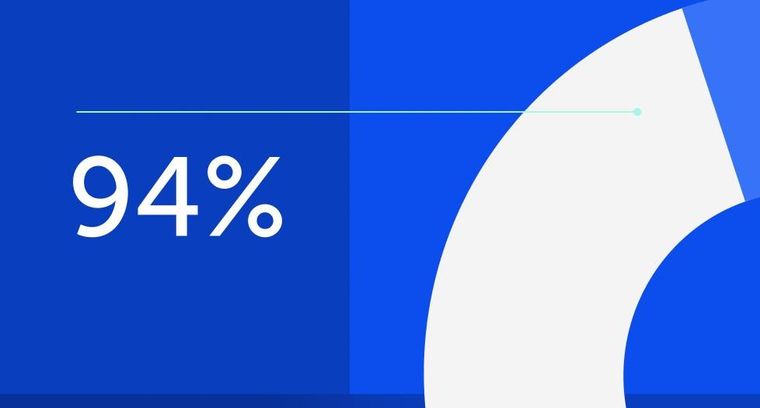
94% of researchers rate our articles as excellent or good
Learn more about the work of our research integrity team to safeguard the quality of each article we publish.
Find out more
ORIGINAL RESEARCH article
Front. Environ. Sci., 22 March 2019
Sec. Water and Wastewater Management
Volume 7 - 2019 | https://doi.org/10.3389/fenvs.2019.00035
Point-of-use (POU) drinking water treatment systems provide solutions for communities where centralized facilities are unavailable. Effective POU systems treat and reduce the number of pathogens in POU water supplies often employing disinfection. Chlorine disinfection results in the formation of disinfection by-products (DBPs), such as trihalomethanes (THMs), through the reaction of chlorine with natural organic matter (NOM) over time. Although THMs are known to be harmful to human health, little is known about their production within POU systems. This study compares the disinfectants; Electrochemically Activated Solutions (ECAS), hypochlorous acid (HOCl), and sodium hypochlorite (NaOCl), with respect to their potential to produce THMs within POU drinking water systems. Headspace solid-phase microextraction (HS-SPME) gas chromatography mass spectrometry (GC-MS) was utilized to quantify THMs in treated water samples containing NOM (Suwannee River humic acid, 4 mg L−1). All disinfection treatments were matched to free chlorine concentrations of 1, 3, and 5 mg L−1, using reaction times of 1, 5, and 10 min. THMs were produced at free chlorine concentrations of 5 mg L−1 and at reaction times of 5 and 10 min for all disinfectants. ECAS or HOCl, resulted in the formation of significantly lower total THM concentrations across all reaction times and free chlorine concentrations, compared to NaOCl. ECAS can be generated at the POU requiring only water, salt and energy for production, and this study demonstrates that its use results in reduced formation of THMs, compared with NaOCl. Further work is required to replicate these findings within scaled-up POU water treatment systems.
Approximately 1 billion people worldwide are living without access to improved drinking water sources (Corcoran et al., 2010; Unicef and World Health Organization, 2014; Ardakanian et al., 2015; World Health Organization, 2015). For the past 100 years chlorine has been crucial in the production of biologically safe drinking water. Chlorine is the most widely used disinfectant in drinking water treatment due to its availability, low cost, and broad spectrum antimicrobial efficacy (Rodriguez and Serodes, 2001; Farghaly et al., 2013; Kumari and Gupta, 2015). Decentralized point-of-use (POU) drinking water treatment systems typically utilize alternative disinfectant solutions (Mbilinyi et al., 2005; Peter-Varbanets et al., 2009; Domènech, 2011; Attisani, 2016; Carratalà et al., 2016; Pooi and Ng, 2018) or chlorine release tablets (Jain et al., 2010; Werner et al., 2016), rather than conventional chlorination solutions (i.e., NaOCl) for the production of biologically safe water. Alternatives to conventional chlorination are adopted due to quicker disinfection times, ease of transport and storage (Clasen and Edmondson, 2006; Jain et al., 2010). Our recent study has shown that Electrochemically Activated Solutions (ECAS) can be utilized within a POU drinking water system to produce biologically safe drinking water (Clayton et al., in press), and this disinfection system is now a Drinking Water Inspectorate (DWI) approved product for use in public water supply in the UK (Drinking Water Inspectorate, 2018). The production of trihalomethanes within drinking water systems is known over the medium (hours) to long-term (days/weeks) (Rodriguez and Serodes, 2001; Rossman et al., 2001; Emmert et al., 2009; Brown et al., 2010; Guilherme and Rodriguez, 2015). However, within POU drinking water treatment systems where alternative disinfectants are applied over a short contact time (≤10 min), THM formation is largely unknown.
It is well-understood that the chlorination of drinking water containing natural organic matter (NOM) can result in the formation of disinfection by-products [DBPs] (World Health Organization, 2000; Grunwald et al., 2002; Di Cristo et al., 2013). Trihalomethanes (THMs) are an important group of DBPs that form during the chlorination of drinking water, and are known to be hazardous to human health (Liang and Singer, 2003). THMs form when free chlorine reacts with natural organic material (NOM) over an extended contact time throughout water treatment processes, as occurs during conventional chlorination (Amy et al., 1987; Brown et al., 2011a; Di Cristo et al., 2013). The short-term (i.e., <10 min) formation of disinfection by-products (THMs) is relatively unknown for decentralized POU drinking water treatment applications, where treated water is often utilized immediately. THMs are a health concern since they have been shown to possess carcinogenic and mutagenic properties, and can be attributed to health concerns such as cancer, liver and kidney damage, miscarriages and birth defects in new born babies (King et al., 2000; Dodds and King, 2001; Wright et al., 2004; Bove et al., 2007; Wang et al., 2007; Rahman et al., 2010; Chowdhury et al., 2011; Grazuleviciene et al., 2013; Siddique et al., 2015). Drinking water quality guidelines state maximum individual concentrations for all THMs; chloroform (CHCl3) [300 μg L−1], bromoform (CHBr3) [100 μg L−1], dibromochloromethane (DBCM; CHBr2Cl) [100 μg L−1], and bromodichloromethane (BDCM; CHBrCl2) [60 μg L−1] (World Health Organization, 2011). This has resulted in maximum guideline values for total THMs (tTHMs) in drinking water worldwide to vary between 80 (USEPA, 2010) and 100 μg L−1 (The Council of the European Union, 1998; Health Canada, 2017). In the UK, the drinking water inspectorate enforces a tTHM limit of 100 μg L−1 (Drinking Water Inspectorate, 2012).
Typically, drinking water treatment systems incorporate disinfection stage(s) that utilize chlorine gas (Cl2), sodium hypochlorite (NaOCl), or calcium hypochlorite [Ca(OCl)2] (World Health Organization, 2000; Drinking Water Inspectorate, 2012) to achieve effective chlorination. These chlorination techniques result in high concentrations of free and residual chlorine that are distributed throughout the network achieving long residual reaction times (World Health Organization, 2011). A minimum free chlorine residual concentration of 0.5 mg L−1 is recommended throughout distribution systems to ensure biologically safe water (World Health Organization, 2011).
The formation of THMs in drinking water supplies occurs when free chlorine reacts with NOM over time (World Health Organization, 2004). Minimizing NOM [encompassing dissolved, suspended, particulate organic carbon, or matter which occur in aquatic systems (Demiral et al., 2006)] concentrations in raw water supplies in decentralized point-of-use (POU) drinking water treatment systems, via filtration prior to chlorination, limits THM formation (World Health Organization, 2000). Other considerations such as temperature, pH, and contact time within the distribution network (World Health Organization, 2000) are also known to drive THM formation (Brown et al., 2010, 2011b; Rasheed et al., 2017). As the knowledge of the toxicological effects of THMs on human health has increased, so has the need to investigate alternative disinfection techniques such as ozonation (Schlichter et al., 2004; Zhu et al., 2014) and UV sterilization (Carratalà et al., 2016). Electrochemical disinfection, either direct or via the application of electrochemically activated solutions (ECAS) has also gained interest (Kerwick et al., 2005; Ghebremichael et al., 2011). Electrochemically activated solutions are referred to as electrolyzed water (EW), electrolyzed oxidizing water (EOW), oxidized water (OW), and mixed oxidants (MIOX) solutions (Venczel et al., 1997). A schematic of ECAS generation is shown in Figure 1, whereby a weak saline solution (typically 1% [w/v]) is passed through an electrochemical cell containing separate anodic and cathodic chambers (Robinson et al., 2010, 2013; Thorn et al., 2013). Solutions produced at the cathode (catholyte) are highly reductive [−800 mV], compared to anodic solutions (anolyte) which are highly oxidative [+1,000 mV] in nature (Inoue et al., 1997; Morita et al., 2000; Liao et al., 2007; Robinson et al., 2010). The reactions which occur at the anodic surface result in the production of chlorine (Cl2) and oxygen, as well as a hydroxyl radicals and transient oxidative functional groups [e.g., OH−, O3, H2O2, and ; (Jeong et al., 2006; Martínez-Huitle and Brillas, 2008)]. The myriad of transient reactive species increase the ORP of the solution, resulting in a pH shift toward the acidic range. This is dependent on redox reactions of strongly adsorbed electro-active water derived intermediate molecular species (Burke and O'Neill, 1979; Erenburg et al., 1984; Boggio et al., 1985; Trasatti, 1991). Initially, water is decomposed at the anode surface
Figure 1. Schematic of ECAS generation. A direct current is applied across two electrodes, an anode (+) and cathode (–) separated by a permeable ion exchange membrane, allowing constant perfusion of an electrolyte solution (1% w/v NaCl). Anolyte solution generated (ECAS) has a high oxidizing potential, the catholyte solution has a high reducing potential.
Dissociated chloride ions from NaCl through direct current polarization are then adsorbed;
Chlorine gas and oxygen are both then produced from these intermediates;
A large scientific body of evidence now exists for the two 1-electron processes shown above (Equations 1 and 2) (Stoner et al., 1982; Trasatti, 1987; Cai, 2005), and the electrochemically generated chlorine then reacts with water producing hypochlorous acid;
This reaction is pH dependent, and (according to the Nernst equation) dictates which free form of chlorine is most prevalent within generated solution; Cl2, HClO, or ClO (Stoner et al., 1982; Sivey et al., 2010). The anodic solutions exhibit antimicrobial properties as a result of chlorine and their high oxidation reduction potential (ORP), disrupting cell membrane function (Inoue et al., 1997; Kumon, 1997; Thorn et al., 2012). This high ORP environment has been shown to damage and rupture both inner and outer microbial membranes, prohibiting microbial functionality, including energy generating mechanisms (Liao et al., 2007). ECAS solutions have been shown to exhibit rapid antimicrobial kinetics, exerting a significant effect after short contact times between 2 (Robinson et al., 2011) and 10 s (Liao et al., 2007), and are still efficacious with suitable storage after 12 months (Robinson et al., 2013). ECAS have shown comparable efficacy to other chlorine based disinfectants at lower free chlorine concentrations (Thorn et al., 2013). The low free chlorine concentrations of ECAS coupled with its high ORP and broad spectrum antimicrobial activity could potentially reduce THM formation within water treatment systems.
ECAS has the potential to be used within decentralized POU drinking water systems, in remote areas or communities, or as part of disaster relief efforts which do not have access to centralized drinking water distribution networks. Decentralized point-of-use applications require reliable and robust treatment systems capable of producing chemically and biologically safe drinking water. Source waters used to feed decentralized POU water treatment systems can often be contaminated with feces, therefore if treatment is not effective then consumed water can result in diarrheal diseases. These diarrheal diseases can result in dehydration, malnutrition and can be fatal, especially to vulnerable groups within the populations such as young children (Cabral, 2010). ECAS has been shown to exhibit significant antimicrobial activity against a range of microorganisms, such as Escherichia coli [E. coli] (Robinson et al., 2011), including a pathogenic enterohemorrhagic E. coli strain: O157:H7 [95% reduction <10 s] (Liao et al., 2007), which can cause hemolytic uremic syndrome (HUS). Young children and the elderly are most at risk of HUS, which can be fatal (The Environment Agency, 2002). Under such challenging situations the formation of THMs resulting from POU disinfection within such systems is often overlooked. The combination of ECAS properties; low pH, high ORP, low comparative free chlorine concentrations and broad spectrum antimicrobial activity, demonstrate that ECAS could be a viable alternative for use within decentralized drinking water treatment systems. Both in producing chemically and biologically safe drinking water, including the potential for reduced THM formation.
This study investigates the comparative formation of total THMs in water when treated with three disinfectants (ECAS, NaOCl, and HOCl) as a function of contact time and free chlorine, for application in point-of-use decentralized drinking water treatment systems.
Ultrapure water with a resistivity output of 18.2 MΩ, was used for preparation of humic acid solutions (Purite Water Purification Solutions, United Kingdom). Calibration solutions, the internal standard Fluorobenzene and THM standard solution (comprising of chloroform, DBCM, BDCM and bromoform) (Sigma Aldrich, Dorset, United Kingdom), were prepared using high grade (HPLC) methanol (Fisher Scientific, United Kingdom).
Hypochlorous acid stock solution was produced through the dissolution of sodium dichloroisocyanurate (NaDCC) within 1 L of deionized water producing a free chlorine concentration of 201 ± 13.55 mg L−1, pH of 5.6 ± 0.25, and an ORP of 958 ± 18.98 mV. Stock solution of sodium hypochlorite was prepared by diluting a commercial bleach (Pattersons bleach; Pattersons Ltd., Bristol, United Kingdom) in deionized water to a final free chlorine concentration of 508 ± 18.19 mg L−1, pH of 11.4 ± 0.1, and an ORP of 588 ± 0.95 mV. Figure 1 shows how electrochemically activated solutions (ECAS) were generated using an electrochemical cell supplied by Bridge Biotechnology Ltd (Fife, Scotland, United Kingdom). Generated ECAS solutions had free chlorine concentrations of 158.63 ± 18.66 mg L−1, pH of 3.3 ± 0.16, and an ORP of 1,134 ± 3.26 mV. ECAS solutions were stored at 4°C in the dark, and used within 5 days of production (Robinson et al., 2011). Disinfectant solutions were diluted in deionized water to produce equal concentrations of free chlorine (1–5 mg L−1) as determined using the N, N-diethyl-p-phenylenediamine sulfate (DPD) no. 1 Palintest test (Palintest Ltd., Gateshead, United Kingdom). The pH and ORP of disinfectant and test solutions were measured using an Orion Dual Star (Fisher Scientific, United Kingdom).
THM standard solutions containing chloroform (CHCl3), bromodichloromethane (CHCl2Br), dibromochloromethane (CHClBr2), and bromoform (CHBr3) each at 20 μg mL−1 were prepared from a standard THM stock solution (200 μg mL−1) and HPLC grade methanol, both supplied by Sigma Aldrich (Dorset, United Kingdom).
A NOM stock solution was prepared by dissolving 4 mg of Suwannee River humic acid (SRHA; International Humic Substances Society, St Paul, MN, United States of America), in 100 mL of ultrapure water (overnight) to achieve a concentration of 40 mg L−1 (Gadad et al., 2007). From this, standard NOM solutions of 4 mg L−1 were prepared (Boggs et al., 1985; Venczel et al., 2004).
The quantification of THMs in pre and post disinfected water samples were determined using the standard method [BS EN ISO 17943, (British Standards Institution, 2016)], which incorporates headspace solid-phase microextraction (HS-SPME) gas chromatography and mass spectrometry (GC/MS). GC/MS was carried out using an Agilent 7820A GC System with an Agilent 5977B high efficiency source with Mass Selective Detection, and a phenyl methyl silox capillary column (Agilent Technologies, Santa Clara, California, United States), see Table 1 (British Standards Institution, 2016). An internal standard (IS) of fluorobenzene (British Standards Institution, 2016), was diluted to a working concentration of 20 μg mL−1 in HPLC grade methanol from a solution of 2,000 μg mL−1 (Sigma Aldrich, Dorset, United Kingdom).
A Supelco solid-phase microextraction (SPME) fiber holder for manual sampling was fitted with an 85 μm carboxen/polydimethylsiloxane (CAR/PDMS) fiber (Sigma Aldrich, Dorset, United Kingdom). The fiber was conditioned at 280°C for 2 h.
A working calibration curve for HS-SPME extracted THMs from water samples was constructed by dissolving mixed THM standard solutions (each at 20 μg mL−1) in ultrapure water containing 6 g NaCl, to produce solutions at 10, 20, 40, 60, 80, and 100 μg L−1, respectively. Regression analysis (r2-values) and mean retention times for each individual THM(s) extracted from the standard mixed solution are shown in Table 2. The total THMs (tTHMs) extracted from standard mixed solutions (i.e., the sum of CHCl3, CHCl2Br, CHClBr2, and CHBr3) is also shown in Table 2.
Table 2. THM calibration regression values for THM calibration mix with dilutions of 10, 20, 40, 60, 80, and 100 μg L−1 (n = 3).
For the reaction vials (sterile glass universals with solid high-density polyethylene screw caps), disinfectants were added to standard NOM solution (4 mg L−1 IHSS Humic acid), maintaining a total reaction volume of 30 mL, to achieve free chlorine concentrations of 0, 1, 3, and 5 mg L−1. Reaction times (1, 5, or 10 min) were controlled by taking a 20 mL sample from the test reaction vial, and injected into a test extraction vial. Test extraction vials (30 mL sterile extraction glass universals with high density polyethylene screw cap with silicone septum) contained 6 g laboratory grade NaCl, 5 g L−1 sodium thiosulfate (British Standards Institution, 2005; Antoniou et al., 2006; Environmental Protection Agency, 2011) and the internal standard, fluorobenzene, at a final concentration of 100 μg L−1. Prior to headspace extraction all samples were incubated at 40°C for 20 min, inclusive of 10 min headspace extraction (fiber exposed). During SPME fiber exposure the manual SPME holder was injected through the septum into the headspace of the sample vial, exposing the CAR/PDMS fiber. After the 10-min fiber exposure period, care was taken to ensure the CAR/PDMS fiber was retracted into the manual SPME holder and inserted into the GC/MS inlet (< 30s), minimizing extraneous exposure of the fiber. All sample fibers had a desorption period of 2-min prior to analysis.
Individual THM concentrations were calculated using Agilent Mass Hunter Enhanced Data Analysis Software (Santa Clara, California, United States). tTHMs were calculated from the sum of CHCl3, CHCl2Br, CHClBr2, and CHBr3. Values reported were blank corrected, and a limit of detection (LoD) of 0.86 μg L−1 for all samples was determined experimentally. Where analysis is below the LoD, then data values are represented by an asterisk (*).
Comparative statistical analysis of THM concentrations (between experimental variables) was performed using a two-way ANOVA with Bonferroni post-hoc test (GraphPad Prism version 5.00 for Windows, San Diego, CA). A P < 0.05 was regarded as significant.
Due to the volatility of THMs, the extraction efficiency and quantitative analysis of THMs in water via HS-SPME vary with temperature and molecular weight. The regression values (r2-values) for the standard THM solutions increase in the order: chloroform < BDCM < DBCM < Bromoform (Table 2). THM compounds become more stable in the headspace with increasing molecular weight, and therefore boiling point, which supports the observed regression values shown in Table 2. The greatest deviations along the regression are associated with the determination of higher concentrations of individual THMs (>60 μg L−1). However, increased linearity for individual and total THM species were observed at lower concentrations between 0, 10, and 60 μg L−1 (regression value of 0.9674 for tTHMs). Only three instances occurred in this study where tTHM concentration values exceeded 60 μg L−1, in the presence of NaOCl at 5 and 10 min reaction times (Figure 2).
Figure 2. Total THM formation with disinfectants (A) NaOCl (Δ), (B) HOCl (□), or (C) ECAS (○) at free chlorine concentrations between 1 (white), 3 (gray), and 5 mg L−1 (black). Contact times were 1, 5, and 10 min (n = 6 ± SD). tTHM Maximum (horizontal dashed line) refers to the maximum guideline value permissible in drinking water (within the UK). tTHMs concentrations below the limit of detection (*).
The reaction of the three disinfectants with NOM produced tTHMs at free chlorine concentrations of 3 and 5 mg L−1 and at reactions times of 5 and 10 min on all occasions (Figure 2). The most abundant individual THM species in all reactions was chloroform (>75% of the total), followed by the three brominated species [bromodichloromethane, dibromochloromethane, and bromoform] (Figure 3). The formation of low concentrations of brominated THM species are a consequence of bromide present within Suwannee River humic acid [NOM] solution, as reported previously (Chowdhury, 2013). The high relative formation of chloroform is in accordance with previous findings regarding THM formation within drinking water (Cho et al., 2003; Ikem, 2010; Yang et al., 2015).
Figure 3. Mean percentage composition of THM species for disinfectants: (A) NaOCl (Δ), (B) HOCl (□), or (C) ECAS (○); as a function of reaction time (rows) and free chlorine; Chloroform (white), bromomodichloromethane (light gray), dibromochloromethane (dark gray), and bromoform (black). N = 6 (±SD).
At a concentration of 1 mg L−1 of free chlorine the observed production of tTHMs for all disinfectants was low or below the level of detection. Regardless, the formation of tTHMs, although low, reached a maximum concentration, for this experimental design, at a reaction time of 10 min for all disinfectants at 1 mg L−1 (Figure 2). Upon increasing the free chlorine concentration (3 and 5 mg L−1) significant differences were then observed between the reaction times (Table 3). NaOCl resulted in peak tTHM concentrations after a 5 min reaction time, except for 1 mg L−1 free chlorine concentration which resulted in peak concentration after 10 min (Figure 2A). Disinfectants HOCl and ECAS both resulted in peak tTHM concentration at 10 min reaction times at all free chlorine concentrations (Figures 2B, C).
Table 3. Analysis of total THM formation between reaction time (minutes) and free chlorine concentration (mg L-1), for each disinfectant type (ECAS, HOCl, and NaOCl).
When comparing the three disinfectants, the maximum observed concentrations of tTHMs were formed by NaOCl across all free chlorine concentrations for all reaction times (Figure 2A). At a free chlorine concentration of 5 mg L−1 and a reaction time of 5 min the mean tTHM formation exceeded the maximum regulatory threshold of 100 μg L−1, the permissible level for UK drinking water (Drinking Water Inspectorate, 2012). However, surprisingly, the mean level of tTHMs decreased between 5 and 10 min reaction time, at free chlorine concentrations 3 and 5 mg L−1. This is contradictory to other published studies which demonstrate an increase in tTHM formation in reaction times in excess of 10 min (i.e., hours, days) (Brown et al., 2010; Ghebremichael et al., 2011; Saidan et al., 2013; Werner et al., 2016). It is postulated that this observed decline could be due to the hydrolysis, or dehalogenation of already formed tTHMs present in solution (Mabey and Mill, 1978; Rahman, 2015; Abusallout et al., 2017), although, it is unknown the extent to which these reactions can occur over such a short contact time (i.e., 10 min). The decline in tTHMs was not observed with either HOCl or ECAS, probably due to a combination of the low tTHM concentrations formed, the rapid reaction kinetics of these agents, and the lower pH of the disinfectant solutions (Robinson et al., 2011; Liao et al., 2017). Further investigations into how tTHM concentrations change over longer reaction times (i.e., tens of minutes, hours, and days) for all of the disinfectants tested would be required. This would answer how the chemical quality of drinking water changes over time if dosed with either HOCl or ECAS and subsequently stored as part of a decentralized point-of-use drinking water treatment system. This study was only concerned with the formation of tTHMs, therefore THM derivatives or other DBPs formed as part of the reactions were not identified. Further studies would be required to investigate other DBPs formed as part of this reaction.
Many studies into THM formation focus on formation dynamics as part of centralized water treatment systems which feed into extensive distribution networks. Decentralized systems require rapid, broad spectrum antimicrobials, and ECAS has been shown to disinfect in <10 s (Liao et al., 2007; Robinson et al., 2011), therefore is a potential alternative within decentralized POU drinking water treatment systems, where there are no extensive distribution networks (Clayton et al., in press).
Lower tTHM concentrations were observed from HOCl and ECAS disinfectants, at all free chlorine concentrations and reaction times (see Figures 2B, C), compared to the NaOCl disinfectant (Figure 2A). Statistical analysis reveals no significant difference between tTHMs formed by HOCl and ECAS disinfectants at matched free chlorine concentrations and contact times (Table 4). However, significant differences were observed between NaOCl, and both HOCl and ECAS (P < 0.01) at all matched reaction times and free chlorine concentrations (Table 4).
Table 4. Analysis of total THM formation between reaction time (minutes) and disinfectant type (NaOCl, HOCl, and ECAS), for each free chlorine concentration (mg L−1).
Free chlorine species present in chlorine solutions are pH dependent (Stoner et al., 1982; Sivey et al., 2010):
(Liu and Margerum, 2001; Heeb et al., 2014)
THMs have been shown to have a greater affinity to form at a higher pH and with increased free chlorine concentrations (Peters et al., 1980; Urano et al., 1983; Brown et al., 2010; Saidan et al., 2013; Rasheed et al., 2017). It is known that HOCl is the dominant compound that results in tTHM formation, although, since tTHM formation is base-catalyzed (Yee et al., 2009), there is a trade-off in terms of the effect of pH. To further understand this phenomenon, changes in these physicochemical parameters within the reaction vessel were measured over the full 10 min reaction time, for each of the disinfectants. The pH and free chlorine concentration of NaOCl remained greater than both HOCl and ECAS over the 10 min reaction time (Figure 4). All disinfectant reaction vials showed a decrease in pH over the 10 min contact time, when reacting with 4 mg L−1 humic acid (Figure 4). Significant reductions in pH were observed for all disinfectants at 5 mg L−1 free chlorine (p = ≤ 0.01). In terms of pH, no significant differences were observed between disinfectants at 1 mg L−1; however, significant differences were observed at free chlorine concentrations of 3 and 5 mg L−1, whereby the pH of NaOCl was greater than HOCl, and HOCl greater than ECAS (Table 5). All disinfectant reaction vials showed a reduction in free chlorine over the 10 min contact time from a starting free chlorine concentration of 5 mg L−1 (Figure 4). ECAS treatment resulted in the greatest comparative decrease after 10 min (34.68%), whilst NaOCl had the smallest reduction (19.34%). Collectively, this physicochemical data is in-line with previous research studies, whereby a positive correlation has been observed between higher free chlorine concentrations, higher pH and increased THM formation (Stevens et al., 1976; Kim et al., 2002; Liang and Singer, 2003; Hua and Reckhow, 2007; Chowdhury and Champagne, 2008; David, 2014). Free chlorine remains in excess after 10 min for all experiments conducted, which is consistent with previous studies (Brown et al., 2010). Therefore, further investigations should investigate whether the remaining excess free chlorine present after ECAS or HOCl reacting with NOM results in significantly increased THMs at extended contact times (i.e., >10 min).
Figure 4. Physicochemical parameters of disinfectant solutions reacting with 4 mg L−1 SRHA, over a 10 min reaction time. (A) NaOCl, (B) HOCl, and (C) ECAS. Left column: pH; Right column: free chlorine concentrations: 1 mg L−1 (Δ); 3 mg L−1 (■), and 5 mg L−1 (•). N = 3 (± SD).
Table 5. Significant difference of physicochemical characteristics between NaOCl, HOCl, and ECAS at 1, 3, and 5 mg L−1 free chlorine dosing concentrations.
This study has demonstrated that both HOCl and ECAS result in reduced THM formation under the experimental conditions. Reactions between NaOCl and NOM resulted in significantly increased tTHM formation compared to HOCl and ECAS under the experimental conditions. The increased formation of tTHMs in the presence of NaOCl is likely a result of the higher pH of the disinfectant (11.4 ± 0.1) compared to HOCl (5.6 ± 0.25) or ECAS (3.3 ± 0.16). THMs have shown to have a greater formation affinity in more alkaline conditions (Peters et al., 1980; Urano et al., 1983; Brown et al., 2010; Saidan et al., 2013;Rasheed et al., 2017).
The maximum observed tTHM concentrations occurred in the presence of NaOCl; however, a decline in tTHMs between 5 and 10 min was observed at 3 and 5 mg L−1. This decline is potentially a result of hydrolysis (Mabey and Mill, 1978; Rahman, 2015), or dehalogenation (Hua and Reckhow, 2012; Rahman, 2015; Abusallout et al., 2017) of already formed tTHMs present in solution. The extent of tTHM degradation as a result of hydrolysis or dehalogenation over such a short contact time (i.e., 10 min) is unknown, however, the percentage composition of chloroform increases with reaction time, whilst brominated species decline (Figure 3). Bromine-carbon bonds are more tolerant to dissociation, compared to chlorine, as a result of lower dissociation energies (Abusallout et al., 2017). Dehalogenation is affected by pH, whereby, more alkaline conditions increase the rate of dehalogenation (Rahman, 2015). The decline in tTHMs was not observed with either HOCl or ECAS, potentially as a result of the neutral/acidic disinfectant properties, reducing the overall pH of the reaction (Figure 4). To better understand the formation of tTHMs over these short reaction times (<10 min) future studies could utilize real-time monitoring of formation and decay using selected ion flow tube mass spectrometry (SIFT-MS) (Smith and Španěl, 2005, 2011; Ioannidis et al., 2018).
The results of this study are in line with the limited number of other studies which have investigated THM formation in the presence of a neutral ECAS, when reacted with NOM (Ghebremichael et al., 2011), or within food processing applications (Gómez-López et al., 2013). A key difference between HOCl and ECAS is the antimicrobial mode of action. HOCl relies upon free chlorine for effective disinfection (Fair et al., 1948; National Academy of Sciences, 1980; Clasen and Edmondson, 2006), whereas ECAS relies upon a myriad of reactive oxidative chemical species such as OH−, O3, H2O2, and (Jeong et al., 2006; Martínez-Huitle and Brillas, 2008), in addition to free chlorine, resulting in a high ORP (>+1,130 mV; Suslow, 2004). This high ORP is known to result in the rupture of inner and outer microbial membranes, prohibiting microbial functionality, such as the energy generating mechanism (Liao et al., 2007). Therefore, to achieve the same levels of disinfection, lower free chlorine concentrations of ECAS are required in comparison to HOCl, or indeed NaOCl, therefore reducing the potential for THM formation (Di Cristo et al., 2013). In addition, ECAS can be generated on site and in-situ, requiring only water, salt and energy to produce the disinfectant (Kim et al., 2000; Huang et al., 2008; Thorn et al., 2012; Clayton et al., in press). Whereas, conventional treatment systems require the transport, storage and use of, potentially hazardous chemicals such as NaOCl or chlorine gas, which can have short “shelf-lives” if stored incorrectly (direct sunlight or inadequate closure) whereby the antimicrobial efficacy can decay over time (Clarkson et al., 2001). The bespoke ECAS generator (60 L h−1) used in this study has an operating current range of between 4 and 30 amps, therefore the power requirement ranges between 0.345 kW (4 amps) −0.69 kW (30 amps). Alternative ECAS generators are available which have lower power requirements, and can generate ECAS utilizing solar power (Witt and Reiff, 1993; Centrego, 2015). Such alternatives provide more practical solutions for remote locations, or as part of disaster relief efforts.
Ensuring sufficient disinfection in conventional centralized drinking water treatment and distribution systems (i.e., sodium hypochlorite or calcium hypochlorite) a residual free chlorine concentration of 0.5 mg L−1 is required after a contact time of 30 min (World Health Organization, 2011). Disinfectant contact in excess of minutes can cause residual free chlorine to react with NOM present in treated water, or within organic material (e.g., biofilms), in distribution network pipeworks, leading to the formation of THMs, or other DBPs. Limiting the contact time, reducing the pH of the disinfectant used (HOCl or ECAS), and reducing the organic load (i.e., humic acid or biofilm) within bulk water can help to reduce the formation of DBPs/THMs through drinking water disinfection processes (Amy et al., 1987; Brown et al., 2011a; Di Cristo et al., 2013). The results of this study have shown that ECAS treatment of water could lead to reduced formation of THMs, or other DBPs, compared to traditional chlorination (NaOCl). Further investigations are required to determine whether this observation occurs in scaled-up POU water treatment systems, as well as the role of pH, specifically within the treated water holding tank, in comparison to traditional clearwells. In addition, the free chlorine concentration of ECAS used within water treatment systems can be lower compared to conventional chlorination solutions, due to comparatively higher efficacy as a result of a high ORP and the presence of other transient oxidative functional groups. As such, this integral THM precursor will be reduced at the point of disinfection, diminishing the potential for THM formation.
Formation of tTHMs were quantified for three disinfectants, NaOCl, HOCl, and ECAS, when reacted with NOM for 1, 5, and 10 min. NaOCl produced the highest concentration of THMs across all reaction times and free chlorine concentrations (1, 3, and 5 mg L−1). The reaction between NOM, and ECAS or HOCl, formed comparable tTHM concentrations, significantly lower than NaOCl at all free chlorine concentrations and contact times. Chloroform was the dominant THM species formed for all three disinfectants (NaOCl, HOCl, and ECAS) at all contact times and free chlorine concentrations tested. The analytical technique employed in this study (HS SPME GC/MS) appropriately allowed for the comparison of tTHMs formed in the presence of three disinfectants in model water. As part of any future work, where a more quantitative approach is required (i.e., focusing on individual THM species formation), then an alternative analytical technique, such as GC-ECD, could be employed.
The comparative disinfection properties of ECAS, at point of generation, including reduced free chlorine compared to conventional disinfectants, high ORP (+1,130 mV), and rapid reaction time (<10 s), considerably reduces key precursors required for THM formation i.e., contact time and free chlorine availability. It has been shown that generating and storing ECAS in a reservoir tank, and dosing a low concentration (v/v) within a decentralized POU drinking water production system, is effective at producing Drinking Water Inspectorate drinking water (Clayton et al., in press). ECAS can be generated as a concentrated stock, and continuously dosed in-line to achieve the desired final concentration within the bulk water. No tTHMs were detected after a 1 min contact time, and only low concentrations of tTHMs were detected after 5 and 10 min, which were considerably lower than the maximum guideline value permissible in drinking water. Therefore, ECAS could be considered a safe alternative to conventional chorine disinfection for decentralized point-of-use water treatment systems, as free chlorine concentrations can be lower compared to conventional chlorination solutions due to comparative higher efficacy. This reduces an integral THM precursor, decreasing the formation potential of THMs.
This study focussed on the quantification of four specific THMs (chloroform, BDCM, DBCM, and bromoform). As such, other associated THM derivatives or DBPs were not identified, and identification of these possible derivatives would be of interest. Further work is required to determine any DBP derivatives that may form in place of, or in addition to, THMs. Finally, scaled-up testing is required to determine whether the results obtained in this study are representative within decentralized POU water treatment systems.
All datasets generated for this study are included in the manuscript and/or the supplementary files.
GC: method development, data collection and analysis, writing—original draft manuscript preparation, editing and review; DR: conceptualization, methodology; RT and DR: supervision, funding acquisition, writing—review and editing.
This study was funded equally by the University of the West of England, Bristol, and Portsmouth Aviation Ltd.
The authors declare that the research was conducted in the absence of any commercial or financial relationships that could be construed as a potential conflict of interest.
The authors would like to thank Paul Bowdler and Dr. Natasha McGuire for their assistance and support.
Abusallout, I., Rahman, S., and Hua, G. (2017). Effect of temperature and pH on dehalogenation of total organic chlorine, bromine and iodine in drinking water. Chemosphere. 187, 11–18. doi: 10.1016/j.chemosphere.2017.07.149
Amy, G. L., Chadik, P. A., and Chowdhury, Z. K. (1987). Developing models for predicting trihalmethane formation potential and kinetics. J. Am. Water Work. Assoc. 79, 89–97. doi: 10.1002/j.1551-8833.1987.tb02878.x
Antoniou, C. V., Koukouraki, E. E., and Diamadopoulos, E. (2006). Determination of chlorinated volatile organic compounds in water and municipal wastewater using headspace–solid phase microextraction–gas chromatography. J. Chromatogr. A 1132, 310–314. doi: 10.1016/j.chroma.2006.08.082
Ardakanian, R., Liebe, J., and Bernhart, L. M. (2015). Water for Life 2005 - 2015: Report on the Achievements During the International Decade for Action. Germany: UN-Water. Available online at: http://www.unwater.org/publications/report-achievements-international-decade-action-water-life-2005-2015/
Attisani, M. (2016). Can solar technology generate clean water for developing nations? Renew. Energy Focus 17, 138–139. doi: 10.1016/j.ref.2016.07.003
Boggio, R., Carugati, A., Lodi, G., and Trasatti, S. (1985). Mechanistic study of C12 evolution at Ti-supported Co3O4 anodes. J. Appl. Electrochem. 15, 335–349. doi: 10.1007/BF00615986
Boggs, S., Livermore, D., and Seltz, M. G. (1985). Humic Substances in Natural Waters and Their Complexation with Trace Metals and Radionuclides: A Review. Available online at: http://www.iaea.org/inis/collection/NCLCollectionStore/_Public/17/012/17012358.pdf (Accessed July 16, 2018).
Bove, G. E., Rogerson, P. A., and Vena, J. E. (2007). Case control study of the geographic variability of exposure to disinfectant byproducts and risk for rectal cancer. Int. J. Health Geogr. 6:18. doi: 10.1186/1476-072X-6-18
British Standards Institution (2005). Chemical Disinfectants and Antiseptics — Quantitative Suspension Test for the Evaluation of Basic Bactericidal Activity of Chemical Disinfectants and Antiseptics — Test Method and Requirements (Phase 1). Available online at: https://shop.bsigroup.com/ProductDetail/?pid=000000000030129220
British Standards Institution (2016). Determination of Volatile Organic Compounds in Water — Method Using Headspace Solid- Phase Micro- Extraction (HS-SPME) Followed by Gas Chromatography-Mass Spectrometry (GC-MS).
Brown, D., Bridgeman, J., and West, J. R. (2011a). Predicting chlorine decay and THM formation in water supply systems. Environ. Sci. Biotechnol. 10, 79–99. doi: 10.1007/s11157-011-9229-8
Brown, D., Bridgeman, J., and West, J. R. (2011b). Understanding data requirements for trihalomethane formation modelling in water supply systems. Urban Water J. 8, 41–56. doi: 10.1080/1573062X.2010.546863
Brown, D., West, J. R., Courtis, B. J., and Bridgeman, J. (2010). Modelling THMs in water treatment and distribution systems. Proc. Inst. Civ. Eng. Water Manag. 163, 165–174. doi: 10.1680/wama.2010.163.4.165
Burke, L. D., and O'Neill, J. F. (1979). Some aspects of the chlorine evolution reaction at ruthenium dioxide anodes. J. Electroanal. Chem. Interfacial Electrochem. 101, 341–349.
Cabral, J. P. S. (2010). Water microbiology. Bacterial pathogens and water. Int. J. Environ. Res. Public Health 7, 3657–3703. doi: 10.3390/ijerph7103657
Cai, Z. (2005). Characterisation of Electrochemically Activated Solutions for Use in Environmental Remediation. Available online at: https://ethos.bl.uk/OrderDetails.do?uin=uk.bl.ethos.418216 (Accessed February 21, 2019).
Carratalà, A., Dionisio Calado, A., Mattle, M. J., Meierhofer, R., Luzi, S., and Kohn, T. (2016). Solar disinfection of viruses in polyethylene terephthalate bottles. Appl. Environ. Microbiol. 82, 279–288. doi: 10.1128/AEM.02897-15
Centrego (2015). Centrego Water Tank Guard. Available online at: https://cdn.shopify.com/s/files/1/1891/3905/files/Water_Tank_Guard_V2A.pdf?7388658025877884720 (Accessed February 6, 2019).
Cho, D.-H. H., Kong, S.-H. H., and Oh, S.-G. G. (2003). Analysis of trihalomethanes in drinking water using headspace-SPME technique with gas chromatography. Water Res. 37, 402–408. doi: 10.1016/S0043-1354(02)00285-3
Chowdhury, S. (2013). Trihalomethanes in drinking water: effect of natural organic matter distribution. Water. 39, 1–8. doi: 10.4314/wsa.v39i1.1
Chowdhury, S., and Champagne, P. (2008). An investigation on parameters for modeling THMs formation. Glob. NEST. 10, 80–91. doi: 10.30955/gnj.000518
Chowdhury, S., Rodriguez, M. J., and Sadiq, R. (2011). Disinfection byproducts in Canadian provinces: associated cancer risks and medical expenses. J. Hazard. Mater. 187, 574–584. doi: 10.1016/j.jhazmat.2011.01.085
Clarkson, R., Moule, A., and Podlich, H. (2001). The shelf-life of sodium hypochlorite irrigating solutions. Aust. Dent. J. 46, 269–276. doi: 10.1111/j.1834-7819.2001.tb00291.x
Clasen, T., and Edmondson, P. (2006). Sodium dichloroisocyanurate (NaDCC) tablets as an alternative to sodium hypochlorite for the routine treatment of drinking water at the household level. Int. J. Hyg. Environ. Health 209, 173–181. doi: 10.1016/j.ijheh.2005.11.004
Clayton, G. E., Thorn, R. M. S., and Reynolds, D. M. (in press). Development of a novel off-grid drinking water production system integrating electrochemically activated solutions ultrafiltration membranes. J. Water Process Eng. doi: 10.1016/j.jwpe.2017.08.018
Corcoran, E., Nellemann, C., Baker, R., Bos, R., Osborn, D., and Savelli, H. (2010). “Sick water?” in The Central Role of Wastewater Management in Sustainable Development, eds E. Corcoran, C.Nellemann, E. Baker, R. Bos, D. Osborn, and H. Savelli (United Nations Environmental Programme; United Nations HABITAT and GRID-Arendal). Available online at: https://wedocs.unep.org/bitstream/handle/20.500.11822/9156/Sick%20Water.pdf?sequence=1&isAllowed=y (Accessed January 14, 2016).
David, D. (2014). The Effects of Alkalinity, Hardness, and pH on the Formation Potential of Disinfection By-Products. Available online at: https://mospace.umsystem.edu/xmlui/bitstream/handle/10355/44419/research.pdf?sequence=1andisAllowed=y (Accessed June 20, 2018).
Demiral, C. U., Bekbolet, M., and Swietlik, J. (2006). “Natural organic matter : definitions and characterization,” in Advances in Control of Disinfection By-Products in Drinking Water Systems, eds A. Nikolau, L. Rizzo and H. Selcuk (New York, NY: Nova Science Publishers, Inc), 26.
Di Cristo, C., Esposito, G., and Leopardi, A. (2013). Modelling trihalomethanes formation in water supply systems. Environ. Technol. 34, 61–70. doi: 10.1080/09593330.2012.679702
Dodds, L., and King, W. (2001). Relation between trihalomethane compounds and birth defects. Occup. Environ. Med. 58, 443–446. doi: 10.1136/oem.58.7.443
Domènech, L. (2011). Rethinking water management: from centralised to decentralised water supply and sanitation models. Doc. d'Anàlisi Geogr. 57, 293–310. doi: 10.5565/rev/dag.280
Drinking Water Inspectorate (2012). Guidance on the Implementation of the Water Supply (Water Quality) Regulations 2000 (as Amended) in England. London: Drinking Water Inspectorate.
Drinking Water Inspectorate (2018). List of Approved Products for Use in Public Water Supply in the United Kingdom. Available online at: http://dwi.defra.gov.uk/drinking-water-products/approved-products/soslistcurrent.pdf
Emmert, G. L., Geme, G., Brown, M. A., and Simone, P. S. (2009). A single automated instrument for monitoring total trihalomethane and total haloacetic acid concentrations in near real-time. Anal. Chim. Acta 656, 1–7. doi: 10.1016/j.aca.2009.10.002
Environmental Protection Agency (2011). Water Treatment Manual: Disinfection. Available online at: https://www.epa.ie/pubs/advice/drinkingwater/Disinfection2_web.pdf (Accessed March 10, 2017).
Erenburg, R., Krishtalik, L., and Rogozhina, N. (1984). pH effect on chlorine reaction kinetics on ruthenium titanium oxide anode. Elekrokhimiya 20:1183.
Fair, G. M., Morris, J. C., Chang, S. L., Weil, I., and Burden, R. P. (1948). The behavior of chlorine as a water disinfectant. J. Am. Water Work. Assoc. 40, 1051–1061.
Farghaly, A. M., Ahmed, A. M., Gad, A. A., and Hashem, M. A. (2013). A study for producing drinking water with safe trihalomethane concentrations. Clean Technol. Environ. Policy 16, 807–818. doi: 10.1007/s10098-013-0672-9
Gadad, P., Nanny, M. A., Lei, H., and Nanny, M. A. (2007). Characterization of noncovalent interactions between 6-propionyl-2-dimethylaminonaphthalene (PRODAN) and dissolved fulvic and humic acids. Water Res. 41, 4488–4496. doi: 10.1016/j.watres.2007.06.011
Ghebremichael, K., Muchelemba, E., Petrusevski, B., and Amy, G. (2011). Electrochemically activated water as an alternative to chlorine for decentralized disinfection. J. Water Supply Res. Technol. Aqua 60, 210–218. doi: 10.2166/aqua.2011.034
Gómez-López, V. M., Marín, A., Medina-Martínez, M. S., Gil, M. I., and Allende, A. (2013). Generation of trihalomethanes with chlorine-based sanitizers and impact on microbial, nutritional and sensory quality of baby spinach. Postharvest Biol. Technol. 85, 210–217. doi: 10.1016/j.postharvbio.2013.05.012
Grazuleviciene, R., Kapustinskiene, V., Vencloviene, J., Buinauskiene, J., and Nieuwenhuijsen, M. J. (2013). Risk of congenital anomalies in relation to the uptake of trihalomethane from drinking water during pregnancy. Occup. Environ. Med. 70, 274–282. doi: 10.1136/oemed-2012-101093
Grunwald, A., Nikolaou, A. D., Golfinopoulos, S. K., and Lekkas, T. D. (2002). Formation of organic by-products during chlorination of natural waters. J. Environ. Monit. 4, 910–916. doi: 10.1039/B202965K
Guilherme, S., and Rodriguez, M. J. (2015). Short-term spatial and temporal variability of disinfection by-product occurrence in small drinking water systems. Sci. Total Environ. 518–519, 280–289. doi: 10.1016/j.scitotenv.2015.02.069
Health Canada (2017). Guidelines for Canadian Drinking Water Quality - Summary Table. Ottawa, ON. Available online at: https://www.canada.ca/content/dam/hc-sc/migration/hc-sc/ewh-semt/alt_formats/pdf/pubs/water-eau/sum_guide-res_recom/sum_guide-res_recom-eng.pdf (Accessed April 23, 2018).
Heeb, M. B., Criquet, J., Zimmermann-Steffens, S. G., and von Gunten, U. (2014). Oxidative treatment of bromide-containing waters: formation of bromine and its reactions with inorganic and organic compounds — a critical review. Water Res. 48, 15–42. doi: 10.1016/j.watres.2013.08.030
Hua, G., and Reckhow, D. A. (2007). Comparison of disinfection byproduct formation from chlorine and alternative disinfectants. Water Res. 41, 1667–1678. doi: 10.1016/j.watres.2007.01.032
Hua, G., and Reckhow, D. A. (2012). Effect of alkaline pH on the stability of halogenated DBPs. J. Am. Water Works Assoc. 104, 49–50. doi: 10.5942/jawwa.2012.104.0025
Huang, Y. W. Y. R., Hung, Y. C., Hsu, S. Y., Huang, Y. W. Y. R., and Hwang, D. F. (2008). Application of electrolyzed water in the food industry. Food Control 19, 329–345. doi: 10.1016/j.foodcont.2007.08.012
Ikem, A. (2010). Measurement of volatile organic compounds in bottled and tap waters by purge and trap GC-MS: are drinking water types different? J. Food Compos. Anal. 23, 70–77. doi: 10.1016/j.jfca.2009.05.005
Inoue, Y., Endo, S., Kondo, K., Ito, H., Omori, H., and Saito, K. (1997). Trial of electrolyzed strong acid aqueous solution lavage in the treatment of peritonitis and intraperitoneal abscess. Artif. Organs 21, 28–31. doi: 10.1111/j.1525-1594.1997.tb00695.x
Ioannidis, K., Niazi, S., Deb, S., Mannocci, F., Smith, D., and Turner, C. (2018). Quantification by SIFT-MS of volatile compounds produced by the action of sodium hypochlorite on a model system of infected root canal content. PLoS ONE 13:e0198649. doi: 10.1371/journal.pone.0198649
Jain, S., Sahanoon, O. K., Blanton, E., Schmitz, A., Wannemuehler, K. A., Hoekstra, R. M., et al. (2010). Sodium dichloroisocyanurate tablets for routine treatment of household drinking water in periurban Ghana: a randomized controlled trial. Am. J. Trop. Med. Hyg. 82, 16–22. doi: 10.4269/ajtmh.2010.08-0584
Jeong, J., Kim, J. Y., and Yoon, J. (2006). The role of reactive oxygen species in the electrochemical inactivation of microorganisms. Environ. Sci. Technol. 40, 3–4. doi: 10.1021/es0604313
Kerwick, M. I., Reddy, S. M., Chamberlain, A. H. L., and Holt, D. M. (2005). Electrochemical disinfection, an environmentally acceptable method of drinking water disinfection? Electrochim. Acta 50, 5270–5277. doi: 10.1016/j.electacta.2005.02.074
Kim, C., Hung, Y. C., and Brackett, R. E. (2000). Roles of oxidation-reduction potential in electrolyzed oxidizing and chemically modified water for the inactivation of food-related pathogens. J. Food Prot. 63, 19–24. doi: 10.4315/0362-028X-63.1.19
Kim, J., Chung, Y., Shin, D., Kim, M., Lee, Y., Lim, Y., et al. (2002). Chlorination by-products in surface water treatment process. Desalination 151, 1–9. doi: 10.1016/S0011-9164(02)00967-0
King, W. D., Dodds, L., and Allen, A. C. (2000). Relation between stillbirth and specific chlorination by-products in public water supplies. Environ. Health Perspect. 108, 883–886. doi: 10.1289/ehp.00108883
Kumari, M., and Gupta, S. K. (2015). Modeling of trihalomethanes (THMs) in drinking water supplies: a case study of eastern part of India. Environ. Sci. Pollut. Res. 22, 12615–12623. doi: 10.1007/s11356-015-4553-0
Liang, L., and Singer, P. C. (2003). Factors influencing the formation and relative distibution of haloacetic acids and trihalomethanes in drinking water. Environ. Sci. Technol. 37, 2920–2928. doi: 10.1021/es026230q
Liao, L. B., Chen, W. M., and Xiao, X. M. (2007). The generation and inactivation mechanism of oxidation–reduction potential of electrolyzed oxidizing water. J. Food Eng. 78, 1326–1332. doi: 10.1016/j.jfoodeng.2006.01.004
Liao, X., Xuan, X., Li, J., Suo, Y., Liu, D., Ye, X., et al. (2017). Bactericidal action of slightly acidic electrolyzed water against Escherichia coli and Staphylococcus aureus via multiple cell targets. Food Control 79, 380–385. doi: 10.1016/j.foodcont.2017.03.050
Liu, Q., and Margerum, D. W. (2001). Equilibrium and kinetics of bromine chloride hydrolysis. Environ. Sci. Technol. 35, 1127–1133. doi: 10.1021/es001380r
Mabey, W., and Mill, T. (1978). Critical review of hydrolysis of organic compounds in water under environmental conditions. J. Phys. Chem. Ref. Data 7, 383–415. doi: 10.1063/1.555572
Martínez-Huitle, C. A., and Brillas, E. (2008). Electrochemical alternatives for drinking water disinfection. Angew. Chem. Int. Ed. 47, 1998–2005. doi: 10.1002/anie.200703621
Mbilinyi, B. P., Tumbo, S. D., Mahoo, H. F., Senkondo, E. M., and Hatibu, N. (2005). Indigenous knowledge as decision support tool in rainwater harvesting. Phys. Chem. Earth Parts A B C. 30, 792–798. doi: 10.1016/j.pce.2005.08.022
Morita, C., Sano, K., Morimatsu, S., Kiura, H., Goto, T., Kohno, T., et al. (2000). Disinfection potential of electrolyzed solutions containing sodium chloride at low concentrations. J. Virol. Methods. 85, 163–174. doi: 10.1016/S0166-0934(99)00165-2
National Academy of Sciences (1980). Drinking Water and Health, 2nd Edn. Washington, DC: National Academic Press.
Peters, C. J., Young, R. J., and Perry, R. (1980). Factors influencing the formation of haloforms in the chlorination of humic materials. Environ. Sci. Technol. 14, 1391–1395.
Peter-Varbanets, M., Zurbrügg, C., Swartz, C., and Pronk, W. (2009). Decentralized systems for potable water and the potential of membrane technology. Water Res. 43, 245–265. doi: 10.1016/j.watres.2008.10.030
Pooi, C. K., and Ng, H. Y. (2018). Review of low-cost point-of-use water treatment systems for developing communities. npj Clean Water 1:11. doi: 10.1038/s41545-018-0011-0
Rahman, M. B., Driscoll, T., Cowie, C., and Armstrong, B. K. (2010). Disinfection by-products in drinking water and colorectal cancer: a meta-analysis. Int. J. Epidemiol. 39, 733–745. doi: 10.1093/ije/dyp371
Rahman, S. S. (2015). Effect of pH and temperature on halogenated DBPs. PRAIRIE: Open Public Research Access Institutional Repository and Information Exchange. doi: 10.1038/2001235b0
Rasheed, S., Hashmi, I., Kim, J. K., Zhou, Q., and Campos, L. C. (2017). Species specific interaction of trihalomethane (THM) precursors in a scaled up distribution network using response surface methodology (RSM). Environ. Technol. 39:1–27. doi: 10.1080/09593330.2017.1301564
Robinson, G., Thorn, R., and Reynolds, D. (2013). The effect of long-term storage on the physiochemical and bactericidal properties of electrochemically activated solutions. Int. J. Mol. Sci. 14, 457–469. doi: 10.3390/ijms14010457
Robinson, G. M., Lee, S. W.-H., Greenman, J., Salisbury, V. C., and Reynolds, D. M. (2010). Evaluation of the efficacy of electrochemically activated solutions against nosocomial pathogens and bacterial endospores. Lett. Appl. Microbiol. 50, 289–294. doi: 10.1111/j.1472-765X.2009.02790.x
Robinson, G. M., Tonks, K. M., Thorn, R. M. S., and Reynolds, D. M. (2011). Application of bacterial bioluminescence to assess the efficacy of fast-acting biocides. Antimicrob. Agents Chemother. 55, 5214–5219. doi: 10.1128/AAC.00489-11
Rodriguez, M. J., and Serodes, J.-B. (2001). Spatial and temporal evolution of trihalomethanes in three water distribution systems. Water Res. 35:1572. doi: 10.1016/S0043-1354(00)00403-6
Rossman, L. A., Brown, R. A., Singer, P. C., and Nuckols, J. R. (2001). DBP formation kinetics in a simulated distribution system. Water Res. 35, 3483–3489. doi: 10.1016/S0043-1354(01)00059-8
Saidan, M., Rawajfeh, K., and Fayyad, M. (2013). Investigation of factors affecting THMs formation in drinking water. Am. J. Environ. Eng. 3, 207–212. doi: 10.5923/j.ajee.20130305.02
Schlichter, B., Mavrov, V., and Chmiel, H. (2004). Study of a hybrid process combining ozonation and microfiltration/ultrafiltration for drinking water production from surface water. Desalination. 168, 307–317. doi: 10.1016/j.desal.2004.07.014
Siddique, A., Saied, S., Mumtaz, M., Hussain, M. M., and Khwaja, H. A. (2015). Multipathways human health risk assessment of trihalomethane exposure through drinking water. Ecotoxicol. Environ. Saf. 116, 129–136. doi: 10.1016/j.ecoenv.2015.03.011
Sivey, J. D., McCullough, C. E., and Roberts, A. L. (2010). Chlorine Monoxide (Cl 2 O) and molecular chlorine (Cl 2) as active chlorinating agents in reaction of dimethenamid with aqueous free chlorine. Environ. Sci. Technol. 44, 3357–3362. doi: 10.1021/es9038903
Smith, D., and Španěl, P. (2005). Selected ion flow tube mass spectrometry (SIFT-MS) for on-line trace gas analysis. Mass Spectrom. Rev. 24, 661–700. doi: 10.1002/mas.20033
Smith, D., and Španěl, P. (2011). Ambient analysis of trace compounds in gaseous media by SIFT-MS. Analyst 136:2009. doi: 10.1039/c1an15082k
Stevens, A. A., Slocum, C. J., Seeger, D. R., and Robeck, G. (1976). Chlorination of organics in drinking water. J. Am. Water Works Assoc. 68, 615–620. doi: 10.1002/j.1551-8833.1976.tb02506.x
Stoner, G. E., Cahen, G. L., Sachyani, M., and Gileadi, E. (1982). The Mechanism of Low-frequency A.C. electrochemical disinfection. Bioelectrochem. Bioenerg. 9, 229–243. doi: 10.1016/0302-4598(82)80013-5
Suslow, T. V. (2004). Oxidation-reduction potential (ORP) for water disinfection monitoring, control, and documentation. ANR Publ. 8149, 1–5. doi: 10.3733/ucanr.8149
The Council of the European Union (1998). Council directive 98/83/EC of 3 November 1998 on the quality of water intended for human consumption. Off. J. Eur. Commun. L330, 32–54.
The Environment Agency (2002). The Microbiology of Drinking Water (2002) -Part 1 - Water Quality and Public Health Methods for the Examination of Waters and Associated Materials.
Thorn, R. M. S., Lee, S. W. H., Robinson, G. M., Greenman, J., and Reynolds, D. M. (2012). Electrochemically activated solutions: evidence for antimicrobial efficacy and applications in healthcare environments. Eur. J. Clin. Microbiol. Infect. Dis. 31, 641–653. doi: 10.1007/s10096-011-1369-9
Thorn, R. M. S., Robinson, G. M., and Reynolds, D. M. (2013). Comparative antimicrobial activities of aerosolized sodium hypochlorite, chlorine dioxide, and electrochemically activated solutions evaluated using a novel standardized assay. Antimicrob. Agents Chemother. 57, 2216–2225. doi: 10.1128/AAC.02589-12
Trasatti, S. (1987). Progress in the understanding of the mechanism of chlorine evolution at oxide electrodes. Electrochim. Acta 32, 369–382. doi: 10.1016/0013-4686(87)85001-6
Trasatti, S. (1991). Physical electrochemistry of ceramic oxides. Electrochim. Acta 36, 225–241. doi: 10.1016/0013-4686(91)85244-2
Unicef and World Health Organization (2014). Progress on Drinking Water and Sanitation - 2014 Update. Geneva: World Health Organization.
Urano, K., Wada, H., and Takemasa, T. (1983). Empirical rate equation for trihalomethane formation with chlorination of humic substances in water. Water Res. 17, 1797–1802. doi: 10.1016/0043-1354(83)90202-6
USEPA (2010). Comprehensive Disinfectants and Disinfection Byproducts Rules (Stage 1 and Stage 2): Quick Reference Guide Overview of the Rules. United States Environmental Protection Agency.
Venczel, L. V., Arrowood, M., Hurd, M., and Sobsey, M. (1997). Inactivation of Cryptosporidium parvum oocysts and Clostridium perfringens spores by a mixed-oxidant disinfectant and by free chlorine. Appl. Environ. Microbiol. 63, 1598–1601.
Venczel, L. V., Likirdopulos, C. A., Robinson, C. E., and Sobsey, M. D. (2004). Inactivation of enteric microbes in water by electro-chemical oxidant from brine (NaCl) and free chlorine. Water Sci. Technol. 50, 141–146. doi: 10.2166/wst.2004.0042
Wang, G.-S., Deng, Y.-C., and Lin, T.-F. (2007). Cancer risk assessment from trihalomethanes in drinking water. Sci. Total Environ. 387, 86–95. doi: 10.1016/j.scitotenv.2007.07.029
Werner, D., Valdivia-Garcia, M., Weir, P., and Haffey, M. (2016). Trihalomethanes formation in point of use surface water disinfection with chlorine or chlorine dioxide tablets. Water Environ. J. 30, 271–277. doi: 10.1111/wej.12209
Witt, V. M., and Reiff, F. M. (1993). La Desinfección del Agua a Nivel Casero en Zonas Urbanas Marginales y Rurales. Washington DC.
World Health Organization (2000). Environmental Health Criteria 216 - Disinfectant and Disinfectant by-Products. Geneva.
World Health Organization (2015). Drinking-Water - Fact Sheet N°391. Available online at: http://www.who.int/mediacentre/factsheets/fs391/en/
Wright, J. M., Schwartz, J., and Dockery, D. W. (2004). The effect of disinfection by-products and mutagenic activity on birth weight and gestational duration. Environ. Health Perspect. 112, 920–925. doi: 10.1289/ehp.6779
Yang, L., Hur, J., and Zhuang, W. (2015). Occurrence and behaviors of fluorescence EEM-PARAFAC components in drinking water and wastewater treatmentx systems and their applications: a review. Environ. Sci. Pollut. Res. 22, 6500–6510. doi: 10.1007/s11356-015-4214-3
Yee, L. F., Abdullah, M. P., Abdullah, A., Ishak, B., Nidzham, K., and Abidin, Z. (2009). Hydrophobicity characteristics of natural organic matter and the formation of THM. Malaysian J. Anal. Sci. 13, 94–99.
Keywords: electrochemically activated solutions, hypochlorous acid, point-of-use decentralized drinking water treatment, sodium hypochlorite, trihalomethane formation
Citation: Clayton GE, Thorn RMS and Reynolds DM (2019) Comparison of Trihalomethane Formation Using Chlorine-Based Disinfectants Within a Model System; Applications Within Point-of-Use Drinking Water Treatment. Front. Environ. Sci. 7:35. doi: 10.3389/fenvs.2019.00035
Received: 04 January 2019; Accepted: 28 February 2019;
Published: 22 March 2019.
Edited by:
Prosun Bhattacharya, Royal Institute of Technology, SwedenReviewed by:
Tiziana Tosco, Politecnico di Torino, ItalyCopyright © 2019 Clayton, Thorn and Reynolds. This is an open-access article distributed under the terms of the Creative Commons Attribution License (CC BY). The use, distribution or reproduction in other forums is permitted, provided the original author(s) and the copyright owner(s) are credited and that the original publication in this journal is cited, in accordance with accepted academic practice. No use, distribution or reproduction is permitted which does not comply with these terms.
*Correspondence: Darren M. Reynolds, ZGFycmVuLnJleW5vbGRzQHV3ZS5hYy51aw==
Disclaimer: All claims expressed in this article are solely those of the authors and do not necessarily represent those of their affiliated organizations, or those of the publisher, the editors and the reviewers. Any product that may be evaluated in this article or claim that may be made by its manufacturer is not guaranteed or endorsed by the publisher.
Research integrity at Frontiers
Learn more about the work of our research integrity team to safeguard the quality of each article we publish.