- 1Department of Environmental Sciences - Botany, University of Basel, Basel, Switzerland
- 2Research Institute of Organic Agriculture (FiBL), Frick, Switzerland
Legume-cereal intercropping is well known in traditional dry land agriculture. Here, we tested whether finger millet, a shallow-rooted cereal, can profit from neighboring pigeon pea, a deep-rooted legume, in the presence of “biofertilization” with arbuscular mycorrhizal fungi (AMF) and plant growth-promoting rhizobacteria (PGPR), under drought conditions. We conducted a greenhouse experiment using compartmented microcosms. Pigeon pea was grown in a deep compartment with access to a moist substrate layer at the bottom, whereas finger millet was grown in a neighboring shallow compartment, separated by 25-μm nylon mesh, without access to the moist substrate layer. In the presence of a common mycorrhizal network (CMN), with or without PGPR, a drought condition had little negative effect on the biomass production of the finger millet plant whereas in absence of biofertilization, finger millet biomass production was less than half compared to well-watered condition. Biofertilization strongly increased nitrogen and phosphorus uptake by both plants, both under well-watered and drought conditions. In the presence of AMF, both plants also acquired 15N and 33P, offered in a labeling compartment accessible to fungal hyphae but not to roots. Our results show that “biofertilization” with AMF alleviates the negative effects of drought condition on finger millet, indicating that the CMN connecting pigeon pea and finger millet exert clearly a positive influence in this simulated intercropping system.
Introduction
Soil microorganisms play a major role in plant productivity and plant health, both in natural (van der Heijden et al., 2008) and agricultural (Artursson et al., 2006) ecosystems. They may form symbiotic networks and mutualistic associations with the roots of the host plants, exerting a positive influence on plant growth, nutrient uptake, and stress resistance (Bender et al., 2016). In particular, arbuscular mycorrhizal fungi (AMF; Gianinazzi et al., 2010; Smith and Smith, 2011) and plant-growth-promoting rhizobacteria (PGPR; Lugtenberg and Kamilova, 2009) may serve as “biofertilizers” (Vessey, 2003) to improve performance and sustainability of crop production, particularly under stress conditions (Dimkpa et al., 2009; Smith et al., 2010).
Another important element of integrated soil fertility management is plant intercropping, the technique of cultivating two or more crops simultaneously in the same field (Brooker et al., 2015). In comparison to monocropping, this may enhance agricultural productivity through the more effective utilization of naturally available resources (Li et al., 2007, 2014). Thus, intercropping offers farmers the opportunity to achieve yield advantages and increased yield stability by exploiting nature's principle of diversity, even on marginal land (Brooker et al., 2015).
To improve crop productivity and soil fertility in a sustainable way, “biofertilization” and intercropping may be combined (Wezel et al., 2014). For example, it has been reported that nutrient uptake (phosphorus and nitrogen) was greatly improved by intercropping legume and cereal crops and linking them via a common mycorrhizal network (Hauggaard-Nielsen and Jensen, 2005). Specific root-microbe interactions may also affect nutrient mobilization in the rhizosphere and contribute efficiently to nutrient acquisition (Li et al., 2014).
One interesting aspect of intercropping is the potential to make use of the so-called hydraulic lift (Caldwell et al., 1998; Liste and White, 2008), also called, more precisely, hydraulic redistribution (Burgess and Bleby, 2006; Burgess, 2011): Under drought conditions, a deep-rooting plant may lift water from the moist bottom soil layers to the dry topsoil along the water potential gradient. In the top soil, intercropped shallow-rooting plants may profit from the lifted water (Prieto et al., 2012), a process that has also been called, quite fittingly, “bioirrigation” (Liste and White, 2008). We have to note that the term “bioirrigation” is frequently used by marine biologists for a process more adequately called “bioturbation,” i.e., the supply of water to deep sediment layers in waterbodies through the activity of marine animals (Kristensen et al., 2012).
In this context, AMF used as “biofertilizers” may play a fundamental role in the redistribution and use of available water. On the one hand, AMF may form a common mycorrhizal network (CMN) and a bridge between the deep-rooted and the shallow-rooted plants and thereby provide water to the latter. The potential of such a CMN for water delivery was shown in several studies (Egerton-Warburton et al., 2007; Querejeta et al., 2012), and theoretical considerations indicated that the hyphae of AMF may redistribute water at a much higher rate than commonly thought (Allen, 2007). On the other hand, the water redistributed by AMF may also be delivered to the dry soil to promote there the activities of PGPRs and help them to mobilize nutrients, a possibility that has not received much attention yet. Two recent model studies with mycelia of Agaricus bisporus and Pythium ultimum have experimentally verified the potential of fungal hyphae to move water along a water potential gradient from moist soil to dry soil, and to promote the water uptake and metabolism of bacteria in the latter (Guhr et al., 2015; Worrich et al., 2017).
Here, we use an intercropping system of deep-rooting pigeon pea and shallow-rooting finger millet to study the performance of the two plants grown side-by-side, in the presence of biofertilizers. Pigeon pea has already been documented to be capable of hydraulic lift (Sekiya and Yano, 2004; Sekiya et al., 2011), and it is frequently used for intercropping with finger millet, particularly in India (Maitra et al., 2000; Padhi et al., 2010), and with other cereals such as maize (Garland et al., 2016). We hypothesized that in this situation, the deep-rooted pigeon pea may provide an opportunity for the neighboring finger millet to utilize water from deeper soil layers. Pigeon pea (Varshney et al., 2012) and finger millet (Thilakarathna and Raizada, 2015) are two important food crops for resource-poor farms in India and have the potential to be used for intercropping and saving natural resources such as water and nutrients.
Our model experiment, with compartmented microcosms and isotope tracers (33P, 15N), clearly demonstrates a strong positive effect of “biofertilization” with AMF and PGPR on both pigeon pea and finger millet, both under well-watered and drought conditions. Furthermore, it indicates that under drought stress, the CMN helps to promote growth and nutrient uptake of finger millet plants.
Materials and Methods
Plant Materials, Biofertilizers, and Treatments
The host plants used in this experiment were pigeon pea (Cajanus cajan cv. TTB7) and finger millet (Eleusine coracana cv. GPU28) (Ankur Seeds Pvt. Ltd, Bangalore, India). Seeds of the plants were surface-sterilized using 95% ethanol (v/v) for 5 min, followed by 0.1% HgCl2 (v/v) for 3 min, and then washed extensively in water. An arbuscular mycorrhizal fungus, Rhizophagus irregularis strain BEG 75, and two PGPR strains, Pseudomonas fluorescens R62 and R81 (Mathimaran et al., 2012), were used as the “biofertilizers.” Bradyrhizobium sp. (DSMZ-5969) (= TAL1132), Leibniz Institute DSMZ-German Collection of Microorganisms and Cell Cultures, Germany), a strain well-suited to nodulate pigeon pea (Bidlack et al., 2001), was used for inoculating the pigeon pea in all treatments in order to allow symbiotic nitrogen fixation.
The experiment involved four treatments: (i) No biofertilizer (NBf); (ii) Rhizophagus irregularis (Ri); (iii) Rhizophagus irregularis plus two strains of Pseudomonas fluorescens, namely R62 and R81 (Mathimaran et al., 2012) (Ri+Pf); and (iv) the two strains of Pseudomonas fluorescens alone (Pf). Each treatment was performed under two water regimes: (i) well-watered and (ii) drought (see below). All treatments were replicated four times, resulting in 32 pots for the whole experiment, laid out in a completely randomized design.
Construction of Compartmented Microcosms
We designed a multilayered compartment system for spatially separating the deep-rooting pigeon pea in a “pigeon pea compartment” (PC) and the shallow-rooted finger millet in a “finger millet compartment” (FC), as shown in Figure 1A. In brief, we used a surface sterilized polypropylene (plastic) pot of 23 cm (h) × 12 cm (w) and filled it with six layers of substrates (SL1–SL6) of varying height (Figure 1B). Each layer consisted of one or two types of particle sizes ranging from 0.125 to 4.0 mm. The four different types of particles used were: (P1) expanded Montmorillonite clay (“terragreen”) (0.125–0.8 mm); (P2) fine sand (0.15–0.25 mm); (P3) medium sand (0.25–0.5 mm); (P4) gravel (2–4 mm) purchased from Maagtechnic AG, Dübendorf and Quartz d'Alsace S.A., France. All particles were autoclaved before filling the pots with substrate layers (SL) of the following order and height: (i) SL1–particles “P1” & “P3” (1:1 v/v), 3 cm; (ii) SL2–particle “P4” (gravel), 6 cm; (iii) SL3 – particle “P3,” 3 cm; (iv) SL4–particle “P2,” 3 cm; (v) SL5–same as SL1, 7 cm and (iv) SL6–same as SL2, 0.5 cm. Before adding SL5, the inner finger millet compartment (FC), delineated by a 25-μm nylon mesh, was prepared using recycled yogurt cups (500 g size) as a tooling-aid (Figure 1A). From one cup, the bottom-part was cut-off, leaving a conical placeholder of 10 cm height. A nylon mesh (25 μm) was tightly covered over this cup. The mesh was fixed with a ring made out of the top of a similar, second yogurt cup (approximately 3 cm in height) which was slid-up from below until it fixed the mesh. The assembly was put on top of SL4. Then, both the outer (PC) and the inner (FC) compartment were filled with the same substrate (SL5), later containing the biofertilizers as appropriate (see below). Two 50 ml falcon tubes were placed on either side of the center of the inner compartment (FC), as placeholders to allow subsequent insertion of the labeling compartment (LC). After filling, the inner cup was gently pulled out, leaving only the ring holding the nylon mesh at the top and the soil-filled mesh-bag (Figure 1A).
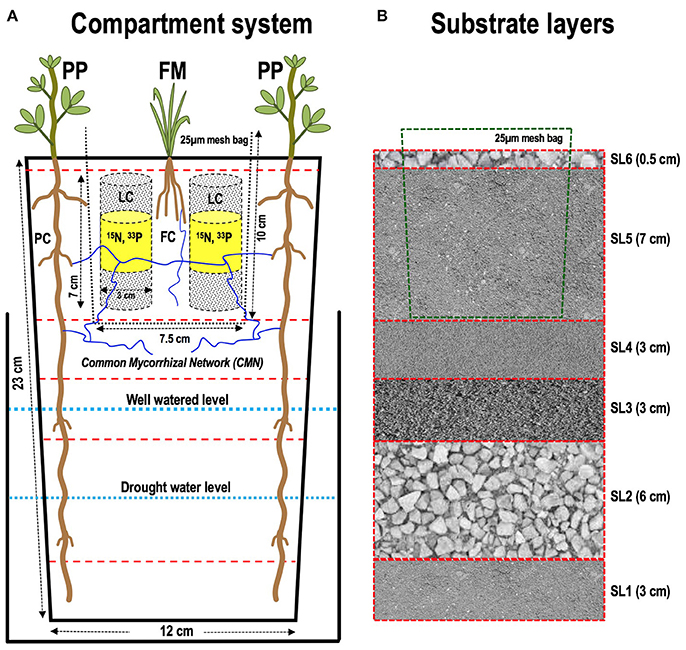
Figure 1. (A) Design of the compartmented microcosm separating the root systems of deep-rooted pigeon pea (PP) and shallow-rooted finger millet (FM). Main outer compartment (PC), harboring two PP plants, is a rectangular shaped plastic pot (12 × 12 cm) with 23 cm height. The center shallow compartment (FC) harboring one FM plant is a round shaped (7.5 cm diameter) nylon mesh (25-μm size) with 10 cm height. The FC also contains two isotope label compartments (LC) containing (15N and 33P), again delineated by a 25-μm mesh bag, (B) substrate layers allowing a separation of the moist bottom part of the microcosm from the upper soil layers. Refer to Materials and Methods section for details of the soil layer composition.
Plant Growth, Inoculation, and Environment
After filling the soil substrate layers into the above described growth-compartments (pots), all were watered from top to create a pre-wetted soil base before inserting the seedlings. The seedlings were placed into planting holes, made with a 15 ml falcon tube, one in the center of the FC (for finger millet) and two in opposite corners of the PC (for pigeon pea).
Each hole was inoculated with 5 g of AMF inoculum containing about 500 spores of Rhizophagus irregularis in vermiculite as carrier material for the “Ri” treatment, and/or 1 ml of each bacterial inoculum containing 1 × 106 cfu/ml for the “Pf” treatment. All inocula were mixed with appropriate soil substrate and added according the respective treatments into the planting holes before sowing the seeds. For controls without mycorrhizal inoculum treatments, we used 5 g carrier material (vermiculite, heated to 80°C for 1 h); for those without PGPR treatment, 1 ml of the cell-free broth of the PGPR inoculum per pot was used.
Each pot received 1 mL of a microbial wash to correct for possible differences in microbial communities (Koide and Elliott, 1989). The wash was prepared by wet sieving 50 g of AMF inoculum mix through a 32 μm sieve and a paper filter (pore size 5–7 μm, FS 14 1/2; Schleicher & Schuell BioScience GmbH), yielding a final volume of 500 ml. Three surface-sterilized pigeon pea seeds were sown separately in the planting holes of the outer compartment (PC) and 2–3 finger millet seeds were sown in the inner compartment (FC) of each pot. After their germination (8–9 days), the pigeon pea and finger millet seedlings were thinned-out, leaving one-one healthy pigeon pea seedling plant in each of two corners of the outer side compartment and one healthy finger millet seedlings in the inner compartment.
During the first 6 weeks, all microcosms were watered twice a week with sterile tap water from top. Then, for the next 8 weeks, two water regimes were generated by a dipping method. The level of water was decided based on the field capacity of the different substrate layers used in the pot. For well-watered conditions (60–80% field capacity) the pot was dipped (in a beaker) to a water height of 10.5 cm from the bottom up to midst of layer SL3, i.e., 4.5 cm below the mesh bag containing the finger millet. Under these conditions, we expected SL5 to obtain water through capillary rise in SL3 and SL4. For drought-conditions (35–40% field capacity) the pot was dipped 6 cm from the bottom up to the midst of layer SL2, i.e., 9 cm below the mesh bag (see Figure 1A). Under these conditions, SL2 with the coarse gravel should prevent capillary rise of water to layers SL3, SL4, and SL5. During watering, each pot was dipped for 3 min duration. To avoid cross-contaminations, different beakers were used for each treatment. Watering was done every third day during the experiment. Each pot received a single dose of a phosphorus-free full strength Hoagland solution (10 ml), 4 weeks after the start of the experiment. The plants were grown in growth chambers under controlled conditions with 16 h light at 25°C and 220 μE m−2 s−1, 8 h dark at 20°C, and constant relative humidity of 65%. The pot arrangement in the growth chambers was in a completely randomized order.
Isotope Labeling
Compartments for isotope labeling (“labeling compartments,” LC, Figure 1A) were constructed essentially as described (Zhu et al., 2003), using a 25 μm nylon mesh, which excluded plant roots but allowed transition of AMF and PGPR. Two empty Falcon tubes were placed initially as “space holders” in the finger millet compartment for the nutrient label. At the appropriate time (11 weeks after the start of the experiment, i.e., 3 weeks before harvest), these space holders were carefully removed from the finger millet compartment and replaced by the nylon mesh, which was wrapped around a Falcon tube of which the conical tip had been cut off. Through this open-ended tube, substrate was filled into the compartment in a “sandwich manner,” adding first 7.5 g unlabeled substrate; second, 15 g substrate labeled with 15N (25 mg 15NO3, Cambridge Isotopes Laboratories, Inc., Andover, MA, USA) and carrier free 33PO4 (400 kBq, Hartmann Analytic, Braunschweig, Germany); and third, 7.5 g unlabeled substrate (Figure 1A). The substrate was the same as used in the adjacent SL5. Finally, the open-ended falcon tube was removed from the nylon net bag, and the “labeling compartments” were gently pressed from above to ensure a good contact with the surrounding substrate. Handling of 33PO4 was done in a certified isotope laboratory at the University of Basel, and the microcosms were incubated in plant growth chamber similarly certified by the Swiss radiosafety authorities.
Harvest, Sampling, and Analysis
Shoots and roots were harvested separately. The whole soil in the pot was removed and placed individually for each compartment on a sieve with 1 mm mesh size to pick up the nodules. The roots of pigeon pea and finger millet were then washed with running tap water and separated the same way as the shoots. All of the fresh nodules (including the nodules removed from the soil) of the pigeon pea roots were counted and recorded (Table S1). The fresh shoot and root weight was determined for each pot and each compartment. Subsamples of the harvested roots were used for determining dry weight and for mycorrhizal-structure identification inside the root, after being cleared by using a 10% KOH solution and stained in Trypan Blue. For estimating the percentage of root length occupied by mycorrhizal fungi, each root sub-sample was analyzed by a modified line intersection method (McGonigle et al., 1990). For each root sample, a minimum of 50 line intersections was scored. The samples of shoot and root were dried for 24 h at 80°C, and weighed separately for measuring their biomass. Dried shoots and roots were ground to a fine powder at 30 Hz using a mixer mill (MM2224, Retsch, Haan, Germany). Nitrogen and 15N content of the plants (shoot and roots individually) was determined in subsamples of 2 mg, using a stable isotope ratio mass spectrometer (Delta V Plus, Thermo Fisher Scientific, Germany). Data are presented as δ15N (‰), compared to atmospheric nitrogen. P content of shoots and roots was measured using the molybdate blue method on a Shimadzu UV-160 spectrophotometer (Shimadzu Biotech, Duisburg, Germany) after acid digestion (Murphy and Riley, 1962). Plant 33P content was measured using a Packard 2000 liquid scintillation counter (Hewlett-Packard, Waldbronn, Germany).
Statistical Analysis
For all recorded parameters, the results are presented in the figures as mean value and standard error for each of the eight individual treatments. For all recorded parameters, a statistical analysis of variance (one-way ANOVA) was performed, considering the eight treatments as the independent factors of variation. The ANOVA was implemented in IBM SPSS Statistics version 20 and calculated separately for each plant species. A Tukey-HSD test was used to separate group mean values when the ANOVA was significant at p < 0.05.
Results
Plant Biomass
In our compartmented microcosms, the deep-rooting pigeon pea plants had access to the moist substrate layer at the bottom, throughout the experiment. Therefore, in the absence of biofertilization, pigeon pea was similarly productive, with respect to biomass, under both “well-watered” and “drought conditions” (Figure 2A). When AMF were present as biofertilizer, pigeon pea produced more than twice as much biomass under well-watered conditions and about 1.6 times more under drought conditions; the PGPR treatment “Pf” alone stimulated growth only marginally (Figure 2A). Growth promotion by AMF and PGPR was apparent in both shoots and roots, but shoot biomass responded to biofertilization more strongly (Figures S2A,B).
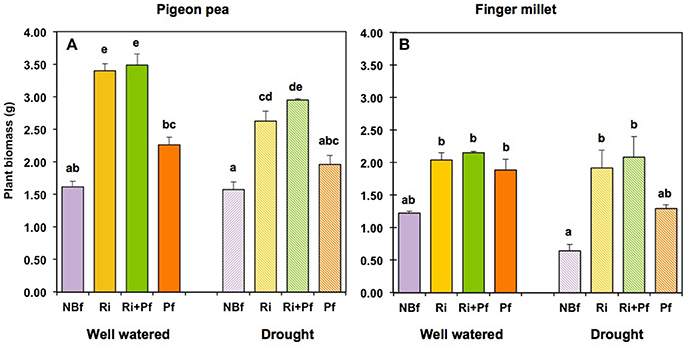
Figure 2. Total plant biomass produced in the compartmented microcosms under well-watered and drought conditions after different initial treatments with biofertilizers. The treatments are indicated as NBf, No biofertilizers; Ri, Rhizophagus irregularis; Ri+Pf, Rhizophagus irregularis + Pseudomonas fluorescens; Pf, Pseudomonas fluorescens alone. (A) Pigeon pea; (B) Finger millet. The experiment was carried out for 90 days. For the first 50 days all microcosms were well-watered from the top. Afterwards, “well-watered” and “drought” conditions were applied until harvesting, by supplying water only from the bottom. The values represent the mean ± SE of four replicates. Columns labeled with the same letter are not significantly different, according to Tukey-HSD (p < 0.05), after ANOVA.
The shallow-rooting finger millet plants, in contrast, were confined to the uppermost soil layers of the microcosms. Thus, in the absence of biofertilizers, finger millet depended on the water available in these layers. It accumulated less than half as much biomass under drought than under well-watered conditions (Figure 2B), both with respect to shoots and roots (Figures S2C,D). The presence of AMF promoted biomass accumulation under well-watered conditions (Figure 2B, Figures S2C,D). Strikingly, under drought conditions, the more than 2-fold reduction of growth was nullified in the presence of AMF, and finger millet accumulated as much biomass as under well-watered conditions (Figure 2B, Figures S2C,D). The PGPR treatment also promoted biomass accumulation, but to a smaller extent (Figure 2B, Figures S2C,D). Note that Tukey-HSD test did not separate most of the mean values reported in Figure 2B, except that the accumulation of biomass in finger millet under drought was significantly enhanced by the presence of AMF.
Mycorrhization and Nodule Formation
When an inoculum of AMF was provided, the roots of pigeon pea (Figure S1A) and finger millet (Figure S1B) became colonized from 65 to 75%, both in the presence and absence of PGPR, and both under well-watered and drought conditions. In the absence of inoculum, AMF colonization was negligible (Figure S1). Pigeon pea roots were well nodulated in the experiment, both under well-watered and drought conditions, but carried less nodules in the absence of biofertilizers than in their presence, namely ca. 20 per plant vs. ca. 50 per plant (Table S1).
Nitrogen and 15N Uptake
The relative nitrogen concentrations of the plants, expressed in mg N per g plant material, is shown in Figure 3. Pigeon pea, which was well nodulated and obtained part of the N through symbiotic nitrogen fixation, contained 16–30 mg N per g dry mass, an N concentration expected for a legume. The relative N content was lowest in the absence of biofertilizer and had higher values under conditions of biofertilization, both under well-watered and drought conditions; the highest values were reached when both AMF and PGPR were present, although most values fell into the same group according to the Tukey-HSD test (Figure 3A). Importantly, since the plant biomass was significantly higher for the pigeon pea plants with AMF (Figure 2A), the total N and P content per plant was significantly higher as well (Figures S4A,C). Similar trend was observed for finger millet as well (Figures S4B,D). For each of the four biofertilizer treatments, values were slightly lower under well-watered conditions when compared to drought conditions (Figure 3A), possibly due to reduced nitrogen fixation in moist soil. When considering shoots and roots separately, their relative nitrogen content was very similar for each of the biofertilization conditions under well-watered conditions, while the shoots contained considerably more nitrogen per g dry mass than the roots under drought conditions (Figures S3A,B).
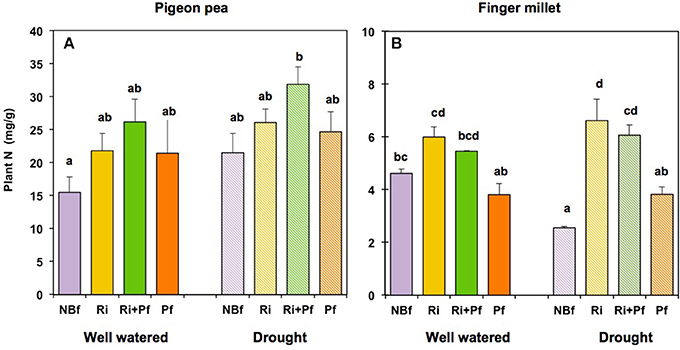
Figure 3. Relative nitrogen content (N) of pigeon pea (A) and finger millet (B) under well-watered and drought conditions after different initial treatments with biofertilizers. Biofertilizer treatments are labeled as in Figure 2. The values show the N content in mg per g dry biomass and represent the mean ± SE of four replicates. Columns labeled with the same letter are not significantly different, according to Tukey-HSD (p < 0.05), after ANOVA.
Finger millet contained 3–6.5 mg N per g dry mass, i.e., about five times less than pigeon pea (Figure 3B). Under well-watered conditions, biofertilization had no significant effect on the relative nitrogen content compared to the control, although the presence of AMF significantly increased the N content of the shoots (Figures S3C,D). Under drought conditions, in the absence of AMF, the relative N content of finger millet was significantly lower, but under drought in the presence of a AMF, it was fully restored to the level of well-watered plants under the same biofertilization conditions (Figure 3, Figures S3C,D).
For the last 3 weeks of the experiment, our microcosms contained, within the finger millet compartment, a labeling compartment containing 15NO3 (and 33P phosphate, see below), which was accessible to microbes but not to roots. To study uptake of 15N from this labeling compartment, we measured the excess of 15N in the plant material using δ15N values (Figure 4). In the absence of AMF, the δ15N value was about 1.5‰ for pigeon pea under well-watered conditions and about 0.5‰ under drought conditions (Figure 4A). These values can be considered as a baseline, due to isotopic fractionations in normal metabolism, including nitrogen fixation in the root nodules, and possibly to a minimal leakage of 15N from the labeling compartment under well-watered conditions. The presence of AMF caused a striking increase in the δ15N value of pigeon pea, both under well-watered and drought conditions (Figure 4A). This increase was also clearly noticed when shoots and roots were analyzed separately (Figures S5A,B). Thus, despite of its capacity for symbiotic nitrogen fixation, pigeon pea acquired nitrogen also through the AMF forming a CMN, over a distance of several cm, as per the situation in the microcosms (see Figure 1A).
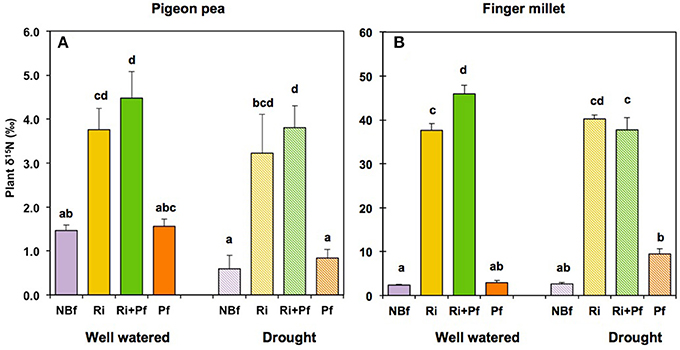
Figure 4. Accumulation of 15N from the labeling compartment in pigeon pea (A) and finger millet (B) under well-watered and drought conditions after different initial treatments with biofertilizers. Biofertilizer treatments are labeled as in Figure 2. The values show the δ15N value of the total plant N and represent the mean ± SE of four replicates. Columns labeled with the same letter are not significantly different, according to Tukey-HSD (p < 0.05), after ANOVA.
Finger millet plants had their roots in close vicinity of the labeling compartment. They showed slightly enhanced δ15N values (ca. 2.5‰) even without biofertilization, both under well-watered and drought conditions (Figure 4B); this may be explained by uptake of the highly mobile 15 from the labeling compartment. However, in presence of AMF were present, the δ15N values were at least 20-fold higher, in the entire plant (Figure 4B) as well as in shoots and roots (Figures S5C,D), and this increase was similar under well-watered as well as under drought conditions.
Phosphorus and 33P Uptake
The relative P content of the plants, expressed in mg P per g plant material, is shown in Figure 5. For pigeon pea, in the absence of biofertilization, the relative P content was extremely low (0.5–0.6 mg P per g biomass), both under well-watered and drought conditions, indicating strong P limitation in our microcosms (Figure 5A). All biofertilizer treatments caused at least a four-fold increase in the relative P content (Figure 5A). Shoots and roots participated similarly in this increase of P accumulation, although shoots had a somewhat higher relative P content than the roots (Figures S6A,B).
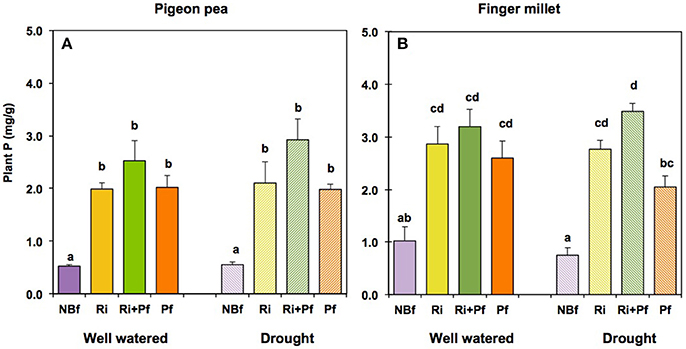
Figure 5. Relative phosphorus content (P) of pigeon pea (A) and finger millet (B) under well-watered and drought conditions after different initial treatments with biofertilizers. Biofertilizer treatments are labeled as in Figure 2. The values show the P content in mg per g dry biomass and represent the mean ± SE of four replicates. Columns labeled with the same letter are not significantly different, according to Tukey-HSD (p < 0.05), after ANOVA.
In the absence of biofertilization, finger millet showed similarly low relative P content (0.8–1 mg P per g biomass), both under well-watered and drought conditions (Figure 5B). Under well-watered conditions, all biofertilizer treatments, even PGPR alone, caused an increase in relative P content by a factor of at least 2.5, to ca. 2.8 mg P per g biomass (Figure 5B). Under drought conditions, PGPR alone were somewhat less effective, causing an increase to 2 mg P per g biomass; the treatment with AMF alone resulted in a P level of 2.8 mg P per g biomass, and the combined AMF + PGPR even of 3.5 mg P per g biomass (Figure 5B). As for pigeon pea, shoots and roots participated similarly in this increase of P accumulation, although shoots had a somewhat higher relative P content than the roots (Figures S6C,D).
As mentioned above, the “labeling compartment,” located within the finger millet compartment but accessible only to microorganisms, not only contained 15N but also 33P phosphate. To study uptake of 33P from the labeling compartment, we measured radioactivity in the plant tissues, expressed in kBq per g biomass (Figure 6). In the absence of biofertilizers, pigeon pea did not accumulate any 33P, both under well-watered and drought conditions (Figure 6A). This was expected because of the low mobility of phosphate in the soil. In the presence of AMF, however, pigeon pea accumulated high levels of 33P, obviously delivered by the CMN; the levels attained were similar under drought as under well-watered conditions (Figure 6A).
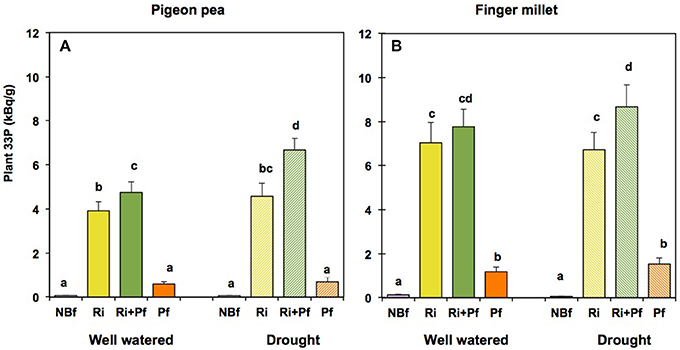
Figure 6. Accumulation of 33P from the labeling compartment in pigeon pea (A) and finger millet (B) under well-watered and drought conditions after different initial treatments with biofertilizers. Biofertilizer treatments are labeled as in Figure 2 The values show the 33P content in kBq per g dry biomass and represent the mean ± SE of four replicates. Columns labeled with the same letter are not significantly different, according to Tukey-HSD (p < 0.05), after ANOVA.
Finger millet, as mentioned previously, had its roots in close vicinity of the labeling compartment. Nevertheless, in the absence of biofertilizers, finger millet barely took up any 33P, neither under well-watered nor under drought conditions (Figure 6B). Application of PGPR caused a moderate accumulation of 33P, both under well-watered and drought conditions; however, this effect was dwarfed by the very strong accumulation of 33P in the presence of AMF (Figure 6B). When comparing shoots and roots of finger millet, the roots contained three to four times as much 33P than the shoots (Figures S7C,D), possibly because part of the 33P was actually still present in fungal tissue.
Discussion
Our model microcosms (Figure 1) were designed to test, in a simple experimental system, the potential of pigeon pea plants to access water in deeper soil layers whilst a neighboring shallow rooted Finger millet plant is suffering from drought. It was also designed in a way to investigate the effects created by biofertilizers (AMF and PGPR's) especially under drought conditions, in terms of plant growth, nutrient uptake and nutrient transfer from a dedicated soil space, inaccessible by the plant roots but by AMF. These microcosms were conceptually similar to the ones devised by others (Murphy and Riley, 1962; McGonigle et al., 1990; Sekiya et al., 2011), but we included a 25-μm nylon mesh between the two plants in order to keep the root systems separated. This nylon mesh could be freely crossed by the hyphae of mycorrhizal fungi and therefore allowed the establishment of a “common mycorrhizal network” between pigeon pea and finger millet, as in our previous experiments (Walder and Niemann, 2012). The soil layers in the microcosms were carefully chosen to control two water regimes (well-watered and drought) by adding the water from the bottom. We included a large coarse gravel layer (SL2 in Figure 1) to prevent capillary rise of water from the lowermost soil layer (SL1) to the upper soil layers (SL3–SL6). Thus, under conditions of drought, when water was supplied from the bottom only up to SL2 the upper soil layers remained dry. Similarly under well-watered conditions, when water was supplied from the bottom up to SL3, all upper soil layers received humidity by capillary rise. (Note that a homogeneous soil structure in the entire pot, which might resemble more natural condition, would have created a gradually decreasing humidity from bottom to top which is much more difficult to control). We established four biofertilization regimes and exposed the microcosms either to normal or to a restricted water supply, which left the medium and fine sand layers (SL3–SL4) either under well-watered or drought conditions.
As expected, and observed in several other studies, the plants grew much better in the presence of biofertilizers, particularly with AMF, than in their absence (Smith and Smith, 2011). Without biofertilizers, the pigeon pea plants performed similarly under well-watered and under drought conditions. Obviously, their roots went deep enough to tap water from the moist bottom soil layer and to lift it to the shoot. Finger millet grew only half as well under drought conditions, in the absence of biofertilization. Thus, they could not profit appreciably from the adjacent pigeon pea under these conditions. However, in the presence of AMF, finger millet growth was similar under drought and well-watered conditions, indicating that in this case the plants had access to sufficient water, even under drought. This result could also mean that AMF inoculated finger millet plants had a better nutrient and water status than non-mycorrhizal plants, and thus better growth conditions (Marulanda et al., 2003; Wu et al., 2008; Lehto and Zwiazek, 2011).
As the finger millet roots were confined within the 25-μm mesh bag, the CMN must have been the source for the provided water. The CMN could easily cross through the mesh bag and deliver water either directly from the wet soil layer (SL1) or from the root-space of the surrounding pigeon pea plants. The exact mechanism of this water redistribution by fungi is unclear; it could be symplastic (within the fungal hyphae) or apoplastic (in the cell walls of living or dead fungal hyphae), where it may move passively by mass-flow along the water potential gradient on the outside of hyphae (Allen, 2007). In this context, it is interesting that fungal hyphae not only can move water along a water potential gradient from moist soil to dry soil, but also can deliver the water to adjacent bacteria and support their functions (Worrich et al., 2017).
Our experiment also highlights the well-known importance of the CMN for mineral nutrient acquisition, particularly of N and P (Smith and Smith, 2011). Clearly, 33P and 15N were moved from the “labeling compartment,” located within the finger millet compartment, by the hyphae of the CMN to both plant species. One interesting aspect of our experiment was the finding that PGPR alone (treatment Pf) was able to increase the P concentration and the 33P-uptake in finger millet, even under drought conditions (Figure 6). The (relatively small) increase in P concentration may be explained by the well-known potential of PGPR to solubilize inaccessible P sources in the soil (Vessey, 2003; Lugtenberg and Kamilova, 2009). The enhancement of 33P-uptake may be due to the fact that the labeling compartment is in very close vicinity to the finger millet compartment; the PGPR (which may pass the nylon net surrounding the labeling compartment) may carry 33P-phosphate to the adjacent plant root.
The potential of the CMN to allocate N (Hauggaard-Nielsen and Jensen, 2005) and P (Mikkelsen et al., 2008) differentially to the interlinked plants has well been documented, as for example in earlier work with Sorghum bicolor and Linum usitatissimum, trading for these nutrients with the CMN at widely differing terms (Walder and Niemann, 2012). The transport of water from roots to soil (oppositely to nutrient flow) would occur only during nighttime when hydraulic lift takes place, during the day, water and nutrient flow occur in the same direction, from soil to roots.
In our experiments, we cannot clearly distinguish the relative importance of AMF and their CMN for hydraulic redistribution of water versus direct or indirect mobilization of nutrients as these processes are closely interdependent. However, we can firmly conclude that AMF and their CNM, in the setting of intercropping, strongly facilitate the growth of finger millet plants under conditions of drought, either directly by delivering water—be it through hyphae reaching the moist bottom layer or, more likely, by transfer of the hydraulically lifted water from the deep-rooting pigeon pea to the shallow-rooting finger millet, or indirectly, for example by increasing the nutrient-mobilizing capacities of PGPR. In either case, the CMN of AMF is of central importance for finger millet to overcome the drought stress artificially imposed by the confinement of the root system by the 25-μm mesh bag.
The combination of intercropping, biofertilization and bioirrigation may help to alleviate one of the major problems of agriculture in arid regions, the scarcity of water (Mekonnen and Hoekstra, 2016). In a sagebrush-steppe, a natural arid ecosystem, the potential of “bioirrigation” by deep-rooting plants to activate microbial communities in the dry surface soil layers has recently been demonstrated (Cardon et al., 2013). A second “green revolution” should take this into account, and re-consider traditional agricultural systems in arid regions, where intercropping between deep-rooting and shallow-rooting plants were practiced successfully and sustainably (Scherr and McNeely, 2008; Den Herder et al., 2010; Brooker et al., 2015; Bender et al., 2016). As aptly stated in an earlier review the mycorrhizal fungi may act as “highways for water and nutrients in arid soils” (Allen, 2007). We propose, as a plausible hypothesis, that the water delivered by the AMF may act directly as a sort of “bioirrigation” to the finger millet, or that it may allow to mobilize otherwise inaccessible nutrient sources such as P directly or indirectly by facilitating the activities of the PGPR.
Conclusion
Our microcosm experiment provides a proof of concept for the efficacy of “biofertilization” and “bioirrigation” in combination with intercropping of pigeon pea and finger millet, as schematically represented in Figure 7. Under drought conditions in the topsoil, the shallow-rooted finger millet may profit by “bioirrigation” from an adjacent deep-rooted pigeon pea, which has access to a bottom wet soil layer, so that it grows better and takes up more mineral nutrients. However, this occurs only in the presence of “biofertilization” in our model system, most likely because hyphae of AMF hydraulically redistribute the water from pigeon pea to finger millet, and perhaps also because they irrigate the hyphosphere and deliver water to PGPR (Figure 7B). We now plan to examine the potential of combining “biofertilization” and “bioirrigation” in pigeon pea-finger millet intercropping under more natural greenhouse and field conditions. Although this is a challenge because of confounding factors such as less rigorously controlled water regimes and soil structures, particularly in the field, and because the partner plants selected for intercropping as well as the biofertilizers may require careful selection depending on climatic factors, soil conditions and the autochthonous microbiome, we are convinced that the concept presented here will ultimately be applicable to intercropping in rainfed dryland agriculture in various regions of the world.
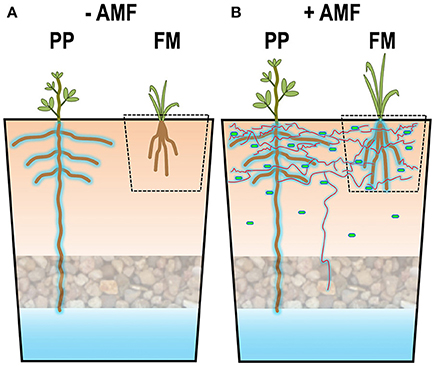
Figure 7. Graphic summary of our model experiment to examine the potential of bioirrigation in combination with biofertilization: (A) Under conditions of drought, deep-rooting pigeon pea (PP) hydraulically lifts water from the wet bottom soil layer to the dry top soil layer, but in the absence of arbuscular mycorrhizal fungi (AMF), finger millet (FM) does not profit from this water. (B) In the presence of AMF (red lines), their hyphae hydraulically re-distribute the water lifted by pigeon pea to finger millet and to PGPRs (green dots) in the hyphosphere.
Author Contributions
KS, NM, TB, AW, and LS designed the study. KS, NM, and LS contributed to the experiments. KS, LS, and NM performed the data analysis. AK provided facilities to analyze phosphorus and nitrogen. TB, AW, AK, and NM provided guidance throughout the study. KS, TB, and NM wrote and revised the manuscript.
Conflict of Interest Statement
The authors declare that the research was conducted in the absence of any commercial or financial relationships that could be construed as a potential conflict of interest.
Acknowledgments
This work was supported by grants from Plant Fellows (to KS), the Indo-Swiss Collaboration of Biotechnology, ISCB (to TB) and the IDP BRIDGES program (to TB).
Supplementary Material
The Supplementary Material for this article can be found online at: https://www.frontiersin.org/articles/10.3389/fenvs.2018.00046/full#supplementary-material
Figure S1. Colonization of the roots of pigeon pea (A) and finger millet (B) by arbuscular mycorrhizal fungi under well-watered and drought conditions after different initial treatments with biofertilizers, expressed as a percentage of root length. Columns labeled with the same letter are not significantly different, according to Tukey-HSD (p < 0.05), after ANOVA.
Figure S2. Plant biomass of pigeon pea (A,B) and finger millet (C,D) under well-watered and drought conditions after different initial treatments with biofertilizer, separated into shoot (A,C) and root (B,D) biomass. For details, see Figure 2. Columns labeled with the same letter are not significantly different, according to Tukey-HSD (p < 0.05), after ANOVA.
Figure S3. Relative nitrogen content (N) of pigeon pea (A,B) and finger millet (C,D) under well-watered and drought conditions after different initial treatments with biofertilizer, separated into shoot (A,C) and root (B,D). For details, see Figure 3. Columns labeled with the same letter are not significantly different, according to Tukey-HSD (p < 0.05), after ANOVA.
Figure S4. Nitrogen content (N) and phosphorus (P) content per plant of pigeon pea (A,C) and finger millet (B,D) under well-watered and drought conditions after different initial treatments with biofertilizers. Biofertilizer treatments are labeled as in Figure 2. The values show the N and P content in mg per plant dry biomass and represent the mean ± SE of four replicates. Columns labeled with the same letter are not significantly different, according to Tukey-HSD (p < 0.05), after ANOVA Total N & P content mg/plant.
Figure S5. Accumulation of 15N from the labeling compartment in pigeon pea (A,B) and finger millet (C,D) under well-watered and drought conditions after different initial treatments with biofertilizers, separated into shoot (A,C) and root (B,D). For details, see Figure 5. Columns labeled with the same letter are not significantly different, according to Tukey-HSD (p < 0.05), after ANOVA.
Figure S6. Relative phosphorus content (P) of pigeon pea (A,B) and finger millet (C,D) under well-watered and drought conditions after different initial treatments with biofertilizers, separated into shoot (A,C) and root (B,D). For details, see Figure 6. Columns labeled with the same letter are not significantly different, according to Tukey-HSD (p < 0.05), after ANOVA.
Figure S7. Accumulation of 33P from the labeling compartment in pigeon pea (A,B) and finger millet (C,D) under well-watered and drought conditions after different initial treatments with biofertilizers, separated into shoot (A,C) and root (B,D). For details, Figure 7. Columns labeled with the same letter are not significantly different, according to Tukey-HSD (p < 0.05), after ANOVA.
Table S1. Number of nodules of pigeon pea under well-watered and drought conditions after different initial treatments with biofertilizers. *Means ± SE. Means followed by the same letter in a column are not significantly different from each other according to Tukey-HSD (p < 0.05), after ANOVA. NBf, No biofertilizers; Ri, Rhizophagus irregularis; Ri+Pf, Rhizophagus irregularis + Pseudomonas fluorescens; Pf, Pseudomonas fluorescens alone.
References
Allen, M. F. (2007). Mycorrhizal fungi: Highways for water and nutrients in arid soils. Vadose Zone J. 6, 291–297. doi: 10.2136/vzj2006.0068
Artursson, V., Finlay, R. D., and Jansson, J. K. (2006). Interactions between arbuscular mycorrhizal fungi and bacteria and their potential for stimulating plant growth. Environ. Microbiol. 8, 1–10. doi: 10.1111/j.1462-2920.2005.00942.x
Bender, S. F., Wagg, C., and van der Heijden, M. G. (2016). An underground revolution: biodiversity and soil ecological engineering for agricultural sustainability. Trends Ecol. Evol. 31, 440–452. doi: 10.1016/j.tree.2016.02.016
Bidlack, J. E., Rao, S. C., and Demezas, D. H. (2001). Nodulation, nitrogenase activity, and dry weight of chickpea and pigeon pea cultivars using different Bradyrhizobium strains. J. Plant Nutr. 24, 549–560. doi: 10.1081/PLN-100104979
Brooker, R. W., Bennett, A. E., Cong, W. F., Daniell, T. J., George, T. S., Hallett, P. D., et al. (2015). Improving intercropping: a synthesis of research in agronomy, plant physiology and ecology. New Phytol. 206, 107–117. doi: 10.1111/nph.13132
Burgess, S. S. O. (2011). Can hydraulic redistribution put bread on our table? Plant Soil 341, 25–29. doi: 10.1007/s11104-010-0638-1
Burgess, S. S., and Bleby, T. M. (2006). Redistribution of soil water by lateral roots mediated by stem tissues. J. Exp. Bot. 57, 3283–3291. doi: 10.1093/jxb/erl085
Caldwell, M. M., Dawson, T. E., and Richards, J. H. (1998). Hydraulic lift: consequences of water efflux from the roots of plants. Oecologia 113, 151–161.
Cardon, Z. G., Stark, J. M., Herron, P. M., and Rasmussen, J. A. (2013). Sagebrush carrying out hydraulic lift enhances surface soil nitrogen cycling and nitrogen uptake into inflorescences. Proc. Natl. Acad. Sci. U.S.A. 110, 18988–18993. doi: 10.1073/pnas.1311314110
Den Herder, G., Van Isterdael, G., Beeckman, T., and De Smet, I. (2010). The roots of a new green revolution. Trends Plant Sci. 15, 600–607. doi: 10.1016/j.tplants.2010.08.009
Dimkpa, C., Weinand, T., and Asch, F. (2009). Plant-rhizobacteria interactions alleviate abiotic stress conditions. Plant Cell Environ. 32, 1682–1694. doi: 10.1111/j.1365-3040.2009.02028.x
Egerton-Warburton, L. M., Querejeta, J. I., and Allen, M. F. (2007). Common mycorrhizal networks provide a potential pathway for the transfer of hydraulically lifted water between plants. J. Exp. Bot. 58, 1473–1483. doi: 10.1093/jxb/erm009
Garland, G., Bünemann, E. K., Oberson, A., Frossard, E., and Six, J. (2016). Plant-mediated rhizospheric interactions in maize-pigeon pea intercropping enhance soil aggregation and organic phosphorus storage. Plant Soil 415, 37–55. doi: 10.1007/s11104-016-3145-1
Gianinazzi, S., Gollotte, A., Binet, M. N., van Tuinen, D., Redecker, D., and Wipf, D. (2010). Agroecology: the key role of arbuscular mycorrhizas in ecosystem services. Mycorrhiza 20, 519–530. doi: 10.1007/s00572-010-0333-3
Guhr, A., Borken, W., Spohn, M., and Matzner, E. (2015). Redistribution of soil water by a saprotrophic fungus enhances carbon mineralization. Proc. Natl. Acad. Sci. U.S.A. 112, 14647–14651. doi: 10.1073/pnas.1514435112
Hauggaard-Nielsen, H., and Jensen, E. S. (2005). Facilitative root interactions in intercrops. Plant Soil 274, 237–250. doi: 10.1007/1-4020-4099-7_13
Koide, R., and Elliott, G. (1989). Cost, benefit and efficiency of the vesicular-arbuscular mycorrhizal symbiosis. Functional Ecol. 3, 252–255.
Kristensen, E., Penha-Lopes, G., Delefosse, M., Valdemarsen, T., Quintana, C. O., and Banta, G. T. (2012). What is bioturbation? The need for a precise definition for fauna in aquatic sciences. Mar. Ecol. Prog. Ser. 446, 285–302. doi: 10.3354/meps09506
Lehto, T., and Zwiazek, J. J. (2011). Ectomycorrhizas and water relations of trees: a review. Mycorrhiza 21, 71–90. doi: 10.1007/s00572-010-0348-9
Li, L., Li, S. M., Sun, J. H., Zhou, L. L., Bao, X. G., Zhang, H. G., et al. (2007). Diversity enhances agricultural productivity via rhizosphere phosphorus facilitation on phosphorus-deficient soils. Proc. Natl. Acad. Sci. U.S.A. 104, 11192–11196. doi: 10.1073/pnas.0704591104
Li, L., Tilman, D., Lambers, H., and Zhang, F. S. (2014). Plant diversity and overyielding: insights from belowground facilitation of intercropping in agriculture. New Phytol. 203, 63–69. doi: 10.1111/nph.12778
Liste, H. H., and White, J. C. (2008). Plant hydraulic lift of soil water - implications for crop production and land restoration. Plant and Soil 313, 1–17. doi: 10.1007/s11104-008-9696-z
Lugtenberg, B., and Kamilova, F. (2009). Plant-growth-promoting rhizobacteria. Annu. Rev. Microbiol. 63, 541–556. doi: 10.1146/annurev.micro.62.081307.162918
Maitra, S., Ghosh, D. C., Sounda, G., Jana, P. K., and Roy, D. K. (2000). Productivity, competition and economics of intercropping legumes in finger millet (Eleusine coracana) at different fertility levels. Indian J. Agric. Sci. 70, 824–828.
Marulanda, A., Azcon, R., and Ruiz-Lozano, J. M. (2003). Contribution of six arbuscular mycorrhizal fungal isolates to water uptake by Lactuca sativa plants under drought stress. Physiol. Plantarum. 119, 526–533. doi: 10.1046/j.1399-3054.2003.00196.x
Mathimaran, N., Srivastava, R., Wiemken, A., Sharma, A. K., and Boller, T. (2012). Genome sequences of two plant growth-promoting fluorescent Pseudomonas strains, R62 and R81. J. Bacteriol. 194, 3272–3273. doi: 10.1128/JB.00349-12
McGonigle, T. P., Miller, M. H., Evans, D. G., Fairchild, G. L., and Swan, J. A. (1990). A new method which gives an objective measure of colonization of roots by vesicular arbuscular fungi. New Phytol. 115, 495–501. doi: 10.1111/j.1469-8137.1990.tb00476.x
Mekonnen, M. M., and Hoekstra, A. Y. (2016). Four billion people facing severe water scarcity. Sci. Adv. 2:e1500323. doi: 10.1126/sciadv.1500323
Mikkelsen, B. L., Rosendahl, S., and Jakobsen, I. (2008). Underground resource allocation between individual networks of mycorrhizal fungi. New Phytol. 180, 890–898. doi: 10.1111/j.1469-8137.2008.02623.x
Murphy, J., and Riley, J. P. (1962). A modified single solution method for determination of phosphate in natural waters. Anal. Chim. Acta 26, 31–36. doi: 10.1016/S0003-2670(00)88444-5
Padhi, A. K., Panigrahi, R. K., and Jena, B. K. (2010). Effect of planting geometry and duration of intercrops on performance of pigeonpea-finger millet intercropping systems. Indian J. Agric. Res. 44, 43–47.
Prieto, I., Armas, C., and Pugnaire, F. I. (2012). Water release through plant roots: new insights into its consequences at the plant and ecosystem level. New Phytol. 193, 830–841. doi: 10.1111/j.1469-8137.2011.04039.x
Querejeta, I. J., Egerton-Warburton, L. M., Prieto, I., Vargas, R., and Allen, M. F. (2012). Changes in soil hyphal abundance and viability can alter the patterns of hydraulic redistribution by plant roots. Plant Soil 355, 63–73. doi: 10.1007/s11104-011-1080-8
Scherr, S. J., and McNeely, J. A. (2008). Biodiversity conservation and agricultural sustainability: towards a new paradigm of ’ecoagriculture’ landscapes. Philos. Trans. R. Soc. Lond. B Biol Sci. 363, 477–494. doi: 10.1098/rstb.2007.2165
Sekiya, N., and Yano, K. (2004). Do pigeon pea and sesbania supply groundwater to intercropped maize through hydraulic lift? Hydrogen stable isotope investigation of xylem waters. Field Crops Res. 86, 167–173. doi: 10.1016/j.fcr.2003.08.007
Sekiya, N., Araki, H., and Yano, K. (2011). Applying hydraulic lift in an agroecosystem: forage plants with shoots removed supply water to neighboring vegetable crops. Plant Soil. 341, 39–50. doi: 10.1007/s11104-010-0581-1
Smith, S. E., and Smith, F. A. (2011). Roles of arbuscular mycorrhizas in plant nutrition and growth: new paradigms from cellular to ecosystem scales. Annu. Rev. Plant Biol. 62, 227–250. doi: 10.1146/annurev-arplant-042110-103846
Smith, S. E., Facelli, E., Pope, S., and Andrew Smith, F. (2010). Plant performance in stressful environments: interpreting new and established knowledge of the roles of arbuscular mycorrhizas. Plant Soil. 326, 3–20. doi: 10.1007/s11104-009-9981-5
Thilakarathna, M., and Raizada, M. (2015). A review of nutrient management studies involving finger millet in the semi-arid tropics of Asia and Africa. Agronomy 5, 262–290. doi: 10.3390/agronomy5030262
van der Heijden, M. G., Bardgett, R. D., and van Straalen, N. M. (2008). The unseen majority: soil microbes as drivers of plant diversity and productivity in terrestrial ecosystems. Ecol. Lett. 11, 296–310. doi: 10.1111/j.1461-0248.2007.01139.x
Varshney, R. K., Chen, W., Li, Y., Bharti, A. K., Saxena, R. K., Schlueter, J. A., et al. (2012). Draft genome sequence of pigeonpea (Cajanus cajan), an orphan legume crop of resource-poor farmers. Nat. Biotechnol. 30, 83–89. doi: 10.1038/nbt.2022
Vessey, J. K. (2003). Plant growth promoting rhizobacteria as biofertilizers. Plant Soil 255, 571–586. doi: 10.1023/A:1026037216893
Walder, F., and Niemann, H. (2012). Mycorrhizal networks: common goods of plants shared under unequal terms of trade. Plant Physiol. 159, 789–797. doi: 10.1104/pp.112.195727
Wezel, A., Casagrande, M., Celette, F., Vian, J.-F., and Ferrer, A. (2014). Agroecological practices for sustainable agriculture. a review. Agron. Sustain. Dev. 34, 1–20. doi: 10.1007/s13593-013-0180-7
Worrich, A., Stryhanyuk, H., Musat, N., Konig, S., Banitz, T., Centler, F., et al. (2017). Mycelium-mediated transfer of water and nutrients stimulates bacterial activity in dry and oligotrophic environments. Nat. Commun. 8:15472. doi: 10.1038/ncomms15472
Wu, Q. S., Xia, R. X., and Zou, Y. N. (2008). Improved soil structure and citrus growth after inoculation with three arbuscular mycorrhizal fungi under drought stress. Eur. J. Soil Biol. 44, 122–128. doi: 10.1016/j.ejsobi.2007.10.001
Keywords: biofertilizer, biorrigation, facilitation, intercropping, pigeon pea (Cajanus cajan), finger millet (Eleusine coracana), arbuscular mycorrhizal fungi (AMF), plant growth promoting rhizobacteria (PGPR)
Citation: Saharan K, Schütz L, Kahmen A, Wiemken A, Boller T and Mathimaran N (2018) Finger Millet Growth and Nutrient Uptake Is Improved in Intercropping With Pigeon Pea Through “Biofertilization” and “Bioirrigation” Mediated by Arbuscular Mycorrhizal Fungi and Plant Growth Promoting Rhizobacteria. Front. Environ. Sci. 6:46. doi: 10.3389/fenvs.2018.00046
Received: 06 February 2018; Accepted: 22 May 2018;
Published: 11 June 2018.
Edited by:
Urs Feller, Universität Bern, SwitzerlandReviewed by:
Radha Prasanna, Indian Agricultural Research Institute (IARI), IndiaChristel Baum, University of Rostock, Germany
Copyright © 2018 Saharan, Schütz, Kahmen, Wiemken, Boller and Mathimaran. This is an open-access article distributed under the terms of the Creative Commons Attribution License (CC BY). The use, distribution or reproduction in other forums is permitted, provided the original author(s) and the copyright owner are credited and that the original publication in this journal is cited, in accordance with accepted academic practice. No use, distribution or reproduction is permitted which does not comply with these terms.
*Correspondence: Natarajan Mathimaran, bWF0aGltYXJhbi5uYXRhcmFqYW5AdW5pYmFzLmNo