of environmental contamination from anthropogenic activities has spread globally due to natural resource exploitation, nuclear weapon production and testing, as well as nuclear power plant operations and accidents (Hu et al., 2010). Gamma (ɤ) radiation is an environmentally important type of ionizing radiation. Due to its high frequency, short wavelength, and high-energy photons it can interact with biological matter and cause ionization and excitation of macromolecules (Vanhoudt et al., 2014). Gamma radiation has been shown to cause DNA damage and induce detrimental effects on growth, reproduction, and morphology (Dallas et al., 2012; Jan et al., 2012; Parisot et al., 2015). These effects can be caused by either direct effects of ɤ-radiation on biomolecules, such as single or double strand DNA breaks, or indirect effects, for example through the production of radiolytic water products, including reactive oxygen species (ROS) (Spitz et al., 2004).
The production of ROS is the most likely effect of ɤ-radiation in organisms, since cells and organisms as a whole consist primarily of water (c. 90%) (Spitz et al., 2004). When the concentration of ROS exceeds the capacity of cellular antioxidants to maintain normal steady state redox potential, the cell is under oxidative stress which, if prolonged, can lead to damaged biomolecules, mutagenesis, and ultimately cell death (Mallick and Mohn, 2000; Choo et al., 2004; Spitz et al., 2004). When a cell is under oxidative stress, the antioxidant defense from ROS scavenging is not effective enough and ROS can readily attack PUFAs in the cell membrane (Mylonas and Kouretas, 1999). This in turn initiates a self-propagating chain reaction that can potentially damage cells or tissues, even if only a few lipids in the cell membrane were oxidized in the first place (Mylonas and Kouretas, 1999). Lipid peroxides can directly induce physiological changes in an organism by collapsing the membrane structure, which reduces membrane fluidity and ion transport (Won et al., 2014a). At the (primary) producer-consumer interface, oxidation of PUFAs can affect the food quality of the primary producer and may therefore indirectly affect the consumer.
While pesticides and heavy metals have been shown to change the biochemical composition of phytoplankton, to our knowledge such effects have not yet been demonstrated as a response to ɤ-radiation exposure. Nascimento and Bradshaw (2016) showed that Daphnia magna fed with the green algae Raphidocelis subcapitata that had been exposed to varying doses of ɤ-radiation incorporated more carbon than D. magna fed with algae that had not been exposed to ɤ-radiation. While this could indicate a change in the food's biochemical composition, no differences in FA composition at the highest exposure (100 Gy) were observed (Nascimento and Bradshaw, 2016). Here, we investigate whether external, short-term ɤ-radiation changes the biochemical composition of two primary producers over time and if radiation-induced changes in the primary producers can propagate to or affect the next trophic level. We exposed two species of phytoplankton—one chlorophyte (R. subcapitata) and one eustigmatophyte (Eustigmatos magnus)—to two doses of external, short-term ɤ-radiation (5 Gy, 25 Gy). We then screened for changes in the metabolic profile and biochemical parameters (total protein content) and compared these to an unexposed control. Based on the phytoplankton exposure and changes in biochemical composition, we chose one time point and ɤ-radiation exposure and fed the exposed and control algae to a primary consumer (D. magna) and measured carbon incorporation in both the primary producers, as a proxy for primary production, and in the consumers, as a measure for grazing rates.
The chlorophyte R. subcapitata and the eustigmatophyte E. magnus (SAG 36.89) were cultured in artificial freshwater enriched with modified Woods Hole (MWC) medium (Guillard and Lorenzen, 1972) in a climate-controlled chamber set at 20°C and 16:8 h light: dark cycle. Two weeks prior to the experiment, batch cultures were set up from the same monoclonal stock culture in triplicates per species and treatment. To keep the cultures in exponential growth, fresh media was supplied every other day. These species were chosen due to their different food qualities for zooplankton species. Eustigmatophyceae, such as E. magnus, are known to be rich in PUFAs and are therefore regarded as high-quality food for zooplankton, while Chlorophyceae, such as R. subcapitata, generally lack PUFAs and are therefore regarded as poorer quality food (Lang et al., 2011). Additionally, R. subcapitata serves as a model species in many ecotoxicology studies and has been used in effect studies of ɤ-radiation and effects of radionuclide exposure (Neves et al., 2015; Nascimento and Bradshaw, 2016; Nascimento et al., 2016); (Gomes et al., 2018).
Raphidocelis subcapitata and E. magnus cultures were exposed to short-term ɤ-radiation using a 6.22 Gy/min 137Cesium source (Gammacell 1,000). Due to the size of the exposure chamber, the cultures were pooled, well-mixed, and then divided into 6 * 500 mL bottles, duplicates of which were exposed to 5 Gy (for 48 s) and 25 Gy (for 4 min), resulting in total doses of 5.0 and 24.9 Gy. The two controls were handled in the same way as the exposed samples and were placed next to the ɤ-radiation wrapped in aluminum foil to simulate the darkness during exposure. These doses were chosen in the lower range at which effects on R. subcapitata have been seen in previous studies by this group (e.g., Nascimento and Bradshaw, 2016). Since no previous data exists for the radio-sensitivity of E. magnus, we used these as fixed dose rates for both algal species. After the exposure, the cultures from each treatment were again pooled and then split into three replicates per dose and time point. Each replicate contained 200 mL of the respective algae culture and 50 mL of fresh modified MWC medium. Destructive samples were taken at five time points (3, 6, 9, 12, and 24 h) after exposure, leading to a total of 45 experimental units per species. At each time point, the 3 replicates per treatment were divided into 5 * 50 mL samples, which were then stored in −80°C until further analyses.
Sample preparation, derivatization, and gas chromatography–mass spectrometry (GC-MS) analyses were performed in accordance to Gullberg et al. (2004). In short, 100 μl of extraction buffer (20/20/60 v/v chloroform/water/methanol) including internal standards were added to 1–7 mg of freeze-dried algae. The samples were then shaken in a mixer mill at 30 Hz for 3 min with tungsten beads, and afterwards the samples were centrifuged at +4°C, 14 000 rpm, for 10 min. Subsamples of 200 μL of the supernatant were transferred to a micro vial and solvents were evaporated. For the derivatization of the samples 30 μL of methoxyamine were added to the dried samples, which were then shaken for 10 min and left to react at room temperature. After 16 h, 30 μL of MSTFA (N-Methyl-N-(trimethylsilyl) trifluoroacetamide) were added and left to react for another hour. Before the analysis, 30 μL of methyl stearate were added and 1 μL of the derivatized samples was injected into splitless mode by a CTC Combi Pal autosampler (CTC Analytics AG, Switzerland) into an Agilent 6,890 gas chromatograph. For more details of the GC-MS analysis see Supplementary Material and Gullberg et al. (2004).
For the GC-MS data, all non-processed MS-files from the metabolic analysis were exported from the ChromaTOF software in NetCDF format to MATLAB® R2016a (Mathworks, Natick, MA, USA), where all data pre-treatment procedures, such as base-line correction, chromatogram alignment, data compression, and multivariate curve resolution were performed using custom scripts. The extracted mass spectra were identified by comparisons of their retention index and mass spectra with libraries of retention time indices and mass spectra (Strelkov et al., 2005). Mass spectra and retention index comparisons were performed using NIST MS 2.0 software. Annotation of mass spectra were based on reverse and forward searches in the library. Masses and ratio between masses indicative for a derivatized metabolite were especially notified. If the mass spectrum was with highest probability indicative of a metabolite and the retention index between the sample and library for the suggested metabolite was ± 5 (usually <3), the deconvoluted “peak” was annotated as an identification of a metabolite in accordance with the Swedish Metabolomics Center standard practice.
The data were then standardized to the original dry weight and the volume of the subsample analyzed to obtain a relative concentration of each metabolite.
Phytoplankton samples were analyzed for total protein content according to the procedure of Spáčil et al. (2010) and Annadotter et al. (2015) with minor modifications. The samples were dissolved in 80% methanol (80/20 methanol/water, v/v). Cell lysis was achieved by sonication for three 45-s cycles at 70% intensity (Sonopuls, Model HD 20170; Bandelin Electronic, Berlin, Germany). To avoid protein degradation, the samples were kept on ice and allowed to cool between each cycle. Protein concentrations were determined using the Bio-Rad RC/DC kit (Bio-Rad, Sundbyberg, Sweden). The spectrophotometric measurements were performed at 750 nm in mQuant Monochromatic Microplate Spectrophotometer at room temperature (BioTek, Winooski, VT, USA). The total protein content was then calculated using a standard dilution series and standardized to sample dry weight.
14C-incorporation (from 14C-labeled phytoplankton to D. magna) was used as a short-term measure of food assimilation. Twenty-four hours was chosen as the grazing period since it is long enough to obtain measureable C-assimilation values without risking substantial changes to the food quality of the algae cells during the grazing period or loss of 14C through respiration.
The cladoceran D. magna was cultured in COMBO media and fed with radiolabeled R. subcapitata or E. magnus. Based on the results from the microalgae exposure experiment, we exposed R. subcapitata and E. magnus to 25 Gy external ɤ-radiation and allowed these and a non-exposed control culture to grow for 24 h in triplicates.
The two algae species were incubated with NaH14CO3-spiked (1.48 MBq) MWC medium for 7 days. After the incubation period, half of the R. subcapitata and E. magnus were exposed to the ɤ-radiation treatment while the other half was kept as an non-irradiated control. After 24 h cells from each treatment and species were harvested by centrifugation at 10,000 g for 5 min. After centrifuging the samples, the supernatant was discarded and the microalgae were rinsed until the supernatant was 0.01% of the initial activity added (Nascimento and Karlson, 2008). The algae pellets were then frozen at −20°C.
Before the experiment, samples of each of the concentrated R. subcapitata and E. magnus suspensions were thawed and observed under a microscope to confirm cell integrity. To quantify the 14C concentrations in the phytoplankton, 500 μl samples were taken, preserved in 1 mL of 70% ethanol, and solubilized in 1 mL Soluene-350 for 2 weeks in the dark at room temperature. Three-day old D. magna neonates, which were reared on the corresponding algae species, were transferred into experimental units, which consisted of 50 mL centrifuge tubes. Each treatment was set up as triplicates per exposure and algae species, with 40 mL fresh COMBO media and 15 D. magna individuals per unit. To ensure that food was not limited, D. magna were fed R. subcapitata or E. magnus at a ratio of ~0.1 mg C/daphniid and were allowed to graze for 24 h. After 24 h, D. magna were transferred to 5 mL of clean COMBO media to clear their guts and ensure that the 14C detected was the 14C incorporated into the tissues and not 14C from undigested microalgae in the gut. After 30 min, they were picked out and preserved in 70% ethanol.
The preserved daphniids from each replicate were then pooled and solubilised in 1 mL Soluene-350 for 2 weeks in the dark at room temperature. After the addition of 10 mL Ultimate Gold XR to each scintillation vail, the radioactivity in the samples D. magna, R. subcapitata, and E. magnus was measured in a liquid scintillation counter (Tri Carb, 2,910 TR, Perkin Elmer). The scintillation counts (Bq) were converted to μg 14C by using the specific activity of 14C (1 Bq equals 6.1−06 μg 14C) and standardized to the number of phytoplankton cells or daphniids in the sample.
The metabolic profile dataset was analyzed using constrained analysis of principal coordinates (CAP) based on Bray-Curtis dissimilarity. First, the overall multivariate metabolite data for both species was constrained by species, ɤ-radiation dose and time after exposure. An interaction between the terms was also included to test potential differences in the effects between species, time after exposure and doses. The minimal adequate model (containing only significant parameters) was identified, but due to the nestedness of the factors, non-significant factors (either time or dose) were included in the model as a condition to partial out their effects. Then separate CAP analyses were performed per species to discern interactive effects. Exposure to ɤ-radiation and time after exposure were used to constrain the multivariate metabolite data for each species. The analyses were performed using the “capscale” function from the {vegan} R package (Oksanen et al., 2016). Significance of the models, terms, and axes were assessed using a permutation procedure with 999 permutations. A similarity percentage test (SIMPER) was used to identify which metabolites drove the metabolic profile effects.
The effects of time and exposure on protein content of the algae, as well as on carbon incorporation and grazing rates of D. magna, were analyzed using two-way analysis of variance (ANOVA) followed by a Tukey's post-hoc test. Interactive effects between time and exposure were also tested and, if significant, are noted in the results section. Prior to analyses, the homogeneity of variance and normality of each variable were tested, and data transformations were applied when necessary.
All statistical analyses were performed in R version 3.3.3 (R Core Team, 2018). The significance levels were set to α = 0.05.
The metabolic profiles of the two phytoplankton species were significantly different from each other [F(1, 80) = 1206.8, p = 0.001] and were also significantly influenced by dose [F(2, 80) = 3.42, p = 0.03], and the interaction between species and dose was also significant [F(2, 80) = 3.32, p = 0.03; Figure S1]. To discern the interactive effect of species and dose the effect of time and dose was investigated on each species separately. Six metabolites contributed to 80% of the dissimilarity between the species (Table S1). Sorbitol, mannitol, and inositol (scyllo) characterized the metabolic profile of E. magnus, while Glycerol-3-phosphate, taurine and aspartic acid characterized the metabolic profile of R. subcapitata.
The metabolic composition of R. subcapitata was influenced by time after exposure [F(4, 38) = 2.37, p < 0.05; Figure 1], but not by dose. Glycerol-3-phosphate and taurine characterized the metabolic profile in R. subcapitata at 9, 12, and 24 h after exposure, while aspartic acid and isoleucine were more characteristic of the metabolic profile at 3 and 6 h after exposure (Figure 1). A total of 9 metabolites contributed to 80% of the dissimilarity between time points: three amino acids, aspartic acid, taurine and isoleucine; two fatty acids, linoleic acid and stearic acid; one lipid, glycerol-3-phosphate; two alcohol sugars, mannitol and sorbitol; and an organic acid, malic acid (Figure 2 and Table S2). Even though there was no significant effect of treatment, there was a trend for higher concentrations at 24 h at the 25 Gy dose compared to 5 Gy and the control (Figure 2 and Figure S2).
Figure 1. CAP (Constrained Analysis of Principal coordinates) biplot based on the relative metabolite concentration matrix of R. subcapitata constrained by time after ɤ-radiation exposure. Points represent individual samples and are shaped and colored by time after exposure (see figure legend). The ellipsoids represent the standard deviation for each time after exposure. The metabolites shown in the figure—Asp (aspartic acid), Tau (taurine), G-3-P (glycerol-3-phosphate), Mal (malic acid), Lin (linoleic acid), Ste (stearic acid), Man (mannitol), Sor (sorbitol), and Ile (isoleucine)—contributed 80% to the dissimilarity as identified in the SIMPER analysis (Table S1).
Figure 2. Time series of average relative concentrations (± SE) of the metabolites identified by the SIMPER analysis as contributing 80% to the cumulative dissimilarity between times after exposure (Table S1) in R. subcapitata (n = 3). (A) Aspartic acid, (B) taurine, (C) isoleucine, (D) linoleic acid, (E) stearic acid, (F) glycerol-3-phosphate, (G) malic acid, (H) sorbitol, and (I) mannitol.
The metabolic composition of E. magnus was influenced by the ɤ-radiation exposure [F(2, 38) = 7.01, p < 0.01; Figure 3 and Figure S3]. The sugar alcohols sorbitol, mannitol, and inositol (scyllo) contributed to 80% of the dissimilarity between doses (Figures 4A–C and Table S3). Higher concentrations of all three sugar alcohols characterized the 25 Gy dose, while the concentrations were generally lower in the 5 Gy dose and the control (Figure 4).
Figure 3. CAP biplot based on the relative metabolite concentration matrix of E. magnus constrained by dose. Points represent individual samples and are shaped and colored by exposure (see figure legend). The ellipsoids represent the standard deviation for each exposure. The metabolites shown in the figure—Sor (sorbitol), Man (mannitol), and Ino (scyllo- inositol)—contributed 80% to the dissimilarity as identified in the SIMPER analysis (Table S2).
Figure 4. Time series of average relative concentrations (± SE, n = 3) of metabolites identified by the SIMPER analysis as contributing 80% to the cumulative dissimilarity between doses (Table S2) in E. magnus. (A) Sorbitol, (B) mannitol, and (C) inositol (scyllo).
The total protein content of R. subcapitata did not change significantly over time or with dose (Figure 5A), while in E. magnus both time after exposure [F(4, 37) = 12.72, p < 0.001] and dose [F(2, 37) = 12.90, p < 0.001] affected the protein content (Table 1). In general, the protein content in E. magnus increased over time and the algae exposed to 25 Gy had lower protein content compared to both the control and the 5 Gy dose (Figure 5B).
Figure 5. Protein content in (A) R. subcapitata and (B) E. magnus over time per dose (mean ± SE; n = 3).
Table 1. Differences from Tukey's all-pair comparison of means shown for protein content of E. magnus for time after exposure and dose.
There was no difference in carbon incorporation into microalgae between the control and the 25 Gy dose, but E. magnus incorporated significantly more 14C than R. subcapitata [F(1, 22) = 24.81, p < 0.001; Figure 6A]. When these algae were fed to D. magna, the cladocerans incorporated more 14C when both algae species were exposed to ɤ-radiation compared to the control [F(1, 9) = 24.09, p < 0.001; Figure 6B]. Furthermore, D. magna incorporated more 14C when fed with R. subcapitata compared to E. magnus [F(1, 9) = 24.36, p < 0.001; Figure 6B].
Figure 6. 14C incorporation in (A) R. subcapitata and E. magnus (mean ± SE; n = 6) and (B) D. magna when reared on R. subcapitata and E. magnus under control conditions and exposed to 25 Gy of ɤ-radiation (mean ± SE; n = 3).
We investigated the effects of external, short-term ɤ-radiation on the biochemical composition of two phytoplankton species and whether radiation-induced effects on primary producers also affected their suitability as food for primary consumers. Our results indicate that ɤ-radiation had a significant effect on the biochemical composition and primary production of phytoplankton, but these effects were species specific. The overall metabolic profile of both phytoplankton species was affected by ɤ-radiation and also by an interaction of ɤ-radiation and time after exposure (Figure S1). However, species specific analysis indicated that the metabolic profile (Figures 1, 2), protein content (Figure 5A) and 14C incorporation (Figure 6A) of R. subcapitata were not affected by dose. In contrast, the metabolic profile of E. magnus was affected by dose (Figures 3, 4), as were the total protein content (Figure 5B) and 14C incorporation (Figure 6A).
The metabolic profile of R. subcapitata was significantly affected by time after exposure, but no significant differences could be detected between doses (Figure 2 and Table S2). Two sugar alcohols (sorbitol and mannitol), taurine, malic acid, aspartic acid, and glycerol-3-phospate showed a time dependent change with a trend to higher relative concentrations at 25 Gy and 24 h after exposure (Figure 1). Similar to sugar alcohols, taurine has been shown to act as a ROS scavenger in various organisms (Shimada et al., 2015; Tevatia et al., 2015). Malic acid is part of the tricarboxylic acid (TCA) cycle, which is part of the energy metabolism being responsible in driving the oxidation of respiratory substrate for ATP synthesis (Sweetlove et al., 2010). This response was similar in other metabolites of the TCA cycle (Figure S2). Previous studies have shown that the synthesis of ATP through the TCA cycle varies with physiological demand of the plants. However, when ATP, and therefore energy demand, increases, most of the ATP is synthesized through the TCA cycle (Sweetlove et al., 2010). Aspartic acid and/or members of the Aspartate family, such as isoleucine, have been shown to feed into the TCA cycle. Under non-stressed conditions, photosynthesis is the main energy source for plants during the day, while during the night, the TCA cycle is used to generate energy, among other pathways (Galili, 2014). The relatively high concentrations of metabolites associated to the TCA cycle at the 25 Gy treatment could indicate that the photosynthetic capacity of R. subcapitata was impaired by ionizing radiation.
Previous studies on another green algae, Chlamydomonas reinhardtii, detected adverse effects of ɤ-radiation. The photosynthetic machinery of C. reinhardtii was impaired, especially at higher dose rates (Gomes et al., 2017). The authors attributed these effects to the production of ROS, which was induced in a dose-dependent manner (Gomes et al., 2017). Similar trends were seen in ɤ-exposed R. subcapitata by Bradshaw et al. (2019), who showed a stimulation of antioxidant production (catalase, thiamine diphosphate) and photoprotective mechanisms (xanthophyll cycle). The metabolic profile of E. magnus was directly affected by ɤ-radiation exposure (Figures 3, 4). The changes in the metabolic profile were mostly characterized by a ~1.5-fold increase in carbohydrates in the highest exposure (25 Gy), namely sorbitol, mannitol, and scyllo- inositol (Figure 4). One of the many cellular roles carbohydrates play is the provision of non-enzymatic antioxidant defense (Demidchik, 2015). Previous studies have shown an increase in carbohydrates and total soluble sugars in plants as a response to ɤ-radiation, using similar dose rates to our study (El-Beltagi et al., 2011; Moussa, 2011). Since no enzymatic scavenging mechanism for hydroxyl radicals (•OH) exists in plants, they rely on non-enzymatic antioxidants to detoxify these highly damaging ROS (Gechev et al., 2006; Keunen et al., 2013). In plants, mono- and disaccharides have been shown to act as •OH scavengers as follows: maltose -> sucrose -> fructose -> glucose -> deoxyribose -> sorbitol (Morelli et al., 2003; Couée et al., 2006; Demidchik, 2015). Additionally, mannitol has been linked to an increased resistance to oxidative stress in transgenic tobacco plants (Shen et al., 1997). The increase in sorbitol, mannitol, and scyllo- inositol may therefore indicate that the exposure to ɤ-radiation induced a state of oxidative stress in E. magnus.
Gamma radiation had no significant effect on the total protein content of R. subcapitata, whereas E. magnus contained significantly less proteins at 25 Gy compared to the control and 5 Gy (Figure 5). While only a rough measure, a decrease in protein content could indicate a decrease in antioxidants, which in turn could be indicative of RNA damage that would subsequently lead to failure in protein synthesis (Bajaj, 1970; El-Beltagi et al., 2011). The two FAs linoleic acid and stearic acid contributed in part to the dissimilarity in the metabolic profile of R. subcapitata with time after exposure. These PUFAs are the major FAs in the lipids of plant membranes, built into galactolipids in the thylakoid membranes and phospholipids in all other membranes (Møller et al., 2007). One of the most common and most severe effects of oxidative stress is lipid peroxidation, which can lead to membrane damage (Gill and Tuteja, 2010; Won et al., 2014b). An increase in lipid peroxidation has been shown in R. subcapitata exposed to ɤ-radiation (Bradshaw et al., 2019). PUFAs are especially susceptible to ROS, since the double bond weakens the C-H bond and facilitates H+ substitution. We would have therefore expected to find higher concentrations of the saturated stearic acid and lower concentrations of the PUFA linoleic acid in ɤ-irradiated microalgae. Similar trends in R. subcapitata were observed in a previous study (Nascimento and Bradshaw, 2016), where no changes in FA concentrations were found after exposure to short-term ɤ-irradiation. However, glycerol-3-phosphate concentrations were highest in R. subcapitata at 25 Gy after 24 h, which could indicate a repair mechanism of cell membranes, as glycerol-3-phosphate is the starting building block for glycerol lipids (Cao et al., 2006).
Nascimento and Bradshaw (2016) investigated the direct and indirect effects of ɤ-radiation on a grazer-phytoplankton interaction using R. subcapitata and D. magna as their model species and exposing them to short-term ɤ-radiation, both together and separately. When unexposed D. magna were fed with R. subcapitata that had been exposed, the daphniids showed the highest 14C incorporation. This increase in carbon incorporation was attributed to an increased grazing rate of D. magna, which in turn could have been caused by a lower food quality of the exposed R. subcapitata. However, the FA profile did not show any changes after exposure (Nascimento and Bradshaw, 2016). Concurring with these results, D. magna in our study also incorporated more 14C when the phytoplankton had been exposed to ɤ-radiation compared to the control (Figure 6B). This trend was the same irrespective of phytoplankton species, i.e., regardless of the original (control) suitability of these species as food; D. magna incorporated more 14C when fed with R. subcapitata than with E. magnus, both when irradiated and non-irradiated. These two phytoplankton species were chosen due to their different FA profiles, which was used as a proxy for overall food quality. Chlorophyta, like R. subcapitata, are generally considered to be of lower food quality for zooplankton, since they contain very little or lack FAs such as DHA and EPA (Lang et al., 2011; Taipale et al., 2013). These FAs have been linked to increased somatic growth in crustaceous zooplankton (Brett and Müller Navarra, 1997).
The higher 14C incorporation of D. magna fed with E. magnus exposed to 25 Gy might also be explained by an increase in feeding stimuli. Chemosensory studies in crustaceans have shown that carnivore species primarily detect amino acids, nucleotides and amines, while herbivores and omnivores are additionally sensitive to carbohydrates (Corotto and O'Brien, 2002; Eriksson Wiklund et al., 2014). These biochemicals can act as cues for location and identification of preferred food items (Corotto and O'Brien, 2002). In Daphnia, sugar receptors have been identified, which suggests that sugars could be used as a feeding stimulus (Peñalva-Arana et al., 2009; Eriksson Wiklund et al., 2014). In our study, E. magnus had higher sugar alcohol content at 25 Gy and, in R. subcapitata, the amino acid content was also higher at 25 Gy compared to the control. These changes in the biochemical composition of E. magnus, and to a certain extent also in R. subcapitata, could have resulted in higher feeding rates due to a higher release of chemical stimuli. However, an increased feeding of D. magna on E. magnus exposed to 25 Gy might have had some adverse effects on the daphniids. Certain sugars have been shown to lead to heart arrhythmia in Daphnia (Campbell, 2004). These changes in heart rate may have in turn also contributed to an increased feeding rate, as heart rate and feeding rate are usually tightly coupled (Eriksson Wiklund et al., 2014).
Another factor that could explain the increased 14C incorporation by the daphniids feeding on R. subcapitata could be that the algae anti-grazing defense mechanisms were decreased due to exposure to ɤ-radiation (Nascimento and Bradshaw, 2016). The metabolic profile of R. subcapitata shows an increase of metabolites that are part of glycolysis (glucose, glucose-6-phosphate, and fructose-6-phosphate; Figure S2) and the citric acid cycle (e.g., malic acid; Figure 2G and Figure S1). This could indicate a shift from photosynthesis to photorespiration, which is less efficient and could therefore lead to a shift in energy allocation toward processes that are needed for cellular functions and away from energy-demanding processes such as grazing defenses.
In conclusion, our results indicate that external, short-term ɤ-radiation can change the biochemical composition of aquatic primary producers. Changes in the metabolic profile indicated that this could be due to responses to oxidative stress, or changes in photosynthetic capacity. However, the response to ɤ-radiation is species specific. Our results also suggest that these biochemical changes can affect primary consumers, such as D. magna, through changes in food quality, increases in chemical feeding stimuli, or decreased grazing defenses by the algae.
This study was carried out using D. magna as study animal, for which no ethics approval was required.
A-LG preformed the experiment, analyzed the data, and wrote the first draft of the manuscript. CB and A-LG designed the study and wrote the manuscript.
This work was supported by Ph.D. funding from the Department of Ecology, Environment and Plant Sciences, Stockholm University and by the European Commission 7th Framework Program through the COMET project, grant agreement no: 604974.
The authors declare that the research was conducted in the absence of any commercial or financial relationships that could be construed as a potential conflict of interest.
We would like to thank the Swedish Metabolomics Center (SMC) for the metabolic profiling and help with data interpretation, N. Stjärnkvist and N. Perman for assistance during sample analysis and FJA Nascimento for valuable input on the manuscript.
The Supplementary Material for this article can be found online at: https://www.frontiersin.org/articles/10.3389/fenvs.2019.00100/full#supplementary-material
Ahlgren, G., Lundstedt, L., Brett, M. T., and Forsberg, C. (1990). Lipid composition and food quality of some freshwater phytoplankton for cladoceran zooplankters. J. Plankton Res. 12, 809–818. doi: 10.1093/plankt/12.4.809
Annadotter, H., Rasmussen, U., and Rydberg, S. (2015). Biotransfer of β-N-Methylamino-l-alanine (BMAA) in a eutrophicated freshwater lake. Mar. Drugs 13, 1185–1201. doi: 10.3390/md13031185
Bajaj, Y. P. S. (1970). Effect of gamma-irradiation on growth, RNA, protein, and nitrogen contents of bean callus cultures. Ann. Bot. 34, 1089–1096. doi: 10.1093/oxfordjournals.aob.a084440
Bradshaw, C., Meseh, D. A., Alasawi, H., Qiang, M., Snoeijs-Leijonmalm, P., and Nascimento, F. J. A. (2019). Joint effects of gamma radiation and cadmium on subcellular-, individual and population-level endpoints of the green microalga Raphidocelis subcapitata. Aquat. Toxicol. 211, 217–226. doi: 10.1016/j.aquatox.2019.04.008
Brett, M. T., and Müller Navarra, D. C. (1997). The role of highly unsaturated fatty acids in aquatic foodweb processes. Ecology 38, 483–499. doi: 10.1046/j.1365-2427.1997.00220.x
Campbell, A. K. (2004). Lactose causes heart arrhythmia in the water flea Daphnia pulex. Comp. Biochem. Physiol. B Biochem. Mol. Biol. 139, 225–234. doi: 10.1016/j.cbpc.2004.07.004
Cao, J., Li, J. L., Li, D., and Tobin, J. F. (2006). Molecular identification of microsomal acyl-CoA: glycerol-3-phosphate acyltransferase, a key enzyme in de novo triacylglycerol synthesis. PNAS 103, 19695–19700. doi: 10.1073/pnas.0609140103
Choo, K.-S., Snoeijs, P., and Pedersén, M. (2004). Oxidative stress tolerance in the filamentous green algae Cladophora glomerata and Enteromorpha ahlneriana. J. Exp. Mar. Biol. Ecol. 298, 111–123. doi: 10.1016/j.jembe.2003.08.007
Corotto, F. S., and O'Brien, M. R. (2002). Chemosensory stimuli for the walking legs of the crayfish Procambarus clarkii. J. Chem. Ecol. 28, 1117–1130. doi: 10.1023/A:1016273431866
Couée, I., Sulmon, C., Gouesbet, G., and Amrani, E. L. A. (2006). Involvement of soluble sugars in reactive oxygen species balance and responses to oxidative stress in plants. J. Exp. Bot. 57, 449–459. doi: 10.1093/jxb/erj027
Dallas, L. J., Keith-Roach, M., Keith-Roach, M., Lyons, B. P., Lyons, B. P., Jha, A. N., et al. (2012). Assessing the impact of ionizing radiation on aquatic invertebrates: a critical review. Radiat. Res. 177, 693–716. doi: 10.1667/RR2687.1
Demidchik, V. (2015). Mechanisms of oxidative stress in plants: from classical chemistry to cell biology. Environ. Exp. Bot. 109, 212–228. doi: 10.1016/j.envexpbot.2014.06.021
Dickman, E. M., Newell, J. M., González, M. J., and Vanni, M. J. (2008). Light, nutrients, and food-chain length constrain planktonic energy transfer efficiency across multiple trophic levels. PNAS 105, 18408–18412. doi: 10.1073/pnas.0805566105
El-Beltagi, H. S., Ahmed, O. K., and El-Desouky, W. (2011). Effect of low doses γ-irradiation on oxidative stress and secondary metabolites production of rosemary (Rosmarinus officinalis L.) callus culture. Rad. Phys. Chem. 80, 968–976. doi: 10.1016/j.radphyschem.2011.05.002
Elser, J. J., and Hassett, R. P. (1994). A stoichiometric analysis of the zooplankton–phytoplankton interaction in marine and freshwater ecosystems. Nature 370, 211–213. doi: 10.1038/370211a0
Eriksson Wiklund, A. K., Adolfsson-Erici, M., Liewenborg, B., and Gorokhova, E. (2014). Sucralose induces biochemical responses in Daphnia magna. PLoS ONE 9:e92771–6. doi: 10.1371/journal.pone.0092771
Filimonova, V., Gonçalves, F., Marques, J. C., De Troch, M., and Gonçalves, A. M. M. (2016). Fatty acid profiling as bioindicator of chemical stress in marine organisms: a review. Acad. Bibliograph. 67, 657–672. doi: 10.1016/j.ecolind.2016.03.044
Galili, G. (2014). The aspartate-family pathway of plants. Plant Signal. Behav. 6, 192–195. doi: 10.4161/psb.6.2.14425
Gechev, T. S., Van Breusegem, F., Stone, J. M., Denev, I., and Laloi, C. (2006). Reactive oxygen species as signals that modulate plant stress responses and programmed cell death. Bioessays 28, 1091–1101. doi: 10.1002/bies.20493
Gill, S. S., and Tuteja, N. (2010). Reactive oxygen species and antioxidant machinery in abiotic stress tolerance in crop plants. Plant Physiol. Biochem. 48, 909–930. doi: 10.1016/j.plaphy.2010.08.016
Gomes, T., Song, Y., Brede, D. A., Xie, L., Gutzkow, K. B., Salbu, B., et al. (2018). Gamma radiation induces dose-dependent oxidative stress and transcriptional alterations in the freshwater crustacean Daphnia magna. Sci. Total Env. 628–629, 206–216. doi: 10.1016/j.scitotenv.2018.02.039
Gomes, T., Xie, L., Brede, D., Lind, O. C., Solhaug, K. A., Salbu, B., et al. (2017). Sensitivity of the green algae Chlamydomonas reinhardtii to gamma radiation: photosynthetic performance and ROS formation. Aquat. Toxicol. 183, 1–10. doi: 10.1016/j.aquatox.2016.12.001
Guillard, R. R. L., and Lorenzen, C. J. (1972). Yellow-green algae with chlorophyllide C1,2. J. Phycol. 8, 10–14. doi: 10.1111/j.0022-3646.1972.00010.x
Gullberg, J., Jonsson, P., Nordström, A., Sjöström, M., and Moritz, T. (2004). Design of experiments: an efficient strategy to identify factors influencing extraction and derivatization of Arabidopsis thaliana samples in metabolomic studies with gas chromatography/mass spectrometry. Anal. Biochem. 331, 283–295. doi: 10.1016/j.ab.2004.04.037
Hu, Q. H., Weng, J. Q., and Wang, J. S. (2010). Sources of anthropogenic radionuclides in the environment: a review. J. Environ. Radioact. 101, 426–437. doi: 10.1016/j.jenvrad.2008.08.004
IAEA (2003). Extent of Environmental Contamination by Naturally Occurring Radioactive Material (NORM) and Technological Options for Mitigation.
Jan, S., Parween, T., Siddiqi, T. O., and Mahmooduzzafar. (2012). Effect of gamma radiation on morphological, biochemical, and physiological aspects of plants and plant products. Environ. Rev. 20, 17–39. doi: 10.1139/a11-021
Keunen, E., Peshev, D., Vangronsveld, J., van den Ende, W., and Cuypers, A. (2013). Plant sugars are crucial players in the oxidative challenge during abiotic stress: extending the traditional concept. Plant Cell Environ. 36, 1242–1255. doi: 10.1111/pce.12061
Kovacik, J., Klejdus, B., Hedbavny, J., and Backor, M. (2010). Effect of copper and salicylic acid on phenolic metabolites and free amino acids in Scenedesmus quadricauda (Chlorophyceae). Plant Sci. 178, 307–311. doi: 10.1016/j.plantsci.2010.01.009
Lang, I., Hodac, L., and Friedl, T. (2011). Fatty acid profiles and their distribution patterns in microalgae: a comprehensive analysis of more than 2,000 strains from the SAG culture collection. BMC Plant Biol. 11:124. doi: 10.1186/1471-2229-11-124
Mallick, N., and Mohn, F. H. (2000). Reactive oxygen species: response of algal cells. J. Plant Physiol. 157, 183–193. doi: 10.1016/S0176-1617(00)80189-3
Malzahn, A. M., Hantzsche, F., Schoo, K. L., and Boersma, M. (2010). Differential effects of nutrient-limited primary production on primary, secondary or tertiary consumers. Oecologia 162, 35–48. doi: 10.1007/s00442-009-1458-y
Møller, I. M., Jensen, P. E., and Hansson, A. (2007). Oxidative modifications to cellular components in plants. Annu. Rev. Plant Biol. 58, 459–481. doi: 10.1146/annurev.arplant.58.032806.103946
Morelli, R., Russo-Volpe, S., Bruno, N., and Lo Scalzo, R. R. (2003). Fenton-dependent damage to carbohydrates: free radical scavenging activity of some simple sugars. J. Agric. Food Chem. 51, 7418–7425. doi: 10.1021/jf030172q
Moussa, H. (2011). Low dose of gamma irradiation enhanced drought tolerance in soybean. Acta Agronom. Hungar.59, 1–12. doi: 10.1556/AAgr.59.2011.1.1
Nascimento, F. J. A., and Bradshaw, C. (2016). Direct and indirect effects of ionizing radiation on grazer–phytoplankton interactions. J. Environ. Radioact. 155–156, 63–70. doi: 10.1016/j.jenvrad.2016.02.007
Nascimento, F. J. A., and Karlson, A. (2008). Settling blooms of filamentous cyanobacteria as food for meiofauna assemblages. Limnol. Oceanogr. 53, 2636–2643. doi: 10.4319/lo.2008.53.6.2636
Nascimento, F. J., Svendsen, C., and Bradshaw, C. (2016). Joint toxicity of cadmium and ionizing radiation on zooplankton carbon incorporation, growth and mobility. Env. Sci. Technol. 50, 1527–1535. doi: 10.1021/acs.est.5b04684
Neves, M., Castro, B. B., Vidal, T., Vieira, R., Marques, J. C., Coutinho, J. A. P., et al. (2015). Biochemical and populational responses of an aquatic bioindicator species, Daphnia longispina, to a commercial formulation of a herbicide (Primextra® Gold TZ) and its active ingredient (S-metolachlor). Ecol. Indicat. 53, 220–230. doi: 10.1016/j.ecolind.2015.01.031
Oksanen, J., Blanchet, F. G., Friendly, M., Kindt, R., Legendre, P., McGlinn, D., et al. (2016). Vegan: Community Ecology Package. 2nd Edn, GLP−2.
Parisot, F., Bourdineaud, J. P., Plaire, D., Adam-Guillermin, C., and Alonzo, F. (2015). DNA alterations and effects on growth and reproduction in Daphnia magna during chronic exposure to gamma radiation over three successive generations. Aquat. Toxicol. 163, 27–36. doi: 10.1016/j.aquatox.2015.03.002
Peñalva-Arana, D. C., Lynch, M., and Robertson, H. M. (2009). The chemoreceptor genes of the waterflea Daphnia pulex: many Grs but no Ors. BMC Evol. Biol. 9, 79–11. doi: 10.1186/1471-2148-9-79
R Core Team, (2018) R: A Language and Environment for Statistical Computing. Vienna: R Foundation for Statistical Computing. Avaliable online at: https://www.R-project.org/.
Rocchetta, I., Mazzuca, M., Conforti, V., Ruiz, L., Balzaretti, V., and Ríos de Molina Mdel, C. (2006). Effect of chromium on the fatty acid composition of two strains of Euglena gracilis. Env. Pollut. 141, 353–358. doi: 10.1016/j.envpol.2005.08.035
Shen, B., Jensen, R. G., and Bohnert, H. J. (1997). lncreased resistance to oxidative stress in transgenic plants by targeting mannitol biosynthesis to chloroplasts. Plant Physiol. 113, 1177–1183. doi: 10.1104/pp.113.4.1177
Shimada, K., Jong, C. J., Takahashi, K., and Schaffer, S. W. (2015). “Role of ROS production and turnover in the antioxidant activity of taurine,” in Taurine 9, eds J. Marcinkiewicz and S. W. Schaffer (Cham: Springer International Publishing), 581–596. doi: 10.1007/978-3-319-15126-7_47
Sommer, U., Adrian, R., De Senerpont Domis, L., Elser, J. J., Gaedke, U., Ibelings, B., et al. (2012). Beyond the Plankton Ecology Group (PEG) model: mechanisms driving plankton succession. Annu. Rev. Ecol. Evol. Syst. 43, 429–448. doi: 10.1146/annurev-ecolsys-110411-160251
Spáčil, Z., Eriksson, J., Jonasson, S., Rasmussen, U., Ilag, L. L., and Bergman, B. (2010). Analytical protocol for identification of BMAA and DAB in biological samples. Analyst 135, 127–132. doi: 10.1039/B921048B
Spitz, D. R., Azzam, E. I., Li, J. J., and Gius, D. (2004). Metabolic oxidation/reduction reactions and cellular responses to ionizing radiation: a unifying concept in stress response biology. Cancer Metast. Rev. 23, 311–322. doi: 10.1023/B:CANC.0000031769.14728.bc
Sterner, R. W., and Hessen, D. O. (1994). Algal nutrient limitation and the nutrition of aquatic herbivores. Ann. Rev. Ecol. Syst. 25, 1–29. doi: 10.1146/annurev.es.25.110194.000245
Strelkov, S., Roessner-Tunali, U., Forbes, M. G., Fernie, A. R., and Kopka, J. (2005). GC-MS libraries for the rapid identification of metabolites in complex biological samples. FEBS Lett. 579, 1332–1337. doi: 10.1016/j.febslet.2005.01.029
Sweetlove, L. J., Beard, K. F. M., Nunes-Nesi, A., Fernie, A. R., and Ratcliffe, R. G. (2010). Not just a circle: flux modes in the plant TCA cycle. Trends Plant Sci. 15, 462–470. doi: 10.1016/j.tplants.2010.05.006
Taipale, S. J., Strandberg, U., Peltomaa, E., Galloway, A. W. E., Ojala, A., and Brett, M. T. (2013). Fatty acid composition as biomarkers of freshwater microalgae: analysis of 37 strains of microalgae in 22 genera and in seven classes. Aquat. Microb. Ecol. 71, 165–178. doi: 10.3354/ame01671
Tevatia, R., Allen, J., Rudrappa, D., White, D., Clemente, T. E., Cerutti, H., et al. (2015). The taurine biosynthetic pathway of microalgae. Alagal Res. 9, 21–26. doi: 10.1016/j.algal.2015.02.012
Vanhoudt, N., Horemans, N., Wannijn, J., Nauts, R., Van Hees, M., and Vandenhove, H. (2014). Primary stress responses in Arabidopsis thaliana exposed to gamma radiation. J. Environ. Radioact. 129, 1–6. doi: 10.1016/j.jenvrad.2013.11.011
Vrede, T., Dobberfuhl, D. R., Kooijman, S., and Elser, J. J. (2004). Fundamental connections among organism C: N: P stoichiometry, macromolecular composition, and growth. Ecology 85, 1217–1229. doi: 10.1890/02-0249
Won, E. J., Dahms, H. U., Kumar, K. S., Shin, K. H., and Lee, J. S. (2014a). An integrated view of gamma radiation effects on marine fauna: from molecules to ecosystems. Environ. Sci. Pollut. Res. 22, 17443–17452. doi: 10.1007/s11356-014-3797-4
Keywords: untargeted metabolite profiling, effects of ionizing radiation, phytoplankton, food quality, biochemical changes
Citation: Golz A-L and Bradshaw C (2019) Gamma Radiation Induced Changes in the Biochemical Composition of Aquatic Primary Producers and Their Effect on Grazers. Front. Environ. Sci. 7:100. doi: 10.3389/fenvs.2019.00100
Received: 31 January 2019; Accepted: 13 June 2019;
Published: 02 July 2019.
Edited by:
Rajeshwar P. Sinha, Banaras Hindu University, IndiaReviewed by:
Joana Luísa Pereira, University of Aveiro, PortugalCopyright © 2019 Golz and Bradshaw. This is an open-access article distributed under the terms of the Creative Commons Attribution License (CC BY). The use, distribution or reproduction in other forums is permitted, provided the original author(s) and the copyright owner(s) are credited and that the original publication in this journal is cited, in accordance with accepted academic practice. No use, distribution or reproduction is permitted which does not comply with these terms.
*Correspondence: Anna-Lea Golz, YW5uYWxlYS5nb2x6QHlhaG9vLmNvbQ==
Disclaimer: All claims expressed in this article are solely those of the authors and do not necessarily represent those of their affiliated organizations, or those of the publisher, the editors and the reviewers. Any product that may be evaluated in this article or claim that may be made by its manufacturer is not guaranteed or endorsed by the publisher.
Research integrity at Frontiers
Learn more about the work of our research integrity team to safeguard the quality of each article we publish.