- 1Environmental Molecular Microbiology Research Laboratory, Department of Biotechnology, Thiruvalluvar University, Vellore, India
- 2Centre of Excellence for Energy Research, Sathyabama Institute of Science and Technology, Chennai, India
- 3Centre for Nanoscience and Nanotechnology, Sathyabama Institute of Science and Technology, Chennai, India
- 4Key Laboratory of Integrated Regulation and Resources Development of Shallow Lakes College of Environment, Hohai University, Nanjing, China
- 5Solar Energy Lab, Department of Chemistry, Thiruvalluvar University, Vellore, India
- 6Institute of Biological and Biomedical Sciences, School of Biological Sciences, University of Portsmouth, Portsmouth, United Kingdom
Addressing heavy metal pollution is one of the hot areas of environmental research. Despite natural existence, various anthropomorphic sources have contributed to an unusually high concentration of heavy metals in the environment. They are characterized by their long persistence in natural environment leading to serious health consequences in humans, animals, and plants even at very low concentrations (1 or 2 μg in some cases). Failure of strict regulations by government authorities is also to be blamed for heavy metal pollution. Several individual treatments, namely, physical, chemical, and biological are being implied to remove heavy metals from the environment. But, they all face challenges in terms of expensiveness and in-situ treatment failure. Hence, integrated processes are gaining popularity as it is reported to achieve the goal effectively in various environmental matrices and will overcome a major drawback of large scale implementation. Integrated processes are the combination of two different methods to achieve a synergistic and an effective effort to remove heavy metals. Most of the review articles published so far mainly focus on individual methods on specific heavy metal removal, that too from a particular environmental matrix only. To the best of our knowledge, this is the first review of this kind that summarizes on various integrated processes for heavy metal removal from all environmental matrices. In addition, we too have discussed on the advantages and disadvantages of each integrated process, with a special mention of the few methods that needs more research attention. To conclude, integrated processes are proved as a right remedial option which has been detaily discussed in the present review. However, more research focus on the process is needed to challenge the in situ operative conditions. We believe, this review on integrated processes will surely evoke a research thrust that could give rise to novel remediation projects for research community in the future.
Introduction
Environment comprises of complex variables that includes air, water, and land. Their positive correlation forms a basis for the existence of humans along with other living creatures, namely, plants, animals, and microbes (Kalavathy, 2004). But, the science and technological advances in the form of industrial societies has contributed to severe environmental pollution of air, soil, and water, which are considered to be the indispensable part of human life. Increasing population, urbanization and rapid industrialization are recognized as significant challenges to the groundwater resources management in developing countries. Many research reports have confirmed the heavy metals pollution existence in several countries, thus signifying it as a worldwide problem. Significant concentrations of toxic heavy metals (Cd, As, Fe, Cr, Zn, Cu, Mn, Pb, Ni, etc.) in soil, surface, and ground water have been reported in various countries like China, Italy, Germany, Hong Kong, India, Turkey, Bangladesh, Greece, Iran etc. (Wuana and Okieimen, 2011; Kaonga et al., 2017). Above all these, lack of knowledge on the proper effluent disposal and failure to imply strict regulatory standards has added to the cause of environmental deterioration (Khalid et al., 2017). Therefore, these factors have ended up in generation of huge amounts of solid waste in various toxic forms which ultimately pollute the entire ecosystem. The disposed wastewaters will also affect the quality of surface water and soil, which on continuous proceeding without proper care may cross permissible limits prescribed by international regulatory agencies (E.P.A, 1992, 2002).
Heavy metals are regarded as significant environmental pollutants due to high density and high toxicity even at low concentrations (Lenntech Water treatment Air purification, 2004). According to United States Environmental Protection Agency (USEPA) compilation, eight heavy metals, namely, lead (Pb), chromium (Cr), arsenic (As), zinc (Zn), cadmium (Cd), copper (Cu), mercury (Hg), and nickel (Ni) are listed to be the most widespread heavy metals in the environment (Moore and Ramamoorthy, 1984; Wang and Chen, 2006). According to coordination chemistry of heavy metals, the above said heavy metals are also categorized as class B metals that are non-essential (highly toxic) trace elements (Nieboer and Richardson, 1980; Rzymski et al., 2015). Broad classification of heavy metals with examples is tabulated in Table 1. Heavy metals constitute an ill-defined group that is most commonly found at contaminated sites. They are characterized by their long persistence in natural environment leading to serious health consequences in humans, animals, and plants even at very low concentrations (1 or 2 μg in some cases) (Atkinson et al., 1998). A wide array of toxic heavy metals like Cr, Cd, Hg, Pb, etc., disposed by industries will remain as non-degradable and contaminate the soil and water to a greater extent (Aksu and Kutsal, 1990). Because of the high propensity nature of the heavy metals, they tend to accumulate in various environmental matrices, resulting of misleadingly higher concentrations than the prescribed average safety levels (Järup, 2003; Rzymski et al., 2014). According to Comprehensive Environmental Response Compensation and Liability Act, USA, the maximum permissible limit of heavy metals in aqueous medium is as follows, Cr-0.01 mg/L, Ar-0.01 mg/L, Cd-0.05 mg/L, Hg-0.002 mg/L, Pb-0.015 mg/L, and Ag-0.05 mg/L, respectively (Jaishankar et al., 2014). If the heavy metal concentration exceeds than those recommended, it can be major sources of many human life-threatening complications such as atherosclerosis, cancer, Alzheimer's disease, and Parkinson's disease, etc. (Muszynska and Hanus-Fajerska, 2015).
This has urged various researchers to develop many technological processes of remediation to bring these contaminant levels within the regulatory limit in the environment (Table 2). Most of the industrial scale remediation involving, physical, chemical, and biological methods are employed as single methods remediation strategies. Despite the success of these processes, they do face certain disadvantages like low efficiency, high cost and toxic sludge generation, etc. However, this can be overcome by upgrading them as integrated processes, which has exhibited more efficiency for heavy metal remediation as reported by many researchers in recent years (Huang et al., 2012; Mao et al., 2016; Selvi and Aruliah, 2018).
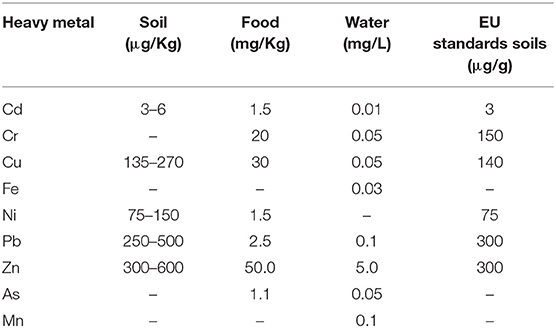
Table 2. Indian and European standards (EU) standards for heavy metals in soil, food and drinking water (Source: Awashthi, 2000).
During recent years, many treatment options like physical, chemical, and biological were implied to remediate heavy metal contaminated soil, water, and sediments. Such methods include thermal treatment, adsorption, chlorination, chemical extraction, ion-exchange, membrane separation, electrokinetics, bioleaching etc. (Table 3). As reported, most of the above said processes are implied as single methods of remediation only. Despite the success of these processes, they do face certain disadvantages like efficiency, cost and failure during large scale implementation, etc. (Volesky, 1990; Selvi et al., 2015). However, these can be overcome by upgrading them as integrated processes, which has various advantages, such as effectiveness, economic feasibility, short duration, versatile, eco-friendliness, on-site adaptability, and large scale treatment options etc. (Huang et al., 2012; Mao et al., 2016). Correlating to these factors, combined or integrated treatment processes were reported to be more effective by many researchers worldwide (Wick, 2009; Kim et al., 2010; Peng et al., 2011). But, integration of two different processes needs careful understanding and the purpose of the processes. Two processes should to be integrated in such a way that, they should be experimentally feasible even under large scale applications, economically viable and relatively efficient than the individual processes. Owing to these outcomes, integrated processes are gaining popularity toward heavy metal removal from various environmental matrices (Huang et al., 2012; Chen et al., 2013). Therefore, we, here in this review have focussed to discuss on various integrated or combined treatment options implied for heavy metal removal in soil, sediment, sludge, and aqueous matrices. To the best of our knowledge, this is the first review that summarizes different integrated remediation options for heavy metal removal.
Metals as environmental pollutants
Heavy metals are naturally occurring elements that are found throughout the earth's crust. Heavy metal pollution is caused as a result of both natural and anthropomorphic activities like mining, smelting, industrial production, using of metals, and metal containing compounds for domestic and agricultural applications. These sources were reported to contribute to human exposure and environmental contamination by various researchers (Herawati et al., 2000; Goyer, 2001; Zouboulis et al., 2004; He et al., 2005; Rahman and Bastola, 2014). The potential sources of environmental contaminations are shown in Figure 1. Toxicological properties of heavy metals are characterized by persistence of metal (long half-life), soil residence time (>1,000 years), chronic, and sub-lethal effects of the metal, bioaccumulation, biomagnification, teratogenic, and carcinogenic properties of the metal (Manzetti et al., 2014).
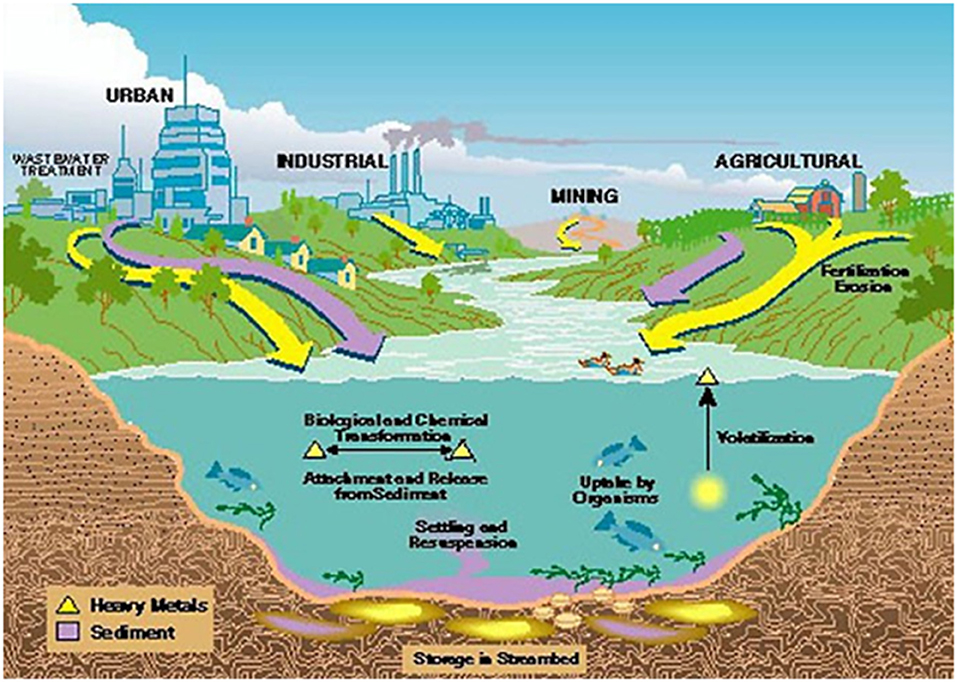
Figure 1. Potential sources of heavy metals in the environment (Source: Garbarino et al., 1995).
Heavy Metals Distribution in Environment
Natural Sources
Heavy Metals in Rocks
Rocks are one of the natural sources for heavy metals in the environment. Rocks are classified into magmatic rocks, sedimentary rocks, and metamorphic rocks. Magma is a molten rock that contains various chemical elements transported to the earth surface by geological process such as volcanism or plate tectonics (Press and Sievers, 1994). Heavy metals are incorporated via isomorphic substitution into the crystal lattice of primary minerals while magma cools down. Variations in natural weather conditions cause physical damage to the rocks and disintegrate into particles as sediment that can hold water, gas, and oil since it is porous in nature. Mineral calcite present in the sediment is precipitated by living organisms or chemical reaction. This isomorphic substitution is decided by ion radius, charge, and electro negativity. The most common heavy metals occur in rock are Ni, Co, Mn, Li, Zn, Cu, Mo, Se, V, Rb, Ba, Pb, Ga, Sr, F, etc. (Mitchell, 1964).
Heavy Metals in Soils
Rocks disintegrate into fine particles or soil by the influence of ice, water, temperature, etc. The soil matrix is a major reservoir or transporting media for heavy metals, because soil and heavy metals associations have rich and diverse binding characteristics. Metals do not biodegrade like organic pollutants, rather they bioaccumulate in the environment. Soil matrix may adsorb, oxidize, exchange, catalyze, reduce, or precipitate the metal ions (Hashim et al., 2011). These processes depend on several factors such as pH, water content, temperature, particle size distribution, nature of metal, and the clay content. This composition will determine the mobility, solubility, and toxicity of heavy metals present in the soil.
Generally, the minerals are dissolved by interacting with carbonic acid and water. The insoluble minerals are dispersed into fine particles. Soils are contaminated by metals and metalloids from metal wastes, gasoline, animal manure, sludge, waste water irrigation, atmospheric deposition, etc. (Khan et al., 2008). Typical sources of ground water contamination are given in Table 4 (Spiegel and Maystre, 1998). The most common heavy metals found in soils are Pb, Cr, Zn, Cd, and Hg. Due to bioaccumulation and biomagnification, these metals decrease the crop production and affects the food chain. The soil concentration ranges and regulatory guidelines for some heavy metals are given in Table 5 (Wuana and Okieimen, 2011).
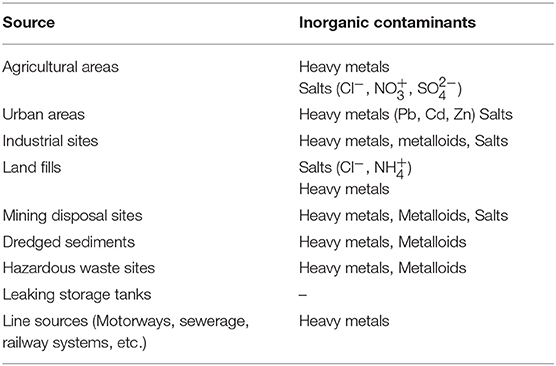
Table 4. Typical sources of inorganic substances contributing for ground water contamination (Source: Spiegel and Maystre, 1998).
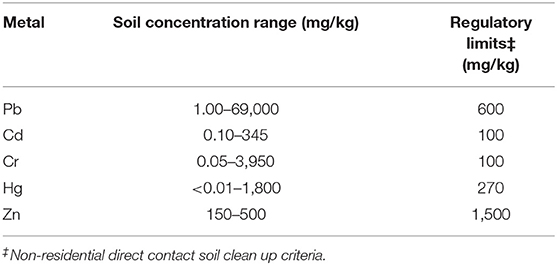
Table 5. Soil concentration ranges and regulatory guidelines of heavy metals (Wuana and Okieimen, 2011).
The heavy metals present in the soil become contaminant due to the following reasons, (i) Rapid generation via man made cycle, (ii) Direct exposure of mine samples due to transportation from mines to environmental location, and (iii) High metal dispose, etc. The heavy metal balance in the soil can be expressed in the form of equation shown below,
where “M” is the heavy metal, “p” is the parent material, “a” is the atmospheric deposition, “f ” is the fertilizer sources, “ag” are the agrochemical sources, “ow” are the organic waste sources, “ip” are other inorganic pollutants, “cr” is the crop removal, and “l” is the losses by leaching, volatilization (Alloway, 1995; D'amore et al., 2005).
Heavy Metals in Water
Metal composition in surface water like rivers, lakes, ponds, etc. is influenced by the type of soil, rock and water flow. Metals present on the surface of soil are carried out from its path, which ends up in sewage and reservoirs (Salem et al., 2000). The rain water gets contaminated while passing through the atmosphere. Water sources get contaminated by the flow of various industrial effluents into it. These industrial effluents were reported to contain many heavy metals as stated in Table 6. Ground waters are contaminated from landfill leachates, deep well liquid disposal, industrial wastes, etc. (Oyeku and Eludoyin, 2010). Factors such as temperature, pH, living organism, cation exchange, evaporation, absorption, etc., will also influence the metal composition in the water.
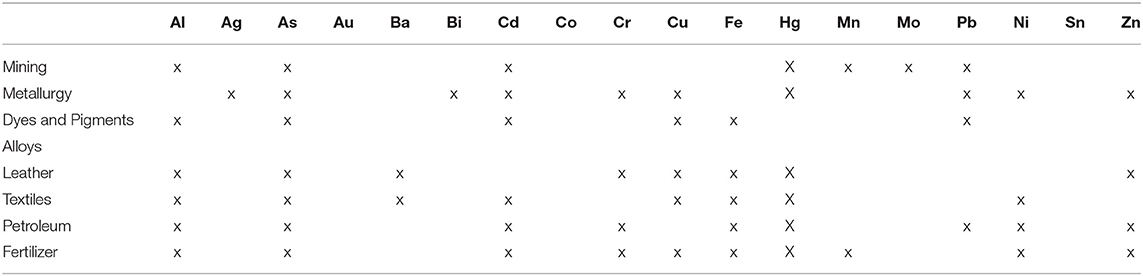
Table 6. Occurrence of metals or their compounds in effluents from various industries (Source: Nagajyoti et al., 2010), Copyright 2018 Springer Nature.
Heavy Metals in Atmosphere
Heavy metals are released into the atmosphere as gases and particulates by surface erosion and colloid loss. Sources of heavy metals in the atmosphere include, mineral dusts, sea salt particles, volcanic eruption, forest fires (Colbeck, 1995). Other than these natural sources, heavy metal air pollution can also originate from various industrial processes that involve the formation of dust particles, e.g., metal smelters and cement factories. Volatile metals such as Se, Hg, As, and Sb are transmitted in gaseous and particulate form in the atmosphere. Metals such as Cu, Pb, and Zn are transported as particulate form. The presence of heavy metal depends upon number of site-specific factors such as (1) the quantity and characteristics of the industrial pollutants, (2) environmental sensitivity, (3) potential for environmental release, (4) proximity of these heavy metals in humans and its effect on their health (Hassanien, 2011).
Anthropogenic Sources of Heavy Metals
Heavy metals are released into environment by various anthropogenic activities. The introduction of heavy metals due to continuous input of pesticides and fertilizer for food production is transported to surface water by infiltration (Darby et al., 1986). Zn and Cd are commonly present in phosphate fertilizers and the input of these fertilizers is directly proportional to the concentration of heavy metals. In addition to Zn and Cd, pesticides used in agriculture have elements such as Hg, As and Pb too. Thought he metal based pesticides are no longer in use, the earlier unregulated pesticide application has led to increased accumulation of heavy metals in various environmental matrices. Added to these, various industrial activities such as mining, coal combustion, effluent streams, and waste disposal has increased the heavy metal contamination in the environment (Herawati et al., 2000; Goyer, 2001; He et al., 2005). The most common anthropogenic sources contributing to heavy metals into the environment are listed in Figure 2.
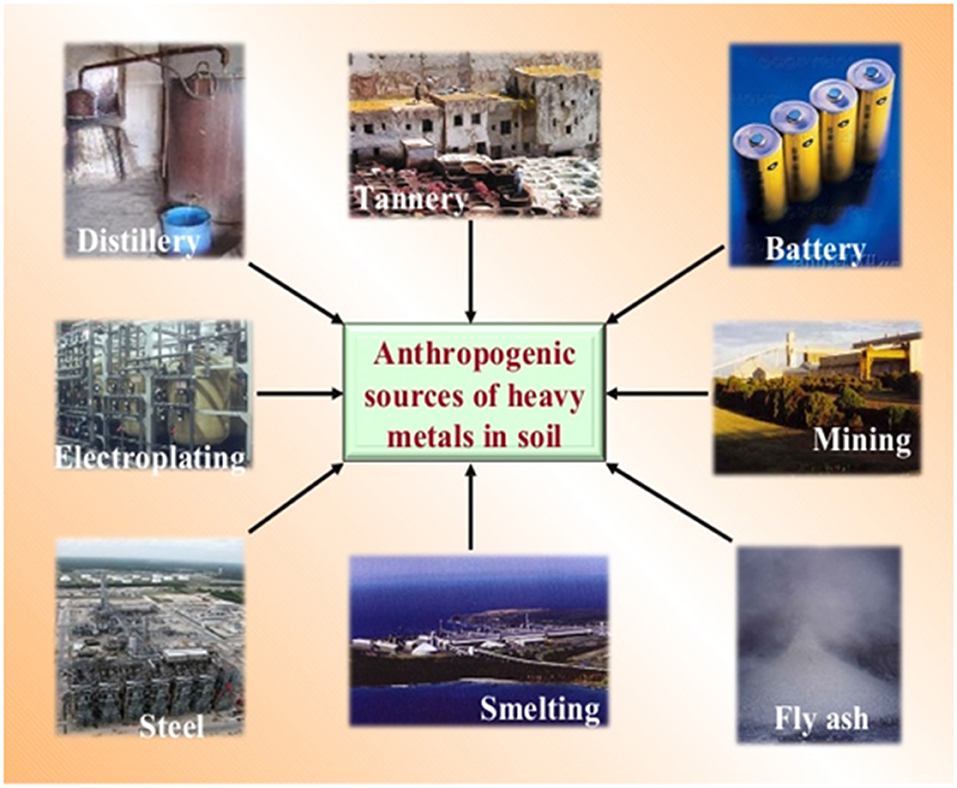
Figure 2. Anthropogenic sources of heavy metals. (Source: https://www.slideshare.net/tutan2009/heavy-metal-pollution-in-soil-and-its-mitigation-aspect-by-dr-tarik-mitran).
Need for Remediation of Metals in the Environment
The presence of heavy metals released from various sources is either directly or indirectly released into the environment that affects humans, animals, and plants. The main pathways of exposure are through inhalation, ingestion, and dermal contact. Due to increased risk of human exposure to heavy metals, it leads to serious health implications and environmental deterioration (Rzymski et al., 2015). Hence, these metals are categorized as systemic toxicants that can induce adverse health effects in humans that include cardiovascular diseases, developmental abnormalities, neurologic and neurobehavioral disorders, diabetes, hearing loss, hematologic and immunologic disorders, and various types of cancer (IARC, 1993; Mandel et al., 1995; Hotz et al., 1999; Steenland and Boffetta, 2000; WHO, 2001; Järup, 2003). Human health implications of heavy metal are shown in Figure 3. The severity of adverse health effects differs with the type of heavy metal, the chemical form, time of exposure, and the dosage. These heavy metal contaminants in soil were also reported to affect the ecosystem by disturbing the food chain, reducing the food quality due to phytotoxicity, and loss of soil fertility etc. (McLaughlin et al., 2000a,b).
In India, the heavy metal concentration in industrial areas is much higher than the permissible level as reported by World Health Organization (WHO), thus exposing humans to occupational hazards (Manivasagam, 1987). This scenario of the serious health hazards due to heavy metal pollution can be contributed to negligence of the industries in the form of direct discharge of untreated effluent into environment, failure to imply strict regulations by government environmental protection agencies in developing countries, and the non-reliability of the present individual remediation methods toward in situ and large scale applications.
Types of Integrated Processes
Chemical-Biological Remediation Approach
This process of chemical–biological integrated treatment is considered to be a highly economical and eco-friendly alternative to treat heavy metals containing wastewater. Implementation of this integrated treatment than the individual chemical or biological treatment has been reported to be advantageous and has shown significant results of heavy metal removal by many researchers worldwide (Rahman and Murthy, 2005; Abdulla et al., 2010; Rahman and Bastola, 2014; Greenwell et al., 2016; Mao et al., 2016; Pradhan et al., 2017). When implied alone, both the treatments face, their merits, and demerits. In case of chemical method of remediation, its simple operation and quick results have made this method as one of the most widely used remediation worldwide. However, the production of insoluble metal precipitates and toxic by-products has greatly limited this method (Fu and Wang, 2011). On the other hand, biological treatment is considered advantageous due to its environmental friendliness and economic feasibility. But their limitations include, long acclimatization time, changes in the biodegradable efficiency of the isolate and generation of sludge (Lohner and Tiehm, 2009). However, these limitations can be ruled out by integrating both the methods with a proper understanding of individual method's mechanism. Generally, this type of integrated system involve biological treatment followed by chemical treatment and vice-versa, that acts as a polishing step due to its effectiveness and economic feasibility as reported by few researchers (Ayres et al., 1994; Goswami and Mazumder, 2014). In one of the study by Ahmed et al. (2016), a combined approach of chemical precipitation and biological treatment toward Cr(VI) removal from tannery effluent was reported with a successful recovery of 99.3 and 98.4% of total Cr and Cr(VI), respectively. It was also shown to reduce 77% of chemical oxygen demand (COD) and 81% of turbidity. A similar study of combined process of chemical precipitation and biological system using Fusarium chlamydosporium was reported to reduce 64.69% of turbidity, 71.80% of COD, and 62.33% of total chromium (Sharma and Malaviya, 2014). Though this method has gained popularity among researchers, a responsible and an eco-friendly choice of non-toxic chemicals will surely aid in the success of this method.
Electro-Kinetic Microbial Remediation Approach
In this kind of remediation process, the organic matter is electrochemically converted to generate useful by-products, produce bioelectricity, and fuel by the action of microbial metabolic processes (Logan and Rabaey, 2012). As soil contains the majority of heavy metal in insoluble form, their removal rate was minimum, so the solubility can be achieved by coupling electrokinetic with other techniques. On the other hand, if the metal ion was in “soluble” form in the soil, then the remediation rate will be maximized. Based on these implications, electro-kinetic (EK) technique was introduced around 1980s and was widely employed to manage heavy metal contaminated fine-grain soils of low hydraulic conductivity (Maini et al., 2000). Here a direct electric current was used to remove fine and low permeability heavy metal particles from the soil with minimum disturbance to the surface. As voltage was applied between two sides of the electrolytic tank containing contaminated soil, an electric field gradient was created. This low-level electric current aid as a cleaning agent by stimulating the pollutants to transport toward recovery wells involving mechanisms such as electro-osmotic flow, electromigration, and electrophoresis thereby inducing electrochemical reactions (Acar and Alshawabkeh, 1993). The main advantage of this method is its simple operation, cost-effective, and no subsequent pollution (Zhou et al., 2004; Deng et al., 2009; Violetta and Sergio, 2009; Ma et al., 2010). But, EK method has also certain restrictions like, bioavailability of the heavy metal and mass transfer between the electrode and pollutants (Simoni et al., 2001; Lohner and Tiehm, 2009).
In order to increase its overcome these restrictions and to achieve high efficiency, an interesting idea of integrating EK remediation with biological method was used and got succeeded by many researchers. This integration was reported to promote increased bioavailability of the pollutants, enhancement in biodegradation efficiency by generating oxidization and reduction zones, releasing of soil/sediment bound pollutant, improved nutrient transport, improved performance, and availability of terminal electron acceptors (Maini et al., 2000; Luo et al., 2005; Wick, 2009; Kim et al., 2010; Peng et al., 2011; Selvi and Aruliah, 2018). As a biological counterpart, both acidophilic and alkalophilic microbes were employed. If the acidic bacterium is involved, it will favor EK, whereas the alkalophilic will aid in metal precipitation. In a few instances, few microbes may require additional nutrients as an energy source (glucose, starch etc.) to survive in the EK cell (Choi et al., 2013). Some of the interesting works on Bio-EK integrated system, implied by the scientific community were discussed in detail here. One such study by Rosestolato et al. (2015) on bio-electrokinetic method was reported in which, 400 kg of mercury contaminated soil was successfully remediated by with a maximum removal of 60%. In a study of EK assisted bioremediation carried out by Azhar et al. (2016a) removal of mercury from the contaminated soil was reported. Electrokinetic study was conducted using electric current of voltage 50V for a period of 7 days, which was followed by microbial remediation using Lysinibacillus fusiformis bacteria. The result concluded that higher removal rate of mercury to 78% was achieved within a shorter period of 7 days. In another study of zinc removal, EK assisted bioremediation using Pseudomonas putida showed 89% removal in 5 days (Azhar et al., 2016b).
With a future perspective of symbiotic combination strategies using electrochemically active bacterial cells and electrified interfaces, Varia et al. (2013) reported on bioelectrochemical remediation of gold, cobalt, and iron metal ions using gamma Proteobacteria, Shewanella putrefaciens CN32. Their demonstration concluded on microbial influenced electronation thermodynamics of the metal ion, with an outcome of prospective energy savings. A similar study by Kim et al. (2012) demonstrated removal of heavy metals such as arsenic, copper, and leads using an integrated system of bioelectrokinetics (bioleaching-electrokinetic). They have employed Acidithiobacillus ferrooxidans species to carry out bioleaching process as it was capable to oxidize the reduced sulfur and ferrous ions. This creates an acidic environment in the soil, which was reported to as a suitable condition for removal of heavy metals (Nareshkumar et al., 2008). Peng et al. (2011) too reported on significant reduction of 296.4 to 63.4 mg/Kg of Cu and 3,756 to 33.3 mg/kg of Zn in sewage sludge, within 10 days using an indigenous iron-oxidizing bacteria and EK remediation.
In this Bio-EK integrated remediation, bioleaching process was carried out initially to convert the metal to soluble form which favors a faster and higher rate of remediation in electrokinetic method a follow up process in bioelectrokinetics. From the obtained results they have concluded that the maximum removal of heavy metal was achieved with minimal power consumption, than used for conducting individual EK remediation. A similar bioelectrokinetics remediation work was reported by Huang et al. (2012) to remove copper, zinc, chromium and lead from the polluted soils. In this experiment, soil samples were collected and oxidized using iron containing bacterial species and the soil was further treated by electrokinetic method, by which, the metals will start to eliminate with change in the pH of soil. The corresponding elimination of metal ions of copper, zinc, chromium, and lead was monitored and reported with maximum removal rate.
Dong et al. (2013) used electrokinetic coupled biostimulation method to remove lead from Pb-oil co-contaminated soil. A pilot study was conducted for a period of 30 days in which surfactant (Tween 80) and chelating agents (EDTA) was added to enhance EK operating conditions. The addition of EDTA was found to play a role in eliminating the heavy metal toxicity in soil and this coupled technique reported 81.7% removal of lead from the soil.
Electrokinetic-Phytoremediation Approach
This is an emerging method of remediation that has proved to be more effective in terms of metal recovery and being more economical than the other integrated approaches discussed previously. This combination was initiated with the outstanding results of EK remediation and its compatible operation with phytoremediation (Figure 4). When phytoremediation is employed as an individual process, they may offer an economical solution, but, its in situ application is limited by climatic conditions, metal bioavailability, and shallow depths (Barber, 1995). The recovery yield and process rate also require a significant improvement. However, this can be enhanced by combining phytoremediation with different strategies like transgenic technology, bioaugmentation, remediation with electrokinetics, permeable reactive barrier (Cameselle et al., 2013). Laboratory studies on EK and phytoremediation approach has exhibited a respectable vision in heavy metal remediation of Zn, Pb, Cu, Cd, and As. Electrokinetics was also found to play an important role in phytoremediation. A direct current passed between electrodes which placed vertically in soil separates organic and inorganic molecules (Cao et al., 2003; Santos et al., 2008). Depending on the plant's uptake mechanisms, different strategies like, phytoextraction, phytoevaporation, phytostabilization, rhizodegradation, and rhizofiltration were employed for phytoremediation (Halim et al., 2003; Cui et al., 2007; Kotrba et al., 2009; Ghosh, 2010; Lotfy and Mostafa, 2014; Mao et al., 2016).
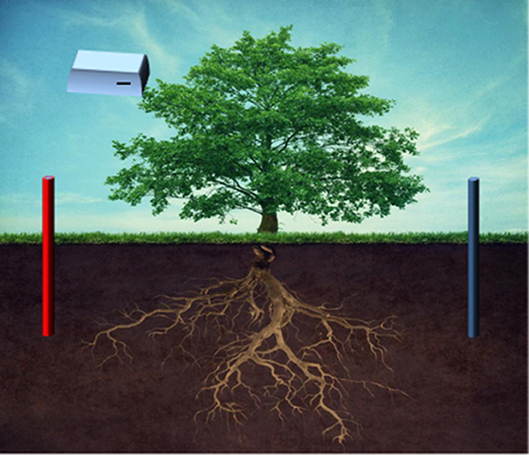
Figure 4. Schematic diagram of electro-kinetic coupled/enhanced phytoremediation (Source: Mao et al., 2016) Copyright 2018, Elsevier.
Bhargavi and Sudha (2015) used an electrokinetic assisted phytoremediation process to reduce the levels of chromium and cadmium. In their study, the samples were taken from Bharathi Nagar and Tandalam village of the Ranipet Industrial area. The collected samples were first remediated using EK method, followed by phytoremediation by extruding the remediated soil samples from the electrokinetic cell. The EK remediated soil was potted to grow the plant Brassica Juncea. For electrokinetic treatment, 50 V of electric current was applied and the removal rate was monitored at a regular interval of time from 5 to 25 days. They reported on 67.43 and 59.78% removal efficiency of cadmium and chromium after 25 days of treatment. This EK remediated soil, employed for phytoremediation showed a promising accumulation of cadmium and chromium in single harvest, which was further increased in subsequent harvests. A similar study was conducted by Lim et al. (2004) to remove lead from polluted soil using mustard plant. Compared to controls, the electric field assisted phytoremediation showed 2–4 times effective removal of lead in the soil. Cang et al. (2012) have remediated cadmium, copper, lead and zinc from the soil by using integrated methods of electrokinetic assisted phytoremediation. They concluded that the property of the soil was directly influenced by the voltage applied and the growth of plant increased the enzymatic activity of soil to achieve a maximum heavy metal remediation.
Electrokinetic coupled phytoremediation using species Lemna minor was tested by Kubiak et al. (2012) to remediate toxic arsenic in water. For this test, artificial arsenic water was prepared using sodium arsenate at a concentration of 150 μg L−1. Their preliminary results showed a higher removal rate of 90% at the end of the experiment. In an another study of lead removal from soil was reported by Hodko et al. (2000), in which the EK remediation was carried out by applying several electrode configurations to enhance phytoremediation by increasing the depth of soil to prevent the leaching of mobile metals on the ground surface.
Phyto-electrokinetic remediation under laboratory scale was studied by O'Connor et al. (2003) in which the soil samples were contaminated artificially with metal ions followed by the measuring of removal rate. One of the soil samples was contaminated by copper and the other by cadmium with arsenic. The test soils were filled in the reactor in two separate chambers. An electric current of 30 V was applied simultaneously by seeding with rye grass. A significant removal rate was reported over a period of 98 and 80 days for Cu and Cd-As soil, respectively. EK enhanced phytoextraction demonstrated by Mao et al. (2016) removed lead, arsenic and caesium from soil by lowering the pH of soil to 1.5, which resulted in dissolution of heavy metals to a larger extent with an increased solubility and bioavailability of heavy metals. It was then followed by phytoextraction using plants that enhanced the effectiveness of metal removal from the soil.
Phytobial Remediation Approach
Phytobial remediation is an efficient and eco-friendly solution to remove heavy metals from soil and water. Phytobial remediation utilizes plants as well as microbes to remove heavy metals from soil and water. As mentioned in literature, phytobial based remediation utilizes the plants to uptake the heavy metals and the microbes will help in degradation of those metallic substances (Lynch and Moffat, 2005). Figure 5 portrays different mechanism viz., (i) Bioprecipitation of metals, (ii) Bioaccumulation of metal by metal binding proteins, (iii) Binding of metals on the cell surface, (iv) Biotransformation of metals, (v) Methylation of metals, (vi) Solubilisation of metals, (vii) Biosorption of metals, (ix) Metal reduction, (x) Siderophores secretion, (xi) DNA-mediated interaction toward heavy metal removal (Ahemad, 2015). These mechanisms can be enhanced by integrating a suitable bacterium that can secrete multiple plant growth promoting substances (PGPS) (Martin and Ruby, 2004). These substances include organic acids, ACC deaminase, siderophores, and biosurfactants that will transform the metals into a bioavailable form (Roy et al., 2015). Table 7 summarizes the PGPS secreted by various phosphate solubilizing bacteria (PSB).
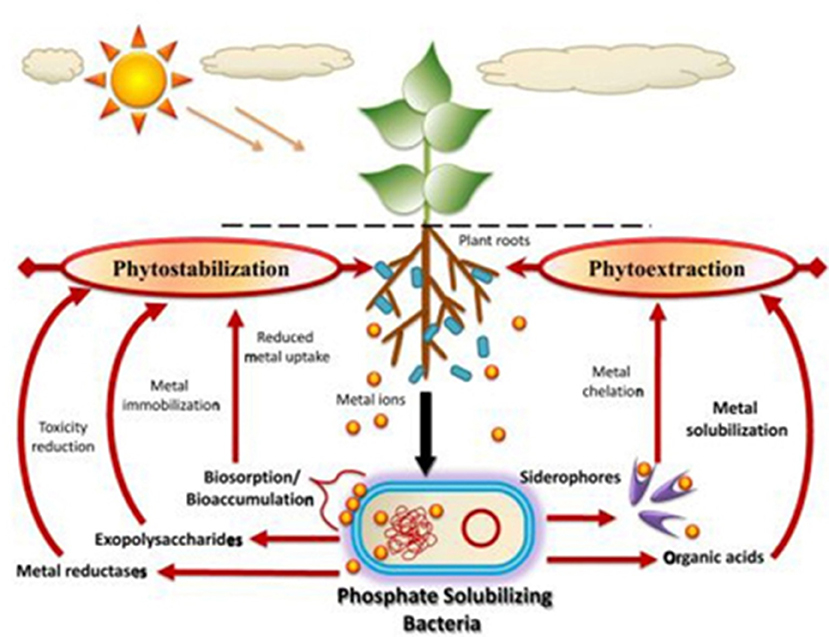
Figure 5. Various microbial interaction with heavy metals (Source: Ahemad, 2015) Copyright 2018, Springer Nature.
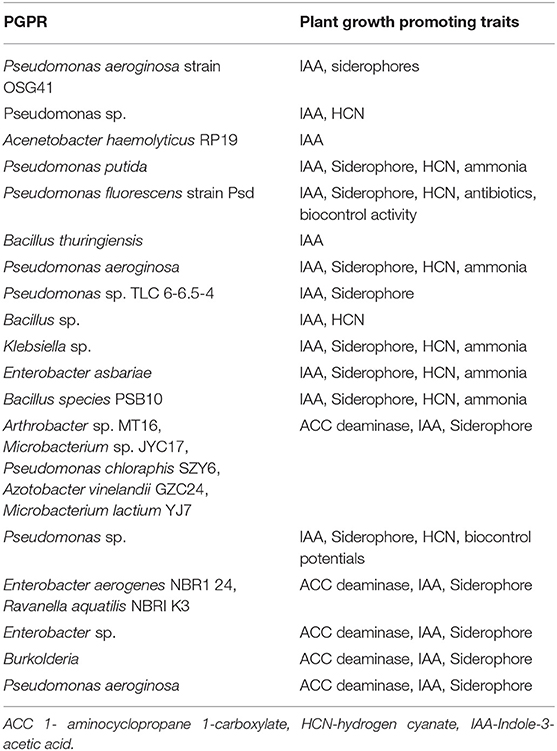
Table 7. Plant growth promoting substances realeased by phosphate solubilizing bacteria (Source: Ahemad, 2015), Copyright 2018 Springer Nature.
Phytobial remediation is recognized as cleanest and cheapest approach unlike other invasive technologies. It also has an advantage of being applied to vast areas of contaminated groundwater, soil and sediment. In addition, its in situ application option was found to decrease the heavy metal distribution in the soil and aids in preserving the top soil. Despite these advantages, this method is restricted to shallow aquifer and soil due to plant root length restriction, potential fear of transfer of heavy metals to the food chain, long duration (may require several seasons), regular monitoring (due to litter fall), lack of safe proper disposal method, tough metal recovery procedures, and high recycle economy. Roy et al. (2015) has offered few solutions to overcome these issues by using deep rooted plants, designing of transgenic plants that distracts herbivores, development of suitable evaluation methods, to integrate with other methods like bioremediation, EK, and bioaugmentation, etc. Different types of microbes involved in phytobial remediation are discussed here in detail.
Phytobial Remediation Using Free Living Organism
Free living microbes assist phytoremediation by mobilization, immobilization, and volatilization. Mobilization of metals occurs by different reactions such as volatilization, redox transformation, leaching, and chelation. The microbes like Sulfurospirillum barnesii, Geobacter, and Bacillus selenatarsenatis are used for arsenic removal. Lee et al. (2009) developed a hybrid method by using anaerobic bioleaching and electrokinetics. The plant used for phytoremediation accumulates heavy metals as harvested tissue, which can be disposed off. Introducing mobilizing microbes into contaminated water speed up the process of heavy metal accumulation (Wang et al., 2005). During the immobilization process, the mobility of the contaminant is prohibited by altering the physical and chemical properties (Leist et al., 2000). The oxidase enzymes present in the microbes oxidize the metals and make them immobilize and less toxic. The microbes such as Sporosarcina ginsengisoli, Candida glabrata, Bacillus cereus, and Aspergillus niger were used in immobilization technique to remove heavy metals (Littera et al., 2011; Giri et al., 2012). In a biotransformation process, a large number of bacteria, fungi, and algae were employed in heavy metal removal using biomethylation process (Frankenberger and Arshad, 2002).
Endophyte Remediation
Certain bacteria and fungi that live within the plants are called endophytes. They live within the plant for at least a part of their life cycle without damaging the host. They are ubiquitously associated with most of the plant, of which some can promote plant growth (Ryan et al., 2008). Few fungal endophytes will produce secondary metabolites too. Methylobacterium strains from Pteris vittata herb was reported to exhibit heavy metal tolerance (Dourado et al., 2012). However, this endophyte remediation needs more research to explore the potential of unstudied endophytobiome.
Rhizomicrobe Remediation
Rhizosphere refers to the root region of the plant. Certain microbes present in this region forms a symbiotic association with the plant by secreting exudates, secretions, mucilages, mucigel, and lysates that help in plant growth (Kirk et al., 1999). For example, siderophores secreted by microbes will help in chelation and solubilisation of metals. Based on these secretions, rhizo-remediation can induce plant growth, immobilize heavy metals, and accumulation of metals. Siderophores having different ligand binding groups can bind to different metals. Siderophores produced by Pseudomonas azotoformans reported to mobilize and remove arsenic (Díaz de Villegas et al., 2002). Since the root microbes are aerobic in nature, the increased pH at the rhizosphere zone favors the mobilization and uptake of heavy metals. The increased pH is due to the cation and the anion uptake ratio in rhizospheric region (Nair et al., 2007). Yang et al. (2012) reported that the plant-microbial consortium secretes biosurfactants that helps in immobilizing metals by increasing the pH of the rhizosphere.
Fungal Phytoremediation
Many plants have an association with mycorrhizal fungi which increase the surface area of plant roots and help them to get more water and nutrients (Sylvia et al., 2005). Recent studies demonstrated that the mycorrhizal fungi can enhance the accumulation and uptake of heavy metals by plants. Glomus mosseae, Glomus geosporum, and Glomus etunicatum are mycorrhizal fungi present in Plantago lanceolata L, that were reported to enhance arsenic (As) accumulation by few researchers (Wu et al., 2009; Orłowska et al., 2012).
Algal Phytoremediation
Algae are regarded as an important component of aquatic system that plays a significant role in bio-geochemical cycle. It has received immense attention of researchers worldwide due to their exceptional absorption and sequestration capability. It also possesses high tolerance to heavy metals, selective removal, ability to grow both autotrophically and heterotrophically, synthesis of metallothioneins and phytochelatins, and can serve as potential agents for genetic alterations (Hua et al., 1995). Algal species such as microalgae (e.g., Dunaliella salina), macroalgae (Ulva sp., Enteromorpha sp., Cladophora sp., and Chaetomorpha sp), green algae (Enteromorpha, Cladophora), and brown algae (Fucus serratus) were extensively reported to accumulate appreciable quantities various heavy metals (Rainbow, 1995; Gosavi et al., 2004; Al-Homaidan et al., 2011). Aquatic plants such as Eichhornia crassipes, Pistia stratiotes, Colocasia esculenta, Spirodela polyrhiza, and Lemna minor have also been widely studied toward heavy metal remediation.
Enhanced Phytoremediation Approaches
It is quite obvious that an extensive technology is needed to remove heavy metals from the environment to bring them down to the permissible levels. Though it can be achieved by various integrated processes as discussed above, recombinant genetic engineering of bacteria and plants has also proved to be worthy in terms of heavy metal removal applications. Microbes have tremendous remediation potential when they are subjected to genetic modification, by which they can perform better than the wild type. Similarly, phytoremediation can also be triggered by genetic engineering to enhance the accumulation and uptake of heavy metals. The “ars” operon in “arsR” gene code for a regulatory protein which aid in sensing arsenic contamination. Kostal et al. (2004) prepared a recombinant E. coli with “ars,” gene which accumulated 60-fold higher level of arsenic than the control organism. “Ars” operon incorporated recombinant strain is best suited for in situ remediation option to perform bioremediation under real conditions (Ryan et al., 2007). Transgenic canola plants incorporated with Enterobacter cloacae CAL2 has accumulated four times more heavy metals than the control cells. Introduction of transgenic plant was reported to enhance the capacity of the plant toward heavy metal removal from soil (Eapen et al., 2003).
Other Integrated Approaches
With the successful remediation of the integrated processes discussed above, there are few other novel research attempts on integrated processes. Jones (1996) was the first person to conduct an electrokinetic-geosynthetic approach to remove metals from the contaminated soil. Geosynthetic material increases the mobility of pollutants and so the remediation rate using electric current will also be increased. This method was also proven to be successful for heavy metal removal from the soil. An integrated approach of using permeable reactive barrier along with microbes is a technique where the dissolved contaminants filter out as it flows. The removal occurs when the contaminated water flows through the permeable reactive barrier treated area in its flow path (Köber et al., 2005). This material is incorporated with microbes and/or plants which have the capability to absorb heavy metal present in ground water. Peng et al. (2015) have conducted integrated electrokinetic remediation coupled with membrane filtration to reduce the level of iron, zinc and calcium. They have made a comparative study and reported on the nanofiber assisted removal, which showed a maximum efficiency of metal ion removal than the individual electrokinetic method. An electric voltage of 25 V and 50 V were applied to carry out the electrokinetic study followed by filtration using polyacrylonitrile nanofiber (PANN) membrane. The removal rates of Zn2+, Fe3+ and Ca2+ were about 99.15, 98.03, and 99.73%, respectively. Vocciante et al. (2016) have conducted electrokinetic coupled soil washing to remediate heavy metal as it will convert insoluble metal ions in the soil to mobile forms and thereby facilitating the rate of metal removal to a greater extent. Using this coupled technique heavy metals such as antimony, arsenic, cadmium, chromium and mercury was removed effectively. The process occurs based on in situ soil washing. However, this technique needs to be validated in large scale (Aboughalma et al., 2008).
Future Projections
Implementation of biotechnological approaches is gaining increasing prominence in the field of remediation, as they are often considered as a promising strategy for the eventual treatment of contaminated sediments. As far as heavy metal removal is concerned, a detailed understanding of metal-induced mechanisms are imperative to devise an effective remediation option, because the heavy metals are known to cause serious health implications such as fertility impairment, genetic, epigenetic, and biochemical alterations as discussed in above sections of this review (Rzymski et al., 2015). This is due to the complexity and uniqueness of the contaminated sites caused by heavy metals.
Remediation methods in general use include physical separation, isolation, immobilization, toxicity reduction, and extraction. But, implementation of two or more techniques in a synergistic mode had resulted in better results, which were quite evident with the results discussed in the present review. Based on the wide literary review, any integrated processes involving EK processes had shown promising results. However, the more research focus is needed on the right remedial option that can challenge in situ operative conditions such as site characteristics (geographical location, pH levels, particle size, clay, soil type, depth, water content, climate, types of co-contaminants, etc.). Hence, remediation projects of the future should be capable of assessing the ecological impact, an important environmental criterion. And research innovations in terms of more integrated processes are in great demand. Owing to their wide application, effectiveness, and economic feasibility, few processes viz., phytobial remediation, chelate extraction, and chemical soil washings processes needs more research evaluations. Therefore, more attention should be paid to the evaluation methods for assessing the remediation effectiveness while developing new remediation technologies in future research. Above all, a strict implementation of standard regulations by government agencies and stern action against industries that are responsible for toxic environmental discharges will certainly make a noticeable change in levels of heavy metals in the environment.
Conclusion
This review discusses on different sources, need for removal, and related health hazards due to heavy metal in the environment. From the study, it is quite obvious that the anthropogenic activities have been significantly contributing to high concentrations of heavy metal discharge into the environment. Therefore, a serious and strict monitoring of these activities is suggested as an effective solution to address heavy metal pollution. However, a complete background knowledge on the sources of heavy metal, their chemistry, and potential risks posed to environment and humans are needed to select an appropriate remedial option. In this regard, many research investigations of various integrated options that are available for heavy metal removal/recovery from the contaminated environment are systematically summarized in this review. Based on our reviewed literature, processes with an integrated approaches were found to a serve as an effective alternative for removal of toxic heavy metals and recovery of valuable metals from highly contaminated industrial sites. Therefore, we conclude that the integrated processes involving EK processes and phyto-remediation had shown astonishing results of considerable reduction in the level and toxicity of heavy metals, with minimal disturbance to the natural environment. We also believe that these integrated technologies can be highly applicable for in situ operations in both developed and developing countries where, urbanization, agriculture, and industrialization are leaving an inheritance of environmental degradation.
Author Contributions
All authors listed have made a substantial, direct and intellectual contribution to the work, and approved it for publication.
Conflict of Interest Statement
The authors declare that the research was conducted in the absence of any commercial or financial relationships that could be construed as a potential conflict of interest.
Acknowledgments
The authors acknowledges Science and Engineering Research Board (SERB), Department of Science and Technology (DST), Government of India for funding this work under N-PDF scheme (File no. PDF/2016/002558).
References
Abdulla, H. M., Kamal, E. M., and Hassan, A. (2010). “Chromium removal from tannery wastewater using chemical and biological techniques aiming zero discharge of pollution,” in Proceedings of 5th, Science Environment Conference (Zagazig), 171–183.
Aboughalma, H., Bi, R., and Schlaak, M. (2008). Electrokinetic enhancement on phytoremediation in Zn, Pb, Cu and Cd contaminated soil using potato plants. J. Environ. Sci. Health A Tox. Hazard. Subst. Environ. Engg. 43, 926–933. doi: 10.1080/10934520801974459
Acar, Y. B., and Alshawabkeh, A. N. (1993). Principles of electrokinetic remediation. Environ. Sci. Technol. 27, 2638–2647. doi: 10.1021/es00049a002
Ahemad, M. (2015). Phosphate-solubilizing bacteria-assisted phytoremediation of metalliferous soils: a review. 3 Biotech 5, 111–121. doi: 10.1007/s13205-014-0206-0
Ahmed, E., Abdulla, H. M., Mohamed, A. H., and El-Bassuony, A. D. (2016). Remediation and recycling of chromium from tannery wastewater using combined chemical–biological treatment system. Process Saf. Environ. Protect. 104, 1–10. doi: 10.1016/j.psep.2016.08.004
Aksu, Z., and Kutsal, T. (1990). A comparative study for biosorption characteristics of heavy metals ions with C. vulgaris. Environ. Technol. 11, 979–987. doi: 10.1080/09593339009384950
Al-Homaidan, A. A., Al-Ghanayem, A. A., and Areej, A. H. (2011). Green algae as bioindicators of heavy metal pollution in wadi hanifah stream, Riyadh, Saudi Arabia. Internat. J Water Res. Arid Environ. 1:10.
Alloway, B. J. (1995). Heavy Metals in Soils, 2nd ed. London: Blackie Academic and Professional. doi: 10.1007/978-94-011-1344-1
Al-Rashdi, B., Somerfield, C., and Hilal, N. (2011). Heavy metals removal using adsorption and nanofiltration techniques. Sep. Purif. Rev. 40, 209–259. doi: 10.1080/15422119.2011.558165
Atkinson, B. W., Bux, F., and Kasan, H. C. (1998). Considerations for application of biosorption technology to remediate metal-contaminated industrial effluents. Water SA. 24, 129–135.
Awashthi, S. K. (2000). Prevention of Food Adulteration Act no. 37 of 1954. Central and State Rules as Amended for 1999. Ashoka Law House, New Delhi.
Ayres, R. U., Ayres, L. W., and Tarr, J. A. (1994). “A historical reconstruction of carbon monoxide and methane emissions in the United States, 1880–1980,” in Industrial Metabolism. Restructuring for Sustainable Development, eds R. U. Ayres, et al (Tokyo: United Nations University Press), 194–238.
Azhar, A. T. S., Nabila, A. T. A., Nurshuhaila, M. S., Shaylinda, M. Z. N., and Azim, M. A. M (2016b). Electromigration of contaminated soil by electrobioremediation technique. Soft soil engineering international conference, 2015 (SEIC2015), IOP Publishing IOP Conf. Series. Mater. Sci. Engg. 136, 1–5. doi: 10.1088/1757-899X/136/1/012023
Azhar, A. T. S., Nabila, A. T. A., Nurshuhaila, M. S., Zaidi, E., Azim, M. A. M., and Farhana, S. M. S. (2016a). Assessment and comparison of electrokinetic and electrokinetic bioremediation techniques for mercury contaminated soil. International engineering research and innovation symposium (IRIS) IOP Publishing IOP Conf. Series. Mater. Sci. Engg. 160, 1–8. doi: 10.1088/1757-899X/160/1/012077
Barber, S. A. (1995). Soil Nutrient Bioavailability: A Mechanistic Approach, 2nd ed. New York, NY: John Wiley and Sons Inc.
Bhargavi, V. L. N., and Sudha, P. N. (2015). Removal of heavy metal ions from soil by electrokinetic assisted phytoremediation method. Internat. J. ChemTech Res. 8, 192–202.
Cameselle, C., Chirakkara, R. A., and Reddy, K. R. (2013). Electrokinetic-enhanced phytoremediation of soils, status and opportunities. Chemosphere 93, 626–636. doi: 10.1016/j.chemosphere.2013.06.029
Cang, L., Zhou, D. M., Wang, Q. Y., and Fan, G. P. (2012). Impact of electrokinetic-assisted phytoremediation of heavy metal contaminated soil on its physicochemical properties, enzymaticand microbial activities. Electrochim. Acta 86, 41–48. doi: 10.1016/j.electacta.2012.04.112
Cao, X. D., Ma, L. Q., and Shiralipour, A. (2003). Effects of compost and phosphate amendments on arsenic mobility in soils and arsenic uptake by the hyperaccumulator Pteris vittata L. Environ. Pollut. 126, 157–167. doi: 10.1016/S0269-7491(03)00208-2
Chen, S., Chao, L., Sun, L. N., and Sun, T. H. (2013). Plant-microorganism combined remediation for sediments contaminated with heavy metals. Adv. Mater. Res. 123, 610–613. doi: 10.4028/www.scientific.net/AMR.610-613.1223
Choi, J. H., Maruthamuthu, S., Lee, Y. J., and Alshawabkeh, A. N. (2013). Reduction of nitrate in agricultural soils by bio-electrokinetics, soil and sediment contamination. Internat. J. 22, 767–782. doi: 10.1080/15320383.2013.768202
Colbeck, I. (1995). “Particle emission from outdoor and indoor sources,” in Airborne Particulate Matter. The Handbook of Environmental Chemistry, Vol. 4/4D, eds T. Kouimtzis and C. Samara (Berlin; Heidelberg: Springer), 1–33. doi: 10.1007/978-3-540-49145-3_1
Cui, S., Zhou, Q. X., and Chao, L. (2007). Potential hyperaccumulation of Pb, Zn, Cu and Cd in endurant plants distributed in an old smeltery, Northeast China. Environ. Geol. 51, 1043–1048. doi: 10.1007/s00254-006-0373-3
D'amore, J. J., Al-Abed, S. R., Scheckel, K. G., and Ryan, J. A. (2005). Methods for speciation of metals in soils. J. Environ. Qual. 34, 1707–1745. doi: 10.2134/jeq2004.0014
Darby, D. A., Adams, D. D., and Nivens, W. T. (1986). “Sediment and water interaction,” in Early Sediment Changes and Element Mobilization in a Man-made Estuarine Marsh, ed P. G. Sly (Berlin; Heidelberg; New York, NY: Springer), 343–351. doi: 10.1007/978-1-4612-4932-0_29
De Villiers, P. G. R., Van.Deventer, J. S. J., and Lorenzen, L. (2005). The extraction of species from slurries of insoluble solids with ion-exchange resins. Miner. Engg. 8, 1309–1326. doi: 10.1016/0892-6875(95)00098-B
Deng, J. C., Xin, F., and Qiu, X. H. (2009). Extraction of heavy metal from sewage sludge using ultrasound-assisted nitric acid. Chem. Eng. J. 152, 177–182. doi: 10.1016/j.cej.2009.04.031
Díaz de Villegas, M. E., Villa, P., and Frías, A. (2002). Evaluation of the siderophores production by Pseudomonas aeruginosa PSS. Rev. Latinoam Microbiol. 44, 112–117.
Dong, Z. Y., Huang, W. H., Xing, D. F., and Zhang, H. F. (2013). Remediation of soil co-contaminated with petroleum and heavy metals by the integration of electrokinetics and biostimulation. J. Hazard. Mater. 15, 399–408. doi: 10.1016/j.jhazmat.2013.05.003
Dourado, M. N., Ferreira, A., Araújo, W. L., Azevedo, J. L., and Lacav, P. T. (2012). The diversity of endophytic methylotrophic bacteria in an oil-contaminated and an oil-free mangrove ecosystem and their tolerance to heavy metals. Biotechnol. Res. Internat. 2012:759865. doi: 10.1155/2012/759865
E.P.A (1992). Framework for Ecological Risk Assessment. EPA/630/R-92/001. Risk Assessment Forum, U.S. Environmental Protection Agency, Washington, DC.
E.P.A (2002). Environmental Protection Agency, Office of Groundwater and Drinking Water. Implementation Guidance for the Arsenic Rule. EPA Report 816-D-02-005, Cincinnati, OH.
Eapen, S., Suseelan, K., Tivarekar, S., Kotwal, S., and Mitra, R. (2003). Potential for rhizofiltration of uranium using hairy root cultures of Brassica juncea and Chenopodium amaranticolor. Environ. Res. 91, 127–133. doi: 10.1016/S0013-9351(02)00018-X
Feng, Y., Gong, J. L., Zeng, G. M., Niu, Q. Y., Zhang, H. Y., and Niu, C. G. (2010). Adsorption of Cd (II) and Zn (II) from aqueous solutions using magnetic hydroxyapatite nanoparticles as adsorbents. Chem. Eng. J. 162, 487–494. doi: 10.1016/j.cej.2010.05.049
Fraissler, G., Jöller, M., Mattenberger, H., Brunner, T., and Obernberger, I. (2009). Thermodynamic equilibrium calculations concerning the removal of heavy metals from sewage sludge ash by chlorination. Chem. Engg. Process Proces Intensification 48, 152–164. doi: 10.1016/j.cep.2008.03.009
Frankenberger, W., and Arshad, M. (2002). “Volatilization of arsenic,” in Environmental Chemistry of Arsenic, ed W. Frankenberger (Boca Raton, FL: Marcel Dekker), 363–380. doi: 10.1201/9781482271102
Fu, F., and Wang, Q. (2011). Removal of heavy metal ions from wastewaters: a review. J. Environ. Manage. 92, 407–418. doi: 10.1016/j.jenvman.2010.11.011
Garbarino, J. R., Hayes, H. C., Roth, D. A., Antweiler, R. C., Brinton, T. I., and Taylor, H. E. (1995). “Heavy metals in the Mississippi river,” in Contaminants in the Mississippi River, ed R. H. Meade (Reston, VA: U.S. Geological Survey Circular 1133).
Ghosh, S. (2010). Wetland macrophytes as toxic metal accumulators. Int. J. Environ. Sci. 1, 523–528.
Giri, A. K., Patel, R. K., and Mishra, P. C. (2012). Biosorption of As(V) from aqueous solutions by living cells of Bacillus cereus. Water Sci. Technol. 66, 699–707. doi: 10.2166/wst.2012.332
Gomez-Eyles, J. L., Yupanqui, C., Beckingham, B., Riedel, G., Gilmour, C., and Ghosh, U. (2013). Evaluation of biochars and activated carbons for in situ remediation of sediments impacted with organics, mercury, and methylmercury. Environ. Sci. Technol. 47, 13721–13729. doi: 10.1021/es403712q
Gómez-Sagasti, M. T., Alkorta, I., Becerril, J. M., Epelde, L., Anza, M., and Garbisu, C. (2012). Microbial monitoring of the recovery of soil quality during heavy metal phytoremediation. Water Air Soil Pollut. 223, 3249–3262. doi: 10.1007/s11270-012-1106-8
Gosavi, K., Sammut, J., Gifford, S., and Jankowski, J. (2004). Macroalgal biomonitors of trace metal contamination in acid sulfate soil aquaculture ponds. Sci. Total Environ. 25, 25–39. doi: 10.1016/j.scitotenv.2003.11.002
Goswami, S., and Mazumder, D. (2014). Scope of biological treatment for composite tannery wastewater. Int. J. Environ. Sci. 5, 1–6.
Goyer, R. A. (2001). “Toxic effects of metals,” in Cassarett and Doull's Toxicology: The Basic Science of Poisons, C. D. Klaassen (New York, NY: McGrawHill Publisher), 811–867.
Greenwell, M., Sarker, M., and Rahman, P. K. S. M. (2016). Biosurfactant production and biodegradation of leather dust from tannery. Open Biotechnol. J. 10(Suppl. 2), 312–325. doi: 10.2174/1874070701610010312
Halim, M., Conte, P., and Piccolo, A. (2003). Potential availability of heavy metals to phytoextraction from contaminated soils induced by exogenous humic substances. Chemosphere 52, 265–275. doi: 10.1016/S0045-6535(03)00185-1
Hashim, M. A., Mukhopadhyay, S., Sahu, J. N., and Sengupta, B. (2011). Remediation technologies for heavy metal contaminated groundwater. J. Environ. Manage. 92, 2355–2388. doi: 10.1016/j.jenvman.2011.06.009
Hassanien, M. A. (2011). “Atmospheric heavy metals pollution: exposure and prevention policies in mediterranean basin,” in Environmental Heavy Metal Pollution and Effects on Child Mental Development. NATO Science for Peace and Security Series C: Environmental Security, 1, eds L. Simeonov, M. Kochubovski, and B. Simeonova (Dordrecht: Springer).
He, Z. L., Yang, X. E., and Stoffella, P. J. (2005). Trace elements in agroecosystems and impacts on the environment. J. Trace Elem. Med. Biol. 19, 125–140. doi: 10.1016/j.jtemb.2005.02.010
Herawati, N., Suzuki, S., Hayashi, K., Rivai, I. F., and Koyoma, H. (2000). Cadmium, copper and zinc levels in rice and soil of Japan, Indonesia and China by soil type. Bull. Environ. Contam. Toxicol. 64, 33–39. doi: 10.1007/s001289910006
Hodko, D., Hyfte, J. V., Denvir, A., and Magnuson, J. W. (2000). Methods for enhancing phytoextraction of contaminants from porous media using electrokinetic phenomena. US Patent No 6,145–244.
Hotz, P., Buchet, J. P., Bernard, A., Lison, D., and Lauwerys, R. (1999). Renal effects of low level environmental cadmium exposure: 5-year follow-up of a subcohort from the Cadmibel study. Lancet 354, 1508–1513. doi: 10.1016/S0140-6736(99)91145-5
Hu, X. J., Wang, J. S., Liu, Y. G., Li, X., Zeng, G. M., and Bao, Z. L. (2011). Adsorption of chromium (VI) by ethylenediamine-modified cross-linked magnetic chitosan resin: isotherms, kinetics and thermodynamics. J. Hazard. Mater. 185, 306–314. doi: 10.1016/j.jhazmat.2010.09.034
Hua, C. X., Traina, S. J., Logan, T. J., Gustafson, T., Sayre, R. T., and Cai, X. H. (1995). Applications of eukaryotic algae for the removal of heavy metals from water. Mol. Mar. Biol. Biotechnol. 4, 338–344.
Huang, D., Xu, Q., Cheng, J., Lu, X., and Zhang, H. (2012). Electrokinetic remediation and its combined technologies for removal of organic pollutants from contaminated soils. Int. J. Electrochem. Sci. 7, 4528–4544.
IARC (1993). “Cadmium and cadmium compounds,” in Beryllium, Cadmium, Mercury and Exposure in the Glass Manufacturing Industry. IARC Monographs on the Evaluation of Carcinogenic Risks to Humans, 58. Lyon: International Agency for Research on Cancer, 119–237.
Jaishankar, M., Tseten, T., Anbalagan, N., Mathew, B. B., and Beeregowda, K. N. (2014). Toxicity, mechanism and health effects of some heavy metals. Interdiscip. Toxicol. 7, 60–72. doi: 10.2478/intox-2014-0009
Järup, L. (2003). Hazards of heavy metal contamination. Br. Med. Bull. 68, 167–182. doi: 10.1093/bmb/ldg032
Jones, C. J. E. P. (1996). Earth Reinforcement and Soil Structures. London: Thomas Telford Publishing, 379.
Kalavathy, S. (2004). The Multidisciplinary nature of environmental studies. Environ. Stud. 1, 1–13.
Kaonga, C. C., Kosamu, I. B., Lakudzala, D. D., Mbewe, R., Thole, B., Monjerezi, M., et al. (2017). A review of heavy metals in soil and aquatic systems of urban and semi-urban areas in Malawi with comparisons to other selected countries. Afr. J Environ. Sci. Technol. 11, 448–460. doi: 10.5897/AJEST2017.2367
Khalid, S., Shahid, M., Khan, N., Murtaza, N. B., Bibi, I., and Dumat, C. (2017). A comparison of technologies for remediation of heavy metal contaminated soils.J. Geochem. Explor. 182, 247–268. doi: 10.1016/j.gexplo.2016.11.021
Khan, S., Cao, Q., Zheng, Y. M., Huang, Y. Z., and Zhu, Y. G. (2008). Health risks of heavy metals in contaminated soils and food crops irrigated with wastewater in Beijing, China. Environ. Poll. 152, 686–692. doi: 10.1016/j.envpol.2007.06.056
Kim, H. A., Lee, K. Y., Lee, B. T., Kim, S. O., and Kim, K. W. (2012). Comparative study of simultaneous removal of As, Cu, and Pb using different combinations of electrokinetics with bioleaching by Acidithiobacillus ferrooxidans. Water Res. 46, 5591–5599. doi: 10.1016/j.watres.2012.07.044
Kim, S. H., Han, H. Y., Lee, Y. J., Kim, C. W., and Yang, J. W. (2010). Effect of electrokinetic remediation on indigenous microbial activity and community with in diesel contaminated soil. Sci. Total Environ. 408, 3162–3168. doi: 10.1016/j.scitotenv.2010.03.038
Kirk, G. J. D., Santos, E. E., and Findenegg, G. R. (1999). Phosphate solubilisation by organic anion excretion from rice (Oryza sativa L.) growing in aerobic soil. Plant Soil 211, 11–18. doi: 10.1023/A:1004539212083
Köber, R., Daus, B., Ebert, M., Mattusch, J., Welter, E., and Dahmke, A. (2005). Compost-based permeable reactive barriers for the source treatment of arsenic contaminations in aquifers: column studies and solid-phase investigations. Environ. Sci. Technol. 39, 7650–7655. doi: 10.1021/es0503579
Kostal, J., Yang, R., Wu, C. H., Mulchandani, A., and Chen, W. (2004). Enhanced arsenic accumulation in engineered bacterial cells expressing ArsR. Appl. Environ. Microbiol. 70, 4582–4587. doi: 10.1128/AEM.70.8.4582-4587.2004
Kotrba, P., Najmanova, J., Macek, T., Ruml, T., and Mackova, M. (2009). Genetically modified plants in phytoremediation of heavy metal and metalloid soil and sediment pollution. Biotechnol. Adv. 27, 799–810. doi: 10.1016/j.biotechadv.2009.06.003
Kubiak, J. J., Khankhane, P. J., Kleingeld, P. J., and Lima, A. T. (2012). An attempt to electrically enhance phytoremediation of arsenic contaminated water. Chemosphere 87, 259–64. doi: 10.1016/j.chemosphere.2011.12.048
Lee, K. Y., Yoon, I. H., Lee, B. T., Kim, S. O., and Kim, K. W. (2009). A novel combination of anaerobic bioleaching and electrokinetics for as removal from mine tailing soil. Environ. Sci. Technol. 43, 9354–9360. doi: 10.1021/es901544x
Leist, M., Casey, R. J., and Caridi, D. (2000). The management of As wastes, problems and prospects. J. Hazard. Mater. 76, 125–138. doi: 10.1016/S0304-3894(00)00188-6
Lenntech Water treatment and Air purification (2004). Water Treatment. Lenntech, Rotterdamseweg, Netherlands.
Lim, J. M., Salido, A. L., and Butcher, D. J. (2004). Phytoremediation of lead using Indian mustard (Brassica juncea) with EDTA and electrodics. Microchem. J. 76, 3–9. doi: 10.1016/j.microc.2003.10.002
Lin, S. H., and Kiang, C. D. (2003). Chromic acid recovery from waste acid solution by an ion exchange process: equilibrium and column ion exchange modeling. Chem. Eng. J. 92, 193–199. doi: 10.1016/S1385-8947(02)00140-7
Littera, P., Urík, M., Sevc, J., Kolencík, M., Gardosová, K., and Molnárová, M. (2011). Removal of arsenic from aqueous environments by native and chemically modified biomass of Aspergillus niger and Neosartorya fischeri. Environ. Technol. 32, 1215–1222. doi: 10.1080/09593330.2010.532510
Logan, B. E., and Rabaey, K. (2012). Conversion of wastes into bioelectricity and chemicals by using microbial electrochemical technologies. Science 337, 686–690. doi: 10.1126/science.1217412
Lohner, S. T., and Tiehm, A. (2009). Application of electrolysis to stimulate microbial reductive PCE dechlorination and oxidative VC biodegradation. Environ. Sci. Technol. 18, 7098–7104. doi: 10.1021/es900835d
Lotfy, S. M., and Mostafa, A. Z. (2014). Phytoremediation of contaminated soil with cobalt and chromium. J. Geochem. Explor. 144, 367–373. doi: 10.1016/j.gexplo.2013.07.003
Luo, Q., Wang, H., Zhang, X., and Qian, Y. (2005). Effect of direct electric current on the cell surface properties of phenol-degrading bacteria. Appl. Environ. Microbiol. 71, 423–427. doi: 10.1128/AEM.71.1.423-427.2005
Lynch, J. M., and Moffat, A. J. (2005). Bioremediation-prospects for the future application of innovative applied biological research. Ann. Appl. Biol. 146:217. doi: 10.1111/j.1744-7348.2005.040115.x
Ma, J. W., Wang, F. Y., Huang, Z. H., and Wang, H. (2010). Simultaneous removal of 2,4-dichlorophenol and Cd from soils by electrokinetic remediation combined with activated bamboo charcoal. J. Hazard. Mater. 176, 715–720. doi: 10.1016/j.jhazmat.2009.11.093
Maini, G., Sharman, A. K., Knowles, C. J., Sunderland, G., and Jackman, S. A. (2000). Electrokinetic remediation of metals and organics from historically contaminated soil. J. Chem. Technol. Biotechnol. 75, 657–664. doi: 10.1002/1097-4660(200008)75:8<657::AID-JCTB263>3.0.CO;2-5
Mandel, J. S., McLaughlin, J. K., Schlehofer, B., Mellemgaard, A., Helmert, U., Lindblad, P., et al. (1995). International renal-cell cancer study. IV. Occupation. Internat. J. Cancer 61, 601–605. doi: 10.1002/ijc.2910610503
Manivasagam, N. (1987). Industrial Effluents Origin; Characteristics Effects, Analysis and Treatment. Coimbatore: Shakti Publications, 79–92.
Manzetti, S., Spoel, E. R., and Spoel, D. (2014). Chemical properties, environmental fate, and degradation of seven classes of pollutants. Chem. Res. Toxicol. 27, 713–737. doi: 10.1021/tx500014w
Mao, X., Han, F. X., Shao, X., Guo, K., McComb, J., Arslan, Z., et al. (2016). Electro-kinetic remediation coupled with phytoremediation to remove lead, arsenic and cesium from contaminated paddy soil. Ecotoxicol. Environ Saf. 125, 16–24. doi: 10.1016/j.ecoenv.2015.11.021
Marinos, A. S., Demetra, K., Katherine-Joanne, H., Vassilis, J. I., Konstantinos, G. M., and Maria, D. L. (2007). Effect of acid treatment on the removal of heavy metals from sewage sludge. Desalination 215, 73–81. doi: 10.1016/j.desal.2006.11.015
Martin, T. A., and Ruby, M. V. (2004). Review of in situ remediation technologies for lead, zinc, and cadmium in soil. Remed. J. 14, 35–53. doi: 10.1002/rem.20011
McLaughlin, M. J., Hamon, R. E., McLaren, R. G., Speir, T. W., and Rogers, S. L. (2000b). A bioavailability-based rationale for controlling metal and metalloid contamination of agricultural land in Australia and New Zealand. Soil Res. 38, 1037–1086. doi: 10.1071/SR99128
McLaughlin, M. J., Zarcinas, B. A., Stevens, D. P., and Cook, N. (2000a). Soil testing for heavy metals. Comm. Soil Sci. Plant Anal. 31, 1661–1700. doi: 10.1080/00103620009370531
Mitchell, R. L. (1964). “Chemistry of the soil,” in Trace Elements in Soil, ed F. E. Bear (New York, NY: Reinhold Publishing Corp.), 320–368.
Moore, J. W., and Ramamoorthy, S. (1984). Heavy Metals in Natural Waters-Applied Monitoring and Impact Assessment. (New York, NY: Spring Series on Environmental Management; Springer-Verlag), doi: 10.1007/978-1-4613-9538-6
Muszynska, E., and Hanus-Fajerska, E. (2015). Why are heavy metal hyperaccumulating plants so amazing? BioTechnol. J. Biotechnol. Comput. Biol. Bionanotechnol. 96, 265–271. doi: 10.5114/bta.2015.57730
Nagai, T., Horio, T., Yokoyama, A., Kamiya, T., Takano, H., and Makino, T. (2012). Ecological risk assessment of on-site soil washing with iron (III) chloride in cadmium-contaminated paddy field. Ecotoxicol. Environ. Saf. 80, 84–90. doi: 10.1016/j.ecoenv.2012.02.011
Nagajyoti, P. C., Lee, K. D., and Sreekanth, T. V. M. (2010). Heavy metals, occurrence and toxicity for plants: a review. Environ. Chem. Lett. 8, 199–216. doi: 10.1007/s10311-010-0297-8
Nair, A., Juwarkar, A. A., and Singh, S. K. (2007). Production and characterization of siderophores and its application in arsenic removal from contaminated soil. Water Air Soil Pollut. 180, 199–212. doi: 10.1007/s11270-006-9263-2
Nareshkumar, R., Nagendran, R., and Parvathi, K (2008). Bioleaching of heavy metals from contaminated soil using Acidithiobacillus thiooxidans: effect of sulfur/soil ratio. World J. Microbiol. Biotechnol. 24, 1539–1546. doi: 10.1007/s11274-007-9639-5
Nieboer, E., and Richardson, D. H. S. (1980). The replacement of the nondescript term heavy metals by a biologically and chemistry significant classification of metal ions. Environ. Poll. Series B 1, 3–26. doi: 10.1016/0143-148X(80)90017-8
Nowaka, B., Pessl, A., Aschenbrenner, P., Szentannai, P., Mattenberger, H., Rechberger, H., et al. (2010). Heavy metal removal from municipal solid waste fly ash by chlorination and thermal treatment. J. Hazard. Mater. 179, 323–331. doi: 10.1016/j.jhazmat.2010.03.008
O'Connor, C. S., Lepp, N. W., Edwards, R., and Sunderland, G. (2003). The combined use of electrokinetic remediation and phytoremediation to decontaminate metalpolluted soils: a laboratory-scale feasibility. Environ. Monit. Assess. 84, 141–158. doi: 10.1023/A:1022851501118
Orłowska, E., Godzik, B., and Turnau, K. (2012). Effect of different arbuscular mycorrhizal fungal isolates on growth and arsenic accumulation in Plantago lanceolata L. Environ. Pollut. 168, 121–130. doi: 10.1016/j.envpol.2012.04.026
Oyeku, O. T., and Eludoyin, A. O. (2010). Heavy metal contamination of groundwater resources in a Nigerian urban settlement. Afr. J. Environ. Sci. Technol. 4, 201–214.
Pathak, A., Dastidar, M. G., and Sreekrishnan, T. R. (2009). Bioleaching of heavy metals from sewage sludge by indigenous iron-oxidizing microorganisms using ammonium ferrous sulfate and ferrous sulfate as energysources: a comparative study. J. Hazard. Mater. 171, 273–278. doi: 10.1016/j.jhazmat.2009.05.139
Peng, G., Tian, G., Liu, J., Bao, Q., and Zang, L. (2011). Removal of heavy metals from sewage sludge with a combination of bioleaching and electrokinetic remediation technology. Desalination 271, 100–104. doi: 10.1016/j.desal.2010.12.015
Peng, L., Chen, X., Zhang, Y., Du, Y., Huang, M., and Wang, J. (2015). Remediation of metal contamination by electrokinetics coupled with electrospun polyacrylonitrile nanofiber membrane. Process. Saf. Environ. Prot. 98, 1–10. doi: 10.1016/j.psep.2015.06.003
Pradhan, D., Sukla, L. B., Sawyer, M., and Rahman, P. K. S. M. (2017). Recent bioreduction of hexavalent chromium in wastewater treatment: a review. J. Indus. Eng. Chem. 55, 1–20. doi: 10.1016/j.jiec.2017.06.040
Qdais, H. A., and Moussa, H. (2004). Removal of heavy metals from wastewater by membrane processes: a comparative study. Desalination 164, 105–110. doi: 10.1016/S0011-9164(04)00169-9
Rahman, P. K. S. M., and Bastola, S. (2014). Biological reduction of iron to the elemental state from ochre deposits of Skelton Beck in Northeast England. Front. Environ. Sci. 2, 22–25. doi: 10.3389/fenvs.2014.00022
Rahman, P. K. S. M., and Murthy, M. A. V. (2005). “Stabilisation of chromium by reductase enzyme treatment,” in Advances in S/S for Waste and Contaminated Land, eds A. T. Abir and A. A. Stegemann (London: Balkema Publishers), 347–355.
Rainbow, P. S. (1995). Biomonitoring of heavy metal availability in the marine environment. Marine Poll. Bull. 31, 183–192. doi: 10.1016/0025-326X(95)00116-5
Rosestolato, D., Bagatin, R., and Ferro, S. (2015). Electrokinetic remediation of soils polluted by heavy metals (mercury in particular). Chem. Eng. J. 264, 16–23. doi: 10.1016/j.cej.2014.11.074
Roy, M., Giri, A. K., Dutta, S., and Mukherjee, P. (2015). Integrated phytobial remediation for sustainable management of arsenic in soil and water. Environ. Internat. 75, 180–198. doi: 10.1016/j.envint.2014.11.010
Ryan, R. P., Germaine, K., Franks, A., Ryan, D. J., and Dowling, D. N. (2008). Bacterial endophytes, recent developments and applications. FEMS Microbiol. Lett. 278, 1–9. doi: 10.1111/j.1574-6968.2007.00918.x
Ryan, R. P., Ryan, D., and Dowling, D. N. (2007). Plant protection by the recombinant, root colonizing Pseudomonas fluorescens F113rif PCB strain expressing arsenic resistance, improving rhizoremediation. Lett. Appl. Microbiol. 45, 668–674. doi: 10.1111/j.1472-765X.2007.02248.x
Rzymski, P., Niedzielski, P., Poniedziałek, B., and Klimaszyk, P. (2014). Bioaccumulation of selected metals in bivalves (Unionidae) and Phragmites australis inhabiting a municipal water reservoir. Environ. Monitor. Asses. 186, 3199–3212. doi: 10.1007/s10661-013-3610-8
Rzymski, P., Tomczyk, K., Rzymski, P., Poniedziałek, B., Opala, T., and Wilczak, M. (2015). Impact of heavy metals on the female reproductive system.Ann. Agri. Environ. Med. 22, 259–264. doi: 10.5604/12321966.1152077
Salem, H. M., Eweida, E. A., and Farag, A. (2000). Heavy Metals in Drinking Water and Their Environmental Impact on Human Health. ICEHM, Cairo University, Egypt, 542–556.
Santos, J. A. G., Gonzaga, M. I. S., Ma, L. Q., and Srivastava, M. (2008). Timing of phosphate application affects arsenic phytoextraction by P. vittata L. of different ages. Environ. Pollut. 154, 306–311. doi: 10.1016/j.envpol.2007.10.012
Selvi, A., and Aruliah, R. (2018). A statistical approach of zinc remediation using acidophilic bacterium via an integrated approach of bioleaching enhanced electrokinetic remediation (BEER) technology. Chemosphere 207, 753–763. doi: 10.1016/j.chemosphere.2018.05.144
Selvi, A., Das, D., and Das, N. (2015). Potentiality of yeast Candida sp. SMN04 for degradation of cefdinir, a cephalosporin antibiotic: kinetics, enzyme analysis and biodegradation pathway. Environ. Technol. 36, 3112–3124. doi: 10.1080/09593330.2015.1054318
Shabani, N., and Sayadi, M. H. (2012). Evaluation of heavy metals accumulation by two emergent macrophytes from the polluted soil: an experimental study. Environment 32, 91–98. doi: 10.1007/s10669-011-9376-z
Sharma, S., and Malaviya, P. (2014). Bioremediation of tannery wastewater by chromium resistant fungal isolate Fusarium chlamydosporium SPFS2-g. Curr. Wor. Environ. 9:721. doi: 10.12944/CWE.9.3.21
Sigua, G., Celestino, A., Alberto, R., Paz-Alberto, A., and Stone, K. (2016). Enhancing cleanup of heavy metal polluted landfill soils and improving soil microbial activity using green technology with ferrous sulfate. Internat. J. Environ. Protect. 6, 97–103. doi: 10.5963/IJEP0601009
Simoni, S. F., Schafer, A., Harms, H., and Zehnder, A. J. B. (2001). Factors affecting mass transfer limited biodegradation in saturated porous media. J. Contam. Hydrol. 50, 99–120. doi: 10.1016/S0169-7722(01)00099-7
Spiegel, J., and Maystre, L. Y. (1998). Environmental Pollution Control and Prevention. Encyclopedia of Occupational Health and Safety, 4th ed. Geneva: International Labour Office.
Steenland, K., and Boffetta, P (2000). Lead and cancer in humans: where are we now? Am. J. Ind. Med. 38, 295–299. doi: 10.1002/1097-0274(200009)38:3<295::AID-AJIM8>3.0.CO;2-L
Sylvia, D. M., Fuhrmann, J. J., Hartel, P. G., and Zuberer, D. A. (2005). Principles and Applications of Soil Microbiology, 2nd ed. New Jersey, NY: Prentice Hall Upper Saddle River.
Varia, J., Martínez, S. S., Orta, S. V., Bull, S., and Roy, S. (2013). Bioelectrochemical metal remediation and recovery of Au3+, Co2+ and Fe3+ metal ions. Electrochim. Acta 95, 125–131. doi: 10.1016/j.electacta.2013.02.051
Vilensky, M. Y., Berkowitz, B., and Warshawsky, A. (2002). In situ remediation of groundwater contaminated by heavy- and transition-metal ions by selective ion-exchange methods. Environ. Sci. Technol. 36, 1851–1855. doi: 10.1021/es010313+
Violetta, F., and Sergio, F. (2009). Electrokinetic extraction of surfactants and heavy metals from sewage sludge. Electrochim. Acta 54, 2108–2118. doi: 10.1016/j.electacta.2008.08.048
Virkutyte, J., Sillanpaa, M., and Latostenmaa, P. (2002). Electrokinetic soil remediation-critical overview. Sci. Total Environ 289, 97–121. doi: 10.1016/S0048-9697(01)01027-0
Vocciante, M., Caretta, A., Bua, L., Bagatin, R., and Ferro, S. (2016). Enhancements in electrokinetic remediation technology: environmental assessment in comparison with other configurations and consolidated solutions. Chem. Eng. J. 289, 123–134. doi: 10.1016/j.cej.2015.12.065
Volesky, B. (1990). “Introduction,” in Biosorption of Heavy Metals, ed B. Volesky (Boca Raton, FL: CRC press), 3–5.
Wang, J. L., and Chen, C. (2006). Biosorption of heavy metals by Saccharomyces cerevisiae: a review. Biotechnol. Adv. 24, 427–451. doi: 10.1016/j.biotechadv.2006.03.001
Wang, J. Y., Zhang, D. S., Stabnikova, O., and Tay, J. H. (2005). Evaluation of electrokinetic removal of heavy metals from sewage sludge. J. Hazard. Mater. B 124, 139–146. doi: 10.1016/j.jhazmat.2005.04.036
WHO (2001). Arsenic and Arsenic Compounds, 2nd ed. Environmental Health Criteria 224. United Nations Environment Programme, the International Labour Organization, and the World Health Organization publishers.
Wick, L. Y. (2009). “Coupling electrokinetics to the bioremediation of organic contaminants: principles and fundamental interactions,” in Electrochemical Remediation Technologies for Polluted Soils, Sediments and Groundwater, eds K. R. Reddy and C. Cameselle (New Jersey: John Wiley and Sons), 369–387. doi: 10.1002/9780470523650.ch18
Wu, F. Y., Ye, Z. H., and Wong, M. H. (2009). Intraspecific differences of arbuscular mycorrhizal fungi in their impacts on arsenic accumulation by Pteris vittata L. Chemosphere 20, 1258–1264. doi: 10.1016/j.chemosphere.2009.05.020
Wuana, R. A., and Okieimen, F. E. (2011). Heavy metals in contaminated soils: a review of sources, chemistry, risks and best available strategies for remediation. ISRN Ecol. 402647:20. doi: 10.5402/2011/402647
Yang, Q., Tu, S., Wang, G., Liao, X., and Yan, X. (2012). Effectiveness of applying arsenate reducing bacteria to enhance arsenic removal from polluted soils by Pteris vittata L. Int. J. Phytoremed. 14, 89–99. doi: 10.1080/15226510903567471
Zhou, D. M., Deng, C. F., and Cang, L. (2004). Electrokinetic remediation of a Cu contaminated red soil by conditioning catholyte pH with different enhancing chemical reagents. Chemosphere 56, 265–273. doi: 10.1016/j.chemosphere.2004.02.033
Keywords: integrated approaches, heavy metal, environment, toxicity, review, remediation
Citation: Selvi A, Rajasekar A, Theerthagiri J, Ananthaselvam A, Sathishkumar K, Madhavan J and Rahman PKSM (2019) Integrated Remediation Processes Toward Heavy Metal Removal/Recovery From Various Environments-A Review. Front. Environ. Sci. 7:66. doi: 10.3389/fenvs.2019.00066
Received: 28 November 2018; Accepted: 29 April 2019;
Published: 22 May 2019.
Edited by:
Vincenzo Parrino, University of Messina, ItalyReviewed by:
Naveen Kumar Singh, Manipal University Jaipur, IndiaAna Paula Pinto, University of Evora, Portugal
Copyright © 2019 Selvi, Rajasekar, Theerthagiri, Ananthaselvam, Sathishkumar, Madhavan and Rahman. This is an open-access article distributed under the terms of the Creative Commons Attribution License (CC BY). The use, distribution or reproduction in other forums is permitted, provided the original author(s) and the copyright owner(s) are credited and that the original publication in this journal is cited, in accordance with accepted academic practice. No use, distribution or reproduction is permitted which does not comply with these terms.
*Correspondence: Adikesavan Selvi, c2VsdmkuYTIwMTMmI3gwMDA0MDt2aXRhbHVtLmFjLmlu; c2VsamVldiYjeDAwMDQwO2dtYWlsLmNvbW9yY2lkLm9yZy8wMDAwLTAwMDItMTQyMi02Nzk0
Aruliah Rajasekar, cmFqYXNla2FyZ29vZCYjeDAwMDQwO2dtYWlsLmNvbQ==; cmFqYXNla2FyZ29vZCYjeDAwMDQwO3R2dS5lZHUuaW5vcmNpZC5vcmcvMDAwMC0wMDAxLTUzMjQtMzI5MA==