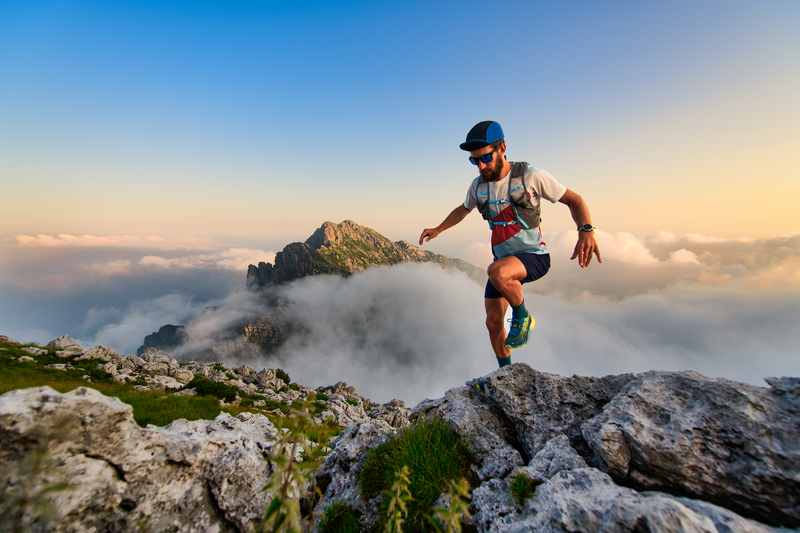
95% of researchers rate our articles as excellent or good
Learn more about the work of our research integrity team to safeguard the quality of each article we publish.
Find out more
REVIEW article
Front. Environ. Sci. , 27 February 2019
Sec. Freshwater Science
Volume 7 - 2019 | https://doi.org/10.3389/fenvs.2019.00025
This article is part of the Research Topic Ecohydraulics and Morphodynamics of Water Systems View all 5 articles
During sea level rise, salt marshes transgress inland invading low-lying forests, agricultural fields, and suburban areas. This transgression is a complex process regulated by infrequent storms that flood upland ecosystems increasing soil salinity. As a result upland vegetation is replaced by halophyte marsh plants. Here we present a review of the main processes and feedbacks regulating the transition from upland ecosystems to salt marshes. The goal is to provide a process-based framework that enables the development of quantitative models for the dynamics of the marsh-upland boundary. Particular emphasis is given to the concept of ecological ratchet, combining the press disturbance of sea level rise with the pulse disturbance of storms.
The retreat of coastal forests and farmland as sea level rises and their replacement with salt marshes is well documented (Williams et al., 1999a; DeSantis et al., 2007; Doyle et al., 2010; Smith, 2013; Kirwan et al., 2016); however, the mechanisms that control this retreat vary with the geomorphological, hydrological, and ecological setting of the marsh-forest interface. Trees and other upland vegetation can be killed by increased tidal flooding and storm surges, either because they are not flood-tolerant or because they are not tolerant to the salinity of flood waters. Saltwater intrusion in which the groundwater table becomes more saline, whether due to sea-level rise or groundwater withdrawals, can also lead to vegetation change (Pezeshki et al., 1990). Droughts can amplify these effects by reducing the supply of fresh water (DeSantis et al., 2007).
Sea-level rise and storms act at very different spatial and temporal scales on the marsh-upland boundary. Storms are a pulse disturbance that affects coastal forests in the short-term, damaging trees and triggering diebacks that make ecological space for new vegetation species. Sea level rise is a press disturbance, which alters in the long-term soil salinity and flooding regime thus favoring the growth of halophytic grasses. Both disturbances are instrumental in the dynamics of the marsh-upland boundary, and they will be presented in detail in sections The pulse disturbance: effect of storms on coastal forests and The press disturbance: sea level rise and marsh migration into forests.
In the ecological ratchet model presented in section The Ecological Ratchet Model of Marsh Migration these two processes are combined. In the ratchet model the transition zone between salt marsh and forest is defined by two boundaries. Above the upper boundary a healthy forest thrives while below the lower boundary only salt marshes are present. Between these limits the forest is replaced by marsh vegetation with a complex dynamics. In this area mature trees can persist but not regenerate and are eventually replaced by marsh vegetation. In the ratchet model sea-level rise moves the upper boundary into the forest, while storm surges advance the lower boundary, expanding the salt marshes.
The landuse and slope of marsh-adjoining areas are two other important factors that determine the rate of upslope migration of salt marshes. We will explore how different coastal slopes affect the dynamics of the marsh-upland boundary in the section The Effect of Landscape Slope on Marsh Migration. Sea level rise does not always trigger marsh migration, but when this happens the marsh directly replaces another land cover type such as agricultural, developed, or managed landscapes that formerly existed. Expansion of salt marshes in suburban lawns in presented in section The Effect of Urban Landuse on Marsh Migration.
Agricultural fields are more exposed to projected sea-level rise effects than grasslands or woodlands (Feng et al., 2018). For coastal communities around the world, sea-level rise is not only a major threat to urban populations (Guneralp et al., 2015), it also causes loss of salt marshes (Kirwan et al., 2010) and loss of farmland through its conversion to salt marshes or mangroves (Nicholls and Leatherman, 1995). In 1995, Nicholls and Leatherman predicted that a one-meter rise in sea-level would result in submergence of 12–15% Egypt's farmland, “tens of thousands of hectares” of agricultural land in China, and loss of 16% of national rice production in Bangladesh. More recently, Clark et al. (2015) report that in Bangladesh there are about 2.5 Mha of low-elevation coastal lands, defined as 0.9–2.1 meters above msl, of which approximately 1.51 Mha (53%) are already affected by increasing salinity, In section Marsh Migration and Farmland Loss we will briefly summarize how sea-level rise threatens agricultural fields, transforming them in saline wetlands.
The conversion from one land cover type into another alters the value of the land. This change in value determines how society will respond to marsh migration. If the value of the ecosystem services provided by the marsh is higher than the value of the upland landuse then marsh migration will not be impeded; if the upland value is higher, coastal communities will try to stop marsh migration, by building sea walls and other hard structures. In section The Economics of Marsh Migration in the USA: a Private vs. Public Zero-Sum Game we will address this important issue and how a cost-benefit analysis of marsh migration can be implemented. A section listing the gaps in our understanding of marsh migration and the most pressing research needs is closing this review.
Global warming not only triggers an increase in sea level, but likely augments the intensity and frequency of storms near the shore (Webster et al., 2005). Storms, and in particular tropical cyclones, can affect the health of coastal forests, speeding up their conversion to salt marshes. In particular, storm surges are magnified by sea level rise, since the frequency and magnitude of flooding dramatically increases when sea level is higher. It is therefore impossible to neglect the effect of storms on the dynamics of the marsh-forest boundary, both in terms of wind damage and flooding, because storms and sea level rise are intertwined consequences of global warming.
The first step in the conversion of forests to salt marsh is the elimination of the forest canopy. Growth of marsh grasses is hindered under the shade of trees, and forest dieback is necessary to provide enough light for the invading species. Very often large forest diebacks at the marsh boundary is caused by storms and hurricanes that kill the trees. In this section we will explore how storms can affect forests, paving the way to marsh transgression.
Along the U.S. Atlantic and Gulf of Mexico coasts, hurricanes have largely damaged forest structure in regions around its landfall location as observed over the last few decades with the advancement of satellite imageries (Wang and Xu, 2009; Hu and Smith, 2018). Strong winds and inundation by storm surge and tide (up to 10 ft above ground level) during Hurricane Harvey caused extensive vegetation damage near Texas (Blake and Zelinsky, 2018). Reports of foliage loss, trees being uprooted, broken, and fallen during hurricanes Irma and Maria have emerged (Cangialosi et al., 2018; Hu and Smith, 2018; Pasch et al., 2018). Similar damage has also been described during hurricanes Sandy and Katrina in the recent past (Chapman et al., 2008; Middleton, 2016). Forests are primarily affected by two types of perils: strong winds and storm surges associated with hurricanes.
Sustained winds and gusts associated with storms alter the structure and dynamics of forests in various ways, including but not limited to defoliation, breakage, and uprooting (Duryea et al., 2007). As the hurricanes make landfall, changes in wind direction and gust intensity affect the transfer of energy from the wind to the tree crown (Drouineau et al., 2000). This most commonly results in defoliation. Trees sway, twist and rock, which stresses their stem and root system (Merry et al., 2009). Individual stems may bend, or if the compression strength of the wood is exceeded, they break or uproot (Merry et al., 2009). Salt spray may also injure the tree crown (Wells and Shunk, 1938).
Storm surges exert multiple stresses on pine forests by saltwater intrusion (Fernandes et al., 2018). Flooding can affect the soil structure, deplete soil O2, accumulate CO2, reduce Fe, and Mn, produce potentially toxic compounds, and induce anaerobic decomposition of organic matter (Ponnamperuma, 1984; Kozlowski, 1997). As salts enter the soil, the increased salinity levels may lead to browning and loss of needles or leaves, along with a decrease in water uptake through roots, nitrogen retention, nutrient use efficiency, and physiochemical retention mechanisms, as seen in loblolly pines (Blood et al., 1991; Merry et al., 2009). High salinity and flooding can also suppress seed germination as well as vegetative and reproductive growth of the trees (Kozlowski, 1997).
Moreover, roots can be damaged by sedimentation and decreased supply of oxygen during flooding. Damage due to such root suffocation may involve inhibition of root formation, growth, and branching, as well as decay (DeBell et al., 1984; Kozlowski, 1985). Poor root aeration may also decrease the ability of trees to absorb nutrients (Shoulders and Ralston, 1975). Flooding also increases the activity of soil fungi which may lead to the development of root rot (Kozlowski, 1997). These stresses may cause root mortality.
In addition to saltwater intrusion, storm surges can also cause physical damage to trees by strong incoming waves. As the waves approach forests in the nearshore environment, they exert pressure on the trees. This force mechanically stresses the trees and causes bending, uprooting or breakage (Stanturf et al., 2007; Puijalon et al., 2011). Debris transported by waves can also damage the bark and underlying cambium creating flood scars (Phillips, 1999; Stoffel et al., 2010). Heavy rains associated with hurricanes affect pine forests too. Flooding can deplete soil O2 and cause tree mortality from anoxia (Stanturf et al., 2007). Furthermore, soil saturation by rainfall may increase the susceptibility of the trees to windthrow (Schaetzl et al., 1989). Damage and dieback of trees can facilitate the encroachment of halophytes plants and the transition from forest to marsh. However, other factors play a role in the transition, because in several locations the forest recovered fast after a hurricane disturbance (Everham and Brokaw, 1996).
Storms can interact with sea level rise in numerous ways to affect coastal forests. One such interaction is the increase in sea level, potentially increasing the storm surge heights and the spatial extent of saltwater intrusion (Smith et al., 2010; Woodruff et al., 2013). Flood index projections along the U.S. East Coast, accounting for changes in the flood magnitude and duration due to both sea level rise and storm surges, are found to be significantly higher (by about 25% in some representative scenarios of global warming) by the 2080–2099 period compared to the projections incorporating sea level rise alone (Little et al., 2015). In addition to the increased flood risk, storm surges can also cause changes in the local geomorphology by erosion or sedimentation (Williams et al., 1999a; Williams and Flanagan, 2009). The net effect of these changes in elevation alter how coastal vegetation interacts with sea level rise, increasing the vulnerability of the forest edge to rising seas (Williams et al., 1999b).
Storms trigger a plethora of biotic responses in forests including shift in ecological equilibrium and forest mortality (Lugo, 2008). Recovery of the forests following hurricanes may be short- or long-term, depending on the nature and severity of the damage. Trees have been observed to start recovering their foliage within a few weeks or months from the passage of a hurricane, which classifies as short-term recovery (Pimm et al., 1994; Everham and Brokaw, 1996). Recovery from physical damage (e.g., bending, root injury) or changes in soil salinity could take from a number of seasons to a few years. Changes in soil salinity can also lead to a shift in ecological space potentially creating non-optimal growth conditions. Furthermore, the effects of changes in soil salinity, uprooting and root damage may cause tree mortality.
Characterizing the susceptibility of different tree species to damage from hurricanes and their trajectories of response is difficult. Stands of longleaf pines are generally more resilient to wind damage compared to loblolly pines or pond pines due to their deep root system, providing them with additional stability (Gresham et al., 1991; Barry et al., 1993). Slash pines are generally more resistant to damage from salinity changes compared to longleaf pines and loblolly pines (Barry et al., 1993). There is no uniform response to all types of damage from various species of trees; however, they are all affected in one way or the other. In addition to the individual species characteristics, the response of a forest to a storm depends on storm characteristics, spatial distribution of trees, soil conditions, and topography (Gardner et al., 1991, 1992; Merry et al., 2009). Figure 1 summarizes the most probable pathways of storm damage on a forest, the amount of time it requires to recover or whether it leads to mortality.
Figure 1. A schematic of the most probable pathways of forest damage during storms. The final outcome is either recovery or mortality based on the nature of the damage, interface of interaction and its associated effects.
Violent storms can also modify the boundary between forest and salt marsh through erosion and sediment deposition. The geomorphic effect of storms on the marsh-boundary ecotone depends on the width of the marsh and the intensity of wind, waves and storm surges. In fact salt marshes dissipate wave energy very effectively (Knutson et al., 1982; Jadhav et al., 2013), with wave height reduced by 20% in the first 40 m of marsh (Möller et al., 2014). Therefore, extensive soil erosion in the forest can be expected only if the adjacent marsh is narrow, or the storm very energetic.
Deposition and soil accretion at the marsh-forest boundary is more common than erosion during storms. Hurricane surges have been recorded to deposit sediments in tropical forests (Whelan et al., 2009). Gardner et al. (1991) measured deposition of mud, up to 3 cm in thickness, as far as 1 km in a pine forest in South Carolina during hurricane Hugo. Loope et al. (1994) observed massive deposition of litter and debris in a freshwater swamp forest in Florida after hurricane Andrew, but minimal erosion due to the storm surge. Sustained deposition in the forest would increase elevation, partly mitigating the effect of sea level rise and flooding. However, contrary to salt marshes, there is no evidence for widespread deposition that enables the coastal forest to keep pace with sea level rise. When present, deposition seems limited to the first tens of meters near the forest boundary (Gardner et al., 1991).
Marsh erosion and forest transgression are affected differently by storms. Marsh erosion is caused by wind waves, and therefore a function of wind intensity, direction and fetch (Mariotti et al., 2010; Leonardi et al., 2016). Forest transgression is influenced by storm surges, which are moderated by the extent of marsh bordering the forest, and depends on the elevation of the forest. Although the ocean/marsh boundary has historically been relatively stable in some areas (e.g., Raabe and Stumpf, 2015), where marsh erosion is faster than forest retreat, storms may reduce marsh extent, exposing the forest to erosion by waves. Uneven shoreline retreat caused by waves can therefore affect the upland boundary. Long-term simulations of wave climate and associated morphological change (i.e., Marani et al., 2011; Leonardi et al., 2016) are therefore important in order to understand the evolution of the marsh-forest boundary.
Once forest dieback occurs, salt marsh species can invade the area. This is possible because sea level rise has changed the edaphic characteristics of the forest, so that the forest cannot regenerate. In the absence of sea-level rise, the forest would likely recover a dieback event caused by storms. In this section we explore how sea level rise can affect tree regeneration, thus paving the way for marsh migration.
Sea-level rise may favor the expansion of salt marshes in forests through several mechanisms. Encroachment of salt marsh species is generally favored by increased flooding and/or salt concentrations on marshy coastlines with low elevation gradients (Williams et al., 1999a, Figure 2). A complex set of interactions governs the salinity of the waters that forest trees are exposed to at coastal margins (Figure 2). Thus, both the proximal cause of forest decline and lack of regeneration at the coast and the composition of the marsh that replaces it (salt marsh or freshwater/oligohaline marsh) may vary along coastlines.
Simplistic bathtub models of sea-level rise can account for both increases in freshwater flooding and increases in salinity with sea-level rise, as long as other conditions affecting water flows and salinity do not change from average historical conditions (Figures 3A,B). This assumption is widely acknowledged to be unrealistic (Williams et al., 1999a; Anisfeld et al., 2016). Bathtub models often overestimate flooding; realistic models describing the propagation and attenuation of water levels are therefore required (e.g., Lentz et al., 2016; Rodríguez et al., 2017; Silvestri et al., 2018).
Figure 3. Interface between salt water and fresh water on (A) coastlines with high freshwater outflows, where swamp forest or hydric hardwood hammock meets freshwater marsh, (B) coastlines with lower freshwater outflow, where upland forest meets salt marsh, (C) coastlines where tree islands exist on topographic highs with a local rain-fed freshwater lens, and (D) coastlines with similar tree islands supplied by water outflows from large aquifers. (A,B) modified from Williams et al. (1999a).
Salt incursions into coastal swamp forests (from various causes) has been credited with tree death and failure of regeneration in those forests (e.g., Penfound and Hathaway, 1938), and freshwater outflows from extensive aquifers may maintain forest at unusually low elevations (Williams et al., 2007, Figure 3D). In general, however, changes in flooding and salinity, are credited with the suppression of tree regeneration, and the movement of the boundary between marsh and forest.
Forest replacement by marsh tends to proceed by a series of steps in which tree regeneration fails before mature tree death occurs (Clark, 1986; Conner and Day, 1988; Williams et al., 1999b), and thinning of the forest canopy occurs before marsh plants colonize the site to any great extent (Brinson et al., 1995; Williams et al., 1999b, 2007; Langston et al., 2017). These patterns occur because tree seedlings tend to be more susceptible to salt and flooding than mature trees (Williams et al., 1999b and references therein), and marsh species often have low shade tolerance (Brinson et al., 1995; Poulter et al., 2009). These stages of transition from healthy forest to coastal marsh may only be visible, however, on coastlines with very shallow elevation gradients (cf. Field et al., 2016).
In relatively salty areas, feedbacks from forest canopy thinning and colonization by halophytic vegetation may further salinize the site, leading to hypersaline conditions at the forest edge. Exposure of soil where coastal forest trees have died, may cause high surface evaporation that, when combined with low tidal flushing at the higher fringes of the marsh, may concentrate salts. Hence, the high salt marsh and barren flats at the forest edge frequently have the highest soil salinity across the marsh/forest ecotone (Kurz and Wagner, 1957). Additionally, halophytes that colonize salty or brackish sites appear to contribute to soil salinization, presumably through continued, superior water uptake in brackish soils that leaves the salts behind (Sternberg et al., 2007; Wendelberger and Richards, 2017). This positive feedback makes the forest edge even more hostile to tree survival, potentially hastening tree death and making glycophytic tree regeneration even more unlikely (Jiang et al., 2012). Conversely, in areas with low salinity, marsh vegetation may protect the site from accumulating salt (Poulter et al., 2008). Thus, feedbacks from marsh vegetation on soil salinity will depend on the initial salinity of the site and, consequently, the type of marsh species that replace forest, either reinforcing the forest boundary by increasing salinity or by moderating salt accumulation.
Resistance of the site to soil salinization and reversibility of salt accumulation depend largely on the sources and dynamics of freshwater inputs to the site. Rainfall that precedes an overland incursion of saltwater (such as a high tide or storm surge) may protect the soil from saltwater infiltration by filling soil pores with fresh water (Gardner et al., 1992; Allen et al., 1998). Flushing of salts that do infiltrate soils is often difficult. However, there are some indications that freshwater inputs are sufficient to counteract salinization of some tidally-flooded sites. Patches of fresh-water marsh species surrounded by salt marsh and the occurrence of forest patches at unusually low elevations, together with isotopic studies suggest that some sites might be supplied with fresh ground water at high enough volume to flush salts and maintain non-halophytic vegetation longer than would be expected from a simple bathtub model of sea-level rise. Patterns of freshwater outflow, especially on karstic coastlines, may be difficult to predict (see Figure 3), and the salinity of water in the aquifer that feeds the coastal fringe is determined by both recent and long-past conditions (Delsman et al., 2014; Figure 3). These will modify the rate at which forest is eliminated and is replaced by salt marsh. Determining the extent to which forest stands at the marsh boundary are supplied by local, rain-fed freshwater lenses (Figure 3C) or by connections to more regional groundwater supplies (Figure 3D) requires site-specific studies (e.g., Williams et al., 2003, 2007; Saha et al., 2010, 2011).
Where forest stands are no longer regenerating due to increased flooding or salinity, forest canopy removal and subsequent replacement by marsh species is irreversible (at least for the foreseeable future). Hypersalinization associated with regional drought may cause notable pulses of tree mortality at the coast; however, where these have been studied, such events remove trees primarily in non-regenerating, already-doomed forest stands (DeSantis et al., 2007). Where forest can regenerate, seedlings may establish and, after wind damage, suppressed trees may be released to replace the damaged forest canopy (Doyle and Gorham, 1996; Rodgers et al., 2006). Where episodic events introduce salts that are not easily removed, such replacement may be irreversible. And where episodic events result in erosion of the soil surface, or disturb tree roots, rendering soil around them more susceptible to erosion (Williams et al., 2003), or kill trees that, in some geologic settings may contribute to building the soil surface above the marsh surface (Sullivan et al., 2014, 2016) the site may irreversibly lose forest and become dominated by marsh species.
In the progression of forest to marsh (Figure 2), the only potentially reversible step is the first: the loss of tree regeneration. Subsequent steps are inevitable with the passage of time and tree aging, although episodic events may hasten tree death and canopy removal. Tree regeneration, however, is episodic in many ecosystems. In coastal areas, pulsed events such as saltwater incursions, hypersalinization, or unusually high water may cause only a temporary pause in tree regeneration. Where local precipitation and topography allow flushing after saltwater incursions, or where local geologic features and regional hydraulic connections allow such flushing, seedling establishment may resume after salinity falls. For some tree species, such incursions cause death of the most recent cohorts of tree seedlings and reduction of salt allows germination of subsequent cohorts. For other species, seedlings may simply be top-killed during salt incursions, with seedlings surviving and resprouting after salinity is reduced (Williams et al., 1998). In the transition from forest to marsh, it is important to distinguish those factors that cause only temporary cessation of tree regeneration from those that cause long-term changes in salinity and flooding. Regional hydrology, geology, topography, development, and precipitation changes will all interact with sea-level rise to affect the pattern and rate of forest replacement by marsh at specific sites along coastlines.
After a forest dieback triggered by storms, sea-level rise may halt regeneration because seedlings can be more sensitive to environmental change and variability (Kozlowski, 1984; Donovan et al., 1988; Titus, 1990; Williams et al., 1999a). There is therefore a distinction between a regeneration niche (Grubb, 1977), in which seedlings are able to establish and a persistence niche (Bond and Midgley, 2001), in which mature plants that have already established maintain their position. Local variability in hydrology and geomorphology creates gradients in flooding and salt stress, which interact with the age-dependent stress response of trees to create demographic patterns across the landscape.
When these patterns are placed in the dynamic hydrologic setting of coastal landscapes, the transition between coastal forest and shrub or marsh ecosystems becomes an ecological ratchet (Jackson et al., 2009). Gradual sea-level rise pushes the lower boundary of regeneration further into the forest while extreme events move the lower boundary of the persistence zone up to the regeneration boundary (Figure 4).
The dynamics of the ratchet model are described in Figure 5. The hydrology of a site is characterized by an exceedance probability for water level. The water level can be measured in wells deployed in the field or modeled with a water balance approach (Bertassello et al., 2018). Pr{h>z} is the probability that the water level exceeds the elevation z in a given year. The inverse of this exceedance probability is the return time for a flood that inundates the forest up to z. The tolerance of young trees to flooding is modeled as a threshold exceedance probability above which they cannot develop. This threshold is a physiological limit to seedling growth modulated by a term representing local ecohydrological processes. Two stands with the same physiological limit and water level distribution may have different thresholds if, for example, fresh groundwater inputs enable seedlings to grow at a lower elevation at one of the sites.
Figure 5. Proposed conceptual model of ecological ratchet. (A) Landscape at time of forest establishment. The distribution on the left is the exceedance probability of water level. Mean sea level (MSL) is labeled (solid blue line). A threshold exceedance probability for regeneration is mapped onto a threshold elevation and horizontal position (solid green lines). Below this elevation, young trees cannot grow. (B) Landscape after sea level rise. The threshold probability remains constant, but the distribution of water levels changes with sea level (SLR; initial MSL labeled with dashed blue line) so that the regeneration boundary must move upslope (from the dashed green line to the solid green line). (C) An increase in the variance of the water level distribution can also lead to demographic zonation because, just as in the sea-level rise case, higher water levels become more likely. For the same threshold probability, the regeneration boundary moves upwards and inland.
Given the local water level distribution and the topography of a site, the threshold probability maps onto a regeneration boundary, which marks the lowest elevation at which young trees can grow. As sea level rises, the water level distribution moves upward. If neither the shape of the distribution nor the threshold exceedance probability for young trees changes, the regeneration boundary moves upslope at the same rate as sea level rises (Figure 5B). Trees that established at the initial regeneration boundary have matured and, because they are less sensitive to environmental stress, may persist below the new regeneration boundary, forming the persistence zone. The actual boundary between the forest and the marsh moves when these mature trees are killed by severe storms. The difference in elevation between the lowest mature trees and the present regeneration boundary is equal to the amount of sea-level rise since the establishment of those trees.
All the ecohydrological processes that structure the vegetation at a particular site are captured in a single threshold probability. As long as that probability remains constant at the site, this model predicts that the zone of persistence will span an elevation corresponding to the amount of sea-level rise since the establishment of the lowest mature trees, regardless of the actual value of the tolerance. The value of the tolerance does determine the elevation of the forest zones relative to sea level, so that forests with a higher threshold probability will lie closer to sea level than forests with a lower threshold probability.
An increase in water level variability, which accompanies an increase in storminess, for instance, can also drive migration of the regeneration boundary (Figure 5C). As the variance of the water level distribution increases, higher water levels become more likely, and the elevation at which the threshold exceedance probability is reached increases. If the variance of water levels increases after the low trees establish, then the regeneration boundary will move upwards, even in the absence of sea-level rise. The shape of the distribution of water levels can also change, because of variations in local climate and differences in hydrological characteristics of the soil when more forest is flooded.
A key assumption of this model is that the tolerance threshold of trees to flooding is constant in time. However, this tolerance could depend on the cumulative amount of stress experienced by the trees from flooding as well as from droughts, extreme temperatures, or disease. It could also depend on competitive or facilitative ecological interactions between trees or between trees and the grass and shrub species of the understory (Poulter et al., 2009). We therefore expect fluctuations around the persistence zone size that is estimated with a constant threshold exceedance probability. Sites which have become more stressed since the trees established would have a larger persistence zone size than estimated from the sea-level rise, while those which have become less stressed should have a smaller zone size. These fluctuations could be studied by comparing the deviation from the predicted persistence zone size to stand-level physiological metrics.
The migration of the regeneration boundary is ultimately driven by the slow press of sea-level rise, but the actual movement of the marsh-forest boundary happens in pulses when storms kill mature trees in the persistence zone. On long time scales, i.e., centuries, the migration of the marsh-forest boundary integrates over these pulses and is likely to resemble a deterministic migration upslope.
Our ecological ratchet model relates the position of the boundary to the water level, assuming an instantaneous adaptation of the boundary to changes in mean sea level. However, it will take some time for the ecosystem to adapt to new edaphic and light conditions. For example, it would take several years for grasses to fully grow in a forested area, and decades for a forest to recover after a storm. Following a disturbance, steady state can be achieved only after a relaxation time. A similar lag time was already found between changes in sea level and marsh elevation (Allen, 1990; Kirwan and Murray, 2008). This lag is a function of physical and biological processes and can span few hundreds of years (D'Alpaos et al., 2011).
A first-order approximation of the size of this persistence zone, obtained under the assumption of a constant threshold exceedance probability, is the amount of sea-level rise experienced since the forest establishment. This proxy gives an estimate for persistence zone size that is insensitive to the particular conditions of any given coastal forest and that should apply in a wide variety of settings. Another test of the hypothesized relationship between sea-level rise and forest persistence would be the reproduction of both the qualitative demographic zonation and the quantitative zone sizes in mechanistic models of coastal forests forced by storms and sea-level rise. The key physiological process to include in these models is the age-dependent response of trees to the stresses induced by sea-level rise without which there is no difference between the persistence and regeneration niche, which is required to produce an ecological ratchet. In the ecological ratchet model the upland slope plays a critical role on both rate and extent of marsh migration. In the next section we will focus on this important morphological driver.
Even at the simple level of a bathtub inundation model, it is obvious that slope has a strong influence on the amount of land potentially available for marsh migration. Given a SLR rate of 3 mm yr−1, a coastal plain with a slope of 0.0004—such as Florida's Big Bend (Raabe and Stumpf, 2015)—has 75 m of land available for marsh migration each decade. On the other extreme, “pocket marshes” in glaciated landscapes such as the Connecticut coast of Long Island Sound are sometimes surrounded by steep bedrock outcrops, with little land available for migration. In between those extremes, Chesapeake Bay marshes have upland slopes ranging from 0.003 to 0.1 (Schieder et al., 2017), corresponding to potential horizontal migration rates of 10 to 0.3 m per decade.
However, these calculations beg the central questions of marsh migration: Do marshes in fact quickly occupy the land that is theoretically made available to them by SLR? And how do factors such as slope and landuse affect their ability to do so? In order to address these questions, it is helpful to focus on rates of migration expressed vertically, rather than horizontally, in order to remove the first-order effects of slope and allow direct comparison to rates of SLR.
Three studies—from Chesapeake Bay (CB; Schieder et al., 2017), Delaware Bay (DB; Smith, 2013), and Florida (Raabe and Stumpf, 2015)—have used aerial photographs and/or historical maps to estimate changes in the marsh-forest boundary over time (note that marsh migration, as measured in these studies, is really about the absence of upland vegetation rather than the presence of marsh vegetation: an area in which marsh plants are growing under trees would be classified as upland—an inherent shortcoming of the aerial photo approach). We have converted these literature values of migration rates from horizontal to vertical units and plotted them as a function of slope (Figure 6). Both the CB and the DB studies show vertical migration rates (VMRs) that are mostly higher than local RSLR, while VMRs in the Florida study are about the same as RSLR. For the CB study, there is a significant positive effect of slope on VMR (R2 = 0.25, p = 0.001), while the DB study shows no effect (possibly because the range of slope values is more limited). The Florida study only reported average migration rates across the entire study area, so we could not analyze the effects of slope, but it is interesting that this low-slope area shows the lowest average VMR of the three studies. Complicating matters, of course, is that each of the studies covers a different time period (CB: 1846–1912 to 2013–2014; DB: 1930–2006; Florida: 1852–1886 to 1995).
Figure 6. Vertical migration rates of marshes as a function of local slope. Black dots are data collected in Chesapeake Bay (Schieder et al., 2017), triangles in Delaware Bay (Smith, 2013), and red crosses in Florida (Raabe and Stumpf, 2015). The dotted line is the linear interpolation of the Chesapeake Bay data.
Overall, however, Figure 1 suggests that in many cases, higher slopes lead to higher VMRs, which is consistent with the study by Hmieleski (1994) and the model of Brinson et al. (1995). Why would higher slopes lead to higher VMRs? Figure 7 outlines several potential effects of slope on VMR. First, higher slopes presumably lead to more rapid drainage (Hmieleski, 1994; Brinson et al., 1995), which might be expected to decrease the impacts of occasional saltwater inundation on soil water content and salinity, and thus retard migration, not facilitate it. In addition, upland plants living on steeper slopes should be better able to survive saltwater inundation, since the distance that roots must traverse to access upslope fresh water is decreased. On the other hand, marsh plants migrating over steeper slopes benefit from the shorter distance they must travel, a factor that might be especially important for plants that spread with rhizomes, such as Juncus gerardii and Distichlis spicata. Furthermore, saltwater pulses (e.g., from storm surges)—which are likely critical for initiating migration—may be dampened in low-slope areas by the expanse of marsh vegetation that they must traverse (Hmieleski, 1994). Based on Figure 6, we hypothesize that these latter factors—the shorter distance for both marsh plants and saltwater pulses to travel—are responsible for the higher-than-expected VMRs in high-slope areas.
Figure 7. Effects of slope on vertical migration rates of marshes. Pluses indicate a positive effect, while minuses indicate a negative effect.
Edaphic conditions might also prevent marsh expansion irrespectively of topographic slope. In coastlines with high freshwater outflows or extensive freshwater lenses the migration is retarded if not stopped (Figure 3). Therefore, the feedbacks between topography (e.g., slope) and groundwater dynamics needs to be characterized in detail at the local scale in order to quantify marsh transgression. So far we have focused on marsh migration in forests. In the following sections we examine the mechanisms of salt marsh migrations in urban landscapes and in agricultural fields.
Most studies of marsh migration have focused on migration into forested areas, with the exception of Wasson et al. (2013) and Anisfeld et al. (2016), which examined shrublands and mowed lawns, respectively. As indicated by the locations of these studies, non-forested uplands are particularly common in semi-arid (e.g., California) and urban (e.g., Long Island Sound) coastlines.
Urban uplands can be broadly classified into hardscapes and mowed lawns. In hardscapes such as parking lots, migration is strongly impeded, which points to the importance of mowed lawns as potential sites for marsh migration. In Connecticut, many of these lawns continue to be mowed even as marsh plants replace lawn grasses (Anisfeld et al., 2016). Migration into lawns is difficult-to-impossible to study using historical maps and aerial photographs, due to the challenge of distinguishing mowed lawn from mowed marsh.
Anisfeld et al. (2016) compared migration into lawns with migration into wooded areas at the same sites, and found no large differences in the extent of migration (expressed as vertical position of the marsh-upland ecotone). However, they found significant differences in the type of marsh vegetation that dominated the ecotone in the two landuses. In particular, migration into lawns was dominated by short-stature, mowing-tolerant plants such as Juncus gerardii and Spartina patens, while migration into wooded areas was dominated by tall and woody plants such as Phragmites australis and Iva frutescens; wooded areas also had more bare ground. This illustrates the important point that not all transitional marshes are identical in structure (and presumably function), a fact not captured by simple migration rates.
Little other data exist on how migration rates are affected by landcover. We suggest that four factors might be important to consider (Figure 8). First, marsh migration will clearly take longer if the upland plants are more salt tolerant. Tolerance of soil salinity and salt spray varies widely among both trees and grasses, and even for different varieties of the same species (Carrow and Duncan, 1998). For example, the forest being replaced by marsh in DB (Smith, 2013) includes both salt-sensitive species like Acer rubrum (threshold soil salinity <2dS/m; Wu and Dodge, 2005) and salt-tolerant ones such as Nyssa sylvatica (6–8 dS/m; Miyamoto et al., 2004); this may explain some of the variability in Figure 6. Likewise, commonly-used turfgrasses range from very salt-sensitive (e.g., Poa annua and Agrostis tenuis) to quite tolerant (e.g., some cultivars of Festuca rubra; Carrow and Duncan, 1998). At Hammonasset Beach State Park in Connecticut, marsh-adjacent lawns that have been disturbed by construction are replanted with a “New England Coastal Salt Tolerant Grass Mix” which includes 7 relatively salt-tolerant species (New England Wetland Plants, Inc., http://newp.com/data/2018/05/COASTAL-SALT-2018.pdf); this practice may retard marsh migration.
Figure 8. Factors affecting marsh migration in non-forested land. Pluses indicate a positive effect, while minuses indicate a negative effect.
Despite the lack of a systematic difference in salt tolerance between trees and grasses, there is likely a difference in turnover rate (Figure 8). Once turfgrasses are salt-stressed, they are likely to die and be replaced by marsh plants relatively quickly, while trees may take longer to die, and their dead trunks and roots are likely to persist longer on the landscape, potentially slowing marsh migration.
A third factor affecting migration rates in non-forested areas (Figure 8) is the mowing regime. As noted above, certain marsh grasses can survive mowing, so it is not clear that mowing impedes marsh migration per se, although ecosystem function is unlikely to be fully developed. Fourth, the near-100% ground cover that is typical of turfgrasses may present a greater barrier to marsh plants than a relatively open forest understory (though a thick litter layer may change this).
Thus, theoretical considerations (Figure 8) suggest different possible impacts of landuse on migration rates. Further data are needed to test these hypotheses, with the ultimate goal of both understanding and facilitating marsh migration across different landscapes. Marsh migration in farmlands is also common in rural areas, and will be examined in the next section.
Most studies of marsh migration into uplands have focused on forested land; fewer studies consider conversion of farmland to wetlands. While an obvious and direct impact of sea-level rise on coastal farms is conversion to subtidal mudflats, indirect factors associated with sea-level rise often are the primary cause of salt marsh transgression into farmland. Such factors include flooding due to sea-level rise enhanced storm-surge (Theiler and Hammar-Klose, 2000; Haigh et al., 2014; Kang et al., 2016), wetter conditions due to higher fresh groundwater levels (McCobb and Weiskel, 2003), and salinization of both soils (Clark et al., 2015) and groundwater (Masterson and Garabedian, 2007).
Efforts to predict farmland conversion to wetlands due to direct and indirect factors associated with sea-level rise have been largely regional and have focused on deltaic systems where the population density and the consequences of agricultural land loss are high (e.g., Katsman et al., 2011; Kang et al., 2016; Feng et al., 2018; Timsina et al., 2018). Conversion of cropland to salt marshes due to sea-level rise in low-lying coastal areas of the mid-Atlantic USA, are just beginning to receive attention (Gewin, 2018). The reasons for focus on the USA mid-Atlantic are similar to those for deltaic regions; that is, the low elevation and shallow landscape slope of these areas allows for landward movement of marshes that results in the conversion of upland, including agricultural land, to salt marsh (Kirwan et al., 2016). The low elevation and shallow slope, coupled with high rates of sea level rise, leads to significant change in farmland to marsh that may offset marsh loss on the seaward marsh edge (Kirwan et al., 2016). Where the slope of the upland is mild, rates of marsh migration will be rapid; where the upland is well-drained and the slope of the boundary between the upland and marsh is steep, there will be a topographic constraint on transgression and marsh migration will stall (Brinson et al., 1995). Additionally, the relatively high rate of sea-level rise in the mid-Atlantic contributes to rapid upland migration of marshes. Sea-level rise in the mid-Atlantic is twice the global average and exceeds that of the rest of the US coast with the exception of coastal Louisiana (Sallenger et al., 2012). Titus et al. (2010) estimate that 192 ha of farmland are converted to salt marsh annually in Accomack and Northampton Counties, Virginia.
Observations of farm-fields undergoing conversion to salt marsh in Northampton, VA, suggest a new pathway for marsh migration into uplands. Farms in this region are generally cropped in a winter wheat-corn-soybean rotation, although other crops frequently include potatoes, cotton, and vegetables (USDA, 2012; Figure 9). Soils of farmlands adjacent to salt marshes are well-drained sandy loams, with low organic matter content, moderate pH, and low cation exchange capacity. Hydrologic fluxes in farm fields are dominated by vertical exchanges of water from the soil to the atmosphere or to the groundwater; these exchanges include precipitation, evaporation and infiltration (Brinson et al., 1995).
Figure 9. Predicted inundation under one- and three-feet of sea-level rise in Dorchester and Somerset Counties, Maryland, USA relative to 2008 data. Red shading indicates inundated areas, from Epanchin-Niell et al. (2018).
As sea-level rises, the groundwater table rises, the thickness of the freshwater lens decreases, bringing saltwater closer to the land surface. A similar dynamics has been reported for Cape Cod, Massachusetts (USA), coastal uplands (Masterson, 2004; Masterson and Garabedian, 2007). On Cape Cod, the rise in water-table levels increased proportionally to rising sea level (McCobb and Weiskel, 2003); although the magnitude of the response was modified by proximity to non-tidal, groundwater-fed streams (Masterson and Garabedian, 2007). A similar response is likely in the mid-Atlantic region because the Cape Cod coastal aquifer system is similar to those along the entire US Atlantic coast (Barlow, 2003).
When the freshwater-saline water interface rises nearer to the land surface, the soils become waterlogged more frequently during rainstorms and take longer to drain, reducing the productivity of the farmland and leading to the eventual abandonment of the land. This scenario is supported by a survey of farmers attending the 2017 Virginia Eastern Shore Agriculture Conference. Over 70% of the survey respondents who farmed land adjacent to the estuary had abandoned portions of their fields due to prolonged periods of standing water after high rainfall events. Once the farmland is abandoned, and during times of low to moderate precipitation when the soil is not saturated, early successional-stage old-field plants colonize the land (Shifflett et al., 2013). When precipitation is of sufficient intensity or duration to saturate the soil for extended periods, the resulting low soil-redox conditions facilitate colonization by wetland plants and favor accumulation of organic matter in the soil (Figure 10).
Figure 10. Conceptual model of the changes in soil drainage and salinity leading to marsh migration into cropped farmland. Plant community composition observed during the stages of transition are shown for coastal Virginia.
While sea-level rise may result in rising groundwater levels and saltwater intrusion of the groundwater, ultimately it is soil salinization that leads to conversion of farmland to salt marshes. As sea-level continues to rise, the frequency of storm-surge flooding the abandoned land increases. The result is that the saline water infiltrates the soil, raising the salinity of both the soil and surficial groundwater, and decreasing the sharpness of the fresh-salt water interface. A further change is that seawater contains abundant sulfate, which in combination with low redox, favors the accumulation of sulfides (Ponnamperuma, 1972). The accumulation of sulfide and the osmotic effect of salt in the soils favors conversion from freshwater-wetland plants to high-salt-marsh plants (Koch and Mendelssohn, 1989) and the low redox conditions favors continued accumulation of soil organic matter (Figure 10).
Low land slope, rapid rates of sea-level rise, and increasing occurrences of coastal storms and droughts will accelerate marsh migration into farmfields. Further, predicting what soil salinity will be is a significant problem due to non-linear interactions among drivers (Clark et al., 2015) thereby compounding the difficulty of predicting how rapidly marsh will migrate into uplands.
What is clear is that marsh migration into farmland is driven by a combination of hydrological, climatological, and geomorphological processes that cause increasing soil saturation and salinization in response to sea-level rise. While the conversion of farmland to salt marsh may preserve and increase the ecosystem services provided by marshes (Craft et al., 2009), there is a cost to the local economy of productive land for farming operations. In the next paragraph we present how we can assess the economic impact of the conversion of upland areas into marshes.
In a period of accelerated sea level rise the survival of salt marshes relies on upland migration (Schuerch et al., 2018). Only conversion of upland areas to marshes can mitigate marsh loss due to rising sea levels. But marsh migration will likely be prevented by coastal communities in many areas, because it translates in loss of agricultural land, lawns, and infrastructure. Only a cost-benefit analysis showing the intrinsic value of wetlands will allow the transgression of marshes along developed shorelines.
A worldwide review of the ecosystem values and services of salt marshes is beyond the scope of this manuscript. Here we decided to focus our analysis on the US Mid-Atlantic region from Delaware to the Maryland-Virginia Eastern Shore, where the flat landscape and elevated rates of sea level rise lead to high rates of marsh migration and where information about agricultural loss is already available. Furthermore, we focus on an important economic aspect of marsh migration: the discount rate applied to salt marsh ecosystem services. Marsh transgression occurs at a timescale of decades, so there is a need to compare the future benefits provided by marshes with the present loss of upland areas triggered by sea level rise. The discount rate assesses future benefits at today's equivalent value and it is used in the USA to economically justify the conversion of land to salt marshes. If the discount rate is not assessed correctly, marshes will not be allowed to migrate upland.
Conversion of productive farmland to salt marshes can have a large economic impact in rural areas. Epanchin-Niell et al. (2018) show large losses of agricultural land by crop type in Maryland's Dorchester and Somerset counties (Figure 9). Gewin (2018) reported that of 11,534 ha of cropland in Somerset County, approximately 1,619 ha have been abandoned due to saturation of the soil in the last several decades. In the Virginia portion of the Eastern Shore, agriculture is the largest contributor to the economy and was valued at $153 million annually in 2014 (http://www.a-npdc.org/wp-content/uploads/2016/02/CEDS-2014-Update_19Dec2014.pdf). Annual monetary productivity of a single hectare of farmland is between $1600 and $2000 such that the annual conversion of 192 ha of farmland to marsh represents a loss to the local economy that increases by $ 307,200 to $ 384,000 every year.
Also the replacement of coastal forests with salt marshes in the eastern United States is a matter of economic concern. These forests host several pine species which are not only home to a large amount of flora and fauna but also prove useful for soil stabilization, controlling areas subject to severe erosion and gullying (Gaby, 1985; Baker and Langdon, 1990; Prestemon and Holmes, 2010). They also serve as an important timber source (Gaby, 1985; Baker and Langdon, 1990).
The loss of farmland or forest can be offset by the value of the ecosystem services provided by the new marsh. The value change can be assessed through traditional means, for example by assessing the real estate price of a piece of land or the selling price of agricultural commodities produced on the land. Such market-based assessments are relatively easy to conduct for uplands.
However, for marsh landscapes, market-based assessments often do not capture the true value of the benefits and services provided by the land to society. Their “ecosystem service values” must be assessed using techniques from the field of ecological economics (Costanza et al., 1997; Martínez et al., 2007).
Generally, marshes have low market value due to flooding risks, yet high ecosystem service value. The value of marshes lies in their provision of fish and bird habitat, sequestration of relatively large quantities of carbon, filtration of nutrients from water, and provision of some flood/storm protection value (Woodward and Wui, 2001). These services can be measured and then converted to monetary value (e.g., Tong et al., 2007).
As a marsh migrates into former uplands, private interests are replaced by public interests. Value shifts from the market economy to non-market ecosystem services. Uplands are typically privately-owned. Marshes in the US can be either publically-owned or privately-owned, yet their resources are heavily regulated according to the public interest through laws such as section 404 of the Clean Water Act or the Migratory Bird Act, or through subsidies such as the NRSC Wetlands Reserve Program (Gardner, 2011). Marsh migration exposes a wide range of economic conflicts between private and public interests (Feagin et al., 2010).
People tend to discount the value of having a given commodity or service in the future, relative to having it today. Imagine that you will pay $5 today for a fish extracted from the marsh today. How much less would you pay, if you had to wait 50 years to receive the fish? The term “discount rate” refers to the diminishing value of a product into the future, relative to today's monetary units. The “discount rate” is also the inverse of “interest rate” or “rate of accumulation,” or “rate of return on investment” minus inflation. Now imagine that you do not have the money to go fishing because you need a boat, but a local bank is willing to lend at a 3% annual interest rate. In this example, the lender is forgoing the opportunity to use that cash for themselves over the term of the loan, and instead giving it to you to buy the boat.
A commodity or service generally is worth more today than it is in the future (using today's monetary unit as the metric), and this relationship can be expressed as:
where Ct is the value at time t in the future and r is the discount rate charged at term n. While r can be set using an empirical value at a given time, for example from the Consumer Price Index (Bureau of Labor Statistics, 2018) or tax appraisal and commodity-pricing data, it is commonly set to 0.03 if no detailed information exists on a particular commodity or service (representing a generalized inflation rate of 3% in the US in modern times). The term n can be removed for simplification, under the assumption that n is equivalent to the minimum increment of t (for example, a time increment and term increment of 1 year in both cases, or annualized discounting).
In the case of a zero-sum tradeoff between two land cover types of equal area, one public and one private, we can define the breakeven condition at which their values must be the same as Ctpriv = Ctpub. We can insert Equation 1 into each part of this relation for private vs. public land respectively, annualize the rate, and obtain a time-dependent breakeven condition where t can vary for private or public land. However, we typically want to compare the value of private vs. public land at the same time t, allowing a proportional relationship to be made as:
This relationship shows that the relative value of private to public property at a future time is more strongly driven by the ratio of the two discount rates, rather than their starting C0 values, particularly as time moves forward; this fact is evidenced by the exponent above the discount rate ratio. For example, we graphed Equation 2 by first setting a public land cover discount rate at the typical r = 0.03, as one might assume is typical for a public value (e.g., fishery value). We then adjusted the private rate from −0.03 to 0.08 (ranging from a loss of 3% annually to a gain in 8% annually, in terms of the rate of return on private real estate investment).
For two lots of land, one a private upland and the second a public marsh—that are equal in value today, but are differentially discounted over time (6% for private, 3% for public)–the private land will be worth over twice as much within 25 years (Figure 11). Even for a difference of only 1% per year between the private vs. public rates, the upland is worth over 10% more than the marsh in only 10 years. Rate differentials such as these are quite common for portions of the US (e.g., Moszoro, 2016), and provide insight into why private interests are often protected at the expense of the public trust.
Figure 11. The difference in discount rates applied to the resources held in private uplands vs. public marshes quickly alters the relative value of the two across time. In this graphic, a Relative Return on Investment (R-ROI) value into private property (colored lines) vs. public at 3% rate, equivalent to mean inflation. The starting price is assumed to be equal for the two types of lands (prefactor = 1).
As local communities grapple with whether to protect uplands or to allow marshes to migrate by removing barriers such as seawalls, bulkheads, levees, concrete and managed lawns, the net economic-benefit is often sought (Figure 12). Coastal lands available for development are considered in short supply and high demand, driving up private interest rates. Conversely, the supply of public resources is often assumed to be consistently available in time or space, or provided by some “offsite” land, and so these resources are discounted. Political, legal, and social choices can often be reduced to an economic calculus of whether it is more valuable for our society to invest in private or public property.
Figure 12. In this example, the value of a privately-held upland and a publically-held marsh are both $1 at t = 0. After 5 years, the difference between the two discount rates results in a substantial net economic benefit for a barrier-laden landscape that will block the migration of the marsh.
As relative sea-level rise is a force that acts into future times, the key ratio that will govern the survival of marshes onto uplands is not the difference between their relative economic values today, but rather the difference between their relative discount rates. As the discount rate is compounded over time, it quickly overwhelms the effect of the original starting value of marshes vs. uplands. Through discounting, differentially inflating prices off into the future can strongly drive decision-making today.
It is important for conservation organizations and public entities to buy and set aside marginal uplands today, as they will one day lie at suitable elevations for marsh migration. If society waits to make the purchase until later in time, the private value of the uplands will have inflated faster than the rate of those public services that can be preserved. Thus, as society waits, the net benefit calculus will shift to further protect those private interests.
Our understanding of the dynamics of the marsh-upland boundary is still limited. The complexity of the hydrological, ecological and geomorphic processes involved requires an interdisciplinary approach that is nowadays absent. In particular, we lack long-term measurements on how the feedbacks between vegetation and hydrology drive the migration of this ecotone.
In the ratchet model presented herein storms and sea-level rise play different roles in marsh migration. Studies have pointed toward episodic disturbances from storms, and the associated storm surges, to cause permanent changes in the forest structure where sea-level rise had already deteriorated regeneration in the stands (Williams et al., 2003). Such processes set in motion by hurricanes in the face of rising seas can affect coastal forests from a stand to an ecosystem level, favoring the replacement of forests with salt marshes. Further research is needed to determine the extent of persistence zones in coastal forests and to identify the sources of variability in persistence zone size.
The effects of sea-level rise and storms on the marsh-upland boundary are rarely studied in conjunction. This is because these processes act at very different temporal scales: storm surges last only few hours while a rise in sea level can be perceived only after decades. A rational framework and experiments bridging these different timescales is needed.
Recent research has mostly focused on the replacement of coastal forests with salt marshes, but many mid-latitude shorelines border urban areas and agricultural fields. More research is needed to understand how marshes migrate in agricultural fields and suburban lawns.
Predictions of global, regional, and local marsh migration into uplands are limited by the availability in many locations of high resolution elevation maps and digital elevation models of coastal topography. There is also a need to forecasting extreme water levels due to tropical and extra-tropical storms (Haigh et al., 2014), and interactions of storm-surge with increased rivers discharge during flooding (Katsman et al., 2011). Finally, we also lack of understanding of the compounding effects of sea-level rise with coastal landforms (Woodruff et al., 2013), and the regional variation in rates of sea-level rise (Woodworth and Player, 2003).
In the next century survival of salt marshes will be insured by their migration in upland areas. Only if we recognize the economic value of salt marshes and their benefits to society we will allow them to replace forests, lawns, and agricultural fields. More research on the ecosystem services provided by salt marshes and their economic value is clearly needed. In particular it is critical to estimate not only the present value of salt marshes, but also how this value will increase in the future because of unforeseen ecosystem services that will benefit forthcoming generations.
All authors listed have made a substantial, direct and intellectual contribution to the work, and approved it for publication.
National Science Foundation USA awards DEB 1832221 and OCE 1637630.
The authors declare that the research was conducted in the absence of any commercial or financial relationships that could be construed as a potential conflict of interest.
Allen, J. A., Conner, W. H., Goyer, R. A., Chambers, J. L., and Krauss, K. W. (1998). Freshwater Forested Wetlands and Global Climate Change. Vulnerability of Coastal Wetlands in the Southeastern United States. A Biological Science Report USGS/BRD/BSR, Hawaii, HI, 33–44
Allen, J. R. L. (1990). Constraints on measurement of sea-level movements from salt-marsh accretion rates. J. Geol. Soc. Lond. 147, 5–7. doi: 10.1144/gsjgs.147.1.0005
Anisfeld, S. C., Cooper, K., R., and Kemp, A. C. (2016). Upslope development of a tidal marsh as a function of upland land use. Glob. Chang. Biol. 23, 755–766. doi: 10.1111/gcb.13398
Barlow, P. M. (2003). “Ground water in fresh water-salt water environments of the atlantic,” in Geological Survey, Vol. 1262 (Denver, CO: USGS).
Barry, P. J., Doggett, C., Anderson, R. L., and Swain, K. M. (1993). “How to evaluate and manage storm-damaged forest areas,” in USDA Forest Service Southern Region Management Bulletin RS-MB 63 (Atlanta, GA).
Bertassello, L. E., Rao, P. S. C., Park, J., and Jawitz, J. W., and Botter, G. (2018). Stochastic modeling of wetland-groundwater systems 2018. Adv. Wat. Res. 112, 214–223. doi: 10.1016/j.advwatres.2017.12.007
Blake, E. S., and Zelinsky, D. A. (2018). Hurricane Harvey (Tropical Cyclone Report No. AL092017). National Hurricane Center.
Blood, E., Anderson, P., Smith, P., Nybro, C., and Ginsberg, K. (1991). Effects of Hurricane Hugo on coastal soil solution chemistry in South Carolina. Biotropica 23, 348–355. doi: 10.2307/2388251
Bond, W. J., and Midgley, J. J. (2001). Ecology of sprouting in woody plants: the persistence niche. Trends Ecol. Evol. 16, 45–51. doi: 10.1016/S0169-5347(00)02033-4
Brinson, M. M., Christian, R., R., and Blum, L. K. (1995). Multiple states in the sea-level induced transition from terrestrial forest to estuary. Estuaries 18, 648–659. doi: 10.2307/1352383
Bureau of Labor Statistics (2018). Bureau of Labor Statistics. Available online at: https://www.bls.gov/cpi
Cangialosi, J. P., Latto, A. S., and Berg, R. (2018). Hurricane Irma (Tropical Cyclone Report No. AL112017). National Hurricane Center.
Carrow, R. N., and Duncan, R. R. (1998). Salt-Affected Turfgrass Sites: Assessment and Management. Chelsea, MI: Ann Arbor Press.
Chapman, E. L., Chambers, J. Q., Ribbeck, K. F., Baker, D. B., Tobler, M. A., Zeng, H., et al. (2008). Hurricane Katrina impacts on forest trees of Louisiana's Pearl River basin. For. Ecol. Manage., 256, 883–889. doi: 10.1016/j.foreco.2008.05.057
Clark, D., Williams, S., Jahiruddin, M., Parks, K., and Salehin, M. (2015). Projection of on-farm salinity in coastal Bangladesh. Environ. Sci. Process. Impacts 17, 1127–1136. doi: 10.1039/C4EM00682H
Clark, J. S. (1986). Coastal forest tree populations in a changing environment, southeastern Long Island, New York. Ecol. Monogr. 56, 259–277. doi: 10.2307/2937077
Conner, W. H., and Day, J. W. (1988). Rising water levels in coastal Louisiana: implications for two coastal forested wetland areas in Louisiana. J. Coast. Res. 4, 589–596.
Costanza, R., d'Arge, R., de Groot, R., Farber, S., Grasso, M., Hannon, B., et al. (1997). The value of the world's ecosystem services and natural capital. Nature 387, 253–260.
Craft, C., Clough, J., Ehman, J., Joye, S., Park, R., Pennings, S., et al. (2009). Forecasting the effects of accelerated sea-level rise on tidal marsh ecosystem services. Front. Ecol. Environ. 7:219. doi: 10.1890/070219
D'Alpaos, A., Mudd, S., M., and Carniello, L. (2011), Dynamic response of marshes to perturbations in suspended sediment concentrations rates of relative sea level rise. J. Geophys. Res. 116:F04020. doi: 10.1029/2011JF002093
DeBell, D., Hook, D., McKee, W., and Askew, J. (1984). Growth and physiology of loblolly pine roots under various water table level and phosphorus treatments. Forest Sci. 30, 705–714.
Delsman, J. R., Hu-A-Ng, K. R. M., Vos, P. C., De Louw, P. G., Oude Essink, G. H., Stuyfzand, P. J., et al. (2014). Paleo-modeling of coastal saltwater intrusion during the Holocene: an application to the Netherlands. Hydrol. Ear. Syst. Sci. 18, 3891–3905. doi: 10.5194/hess-18-3891-2014
DeSantis, L. R. G., Bhotika, S., Williams, K., and Putz, F. E. (2007). Sea-level rise and drought interactions accelerate forest decline on the Gulf Coast of Florida, U. S. A. Glob. Chang. Biol. 13, 2349–2360. doi: 10.1111/j.1365-2486.2007.01440.x
Donovan, L. A., McLeod, K. W., Sherrod, K. C., and Stumpff, N. J. (1988). Response of woody swamp seedlings to flooding and increased water temperatures. I. growth, biomass, and survivorship. Am. J. Bot. 75, 1181–1190. doi: 10.1002/j.1537-2197.1988.tb08831.x
Doyle, T. W., and Gorham, L. E. (1996). “Detecting hurricane impact and recovery from tree rings,” in Tree Rings, Environment, and Humanity, eds J. S. Dean, D. M. Meko, and T. W. Swetnam (Tucson, AZ: Radiocarbon, Department of Geosciences, University of Arizona), 405–412.
Doyle, T. W., Krauss, K. W., Conner, W. H., and From, A. S. (2010). Predicting the retreat and migration of tidal forests along the northern Gulf of Mexico under sea-level rise. For. Ecol. Manage. 259, 770–777. doi: 10.1016/j.foreco.2009.10.023
Drouineau, S., Laroussinie, O., Birot, Y., Terrasson, D., Formery, T., and Roman-Amat, B. (2000). Joint Evaluation of Storms, Forest Vulnerability and Their restoration. European Forest Institute Joensuu. Joensuu: European Forest Institute (EFI).
Duryea, M. L., Kampf, E., and Littell, R. C. (2007). Hurricanes and the urban forest: I. Effects on southeastern United States coastal plain tree species. Arboric. Urban Forest. 33, 83–97.
Epanchin-Niell, R., Gedan, K., Miller, J., and Tully, K. (2018). Saltwater Intrusion and Coastal Climate Adaptation: Building Community Resilience. Available online at: http://www.rff.org/research/publications/saltwater-intrusion-and-coastal-climate-adaptation-building-community (Accessed December7, 2018).
Everham, E. M., and Brokaw, N. V. (1996). Forest damage and recovery from catastrophic wind. Botan. Rev. 62, 113–185. doi: 10.1007/BF02857920
Feagin, R. A., Martinez, M. L., Mendoza-Gonzalez, G., and Costanza, R. (2010). Salt marsh zonal migration and ecosystem service change in response to global sea level rise: a case study from an urban region. Ecol. Soc. 15:14.
Feng, A., Gao, J., Wu, S., Liu, L., Li, Y., and Yue, X. (2018). Assessing the inundation risk resulting from extreme water levels under sea-level rise: a case study of Rongcheng, China. Geomat. Nat. Hazards Risk 9, 456–470. doi: 10.1080/19475705.2018.1447026
Fernandes, A., Rollinson, C. R., Kearney, W. S., Dietze, M. C., and Fagherazzi, S. (2018). Declining radial growth response of coastal forests to hurricanes and nor'easters. J. Geophys. Res. 123, 832–849. doi: 10.1002/2017JG004125
Field, C. R., Gjerdrum, C., and Elphick, C. S. (2016). Forest resistance to sea-level rise prevents landward migration of tidal marsh. Biol. Conserv. 201, 363–369. doi: 10.1016/j.biocon.2016.07.035
Gaby, L. I. (1985). The Southern Pines, an American Wood. Washington, DC: USDA Forest Service, FS-256.
Gardner, L., Michener, W., Blood, E., Williams, T., Lipscomb, D., and Jefferson, W. (1991). Ecological impact of Hurricane Hugo - salinization of a coastal forest. J. Coast. Res. 301–317.
Gardner, L. R., Michener, W. K., Williams, T. M., Blood, E. R., Kjerve, B., Smock, L. A., et al. (1992). Disturbance effects of Hurricane Hugo on a pristine coastal landscape: North Inlet, South Carolina, U. S. A. Netherl. J. Sea Res. 30, 249–263. doi: 10.1016/0077-7579(92)90063-K
Gardner, R. C. (2011). Lawyers, Swamps, and Money: U.S. Wetland Law, Policy, and Politics. Washington, DC: Island Press. doi: 10.5822/978-1-61091-025-5
Gewin, V. (2018). The Slow-Motion Catastrophe Threatening 350-Year-Old Farms. The Atlantic. Available online at: https://www.theatlantic.com/science/archive/2018/03/maryland-salt-farms/554663/ (Accessed June 30, 2018).
Gresham, C. A., Williams, T. M., and Lipscomb, D. J. (1991). Hurricane Hugo wind damage to southeastern US coastal forest tree species. Biotropica 23, 420–426. doi: 10.2307/2388261
Grubb, P. J. (1977). The maintenance of species-richness in plant communities: the importance of the regeneration niche. Biol. Rev. 52, 107–145. doi: 10.1111/j.1469-185X.1977.tb01347.x
Guneralp, B., Guneralp, I., and Liu, Y. (2015). Changing global patterns of urban expsorure to flood and drought hazards. Glob. Environ. Change 31, 217–225. doi: 10.1016/j.gloenvcha.2015.01.002
Haigh, I., Wijeratne, E. M. S., MacPherson, L., Pattiaratchi, C., Mason, M., Crompton, R., and George, S. (2014). Estimating present day extreme water level exceedance probabilities around the coastline of Australia: tides, extra-tropical storm surges and mean sea level. Clim. Dynam. 42, 121–138. doi: 10.1007/s00382-012-1652-1
Hmieleski, J. I. (1994). High Marsh-Forest Transitions in a Brackish Marsh: The Effects of Slope. M.S. thesis, East Carolina University. Available online at: https://www.vcrlter.virginia.edu/thesis/Hmieleski94/Hmieleski.html
Hu, T., and Smith, R. B. (2018). The impact of Hurricane Maria on the vegetation of Dominica and Puerto Rico using multispectral remote sensing. Remote Sens. 10:827. doi: 10.3390/rs10060827
Jackson, S. T., Betancourt, J. L., Booth, R. K., and Gray, S. T. (2009). Ecology and the ratchet of events: Climate variability, niche dimensions, and species distributions. Proc. Natl. Acad. Sci. U.S.A. 106, 19685–19692. doi: 10.1073/pnas.0901644106
Jadhav, R. S., Chen, Q., and Smith, J. M. (2013). Spectral distribution of wave energy dissipation by salt marsh vegetation. Coast. Eng. 77, 99–107. doi: 10.1016/j.coastaleng.2013.02.013
Jiang, J., DeAngelis, D. L., Smith, T. J., Teh, S. Y., and Koh, H. L. (2012). Spatial pattern formation of coastal vegetation in response to external gradients and positive feedbacks affecting soil porewater salinity: a model study. Landsc. Ecol. 27, 109–119. doi: 10.1007/s10980-011-9689-9
Kang, L., Ma, L., and Liu, Y. (2016). Evaluation of farmland losses from sea level rise and storm surges in the Pearl River Delta region under global climate change. J. Geograp. Sci. 26, 439–456. doi: 10.1007/s11442-016-1278-z
Katsman, C. A., Sterl, A., Beersma, J. J., van den Brink, H. W., Chruch, J. A., Hazeleger, W., et al. (2011). Exploring high-end scenarios for local sea level rise to develop flood protection strategies for low-lying delta – the Netherlands as an example. Clim. Change 109, 617–645. doi: 10.1007/s10584-011-0037-5
Kirwan, M. L., Guntenspergen, G. R., d'Alpaos, A., Morris, J. T., Mudd, S. M., and Temmerman, S. (2010). Limits on the adaptability of coastal marshes to rising sea level. Geophys. Res. Lett. 37:L23401. doi: 10.1029/2010GL045489
Kirwan, M. L., and Murray, A. B. (2008), Tidal marshes as disequilibrium landscapes? Lags between morphology and Holocene sea level change. Geophys. Res. Lett. 35:L24401. doi: 10.1029/2008GL036050
Kirwan, M. L., Walters, D. C., Reay, W. G., and Carr, J. A. (2016). Sea level driven marsh expansion in a coupled model of marsh erosion and migration. Geophys. Res. Lett. 43, 4366–4373. doi: 10.1002/2016GL068507
Knutson, P. L., Brochu, R. A., Seelig, W. N., and Inskeep, M. (1982). Wave damping inspartina alterniflora marshes. Wetlands 2, 87–104.
Koch, M. S., and Mendelssohn, I. A. (1989). Sulphide as a soil phytotoxin: differential responses in two marsh species. J. Ecol. 77, 565–578.
Kozlowski, T. (1997). Responses of woody plants to flooding and salinity. Tree Physiol. Monogr. 17:490. doi: 10.1093/treephys/17.7.490
Kurz, H., and Wagner, K. A. (1957). Tidal Marshes of the Gulf and Atlantic Coasts of Northern Florida and Charleston, South Carolina. Florida State University Studies Number 24. Tallahassee, FL: The Florida State University.
Langston, A. K., Kaplan, D. A., and Putz, F. E. (2017). A casualty of climate change? Loss of freshwater forest islands on Florida's Gulf Coast. Glob. Chang. Biol. 23, 5383–5397. doi: 10.1111/gcb.13805
Lentz, E. E., Thieler, E. R., Plant, N. G., Stippa, S. R., Horton, R. M., and Gesch, D. B. (2016). Evaluation of dynamic coastal response to sea-level rise modifies inundation likelihood. Nat. Clim. Chang. 6:696–700. doi: 10.1038/NCLIMATE2957
Leonardi, N., Defne, Z., Ganju, N. K., and Fagherazzi, S. (2016). Salt marsh erosion rates and boundary features in a shallow Bay. J. Geophys. Res. 121, 1861–1875. doi: 10.1002/2016JF003975
Little, C. M., Horton, R. M., Kopp, R. E., Oppenheimer, M., Vecchi, G. A., and Villarini, G. (2015). Joint projections of US East Coast sea level and storm surge. Nat. Clim. Chang. 5, 1114–1120. doi: 10.1038/nclimate2801
Loope, L., Duever, M., Herndon, A., Snyder, J., and Jansen, D. (1994). Hurricane impact on uplands and freshwater swamp forest. BioScience 44, 238–246.
Lugo, A. E. (2008). Visible and invisible effects of hurricanes on forest ecosystems: an international review. Austral Ecol. 33, 368–398. doi: 10.1111/j.1442-9993.2008.01894.x
Marani, M., d'Alpaos, A., Lanzoni, S., and Santalucia, M. (2011). Understanding and predicting wave erosion of marsh edges. Geophys. Res. Lett. 38:L21401. doi: 10.1029/2011GL048995
Mariotti, G., Fagherazzi, S., Wiberg, P. L., McGlathery, K. J., Carniello, L., and Defina, A. (2010). Influence of storm surges and sea level on shallow tidal basin erosive processes. J. Geophys. Res. 115:C11012. doi: 10.1029/2009JC005892
Martínez, M. L., Intralawan, A., Vázquez, G., Pérez-Maqueo, O. M., Sutton, P., and Landgrave, R. (2007). The coasts of our world: ecological, economic and social importance. Ecol. Econom. 63, 254–272. doi: 10.1016/j.ecolecon.2006.10.022
Masterson, J. P. (2004). Simulated Interaction Between Freshwater and Saltwater and Effects of Ground-Water Pumping and Sea-Level Change, Lower Cape Cod Aquifer System, Massachusetts. Denver, CO: US Dept. of the Interior, US Geological Survey. Report 2004-5014.
Masterson, J. P., and Garabedian, S. P. (2007). Effects of sea-level rise on ground water flow in a coastal aquifer system. Ground Water 45, 209–217. doi: 10.1111/j.1745-6584.2006.00279.x
McCobb, T. M., and Weiskel, P. K. (2003). Long-Term Hydrologic Monitoring Protocol for Coastal Ecosystems. USGS Open-File Report 02-497. Reston, Virginia:USGS.
Merry, K., Bettinger, P., and Hepinstall, J. (2009). Physical and biological responses of forests to tropical cyclones affecting the United States Atlantic Ocean and Gulf of Mexico coasts. Am. J. Environ. Sci. 5, 16–32. doi: 10.3844/ajessp.2009.16.32
Middleton, B. A. (2016). Differences in impacts of Hurricane Sandy on freshwater swamps on the Delmarva Peninsula, Mid-Atlantic Coast, USA. Ecol. Eng. 87, 62–70. doi: 10.1016/j.ecoleng.2015.11.035
Miyamoto, S., Martinez, I., Padilla, M., Portillo, A., and Ornelas, D. (2004). Photo Guide: Landscape Plant Response to Salinity. El Paso, TX: Texas Agricultural Experiment Station.
Möller, I., Kudella, M., Rupprecht, F., Spencer, T., Paul, M., Van Wesenbeeck, B. K., et al. (2014). Wave attenuation over coastal salt marshes under storm surge conditions. Nat. Geosci. 7, 727–737. doi: 10.1038/ngeo2251
Moszoro, M. W. (2016). Public Versus Private Cost of Capital With State-Contingent Terminal Value. Amsterdam: SSRN. doi: 10.2139/ssrn.2674668
Nicholls, R. J., and Leatherman (1995). “Sea-level rise and coastal management,” in Geomorphology and Land Management in a Changing Environment, eds D. F. M. McGregor and D. A. Thompson (Chichester: John Wiley & Sons LTD), 229–244.
Pasch, R. J., Penny, A. B., and Berg, R. (2018). Hurricane Maria (Tropical Cyclone Report No. AL152017). National Hurricane Center.
Penfound, W. T., and Hathaway, E. S. (1938). Plant communities in the marshlands of southeastern Louisiana. Ecol. Monogr. 8, 1–56. doi: 10.2307/1943020
Pezeshki, S., Delaune, R., and Patrick, W. (1990). Flooding and saltwater intrusion: potential effects on survival and productivity of wetland forests along the U.S. Gulf Coast. For. Ecol. Manage. 33, 287–301. doi: 10.1016/0378-1127(90)90199-L
Phillips, J. D. (1999). Event timing and sequence in coastal shoreline erosion: hurricanes bertha and fran and the neuse estuary. J. Coast. Res. 15, 616–623.
Pimm, S. L., Davis, G. E., Loope, L., Roman, C. T., Smith, T. J., and Tilmant, J. T. (1994). Hurricane andrew. Bioscience 44, 224–229. doi: 10.2307/1312226
Ponnamperuma, F. N. (1972). The chemistry of submerged soils. Adv. Agron. 24, 29–96. doi: 10.1016/S0065-2113(08)60633-1
Ponnamperuma, F. N. (1984). “Effects of flooding on soils,” in Flooding and Plant Growth, ed T. Kozlowski (Orlando, FL: Academic press), 9–45. doi: 10.1016/B978-0-12-424120-6.50007-9
Poulter, B., Christensen, N. L., and Song, Q. S. (2008). Tolerance of Pinus taeda and Pinus serotina to low salinity and flooding: implications for equilibrium vegetation dynamics. J. Veget. Sci. 19, 15–122. doi: 10.3170/2007-8-18410
Poulter, B., Qian, S. S., and Christensen, N. L. (2009). Determinants of coastal treeline and the role of abiotic and biotic interactions. Plant Ecol. 202, 55–66. doi: 10.1007/s11258-008-9465-3
Prestemon, J. P., and Holmes, T. P. (2010). “Economic impacts of hurricanes on forest owners,” in Advances in Threat Assessment and Their Application to Forest and Rangeland Management (Portland, OR: US Department of Agriculture, Forest Service, Pacific Northwest and Southern Research Stations), 207–221.
Puijalon, S., Bouma, T. J., Douady, C. J., van Groenendael, J., Anten, N. P., Martel, E., et al. (2011). Plant resistance to mechanical stress: evidence of an avoidance–tolerance trade-off. New Phytol. 191, 1141–1149. doi: 10.1111/j.1469-8137.2011.03763.x
Raabe, E., and Stumpf, R. (2015). Expansion of tidal marsh in response to sea-level rise: Gulf Coast of Florida, U. S. A. Estuar. Coast. 38:145. doi: 10.1007/s12237-015-9974-y
Rodgers, I. C., Gamble, D. W., McCay, D. H., and Phipps, S. (2006). Tropical cyclone signals within tree-ring chronologies from Weeks Bay National Estuary and Research Reserve, Alabama. J. Coast. Res. 22, 1320–1329. doi: 10.2112/05-0515.1
Rodríguez, J. F., Saco, P. M., Sandi, S., Saintilan, N., and Riccardi, G. (2017). Potential increase in coastal wetland vulnerability to sea-level rise suggested by considering hydrodynamic attenuation effects. Nat. Commun. 8:16094. doi: 10.1038/ncomms16094
Saha, A. K., Saha, S., Sadle, J., Jiang, J., Ross, M., S., et al. (2011). Sea level rise and South Florida coastal forests. Clim. Change 107, 81–108. doi: 10.1007/s10584-011-0082-0
Saha, A. K., Sternberg Lda, SO., Ross, M. S., and Miralles-Wilhelm, F. (2010). Water source utilization and foliar nutrient status differs between upland and flooded plant communities in wetland tree islands. Wetlands Ecol. Manage. 18, 343–355. doi: 10.1007/s11273-010-9175-1
Sallenger, A. H. Jr., Doran, K. S., and Howard, P., A. (2012). Hotspot of accelerated sea-level rise on the Atlantic coast of North America. Nat. Clim. Chang. 2, 884–888. doi: 10.1038/nclimate1597
Schaetzl, R. J., Johnson, D. L., Burns, S. F., and Small, T. W. (1989). Tree uprooting: review of terminology, process, and environmental implications. Can. J. For. Res. 19, 1–11. doi: 10.1139/x89-001
Schieder, N. W., Walters, D., C., and Kirwan, M., L., (2017). Massive upland to wetland conversion compensated for historical marsh loss in chesapeake bay, U. S. A. Estuar. Coast. 47, 940–951. doi: 10.1007/s12237-017-0336-9
Schuerch, M., Spencer, T., Temmerman, S., Kirwan, M. L., Wolff, C., Lincke, D., et al. (2018). Future response of global coastal wetlands to sea-level rise. Nature 561, 231–234. doi: 10.1038/s41586-018-0476-5
Shifflett, S. A., Zinnert, J. C., and Young, D. R. (2013). Changes in plant community composition and structure following restoration of maritime communities. J. Torrey Botanical Soc. 140, 89–100 doi: 10.3159/TORREY-D-12-00008.1
Shoulders, E., and Ralston, C. W. (1975). Temperature, root aeration, and light influence Slash pine nutrient uptake rates. For. Sci. 21, 401–410.
Silvestri, S., D'Alpaos, A., Nordio, G., and Carniello, L. (2018). Anthropogenic modifications can significantly influence the local mean sea level and affect the survival of salt marshes in shallow tidal systems. J. Geophys. Res. 123, 996–1012. doi: 10.1029/2017JF004503
Smith, J. A. M. (2013). The role of Phragmites australis in mediating inland salt marsh migration in a Mid-Atlantic Estuary. PLoS ONE 8:e65091. doi: 10.1371/journal.pone.0065091
Smith, J. M., Cialone, M. A., Wamsley, T. V., and McAlpin, T. O. (2010). Potential impact of sea level rise on coastal surges in southeast Louisiana. Ocean Eng. 37, 37–47. doi: 10.1016/j.oceaneng.2009.07.008
Stanturf, J. A., Goodrick, S. L., and Outcalt, K. W. (2007). Disturbance and coastal forests: a strategic approach to forest management in hurricane impact zones. For. Ecol. Manage. 250, 119–135. doi: 10.1016/j.foreco.2007.03.015
Sternberg, L. S. L., Teh, S. Y., Ewe, S. M., Miralles-Wilhelm, F., and DeAngelis, D. L. (2007). Competition between hardwood hammocks and mangroves. Ecosystems 10, 648–660. doi: 10.1007/s10021-007-9050-y
Stoffel, M., Bollschweiler, M., Butler, D. R., and Luckman, B. H. (2010). Tree Rings and Natural Hazards: A State-of-the-Art, Vol. 41 of Advances in Global Change Research. Heidelberg: Springer Science & Business Media. doi: 10.1007/978-90-481-8736-2_1
Sullivan, P. L., Engel, V., Ross, M. S., and Price, R. M. (2014). The influence of vegetation on the hydrodynamics and geomorphology of a tree island in Everglades National Park (Florida, United States). Ecohydrology 7, 727–744. doi: 10.1002/eco.1394
Sullivan, P. L., Price, R. M., Ross, M. S., Stoffella, S. L., Sah, J. P., Scinto, L., et al. (2016). Trees: a powerful geomorphic agent governing the landscape evolution of a subtropical wetland. Biogeochemistry 128, 369–384. doi: 10.1007/s10533-016-0213-9
Theiler, E. R., and Hammar-Klose, E. S. (2000). National Assessment of Coastal Vulnerability to Sea-Level Rise: Preliminary Results for the U.S. Atlantic Coast. USGS Open-File Report 99-593. Reston, Virginia: USGS.
Timsina, J., Wolf, J., Guilpart, N., van Bussel, L. G. J., Grassini, P., van Wart, J., et al. (2018). Can Bangladesh produce enough cereals to meet future demand? Agric. Syst. 163, 36–44. doi: 10.1016/j.agsy.2016.11.003
Titus, J. G., Hudgens, D. E., Hershner, C., Kassakian, J. M., Penumalli, P. R., Berman, M., et al. (2010). “Virginia,” in The Likelihood of Shore Protection Along the Atlantic Coast of the United States, Vol. 1: Mid-Atlantic, eds G. James Titus and D. Hudgens (Washington, DC: Report to the U.S. Environmental Protection Agency), 697–888.
Titus, J. H. (1990). Microtopography and woody plant regeneration in a hardwood floodplain swamp in Florida. Bull. Torrey Botan. Club, 117, 429–437. doi: 10.2307/2996840
Tong, C., Feagin, R. A., Lu, J., Zhang, X., Zhu, X., Wang, W., et al. (2007). Ecosystem service values and restoration in the urban Sanyang Wetland of Wenzhou, China. Ecol. Eng. 29, 249–258. doi: 10.1016/j.ecoleng.2006.03.002
USDA (2012). Census of Agriculture. United States Department of Agriculture. Available online at: https://www.agcensus.usda.gov/Publications/2012/Full_Report/Volume_1\protect\kern+.1667em\relax_Chapter_2_County_Level/ accessed August 20, (2018).
Wang, F., and Xu, Y. J. (2009). Hurricane Katrina-induced forest damage in relation to ecological factors at landscape scale. Environ. Monit. Assess. 156, 491–507. doi: 10.1007/s10661-008-0500-6
Wasson, K., Woolfolk, A., and Fresquez, C. (2013). Ecotones as indicators of changing environmental conditions: rapid migration of salt marsh-upland boundaries. Estuar. Coast. 36, 654–664. doi: 10.1007/s12237-013-9601-8
Webster, P. J., Holland, G. J., Curry, J. A., and Chang, H. R. (2005). Changes in tropical cyclone number, duration, and intensity in a warming environment. Science 309, 1844–1846. doi: 10.1126/science.1116448
Wells, B. W., and Shunk, I. V. (1938). Salt spray: an important factor in coastal ecology. Bull. Torrey Botan. Club 65, 485–492. doi: 10.2307/2480897
Wendelberger, K. S., and Richards, J. H. (2017). Halophytes can salinize soil when competing with glycophytes, intensifying effects of sea level rise in coastal communities. Oecologia 184, 729–737. doi: 10.1007/s00442-017-3896-2
Whelan, K. R., Smith, T. J., Anderson, G. H., and Ouellette, M. L. (2009). Hurricane Wilma's impact on overall soil elevation and zones within the soil profile in a mangrove forest. Wetlands 29:16.
Williams, H., and Flanagan, W. (2009). Contribution of Hurricane Rita storm surge deposition to long-term sedimentation in Louisiana coastal woodlands and marshes. J. Coast. Res. 2, 1671–1675.
Williams, K., Ewel, K. C., Stumpf, R. P., Putz, F. E., and Workman, T. W. (1999b). Sea-level rise and coastal forest retreat on the west coast of Florida, U. S. A. Ecology 80, 2045–2063.
Williams, K., MacDonald, M., and Lda Sternberg, S. L. (2003). Interactions of storm, drought, and sea-level rise on coastal forest: a case study. J. Coast. Res. 19, 1116–1121.
Williams, K., MacDonald, M., McPherson, K., and Mirti, T. H. (2007). “Chapter 10: Ecology of the coastal edge of hydric hammocks on the Gulf Coast of Florida,” in Ecology of Tidal Freshwater Swamps of the Southeastern United States, eds W. H. Conner, T. W. Doyle, and K. W. Krauss (Dordrecht: Springer Press), 255–289. doi: 10.1007/978-1-4020-5095-4_10
Williams, K., Meads, M. V., and Sauerbrey, D. A. (1998). The roles of seedling salt-tolerance and resprouting in forest zonation on the west coast of Florida, U. S. A. Am. J. Bot. 85, 1745–1752. doi: 10.2307/2446509
Williams, K., Pinzon, Z., Stumpf, R. P., and Raabe, E. A. (1999a). Sea-Level Rise and Coastal Forests on the Gulf of Mexico. U.S. Geological Survey Open File Report 99-441. p. 121. doi: 10.3133/ofr99441
Woodruff, J. D., Irish, J. L., and Camargo, S. J. (2013). Coastal flooding by tropical cyclones and sea-level rise. Nature 504, 44–52. doi: 10.1038/nature12855
Woodward, R. T., and Wui, Y.-S. (2001). The economic value of wetland services: a meta-analysis. Ecol. Econom. 37, 257–270. doi: 10.1016/S0921-8009(00)00276-7
Woodworth, P., and Player, R. (2003). The permanent service for mean sea level: an update to the 21st century. J. Coast. Res. 19, 287–295.
Keywords: marsh upland boundary, sea level rise, effect of storms on forests, salt marsh, soil salinity, marsh transgression, marsh boundary
Citation: Fagherazzi S, Anisfeld SC, Blum LK, Long EV, Feagin RA, Fernandes A, Kearney WS and Williams K (2019) Sea Level Rise and the Dynamics of the Marsh-Upland Boundary. Front. Environ. Sci. 7:25. doi: 10.3389/fenvs.2019.00025
Received: 17 September 2018; Accepted: 07 February 2019;
Published: 27 February 2019.
Edited by:
Carlo Camporeale, Politecnico di Torino, ItalyReviewed by:
Andrea D'Alpaos, University of Padova, ItalyCopyright © 2019 Fagherazzi, Anisfeld, Blum, Long, Feagin, Fernandes, Kearney and Williams. This is an open-access article distributed under the terms of the Creative Commons Attribution License (CC BY). The use, distribution or reproduction in other forums is permitted, provided the original author(s) and the copyright owner(s) are credited and that the original publication in this journal is cited, in accordance with accepted academic practice. No use, distribution or reproduction is permitted which does not comply with these terms.
*Correspondence: Sergio Fagherazzi, c2VyZ2lvQGJ1LmVkdQ==
Disclaimer: All claims expressed in this article are solely those of the authors and do not necessarily represent those of their affiliated organizations, or those of the publisher, the editors and the reviewers. Any product that may be evaluated in this article or claim that may be made by its manufacturer is not guaranteed or endorsed by the publisher.
Research integrity at Frontiers
Learn more about the work of our research integrity team to safeguard the quality of each article we publish.