- Department of Agrifood Production and Environmental Sciences (DISPAA), University of Florence, Florence, Italy
Cyanobacteria are ubiquitous components of biocrust communities and the first colonizers of terrestrial ecosystems. They play multiple roles in the soil by fixing C and N and synthesizing exopolysaccharides, which increase soil fertility and water retention and improve soil structure and stability. Application of cyanobacteria as inoculants to promote biocrust development has been proposed as a novel biotechnological technique for restoring barren degraded areas and combating desertification processes in arid lands. However, previous to their widespread application under field conditions, research is needed to ensure the selection of the most suitable species. In this study, we inoculated two cyanobacterial species, Phormidium ambiguum (non N-fixing) and Scytonema javanicum (N-fixing), on different textured soils (from silt loam to sandy), and analyzed cyanobacteria biocrust development and evolution of physicochemical soil properties for 3 months under laboratory conditions. Cyanobacteria inoculation led to biocrust formation in all soil types. Scanning electron microscope (SEM) images showed contrasting structure of the biocrust induced by the two cyanobacteria. The one from P. ambiguum was characterized by thin filaments that enveloped soil particles and created a dense, entangled network, while the one from S. javanicum consisted of thicker filaments that grouped as bunches in between soil particles. Biocrust development, assessed by chlorophyll a content and crust spectral properties, was higher in S. javanicum-inoculated soils compared to P. ambiguum-inoculated soils. Either cyanobacteria inoculation did not increase soil hydrophobicity. S. javanicum promoted a higher increase in total organic C and total N content, while P. ambiguum was more effective in increasing total exopolysaccharide (EPS) content and soil penetration resistance. The effects of cyanobacteria inoculation also differed among soil types and the highest improvement in soil fertility compared to non-inoculated soils was found in sandy and silty soils, which originally had lowest fertility. On the whole, the improvement in soil fertility and stability supports the viability of using cyanobacteria to restore degraded arid soils.
Introduction
Biotechnological techniques based on the use of microorganisms as soil inoculants are regarded as promising potential tools to improve soil quality and counteract soil degradation in disturbed dryland areas (Bowker, 2007; Maestre et al., 2017; Rossi et al., 2017). Indeed, recent studies suggested that manipulation of the soil community by inoculation can be the key for a successful restoration of terrestrial ecosystems (Wubs et al., 2016).
Cyanobacteria are prokaryotic oxygenic phototrophs that inhabit almost every habitat on Earth (Abed and García-Pichel, 2001). They have been widely used as biofertilizers in agriculture, mainly in paddy rice fields in Asia (Prasanna et al., 2009, 2013; Priya et al., 2015; Singh et al., 2016). However, studies on their application for biofertilizing and bioconditioning degraded arid soils are relatively few. Experiments under laboratory (Maqubela et al., 2009, 2012; Mugnai et al., 2018) and outdoor conditions (Wang et al., 2009; Wu et al., 2013; Lan et al., 2017; Park et al., 2017; Zaady et al., 2017) point to positive results in terms of soil stability and fertility.
A successful inoculation procedure employing cyanobacteria can bring to the development of biological soil crusts or “biocrusts,” i.e., assemblages of soil particles with bacteria, microalgae, microfungi, cyanobacteria, lichens and bryophytes, in varying proportions. Biocrusts are widespread components in drylands, covering from 40 to 100% of the interplant spaces and providing key ecosystem services in these environments (Maestre et al., 2011; Rodríguez-Caballero et al., in press) by affecting hydrological processes and soil water availability (Colica et al., 2014; Chamizo et al., 2016), soil stability (Rodríguez-Caballero et al., 2012), and nutrient cycling (Delgado-Baquerizo et al., 2013). Due to their recognized functions, biocrusts have been identified as relevant communities to effectively restore disturbed arid soils (Doherty et al., 2015; Antoninka et al., 2016; Velasco Ayuso et al., 2017). Within natural biocrust communities, cyanobacteria are pioneer organisms that improve soil conditions and the colonization of later-successional species, such as lichens and mosses (Belnap and Gardner, 1993; Lan et al., 2013). Both cyanobacterial filaments and their extracellular secretions, which are mostly composed of exopolysaccharides (EPSs), act as gluing agents, binding soil particles, and promoting the formation of soil aggregates, thus increasing soil stability (Mazor et al., 1996). EPSs also enhance water retention (Rossi et al., 2012, in press; Colica et al., 2014; Adessi et al., 2018) and protect microorganisms from desiccation and nutrient limitation, helping them to survive (Mazor et al., 1996; Zhang, 2005). Cyanobacteria fix CO2 and some species are also able to fix N2, while also releasing a wide array of substances in the soil, including phytormones, vitamins and phosphorus (Priya et al., 2015). They increase soil fertility in dryland regions (Zhao et al., 2010), where N, together with water, is a major limiting factor for ecosystem functioning (Noy-Meir, 1973). Cyanobacteria also provide a favorable microhabitat for soil biota (Liu et al., 2011) and improve vascular and annual plant performance (Xu et al., 2013; Lan et al., 2014). Due to these features and their high pervasiveness in every environment on Earth, cyanobacteria can be rightfully encompassed between ecosystem engineers, and regarded as potential soil restoration tools in drylands (Rossi et al., 2017).
Nevertheless, numerous knowledge and methodological gaps still need to be reduced before restoration strategies based on cyanobacteria inoculation guarantee a successful performance under field conditions. Selection of the most suitable species in terms of ease for culture growth, amount and type of EPSs synthesized, and resistance to highly stressing environments are crucial factors to be evaluated before any restoration project (Rossi et al., 2017). In addition, the selection of the species should base chiefly on the capability to promote biocrust development and improve soil properties. This selection must also take into account the soil type. In fact, soil properties such as texture, mineralogy composition, organic matter and nutrients contents, pH, and electrical conductivity greatly influence cyanobacterial growth and EPS production. Soil texture is perhaps the most important of these properties, affecting biocrust formation and structure and water dynamics in soils. So far, most cyanobacteria inoculation experiments have been performed on sandy soils (Rozenstein et al., 2014; Lan et al., 2017; Mugnai et al., 2018), although fine-textured soils are common in many desert areas. In addition, physical crusting is common in fine-textured soils and in combination with biocrust formation greatly affects the structure and hydraulic properties of the soil (Malam Issa et al., 2011; Chamizo et al., 2012a).
In this study, the capability of Phormidium ambiguum, a non N-fixing cyanobacterium, and Scytonema javanicum, a N-fixing cyanobacterium, to promote biocrust development on four soils with different particle size distribution and organic carbon (C) and nitrogen (N) contents was explored under laboratory conditions. Inoculation of S. javanicum and Phormidium sp. in varying proportions with other cyanobacterial species has been previously reported to have a positive effect on stability and organic C and N contents of sandy soils (Hu et al., 2002; Xie et al., 2007; Wang et al., 2009; Li et al., 2014). However, the potential of these species alone to induce biocrust formation and modify soil properties in soils with different particle size distributions has not been explored so far, although it would allow having a better understanding of the potential of such species as habitat amelioration agents.
The main goals of this study were to: (i) analyze the effect of inoculation of two cyanobacterial species on biocrust growth and soil stability, hydraulic and fertility properties in different textured soils, under laboratory conditions; (ii) examine changes in biocrust development and soil properties by cyanobacteria inoculation with time.
Material and Methods
Experimental Design
Four types of soil with contrasting particle size distributions were collected from two semiarid areas in the province of Almeria (SE Spain). From the finest to the coarsest, these soils were classified as: (1) silt loam, (2) sandy loam; (3) loamy sand, and (4) sandy. Particle size distribution for each soil type is shown in Table 1. The silt loam soil was collected from the Tabernas desert (37°00′37′′N, 2°26′37′′W), a badlands area characterized by shallow soils with poor structure and low N and organic matter content. Mean annual rainfall is 235 mm and mean annual temperature is 18°C. The other three soil types were collected from three different sites within the Cabo de Gata Natural Park. The climate is similar to the one of the Tabernas desert, with mean annual rainfall of 200 mm and mean annual temperature of 18°C (Chamizo et al., 2016). The loamy sand and sand soils were collected near “Las Amoladeras” experimental site (36°49′40′′N, 2°16′10′′W), a grassland area with flat topography. The sandy loam soil was collected from a semi-intensive rainfed olive crop area (36°48′13′′N, 2°08′32′′W). The sandy loam and loamy sand soils differ in their structure and chemical composition, the “agricultural” soil being less compact due to occasional tillage and having less carbonate and higher organic C and N contents than the loamy sand soil. The sampled soils were air-dried and sieved to 2 mm, and then autoclaved twice for 20 min at 120°C to suppress biological activity. Two cyanobacterial species were selected for the inoculation experiments: the non N-fixing Phormidium ambiguum Gomont NIES-2121, belonging to the order Oscillatoriales, and the widespread desert heterocystous Scytonema javanicum Bornet & Flahault NIES-1956, belonging to the order Nostocales. P. ambiguum was isolated from an African soil, while S. javanicum was isolated from the Tsukuba Botanical Garden (Japan). The genus Phormidium is a common colonizer of biocrusts that dwells in the deep soil layers, in contrast to Scytonema which preferentially colonizes the uppermost layer (Hu et al., 2002). The two cyanobacteria were grown in BG110 (S. javanicum) or BG11 (P. ambiguum) medium in a rotatory shaker (Innova 44B, New Brunswick, USA) at a constant temperature of 30°C, light intensity of 15 μmol photons m−2 s−1, and stirring speed of 100 rpm. For the inoculation experiment, the biomass was separated from the culture medium by centrifugation at 4000 x g for 30 min and then fragmented in a sterile plastic tube using a sterilized spatula. Finally, the biomass was suspended in distilled water and its dry weight determined. A concentration of 5 g (dry weight) m−2 was inoculated on Petri dishes containing the different soil types (henceforth, microcosms). Microcosms had dimensions of 12 mm height × 54 mm diameter and were filled with 30 g of sterilized soil. In these microcosms, ~30 mg of biomass was inoculated. Additionally, bigger microcosms (16 mm height × 88 mm diameter) were prepared with 80 g of each soil type and inoculated with the same concentration of the two cyanobacteria species (5 g m−2 or 40 mg on each microcosm). These samples were used to conduct the reflectance measurements and the SEM micrographs at the end of the experiment (90 days) (see below). Biomass was dispersed using a sterile 10 mL pipette and applying the biomass as homogeneously as possible over the surface, following a spiral distribution (Mugnai et al., 2018). Thus, three conditions were considered on each soil type: soil inoculated with P. ambiguum, soil inoculated with S. javanicum, and soil without any inoculum (control), each treatment replicated three times on each soil type. Three soil samplings were conducted during the experiment (30, 60, and 90 days after cyanobacteria inoculation), totalizing 108 small Petri dishes, whereas a total of 36 big microcosms (3 treatments × 4 soil types × 3 replicates) were prepared for the measurements at the end of the incubation period (90 days). Microcosms were incubated in a plexiglass growth chamber under controlled temperature (30°C), light intensity (45 μmol photons m−2 s−1), and relative humidity (0 %) for 90 days. Twice a week, 2 mm (5 mL in the small microcosms and 13 mL in the bigger microcosms) of distilled water were applied on the microcosms using a sprayer. Such water amount corresponds to the average annual rainfall in the study areas where the soils were from (~215 mm), calculated according to the duration of the experiment.
Crust Sampling and Physico-Chemical Soil Analyses
The crust was collected from the small microcosms after 30, 60, and 90 days since inoculation. Previous to crust sampling, hydrophobicity and penetration resistance were measured in all samples. Then, the crust was collected with a spatula from each microcosm and thickness was measured with a caliber. In the sandy soil, a very thin biocrust (1–2 mm) developed on the surface, while in the silt loam, sandy loam and loamy soils, the crust was thicker (7–8 mm). The entire crust was analyzed and, to compare the effect of biocrusts on soil properties, a weighed mean of all variables with soil depth (considering the 0–2 mm crust and the 2–8 mm underlying soil layer) was calculated for the sandy soil. For the aggregate stability test, a part of the crust was fragmented and sieved, while the rest was manually ground to a fine powder with mortar and pestle for the chemical analyses.
Soil Physical Analyses
Soil hydrophobicity
Soil water repellency was measured in control and inoculated soils at the three sampling dates using two different methods: the water drop penetration time (WDPT, as in Adams et al., 1969) and the repellency index (RI, as in Hallett and Young, 1999). While the former method gives information about the persistance of hydrophobicity in the soil, the latter provides information about the severity of hydrophobicity. The WDPT consists of counting the time a drop of water takes to seep into the soil. Six drops were placed on each microcosm using a small pipette with distilled water. Crusts were classified according to the classification by Adams et al. (1969) as hydrophilic or wettable (<10 s), slightly hydrophobic (10–60 s) and severely hydrophobic (>60 s). The RI was determined from sorptivity measurements at −2 cm pressure head for both water and a 95% ethanol solution using a miniaturized mini-infiltrometer and calculated according to Equation (1):
where SE is the sorptivity of ethanol, SW is the sorptivity of water, and the factor 1.95 accounts for differences in viscosity and surface tension between the two liquids (Tillman et al., 1989). A soil with RI < 1.95 (SE < SW) is considered non-repellent. Three water and ethanol sorptivity measurements were conducted on each microcosm.
Soil stability
Soil stability was assessed by means of two variables: (1) aggregate stability, and (2) maximum penetration resistance of the surface. Aggregate stability was measured at the three sampling dates (30, 60, and 90 days) using the water drop test (Imeson and Vis, 1984). This test simulates the resistance of crust aggregates to raindrop energy impact. Concretely, the energy of impact of the water drops used was equivalent to the energy of 2.18 mm of rain (Imeson and Vis, 1984). To conduct the test, the crusts were first fragmented and sieved to 4–4.8 mm size. The aggregates were placed on a 2.8-mm metal mesh sieve and water-drops of 0.1 g in weight (obtained by fitting a small silicon tube to the burette nozzle) were dropped from 1 m in height through a burette over the aggregates. We counted the number of drops until the aggregate passed through the sieve. The test was done on 15 aggregates per sample, except on the sandy soil, where the test was not performed due to the absence of crust aggregates with 4–4.8 mm size.
Maximum penetration resistance (PRmax) of the crust was measured at the end of the experiment (90 days) using a digital force gauge (Mark-10 Model M7-5, 25N, Mark-10 Corp, USA) equipped with a cone tip (5 mm length and 6 mm diameter). Samples were placed onto a lifting table that was raised up until the entire tip was inserted into the soil (5 mm soil depth). Four penetration-resistance readings were performed on each sample.
Surface reflectance
At the end of the experiment (90 days), surface reflectance was measured on the samples (previously sprayed with water) with an ASD hand held portable spectroradiometer (ASD Inc., Boulder, Colorado, USA), which measures reflectance over the 325–1075 nm range at a sampling interval of 3.5 nm. Spectral measurements were conducted with a fiber optic oriented 16 cm above the sample to measure the total surface of the Petri dish and using two halogen lamps opposite each other. The spectroradiometer was calibrated using a 99% Spectralon(r) panel prior to measuring each sample. Then, two spectra were taken per sample, each one consisting on the internal average of three individual spectra. All reflectance values were expressed proportional to the 99% Spectralon reflectance panel and normalized to 100% reflectance. Data were acquired with the RS3 Spectral Acquisition Software on a laptop connected to the spectroradiometer. Spectra processing included removal of noisy bands between 325 and 400 nm and 950 and 1075 nm and data smoothing using the Savitsky–Golay algorithm (Savitzky and Golay, 1964). From the smoothed spectra, two spectral variables that have been used as indicators of biocrust development or recovery after disturbance were calculated: the Brightness Index (BI) and the Normalized Difference Vegetation Index (NDVI). The brightness index was calculated according to equation 2:
where G, R, and NIR are the integrated reflectances for the green (500–600 nm), red (600–700 nm), and near-infrared bands (NIR, 700–950 nm), respectively. The Normalized Difference Vegetation Index (NDVI) was calculated as follow:
where R and NIR are the integrated reflectances in the red (600–700) and near-infrared bands (700–950 nm), respectively. Higher positive values of this index indicate greater biocrust development.
Sample's surface structure
After performing the reflectance measurements, small crust pieces were collected from the big microcosms and pre-treated for scanning electron microscopy (SEM) analysis by sputtering them with a thin coating of gold (at 50 mA for 1 min). SEM micrographs were taken using an Environmental Scanning Electron Microscopy (Fei Quanta 200 ESEM, Fei Corporation, Eindhoven, Netherlands) operating in high-vacuum mode (10 kV).
Soil Chemical Analyses
Chlorophyll a
Chlorophyll a content was quantified according to Castle et al. (2011). In brief, one g of soil was weighted into a screw-cap vial with 5 mL of ethanol, then shacked in a vortex and heated at 80°C for 5 min. Samples were cooled at 4°C for 24 h and then centrifuged; the supernatant was finally analyzed by a spectrophotometer.
Exopolysaccharide content
Total exopolysaccharides, including both loosely and tightly bound fractions, were recovered from soils using three consecutive extractions with 20 mL of 0.1 M Na2EDTA each (Rossi et al., in press). The carbohydrate content of the extracts was determined using the phenol-sulfuric acid assay, measuring the absorbance at 488 nm with a UV-VIS spectrophotometer (Dubois et al., 1956). A calibration curve was created using glucose as standard. Three instrumental replicates were used for each sample.
Total organic C and N content
Organic C was measured on finely ground sample once treated with excess HCl to remove inorganic C. Total organic C and N contents were measured on 10–20 mg of the pulverized and oven-dried samples by dynamic dry flash combustion (1030°C under O2 flow) using a Carlo Erba NA 1500 CNS Analyzer.
Statistical Analyses
The effect of cyanobacteria treatment (control, P. ambiguum inoculation, and S. javanicum inoculation) and incubation time (30, 60, and 90 days) on RI, chlorophyll content, aggregate stability and total EPS content on each soil type was analyzed using General Linear Models (GLM), after testing for homogeneity of variance and transforming data when necessary. Differences between treatments at each incubation time were further evaluated for significance using the Fisher LSD post hoc test. Statistical differences among cyanobacteria treatments in the variables analyzed at the end of the experiment (organic C and N content, PRmax, BI, and NDVI) were checked by one-way ANOVA and the LSD test. A confidence interval of 95% (p < 0.05) was established. All statistical analyses were performed using STATISTICA 8.0 (StatSoft, Inc., Tulsa, Oklahoma, USA).
Results
Characteristics of the Developed Biocrusts
Inoculation of the two cyanobacteria led to formation of biocrusts in all soil types. A very thin biocrust developed on the surface of the sandy soil, whereas in the finer soils, which were more prone to soil sealing, a thicker crust was formed by association of cyanobacterial filaments with the compacted soil (Figure 1). Average crust thickness was 9.3 ± 1.7 mm in silt loam, 8.3 ± 1.5 mm in sandy loam, 9.2 ± 0.9 mm in loamy sand, and 1.0 ± 0.4 mm in sand soils. Crust thickness was similar for both cyanobacterial species and no significant difference was observed with incubation time.
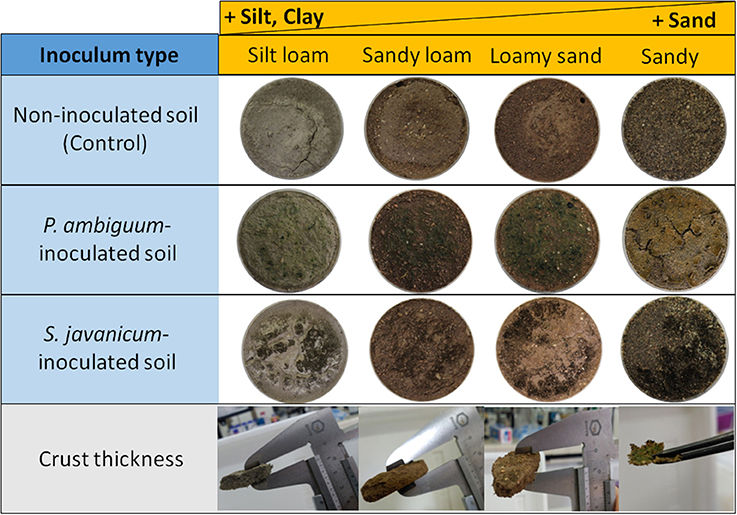
Figure 1. Picture of the control and inoculated samples for the different textured soils, and thickness of the crust developed on each soil type.
Cyanobacteria cover distribution over the soil surface differed between the two species. While P. ambiguum biomass was more homogeneously spread, S. javanicum showed a patchy distribution and tended to form small biomass aggregates near the border of the microcosms (Figure 1). In addition, the biocrust induced by P. ambiguum inoculation on the sandy soil was characterized by surface cracks, while a more even biocrust layer was observed on the same soil inoculated with S. javanicum. The SEM images also revealed differences between the two species in the morphology of cyanobacterial filaments and the spatial organization of soil particles (Figure 2). The thin P. ambiguum filaments wrapped soil particles and formed an entangled network that appeared as a blanket over the surface, while the relatively thicker S. javanicum filaments grouped as bunches in between soil particles, with a structure that resembled a “coral reef.” As expected, there were also major differences in the internal structure of the soil types. The microstructure of biocrusts induced by the inoculation of P. ambiguum on the silt loam soil (Figure 2A) resulted in a dense and tightly packed network without any voids. Conversely, the inoculation of S. javanicum on the same substrate produced biocrusts visibly composed by filament bundles and EPSs tightly bound to the filaments (Figure 2E). In particular, along the filaments, there were some spots where the sheath covered the filaments and other spots where filaments appeared thinner and without any sticky material attached. In the sandy loam soil (Figures 2B–F), biocrust induced by P. ambiguum inoculation resulted in a dense structure formed by web-like patterns of thin filaments. In contrast, S. javanicum-induced biocrust presented a non-homogeneous surface with low-density filaments where the sheath materials kept the soil particles together acting as cementing agents. Biocrust formation triggered by the inoculation of P. ambiguum on loamy sand soil (Figure 2C) was characterized by stable microaggregates where inter-particle cohesion was enhanced by polymer bridges. On the contrary, the S. javanicum crust (Figure 2G) showed filaments bundles that embedded soil particles and were distributed in an upward discontinuous surface without interspaces or porous structures. The biocrust developed on the sandy soil (Figures 2D–H) showed a lighter structure, with frequent air spaces between the sand grains. P. ambiguum tended to envelope soil particles in a thin layer of filaments that surrounded and bound sand particles into a tangled network (Figure 2D). On the same substrate, S. javanicum showed a lower number of filaments, with thicker shape compared to P. ambiguum, which entrapped and interconnected the sand grains (Figure 2H).
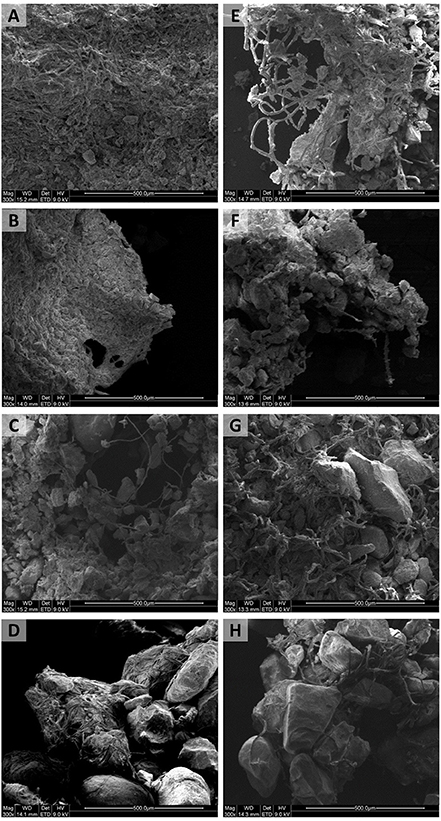
Figure 2. SEM pictures of the soil surface 90 days after inoculation in the different soils. Pictures on the left correspond to P. ambiguum-inoculated soils, while pictures on the right correspond to S. javanicum-inoculated soils. From the top to the bottom: silt loam (A,E), sandy loam (B,F), loamy sand (C,G), and sandy (D,H).
Biocrust Growth
Cyanobacteria inoculation and incubation time had significant effects on chlorophyll content (Table 2). The interaction between both factors was also significant, indicating that the effect of cyanobacteria depended on incubation time. Chlorophyll a content increased with time in both P. ambiguum-inoculated soils and, more significantly, in S. javanicum-inoculated soils, which showed the highest values after 90 days (Figure 3). From 30 to 90 days of incubation, the S. javanicum-inoculated soils showed an average increase in chlorophyll content of 121, 86, 321, and 251% in silt loam, sandy loam, loamy sand, and sand soils, respectively, while P. ambiguum-inoculated soils showed an average increase of 48, 75, 97, and 44%, respectively.
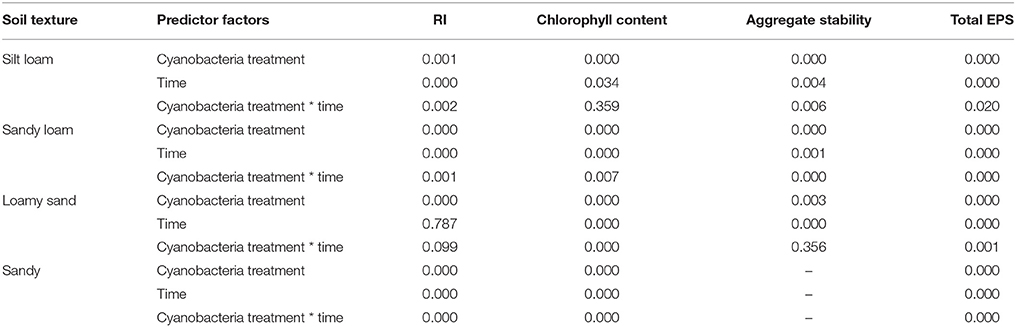
Table 2. p-values resulting from the GLM analysis considering as categorical predictors the cyanobacteria treatment (control, P. ambiguum, S. javanicum) and time of incubation (30, 60, and 90 days).
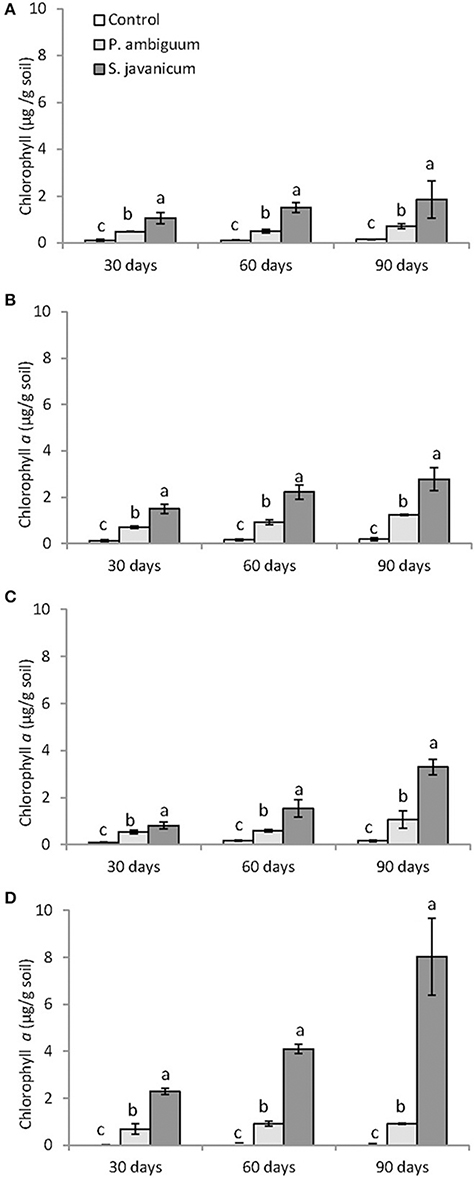
Figure 3. Chlorophyll content in control and inoculated soils after 30, 60, 90 days of incubation in the different soil types: silt loam (A), sandy loam (B), loamy sand (C), and sandy (D). Different letters indicate significant differences among cyanobacteria treatments.
The higher chlorophyll content in inoculated soils and, especially in those inoculated with S. javanicum, was supported by the deeper spectral absorption at 670 nm, which was absent in the non-inoculated soils (Figure 4). Biocrust development in inoculated soils was also reflected by changes in BI and NDVI compared to the non-inoculated ones. Cyanobacteria inoculation significantly decreased BI in sandy and, more notably, in the light-colored silt loam soil, while had no significant effect in the darker sandy loam and loamy sand soils (Table 3). Cyanobacteria inoculation had a significant effect on NDVI in all soils (p < 0.05). NDVI was higher in inoculated soils than in the control ones, and the highest values were observed in those inoculated with S. javanicum. The increase in NDVI differed between soils and was more remarkable in the silt loam and sandy soils compared to the sandy loam and loamy sand ones (Table 3).
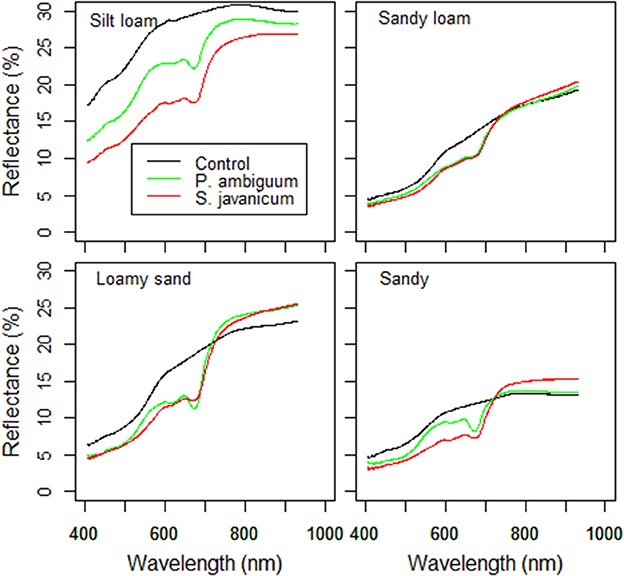
Figure 4. Surface reflectance of control and inoculated soils at the end of the incubation period (90 days).
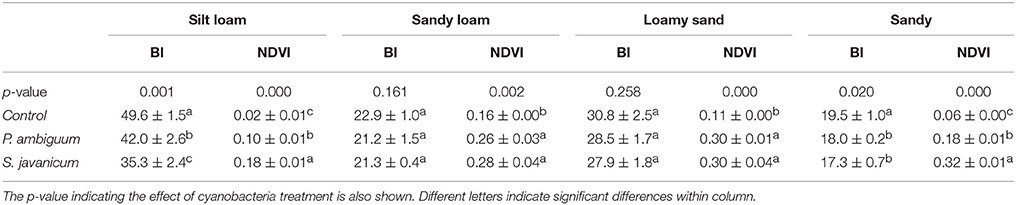
Table 3. Mean ± sd of the Brightness Index (BI) and Normalized Difference Vegetation Index (NDVI) in control and inoculated soils at the end of the incubation period (90 days).
Soil Hydrophobicity
All soils showed WDPT values lower than 5 s, thus indicating a hydrophilic behavior. The GLM analysis showed a significant effect of cyanobacteria treatment and time on RI (Table 2). However, such differences did not change substantially the soil water repellency in the presence of biocrusts, as all RI values were lower than 1.95 (Table 4). Noteworthy is the relatively higher RI values in control and P. ambiguum-inoculated soils compared to the S. javanicum-inoculated ones 30 days after the soil incubation (Table 4), with average values of 1.52 ± 0.45, 1.60 ± 0.26, and 1.00 ± 0.44, respectively. The small differences observed diminished at the end of the incubation period, when average RI values in the control, P. ambiguum-inoculated and S. javanicum-inoculated soils were 1.20 ± 0.50, 1.15 ± 0.45, and 1.02 ± 0.33, respectively.
Soil Stability
Aggregate stability was low in all the samples and, in general, less than 10 water drop impacts were enough to break crust aggregates. Cyanobacteria treatment and time, and their interaction, showed a significant effect on aggregate stability (Table 2). Aggregate stability increased with incubation time. However, this increase varied with inoculant and soil type. S. javanicum inoculation promoted the highest increase in aggregate stability with time, especially in the silt loam soil, which showed higher values than the sandy loam and loamy sand soils (Table 5). Although to a lesser extent, P. ambiguum inoculation as well increased aggregate stability compared to the control soil, mainly at the end of the incubation period (Table 5).
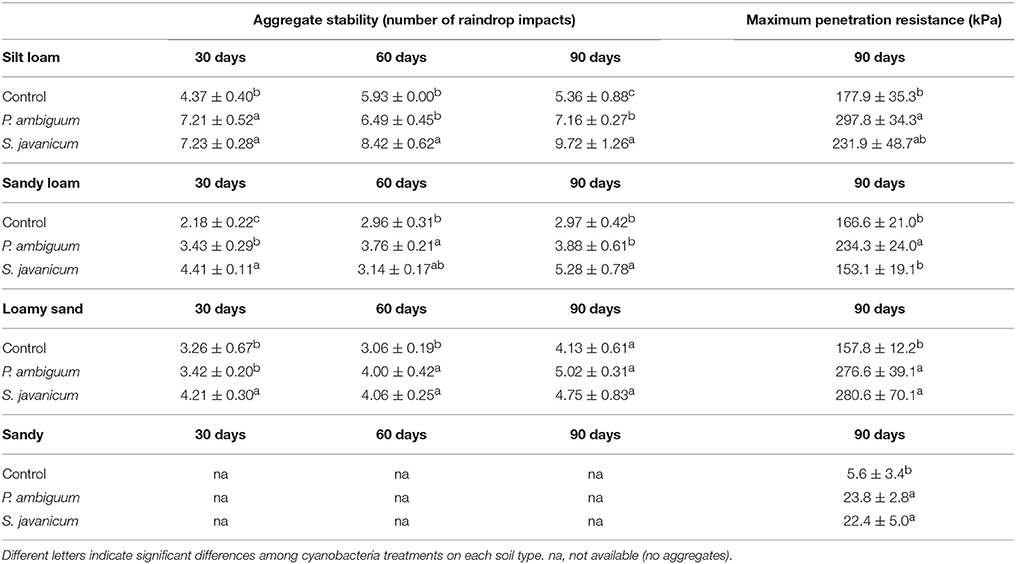
Table 5. Aggregate stability and maximum penetration resistance (mean ± sd) in inoculated and control soils.
Cyanobacteria inoculation increased penetration resistance of soil surface after 90 days of soil incubation, as shown by the significant effect of cyanobacteria treatment on PRmax in all soils (p = 0.029 in silt loam, p = 0.008 in sandy loam, p = 0.030 in loamy sand, and p = 0.002 in sandy soil). In contrast to the pattern observed in aggregate stability, soils inoculated with P. ambiguum showed higher PRmax than those inoculated with S. javanicum. P. ambiguum inoculation significantly increased penetration resistance in all soil types compared to the control soil, while S. javanicum inoculation significantly increased penetration resistance in the loamy sand and sandy soils. Important differences in PRmax were also observed among the four soils, the sandy soil showing lower values than the finer soils.
Organic Carbon and Nitrogen Content of the Soil
Cyanobacteria inoculation promoted a significant increase in EPS content with time in all soils (Table 2, Figure 5). The P. ambiguum-inoculated soils showed higher EPS content than the S. javanicum-inoculated soils in all soils except in the sandy one, where S. javanicum-inoculated soils showed the highest EPS content after 90 days. There were also differences in EPS content among soil types mainly associated to the inherent EPS content of the tested soils. Thus, the sandy loam soil, which was collected from an agricultural arid site, showed per se higher EPS content than the other soils, all from natural arid sites. After 90 days of soil incubation, the P. ambiguum-inoculated soils showed an average increase in EPS content compared to the control soil of 44, 39, 22, and 195% in silt loam, sandy loam, loamy sand, and sandy soils, respectively, while S. javanicum-inoculated soils showed an average increase of 27, 22, 24, and 355%, respectively. The EPS content represented between 9 and 18% of organic C content (on average, 13.1% ± 2.0) in inoculated silt loam, sandy loam, and loamy sand soils, while in the sandy soil, EPS content was 29–36% of organic C.
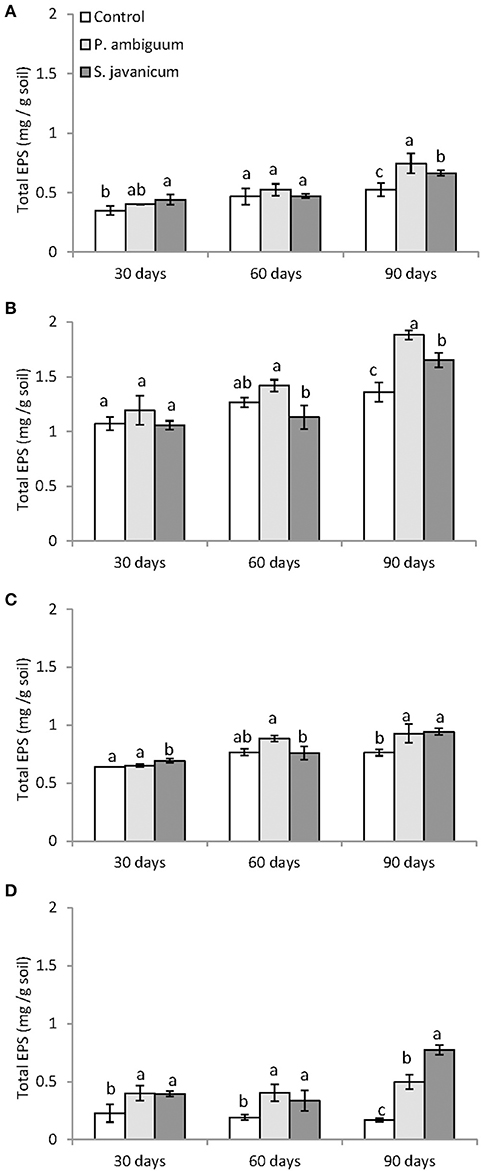
Figure 5. Total exopolysaccharide content in control and inoculated soils after 30, 60, 90 days of incubation in the different soil types: silt loam (A), sandy loam (B), loamy sand (C), and sandy (D). Different letters indicate significant differences among cyanobacteria treatments.
Cyanobacteria inoculation significantly increased organic C compared to the control soil only in the sandy soil, where P. ambiguum and S. javanicum inoculation caused an organic C increase of 42 and 83%, respectively (Table 6). Cyanobacteria inoculation had a significant effect on total N in all soils but in the sandy loam one. The effect of cyanobacteria on the N content depended on the species. In fact, P. ambiguum inoculation only significantly increased N content in the sandy soil, where the inoculated samples showed 15% higher N content compared to the non-inoculated ones. S. javanicum significantly increased the N content in all soils but the sandy loam one. Average increases in N were 11%, 10%, 14%, and 55% in silt loam, sandy loam, loamy sand, and sandy soils, respectively.

Table 6. Total organic carbon and total nitrogen content (mean ± sd) in inoculated and control soils.
Discussion
The soils utilized in this study for the inoculation experiments diverged not only in terms of particle size distribution. The sand soil showed the lowest organic C and N contents and very low penetration resistance, while the silt loam soil showed low organic C and N content, as expected for soils from badlands (Chamizo et al., 2016). The loamy sand soil showed relatively high organic C and N contents due to better conditions of the site where the soil was from, a flat area where runoff erosion is infrequent (Chamizo et al., 2016). The sandy loam soil had even higher organic C and N contents, but lower aggregate stability due to aggregate disruption by occasional tillage. These differences between the soils used for the inoculation tests were functional for investigating the effects of the inoculation of the two cyanobacteria on a range as broad as possible.
A thick and resistant crust developed upon inoculation in the soils with less than 80% of sand, whereas a very thin and more fragile biocrust developed in the sandy soil. In fine-textured soils, biocrusts commonly co-exist with different types of physical crusts (Malam Issa et al., 2011), which increase soil compaction compared to coarser textures. Actually, the SEM images of the inoculated fine-textured soils clearly showed a firmer attachment of the cyanobacterial filaments to finer particles (Figure 2).
The two cyanobacteria showed a completely different way of biocrust formation: P. ambiguum grew forming a homogeneously dispersed biocrust, whereas S. javanicum induced the formation of a patchy biocrust, with areas covered by the cyanobacterium and areas apparently without any filament (Figure 1), as further confirmed by the SEM images (Figure 2).
The two tested species increased soil stability in all soils (Table 5), but in different ways probably owing to the different volume of the two cyanobacterial species as well as differences observed in the microstructure of the biocrusts. The volume of S. javanicum can be more than 100-fold higher than that of Phormidium sp. (Hu et al., 2003). Indeed, SEM images pointed out that S. javanicum filaments were thicker and organized as branches in between soil particles, binding them and leading to greater aggregate stability than P. ambiguum (Figure 2). However, P. ambiguum spred more uniformly over the surface and its thinner filaments surrounded soil particles, creating an entangled web that significantly enhanced crust resistance to penetration. Greater surface resistance could be also due to the higher EPS content of the biocrusts promoted by P. ambiguum inoculation (Figure 5). In addition, S. javanicum and P. ambiguum colonize different soil depths in natural desert crusts, with the former dominating at a depth of 0.02-0.05 mm and the latter growing deeper into the soil and dominating at a depth of 1.0–3.0 mm (Hu et al., 2003; Wu et al., 2011). This capacity of P. ambiguum to occupy a deeper niche in the soil and subsequent dispersion of its EPS in the first millimeters of soil is expected to have a more effective role in increasing soil penetration resistance compared to S. javanicum, whose growth and effects in soil stability could be mostly restricted to the surface. Previous experiments have also shown that inoculation of Nostoc sp., Scytonema sp., Microcoleus vaginatus, and Phormidium sp. increase soil aggregation on sand (McKenna Neuman et al., 1996; Hu et al., 2002; Chen et al., 2006; Xie et al., 2007), sandy loam (Malam Issa et al., 2007), silty and silt loam (Maqubela et al., 2009, 2012) and clay soils (Falchini et al., 1996; de Caire et al., 1997; Nisha et al., 2007).
The above reported microscale differences in the biocrusts are correlated to both the morphological differences of the two cyanobacteria used as inoculants and their different capability of growing in the soil. S. javanicum-inoculated soils showed the highest chlorophyll content with time in all soil types and, more remarkably, in the sandy soil (Figure 3). Higher size of S. javanicum filaments could provide them with a greater ability to trap and grow on coarse soil particles, while the colonization by P. ambiguum seems more confined due to lower size of their filaments and easier movement down into the large pores between coarse sand grains, as reported also for Microcoleus vaginatus (Rozenstein et al., 2014). The greater synthesis of chlorophyll observed in S. javanicum is most probably due to the efficient N metabolism typical of heterocystous cyanobacteria (Hu et al., 2003). S. javanicum is also a desiccation-tolerant species capable of synthesizing UV screening pigments (García-Pichel and Castenholz, 1991; Chen et al., 2013; Rastogi et al., 2014). Despite the low light intensity used in this study, the dry conditions imposed by high temperature and low water availability during the experiment could have favored the synthesis of UV screening and other photosynthetic pigments by S. javanicum, explaining the higher chlorophyll content compared to P. ambiguum.
Together with chlorophyll content, surface spectral indices were good indicators of biocrust development in the different soil types, as also shown by previous studies (Zaady et al., 2007; Belnap et al., 2008; Chamizo et al., 2012b; Rodríguez-Caballero et al., 2015). The BI index reflected the increase in surface darkness with biocrust development and was closely related to chlorophyll content, showing the highest values in S. javanicum-inoculated soils (Table 3). The NDVI was a good indicator not only of biocrust development but also of soil quality, as values of this index were higher in soils which had higher EPS, organic C and N content (Table 3). The NDVI values found in inoculated soils in the current study were in the range reported by Fischer et al. (2012) for incipient and well-developed biocrusts and showed maxima of 0.30 units, similarly to those found for lower plants (Karnieli et al., 1996); this indicates a considerable photosynthetic biomass growth promoted by cyanobacteria inoculation.
Biocrusts have been previously reported to increase soil hydrophobicity through the synthesis of hydrophobic organic compounds or swelling of cyanobacteria filaments and EPS upon wetting (Fischer et al., 2010; Lichner et al., 2012). However, in the current study, in agreement with Mugnai et al. (2018), soil hydrophobicity was not significantly increased by cyanobacteria inoculation, as supported by WDPT < 5 s and RI < 1.95 (Table 4). Moreover, the RI values were slightly higher in the control soils, suggesting that improvement in soil aggregation and EPS content by cyanobacteria addition could even have a decreasing effect on soil water repellence.
Several studies have shown increases in nutrients content in natural and agricultural soils after cyanobacteria inoculation (Nisha et al., 2007; Maqubela et al., 2009; Lan et al., 2013; Singh et al., 2016; Rossi et al., 2017). In the current study, cyanobacteria growth was accompanied by a significant increase in EPS amount in the soil with time. P. ambiguum-inoculated soils, despite showing lower chlorophyll content, generally exhibited higher EPS content than S. javanicum-inoculated soils (Figure 5). Higher synthesis of EPS by P. ambiguum could be a strategy to cope with stress conditions imposed by water scarcity and more limited N availability compared to S. javanicum. Only in the sand soil, inoculation of S. javanicum led to higher EPS content than P. ambiguum, which was associated to a much greater photosynthetic biomass.
The increase in EPS was more remarkable in the sandy soil due to the very low EPS content of such soil compared to the silt loam, loamy sand, and sandy loam ones, which initially showed higher values. Despite the increase in EPS content with cyanobacteria inoculation, no significant increase in organic C content was observed in inoculated compared to control soils, with the exception of S. javanicum on sandy soils. Nevertheless, cyanobacteria inoculation did increase N content of soils, with different effects depending on species (Table 6). The presence of heterocysts in Scytonema sp. enables them to fix N and make an efficient use of light to fix N compared to non-heterocystous species (Johnson et al., 2005; Yeager et al., 2007; Abed et al., 2010). Thus, soils inoculated with S. javanicum showed higher N content than P. ambiguum-inoculated soils. Nonetheless, contribution of non-heterocystous cyanobacteria to N fixation can be important (Abed et al., 2010). In this regard, we found that the inoculation of P. ambiguum also increased the N content in the sandy soil (Table 6).
In summary, our results point out a higher ability of S. javanicum to increase some soil properties crucial for fertility and a greater effect of P. ambiguum in increasing soil stability. In this regard, a recent study has shown that the species Phormidium tenue was dominant in crusts of different ages in the Loess Plateau (China) and, together with Oscillatoria sp., could increase carbon and nitrogen content in later biocrust successional stages, thus making it a suitable candidate for artificial cultivation in both early and later biocrust successional stages (Zhang et al., 2017). On the whole, our findings highlight the appropriateness of species selection to increase the success of restoration strategies based on cyanobacteria application under field conditions.
Conclusions
Soil inoculation with two cyanobacteria species, one N-fixing and one non N-fixing, led to rapid biocrust formation and improved soil stability and/or fertility properties on different soils. The positive effects greatly varied depending on species and soil characteristics, mainly soil texture. A thinner and more fragile biocrust developed in the sandy soil, while a stable and resistant cyanobacterial biocrust developed in the finer soil textures. Inoculation of S. javanicum had a greater effect on photosynthetic biomass and soil nitrogen content, while inoculation of P. ambiguum had a more important effect on EPS amount and soil strength. Moreover, increase in soil fertility properties was greater in soils with initially lower physical and chemical soil quality. In light of these results, it is worth stressing that the selection of cyanobacterial species to be used as soil inoculants based on their main functional roles and suitability for a given soil is an important issue to consider to maximize the positive effects on soil quality. Further research about the applicability of cyanobacteria inoculation in different ecosystems with different soil types and climate conditions are needed to develop a complete, versatile, and applicable decision system for restoration of arid lands based on the induction of biocrust formation.
Author Contributions
SC conceived the idea, designed the experiment, performed the laboratory analyses, and wrote the manuscript. GM and FR designed the experiment, performed the laboratory analyses and improved manuscript editing. GC performed the laboratory analyses and improved manuscript editing. RD conceived the idea, designed the experiment, and wrote the manuscript. All authors contributed with constructive comments to the manuscript.
Funding
This study has received funding from the European Union's Horizon 2020 research and innovation programme under the Marie Sklodowska-Curie grant agreement N° 706351, Project Cyano4REST.
Conflict of Interest Statement
The authors declare that the research was conducted in the absence of any commercial or financial relationships that could be construed as a potential conflict of interest.
Acknowledgments
We greatly thank Matilde Ciani, Chiara Pastacaldi and Lisa Cangioli for their valuable help in the laboratory analyses. Emilio Rodríguez, Raúl Román, Albert Solé, Beatriz Roncero, and Yolanda Cantón are thanked for soil collection from field sites. Montserrat Guerrero is thanked for the soil texture analyses.
References
Abed, R. M., Al Kharusi, S., Schramm, A., and Robinson, M. D. (2010). Bacterial diversity, pigments and nitrogen fixation of biological desert crusts from the Sultanate of Oman. FEMS Microbiol. Ecol. 72, 418–428. doi: 10.1111/j.1574-6941.2010.00854.x
Abed, R. M. M., and Garcia-Pichel, F. (2001). Long-term compositional changes after transplant in a microbial mat cyanobacterial community revealed using a polyphasic approach. Environ. Microbiol. 3, 53–62. doi: 10.1046/j.1462-2920.2001.00159.x
Adams, S., Strain, B. R., and Adams, M. S. (1969). “Water-repellent soils and annual plant cover in desert scrub community of south eastern California,” in Proceedings of the Symposium on Water-Repellent Soils, University of California (Riverside), 289–295.
Adessi, A., Cruz de Carvalho, R., De Philippis, R., Branquinho, C., and Marques da Silva, J. (2018). Microbial extracellular polymeric substances improve water retention in dryland biological soil crusts. Soil Biol. Biochem. 116, 67–69. doi: 10.1016/j.soilbio.2017.10.002
Antoninka, A., Bowker, M. A., Reed, S. C., and Doherty, K. (2016). Production of greenhouse-grown biocrust mosses and associated cyanobacteria to rehabilitate dryland soil function. Restor. Ecol. 24, 324–335. doi: 10.1111/rec.12311
Belnap, J., and Gardner, J. S. (1993). Soil microstructure in soils of the Colorado Plateau: the role of the cyanobacterium Microcoleus vaginatus. Great Basin Nat. 53, 40–47.
Belnap, J., Phillips, S. L., Witwicki, D. L., and Miller, M. E. (2008). Visually assessing the level of development and soil surface stability of cyanobacterially dominated biological soil crusts. J. Arid Environ. 72, 1257–1264. doi: 10.1016/j.jaridenv.2008.02.019
Bowker, M. A. (2007). Biological soil crust rehabilitation in theory and practice: an underexploited opportunity. Restor. Ecol. 15, 13–23. doi: 10.1111/j.1526-100X.2006.00185.x
Castle, S. C., Morrison, C. D., and Barger, N. N. (2011). Extraction of chlorophyll a from biological soil crusts: a comparison of solvents for spectrophotometric determination. Soil Biol. Biochem. 43, 853–856. doi: 10.1016/j.soilbio.2010.11.025
Chamizo, S., Cantón, Y., Lázaro, R., Solé-Benet, A., and Domingo, F. (2012a). Crust composition and disturbance drive infiltration through biological soil crusts in semiarid ecosystems. Ecosystems 15, 148–161. doi: 10.1007/s10021-011-9499-6
Chamizo, S., Cantón, Y., Rodríguez-Caballero, E., and Domingo, F. (2016). Biocrusts positively affect the soil water balance in semiarid ecosystems. Ecohydrology 9, 1208–1221. doi: 10.1002/eco.1719
Chamizo, S., Stevens, A., Cantón, Y., Miralles, I., Domingo, F., and Van Wesemael, B. (2012b). Discriminating soil crust type, development stage and degree of disturbance in semiarid environments from their spectral characteristics. Eur. J. Soil Sci. 63, 42–53. doi: 10.1111/j.1365-2389.2011.01406.x
Chen, L., Deng, S., De Philippis, R., Tian, W., Wu, H., and Wang, J. (2013). UV-B resistance as a criterion for the selection of desert microalgae to be utilized for inoculating desert soils. J. Appl. Phycol. 25, 1009–1015. doi: 10.1007/s10811-012-9906-1
Chen, L., Xie, Z., Hu, C., Li, D., Wang, G., and Liu, Y. (2006). Man-made desert algal crusts as affected by environmental factors in Inner Mongolia, China. J. Arid Environ. 67, 521–527. doi: 10.1016/j.jaridenv.2006.02.018
Colica, G., Li, H., Rossi, F., Li, D., Liu, Y., and De Philippis, R. (2014). Microbial secreted exopolysaccharides affect the hydrological behavior of induced biological soil crusts in desert sandy soils. Soil Biol. Biochem. 68, 62–70. doi: 10.1016/j.soilbio.2013.09.017
de Caire, G. Z., de Cano, M. S., Zaccaro de Mulé, M. C., Palma, R. M., and Colombo, K. (1997). Exopolysaccharide of Nostoc muscorum (Cyanobacteria) in the aggregation of soil particles. J. Appl. Phycol. 9, 249–253. doi: 10.1023/A:1007994425799
Delgado-Baquerizo, M., Maestre, F. T., and Gallardo, A. (2013). Biological soil crusts increase the resistance of soil nitrogen dynamics to changes in temperatures in a semi-arid ecosystem. Plant Soil 366, 35–47. doi: 10.1007/s11104-012-1404-3
Doherty, K. D., Antoninka, A. J., Bowker, M. A., Ayuso, S. V., and Johnson, N. C. (2015). A novel approach to cultivate biocrusts for restoration and experimentation. Ecol. Restor. 33, 13–16. doi: 10.3368/er.33.1.13
Dubois, M., Gilles, K. A., Hamilton, J. K., Rebers, P. A., and Smith, F. (1956). Colorimetric method for determination of sugars and related substances. Anal. Chem. 28, 350–356. doi: 10.1021/ac60111a017
Falchini, L., Sparvoli, E., and Tomaselli, L. (1996). Effect of Nostoc (Cyanobacteria) inoculation on the structure and stability of clay soils. Biol. Fert. Soils 23, 346–352. doi: 10.1007/BF00335965
Fischer, T., Veste, M., Eisele, A., Bens, O., Spyra, W., and Hüttl, R. F. (2012). Small scale spatial heterogeneity of Normalized Difference Vegetation Indices (NDVIs) and hot spots of photosynthesis in biological soil crusts. Flora 207, 159–167. doi: 10.1016/j.flora.2012.01.001
Fischer, T., Veste, M., Wiehe, W., and Lange, P. (2010). Water repellency and pore clogging at early successional stages of microbiotic crusts on inland dunes, Brandenburg, NE Germany. Catena 80, 47–52. doi: 10.1016/j.catena.2009.08.009
García-Pichel, F., and Castenholz, R. W. (1991). Characterization and biological implications of scytonemin, a cyanobacterial sheath pigment. J. Phycol. 27, 395–409. doi: 10.1111/j.0022-3646.1991.00395.x
Hallett, P. D., and Young, I. M. (1999). Changes to water repellence of soil aggregates caused by substrate-induced microbial activity. Eur. J. Soil Sci. 50, 35–40. doi: 10.1046/j.1365-2389.1999.00214.x
Hu, C., Liu, Y., Song, L., and Zhang, D. (2002). Effect of desert soil algae on the stabilization of fine sands. J. Appl. Phycol. 14, 281–292. doi: 10.1023/A:1021128530086
Hu, C., Zhang, D., Huang, Z., and Liu, Y. (2003). The vertical microdistribution of cyanobacteria and green algae within desert crusts and the development of the algal crusts. Plant Soil 257, 97–111. doi: 10.1023/A:1026253307432
Imeson, A. C., and Vis, M. (1984). Assessing soil aggregate stability by water-drop impact and ultrasonic dispersion. Geoderma 34, 185–200. doi: 10.1016/0016-7061(84)90038-7
Johnson, S. L., Budinoff, C. R., Belnap, J., and Garcia-Pichel, F. (2005). Relevance of ammonium oxidation within biological soil crust communities. Environ. Microbiol. 7, 1–12. doi: 10.1111/j.1462-2920.2004.00649.x
Karnieli, A., Shachak, M., Tsoar, H., Zaady, E., Kaufman, Y., Danin, A., et al. (1996). The effect of microphytes on the spectral reflectance of vegetation in semiarid regions. Remote Sens. Environ. 57, 88–96. doi: 10.1016/0034-4257(95)00209-X
Lan, S., Wu, L., Yang, H., Zhang, D., and Hu, C. (2017). A new biofilm based microalgal cultivation approach on shifting sand surface for desert cyanobacterium Microcoleus vaginatus. Bioresource Technol. 238, 602–608. doi: 10.1016/j.biortech.2017.04.058
Lan, S., Wu, L., Zhang, D., and Hu, C. (2013). Assessing level of development and successional stages in biological soil crusts with biological indicators. Microb. Ecol. 66, 394–403. doi: 10.1007/s00248-013-0191-6
Lan, S., Zhang, Q., Wu, L., Liu, Y., Zhang, D., and Hu, C. (2014). Artificially accelerating the reversal of desertification: cyanobacterial inoculation facilitates the succession of vegetation communities. Environ. Sci. Technol. 48, 307–315. doi: 10.1021/es403785j
Li, H., Rao, B., Wang, G., Shen, S., Li, D., Hu, C., et al. (2014). Spatial heterogeneity of cyanobacteria-inoculated sand dunes significantly influences artificial biological soil crusts in the Hopq Desert (China). Environ. Earth Sci. 71, 245–253. doi: 10.1007/s12665-013-2428-6
Lichner, E., Holko, L., Zhukova, N., Schacht, K., Rajkai, K., Fodor, N., et al. (2012). Plants and biological soil crust influence the hydrophysical parameters and water flow in an aeolian sandy soil. J. Hydrol. Hydromech. 60, 309–318. doi: 10.2478/v10098-012-0027-y
Liu, Y., Li, X., Jia, R., Huang, L., Zhou, Y., and Gao, Y. (2011). Effects of biological soil crusts on soil nematode communities following dune stabilization in the Tengger Desert, Northern China. Appl. Soil Ecol. 49, 118–124. doi: 10.1016/j.apsoil.2011.06.007
Maestre, F. T., Bowker, M. A., Cantón, Y., Castillo-Monroy, A. P., Cortina, J., Escolar, C., et al. (2011). Ecology and functional roles of biological soil crusts in semi-arid ecosystems of Spain. J. Arid Environ. 75, 1282–1291. doi: 10.1016/j.jaridenv.2010.12.008
Maestre, F. T., Solé, R., and Singh, B. K. (2017). Microbial biotechnology as a tool to restore degraded drylands. Microb. Biotechnol. 10, 1250–1253. doi: 10.1111/1751-7915.12832
Malam Issa, O., Défarge, C., Le Bissonnais, Y., Marin, B., Duval, O., Bruand, A., et al. (2007). Effects of the inoculation of cyanobacteria on the microstructure and the structural stability of a tropical soil. Plant Soil 290, 209–219. doi: 10.1007/s11104-006-9153-9
Malam Issa, O., Valentin, C., Rajot, J. L., Cerdan, O., Desprats, J. F., and Bouchet, T. (2011). Runoff generation fostered by physical and biological crusts in semi-arid sandy soils. Geoderma 167–168, 22–29. doi: 10.1016/j.geoderma.2011.09.013
Maqubela, M. P., Mnkeni, P. N. S., Issa, O. M., Pardo, M. T., and D'Acqui, L. P. (2009). Nostoc cyanobacterial inoculation in South African agricultural soils enhances soil structure, fertility, and maize growth. Plant Soil 315, 79–92. doi: 10.1007/s11104-008-9734-x
Maqubela, M. P., Muchaonyerwa, P., and Mnkeni, P. N. S. (2012). Inoculation effects of two South African cyanobacteria strains on aggregate stability of a silt loam soil. Afr. J. Biotechnol. 11, 10726–10735. doi: 10.5897/AJB11.2111
Mazor, G., Kidron, G. J., Vonshak, A., and Abeliovich, A. (1996). The role of cyanobacterial exopolysaccharides in structuring desert microbial crusts. FEMS Microbiol. Ecol. 21, 121–130. doi: 10.1111/j.1574-6941.1996.tb00339.x
McKenna Neuman, C., Maxwell, C. D., and Boulton, J. W. (1996). Wind transport of sand surfaces crusted with photoautotrophic microorganisms. Catena 27, 229–247. doi: 10.1016/0341-8162(96)00023-9
Mugnai, G., Rossi, F., Felde, V. J. M. N. L., Colesie, C., Büdel, B., Peth, S., et al. (2018). Development of the polysaccharidic matrix in biocrusts induced by a cyanobacterium inoculated in sand microcosms. Biol. Fert. Soils 54, 27–40. doi: 10.1007/s00374-017-1234-9
Nisha, R., Kaushik, A., and Kaushik, C. P. (2007). Effect of indigenous cyanobacterial application on structural stability and productivity of an organically poor semi-arid soil. Geoderma 138, 49–56. doi: 10.1016/j.geoderma.2006.10.007
Noy-Meir, I. (1973). Desert ecosystems: environment and producers. Annu. Rev. Ecol. Syst. 4, 25–51. doi: 10.1146/annurev.es.04.110173.000325
Park, C. H., Li, X. R., Zhao, Y., Jia, R. L., and Hur, J. S. (2017). Rapid development of cyanobacterial crust in the field for combating desertification. PLoS ONE 12:e0179903. doi: 10.1371/journal.pone.0179903
Prasanna, R., Babu, S., Rana, A., Kabi, S. R., Chaudhary, V., Gupta, V., et al. (2013). Evaluating the establishment and agronomic proficiency of cyanobacterial consortia as organic options in wheat-rice cropping sequence. Exp. Agr. 49, 416–434. doi: 10.1017/S001447971200107X
Prasanna, R., Jaiswal, P., Nayak, S., Sood, A., and Kaushik, B. D. (2009). Cyanobacterial diversity in the rhizosphere of rice and its ecological significance. Indian J. Microbiol. 49, 89–97. doi: 10.1007/s12088-009-0009-x
Priya, H., Prasanna, R., Ramakrishnan, B., Bidyarani, N., Babu, S., Thapa, S., et al. (2015). Influence of cyanobacterial inoculation on the culturable microbiome and growth of rice. Microbiol. Res. 171, 78–89. doi: 10.1016/j.micres.2014.12.011
Rastogi, R. P., Sonani, R. R., and Madamwar, D. (2014). The high-energy radiation protectant extracellular sheath pigment scytonemin and its reduced counterpart in the cyanobacterium Scytonema sp. R77DM. Bioresour. Technol. 171, 396–400. doi: 10.1016/j.biortech.2014.08.106
Rodríguez-Caballero, E., Cantón, Y., Chamizo, S., Afana, A., and Solé-Benet, A. (2012). Effects of biological soil crusts on surface roughness and implications for runoff and erosion. Geomorphology 145–146, 81–89. doi: 10.1016/j.geomorph.2011.12.042
Rodríguez-Caballero, E., Castro, A. J., Chamizo, S., Quintas-Soriano, C., Garcia-Llorente, M., Cantón, Y., et al. (in press). Ecosystem services provided by biocrusts: from ecosystem functions to social values. J. Arid Environ. doi: 10.1016/j.jaridenv.2017.09.005, et al.
Rodríguez-Caballero, E., Knerr, T., and Weber, B. (2015). Importance of biocrusts in dryland monitoring using spectral indices. Remote Sens. Environ. 170, 32–39. doi: 10.1016/j.rse.2015.08.034
Rossi, F., Li, H., Liu, Y., and De Philippis, R. (2017). Cyanobacterial inoculation (cyanobacterisation): Perspectives for the development of a standardized multifunctional technology for soil fertilization and desertification reversal. Earth-Sci. Rev. 171, 28–43. doi: 10.1016/j.earscirev.2017.05.006
Rossi, F., Mugnai, G., and De Philippis, R. (in press). Complex role of the polymeric matrix in biological soil crusts. Plant Soil. doi: 10.1007/s11104-017-3441-4
Rossi, F., Potrafka, R. M., Pichel, F. G., and De Philippis, R. (2012). The role of the exopolysaccharides in enhancing hydraulic conductivity of biological soil crusts. Soil Biol. Biochem. 46, 33–40. doi: 10.1016/j.soilbio.2011.10.016
Rozenstein, O., Zaady, E., Katra, I., Karnieli, A., Adamowski, J., and Yizhaq, H. (2014). The effect of sand grain size on the development of cyanobacterial biocrusts. Aeolian Res. 15, 217–226. doi: 10.1016/j.aeolia.2014.08.003
Savitzky, A., and Golay, M. J. E. (1964). Smoothing and differentiation of data by simplified least squares procedures. Anal. Chem. 36, 1627–1639. doi: 10.1021/ac60214a047
Singh, J. S., Kumar, A., Rai, A. N., and Singh, D. P. (2016). Cyanobacteria: a precious bio-resource in agriculture, ecosystem, and environmental sustainability. Front. Microbiol. 7:529. doi: 10.3389/fmicb.2016.00529
Tillman, R., Scotter, D., Wallis, M., and Clothier, B. (1989). Water repellency and its measurement by using intrinsic sorptivity. Soil Res. 27, 637–644. doi: 10.1071/SR9890637
Velasco Ayuso, S., Giraldo Silva, A., Nelson, C., Barger, N. N., and Garcia-Pichel, F. (2017). Microbial nursery production of high-quality biological soil crust biomass for restoration of degraded dryland soils. Appl. Environ. Microb. 83:e02179–16. doi: 10.1128/AEM.02179-16
Wang, W., Liu, Y., Li, D., Hu, C., and Rao, B. (2009). Feasibility of cyanobacterial inoculation for biological soil crusts formation in desert area. Soil Biol. Biochem. 41, 926–929. doi: 10.1016/j.soilbio.2008.07.001
Wu, L., Lan, S., Zhang, D., and Hu, C. (2011). Small-scale vertical distribution of algae and structure of lichen soil crusts. Microb. Ecol. 62, 715–724. doi: 10.1007/s00248-011-9828-5
Wu, Y., Rao, B., Wu, P., Liu, Y., Li, G., and Li, D. (2013). Development of artificially induced biological soil crusts in fields and their effects on top soil. Plant Soil 370, 115–124. doi: 10.1007/s11104-013-1611-6
Wubs, E. R., Van Der Putten, W. H., Bosch, M., and Bezemer, T. M. (2016). Soil inoculation steers restoration of terrestrial Ecosystems. Nat. Plants 2:16107. doi: 10.1038/nplants.2016.107
Xie, Z., Liu, Y., Hu, C., Chen, L., and Li, D. (2007). Relationships between the biomass of algal crusts in fields and their compressive strength. Soil Biol. Biochem. 39, 567–572. doi: 10.1016/j.soilbio.2006.09.004
Xu, Y., Rossi, F., Colica, G., Deng, S., De Philippis, R., and Chen, L. (2013). Use of cyanobacterial polysaccharides to promote shrub performances in desert soils: a potential approach for the restoration of desertified areas. Biol. Fert. Soils 49, 143–152. doi: 10.1007/s00374-012-0707-0
Yeager, C. M., Kornosky, J. L., Morgan, R. E., Cain, E. C., García-Pichel, F., Housman, D. C., et al. (2007). Three distinct clades of cultured heterocystous cyanobacteria constitute the dominant N2-fixing members of biological soil crusts of the Colorado Plateau, USA. FEMS Microbiol. Ecol. 60, 85–97. doi: 10.1111/j.1574-6941.2006.00265.x
Zaady, E., Karnieli, A., and Shachak, M. (2007). Applying a field spectroscopy technique for assessing successional trends of biological soil crusts in a semi-arid environment. J. Arid Environ. 70, 463–477. doi: 10.1016/j.jaridenv.2007.01.004
Zaady, E., Katra, I., Barkai, D., Knoll, Y., and Sarig, S. (2017). The coupling effects of using coal fly-ash and bio-inoculant for rehabilitation of disturbed biocrusts in active sand dunes. Land Degrad. Dev. 28, 1228–1236. doi: 10.1002/ldr.2510
Zhang, Y. (2005). The microstructure and formation of biological soil crusts in their early developmental stage. Chinese Sci. Bull. 50, 117–121. doi: 10.1007/BF02897513
Zhang, Y., Duan, P., Zhang, P., and Li, M. (2017). Variations in cyanobacterial and algal communities and soil characteristics under biocrust development under similar environmental conditions. Plant Soil. doi: 10.1007/s11104-017-3443-2
Keywords: autotrophic organisms, biocrust development, exopolysaccharides, organic carbon, soil nitrogen, soil degradation
Citation: Chamizo S, Mugnai G, Rossi F, Certini G and De Philippis R (2018) Cyanobacteria Inoculation Improves Soil Stability and Fertility on Different Textured Soils: Gaining Insights for Applicability in Soil Restoration. Front. Environ. Sci. 6:49. doi: 10.3389/fenvs.2018.00049
Received: 07 March 2018; Accepted: 22 May 2018;
Published: 11 June 2018.
Edited by:
Philippe C. Baveye, AgroParisTech Institut des Sciences et Industries du Vivant et de L'environnement, FranceReviewed by:
Ming Li, Northwest A& F University, ChinaAlok Chandra Samal, University of Kalyani, India
Copyright © 2018 Chamizo, Mugnai, Rossi, Certini and De Philippis. This is an open-access article distributed under the terms of the Creative Commons Attribution License (CC BY). The use, distribution or reproduction in other forums is permitted, provided the original author(s) and the copyright owner are credited and that the original publication in this journal is cited, in accordance with accepted academic practice. No use, distribution or reproduction is permitted which does not comply with these terms.
*Correspondence: Sonia Chamizo, c29uaWEuY2hhbWl6b2RlbGFwaWVkcmFAdW5pZmkuaXQ=