- 1Department of Civil and Mechanical Engineering, University of Cassino and Southern Lazio, Cassino, Italy
- 2Laboratoire de Biotechnologie de l'Environnement, INRA, Montpellier SupAgro, Narbonne, France
- 3TRIFYL, Labessiere-Candeil, France
- 4Department of Mathematics and Applications Renato Caccioppoli, University of Naples Federico II, Naples, Italy
- 5UNESCO-IHE Institute for Water Education, Delft, Netherlands
The co-production of biohydrogen and methane from the organic fraction of municipal solid waste was investigated using a two-stage AD system, composed of a pilot scale dark fermenter (DF) and a continuous methanogenic biofilm reactor. From the DF process, a biohydrogen yield of 41.7 (± 2.3) ml H2/gVSadded was achieved. The liquid DF effluent (DFE) was rich in short chain volatile fatty acids, i.e., mainly acetic and butyric acid. The DFE was valorized by producing methane in the methanogenic biofilm reactor. Two methanogenic biofilm reactors were used to assess the biotic and abiotic role of the DFE on the performance of the reactors. Regardless of the different DFE feeding (i.e., biotic and abiotic), similar and stable operational performance of the two methanogenic biofilm reactors were observed with a respective methane yield and COD removal efficiency of 280–300 ml CH4/gCODremoved and 80–90%. Both methanogenic biofilm reactors showed significant resistance toward organic shock loads and recovered fast after reactor disturbance. The total estimated energy recovered in the form of hydrogen and methane gas was, respectively, 28 and 72%, of the initial COD.
HIGHLIGHTS
• Simultaneous production of biohydrogen and methane from OFMSW was investigated.
• A pilot scale dark fermenter and methanogenic biofilm reactor were used for, respectively, biohydrogen and methane production.
• The biotic and abiotic role of the dark fermentation effluent on the methanogenic biofilm reactor was assessed.
• Anaerobic biofilm reactors demonstrated a high tolerance toward an increased OLR.
• H2 and CH4 was 28 and 72%, respectively, of the total energy recovery from the OFMSW.
Introduction
The global increase in fuel prices coupled to the depletion of fossil fuel reserves are creating an energy crisis, which is one of the biggest challenges of the 21st century (Raheem et al., 2016). It is, therefore, necessary to develop alternative energy generation routes (Dareioti and Kornaros, 2015). Owing to its clean production process and being a renewable energy source, biological hydrogen production via dark fermentation processes (DF) has recently gained significant attention (Ghimire et al., 2015a). The DF process allows the use of a broad spectrum of organic wastes, both liquid and solid waste streams, which greatly increases the economics of the process (Guo et al., 2010). Despite its benefits, progress toward a biohydrogen economy using the DF process is hindered by several factors, such as low substrate conversion efficiency and hydrogen yield (Bonk et al., 2015; Ghimire et al., 2015a).
As an alternative strategy to exploit most of the energy content of organic substrates, coupling the DF process (producing biohydrogen) with the anaerobic digestion (AD) process (producing methane) has recently received a renewed attention by many researchers (Cavinato et al., 2012; Monlau et al., 2015; Yeshanew et al., 2016c). Such a coupled system creates a wider scope in developing a sustainable approach by producing a highly valued gaseous fuel: biohythane (Capson-Tojo et al., 2016; Cavinato et al., 2016). Biohythane, a mixture of H2 and CH4, is a promising renewable energy carrier (Yeshanew et al., 2016c) which has higher flame speed, heat efficiency and easy ignition temperature, in addition to having a more stable combustion efficiency in internal combustion engines compared to solely natural gas (Liu et al., 2013). This mixed gas is commercially applied as vehicle fuel in some countries, such as USA and India (Cavinato et al., 2016), and is receiving popularity in the transportation sector (Liu et al., 2013). The production of biohythane is often carried out via a two-stage AD reactor configuration (Monlau et al., 2015), where the faster growing hydrogen producing microorganisms develop in the first stage, and the slow growing acetogenic and methanogenic microbial groups develop in the second stage (Dareioti and Kornaros, 2015). The synergy of the integrated process relies on the maximum utilization of the organic substrate, and hence improved biofuel generation, for a better economic evaluation of the AD process (Monlau et al., 2015).
Studies of the DF process have been mostly conducted at a laboratory scale in batch, semi-continuous, or continuous reactors (Ghimire et al., 2015a). Limited data exist that show pilot and/or industrial scale experiences. Hence, further research on pilot-scale operation is important to expand the process for commercial purposes. For the second methane production stage, the use of biofilm-based reactors has been recommended by several authors (Chu et al., 2008; Yeshanew et al., 2016c), owing to the slow growth rate of methanogenic archaea, their sensitivity toward operational conditions and their vulnerability for washout from the AD reactors. Anaerobic biofilm reactors have shown excellent performances and are robust processes by retaining the slow growing anaerobic biomass through their immobilization on an inert solid carrier material with a large surface area (Van Lier et al., 2015; Yeshanew et al., 2016b). Consequently, this reactor configuration has been widely applied to treat various types of industrial and municipal wastewaters during the last two decades (Karadag et al., 2015; Van Lier et al., 2015; Carrillo-Reyes et al., 2016).
The increased generation of municipal solid waste (MSW) is a natural consequence of population growth, urbanization, and industrialization (Bonk et al., 2015). Nearly 60% of the MSW comprises of the organic fraction (OFMSW), mainly constituted of kitchen waste, urban greening waste and waste paper (Dong et al., 2009). The associated environmental pollution is a serious global concern current societies face (Liu et al., 2006; Bonk et al., 2015). Accordingly, proper waste management is crucial to minimize further environmental degradation and making a sustainable society (Dong et al., 2009). Considering this aspect, the utilization of OFMSW through an integrated DF and AD system represents several benefits that combine waste minimization, energy recovery, and valorization. Moreover, due to the abundant nature of the waste with its high biodegradability and carbohydrate content, scaled-up applications of such an integrated process could be sustained (Ghimire et al., 2015a; Cavinato et al., 2016).
During the DF process, biohydrogen is liberated as a gaseous product along with the production of a liquid effluent: the dark fermentation effluent (DFE). The DFE is characterized by a high soluble organic matter content, consisting of mainly short chain volatile fatty acids (VFAs) and alcohols (Guo et al., 2014). Hence, it can serve as a preferred substrate for the subsequent AD process (Yeshanew et al., 2016c). Recently, several studies have exploited the potential of DFE for several applications, such as production of electricity via microbial fuel cells (Lee et al., 2014), biohydrogen via photofermentation (Ghimire et al., 2015b; Luongo et al., 2016), lipids via growth of heterotrophic microalgae (Turon et al., 2015), methane gas via AD (Yeshanew et al., 2016c), and value added products like biopolymers (Ghimire et al., 2015a). Therefore, the concept of hydrogen production using the DF process can be extended to a biorefinery context, where in addition to hydrogen, different other products of commercial interest can be simultaneously produced (Guo et al., 2010). The cited studies highlighted the suitability of the DFE for its direct application in an anaerobic reactor without further pretreatment compared to the other applications, making the process very cost effective (Lee et al., 2014). The overall metabolic rate and operational stability of the second (methane production) stage depends on the VFAs composition of the DFE (Nathoa et al., 2014).
Apart from soluble metabolites, the DFE may also contain a significant concentration of anaerobic biomass (Zahedi et al., 2016). In particular, when the DF process is carried out using mixed cultures, the microbial community in the DFE is diverse and often dominated by Clostridia species (Guo et al., 2014). The presence of these fermentative bacterial groups in the DFE may influence the downstream methanogenic process. It is, therefore, important to investigate the biotic (in the presence of fermentative bacteria) and abiotic (in the absence of fermentative bacteria) impact of the DFE on the performance of methanogenic biofilm reactors, in order to allow process intensification and implementation of such a two-stage DF-AD system. To the best of our knowledge, such an evaluation of the operation of a two-stage DF-AD system has not yet been reported.
This study focused on the co-production of biohydrogen and methane (biohythane) from OFMSW using a two-stage AD system, comprised of a batch pilot scale DF and a continuous methanogenic biofilm reactor. In addition, the biotic and abiotic role of the DFE on the performance of methanogenic biofilm reactors was assessed, while the reactors were fed with different types of DFEs (biotic and abiotic). Moreover, the impact of organic shock loads on the methanogenic biofilm reactors was studied. The microbial communities in the two methanogenic biofilm reactors were characterized as well.
Materials and Methods
Substrate
Simulated OFMSW was prepared according to the composition of this waste type reported by the solid waste collector and valorization company TRIFYL (Labesssière-Candeil, France). The waste composition by weight was: 7% beef meat, 3.9% coffee, 4.3% rice, 20.9% potatoes, 5.1% biscuit, 5.1% garden waste, 1.9% yogurt, 31.7% white office paper, 16.7% packing cartoons, and 3.4% color cartoons. The physico-chemical characteristics of the OFMSW are given in Table 1.

Table 1. Main characteristics of the organic fraction of municipal solid waste and the inocula used in this study.
The office paper, packing cartoons and colored cartoon, collected from nearby offices and stores, were separately crushed to a size of 1.0 × 1.0 cm using a waste shear crusher (BLiK crusher, BB230 model), and stored under dry conditions at room temperature until use. The garden waste, collected from a nearby garden, and all the remaining waste fractions were prepared freshly based on the waste composition. The mixed waste was then blended with an electrical food blender without adding water, and then used for the DF experiment.
Inoculum
The mixed culture used as a seed for the DF process was obtained from the full scale landfill AD bioreactor of TRIFYL (Labesssière-Candeil, France). The characteristics of both inocula for the DF process and the methanogenic biofilm reactor are presented in Table 1. The mixed culture used in the DF process was initially pretreated thermally at 100°C for 60 min to inactivate the hydrogen consumers (methanogens) and enrich the fermentative hydrogen producing bacteria (Ghimire et al., 2015b). The methanogenic biofilm reactors were inoculated with anaerobic sludge sampled from a full scale AD plant located in Marseille (France). The plant treats sugar processing wastes at mesophilic conditions (35 ± 2°C). The collected sludge was temporarily kept in a 35°C room until use for the experiments.
Reactor Set-Up
Pilot Scale Batch DF Reactor
A pilot scale batch fermenter (Figure 1) was used for the DF process with a dual purpose: (i) to demonstrate the scale-up operation of the DF process and (ii) to obtain a higher volume of the DFE under the same operational conditions. The fermenter had a total and working volume of 60 and 20 liter, respectively, with a depth of 55.9 cm and an inner diameter of 37 cm (Table 2). The temperature was maintained at mesophilic conditions (35 ± 2°C) by recirculating hot water in the outer jacket of the reactor from a thermocouple controller. The pH was monitored during the process with an immersed pH probe inside the reactor (Mettler Toledo 1100 Calimatic). The substrate to inoculum ratio (S/I) was 20 gVSsubstrate/gVSinoculum. This initial organic loading was considered higher compared to the previous studies conducted on the batch DF process (Pan et al., 2008; Nathoa et al., 2014; Ghimire et al., 2016). No initial pH adjustment was performed in this study. Prior to starting the DF operation, the fermenter was flushed with nitrogen gas to ensure an initial anaerobic atmosphere. The total gas volume was measured daily by means of a gas Ritter meter (TG05/S, Bochum, Germany) and samples were taken for gas composition analysis. The batch DF process lasted for seven days and the experiment was performed in duplicate.
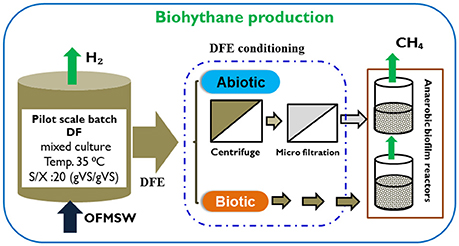
Figure 1. Schematic representation of the pilot scale DF and continuous methanogenic biofilm reactor.
Methanogenic Biofilm Reactor
Two identical methanogenic biofilm reactors, named as Rabiotic and Rbiotic, were used for the second methanogenic stage (Figure 1). The reactors were made up of a PVC column each with an effective volume of 0.5 L (internal diameter 9 cm, height 11 cm). Anox Kaldness-K1 (Veolia, Sweden) was used as biofilm carrier material for both reactors. The Kaldness-K1 carrier is a polyethylene cylinder with a cross inside and “fins” on the external surface. It has a specific weight of 145 kg/m3 and specific surface area of 500 m2/m3. To achieve a good substrate removal efficiency without crowding the reactor, 20% of the reactor (volume basis) was filled with Kaldness-K1 carrier as described by Yeshanew et al. (2016b). The reactors were operated in parallel at mesophilic conditions (35 ± 2°C) by using a thermostatic hot water reservoir. Initial anaerobic conditions were created by flushing both reactors with nitrogen gas.
The Rabiotic was fed with a DFE that further underwent solid separation and microfiltration as discussed below in sub-section Preparation of the DFE (Methanogenic Biofilm Reactor Influent). Conversely, the Rbiotic was fed with the DFE which was not further pretreated. The influent was continuously pumped into the bottom of the methanogenic reactors by means of a peristaltic pump (Masterflex L/S, France). The influent was stored inside a refrigerator throughout the experiment to maintain a constant temperature at 4°C. The liquid inside the reactor was recycled intermittently from the bottom to the top of the reactor at a flow rate of 60 ml/h in order to provide good contact between the biomass and the substrates. The methanogenic biofilm effluent was discharged at the top of the reactor via a coiled-tube designed for the effluent outlet. Effluent samples were taken three times a week for the analysis of pH, COD, and VFAs. Biogas production was measured by a volumetric gas flow Ritter meter (MGC-1 V3.3, PMMA) and gas samples were taken regularly for gas composition analysis.
Preparation of the DFE (Methanogenic Biofilm Reactor Influent)
At the end of each pilot scale DF assay, around 7.5 Liter of the DFE was collected, which initially underwent a residual solid settlement and pre-filtration as reported by Ghimire et al. (2015b). Briefly, the homogenized DFE aliquot (15 Liter in total) was divided into equal volumes and further prepared into two conditions: biotic and abiotic DFE aliquots.
For the abiotic conditions, the aliquot was firstly centrifuged at 15,000 rpm for 20 min. The supernatant was then further filtered through a membrane filter having a 1.2 μm pore size (Nylon WH, Ireland). It was assumed that the majority of the microbiota present in the DFE was eliminated by the membrane filtration (Turon et al., 2015). The DFE for the biotic conditions was used directly without any pretreatment. Both the abiotic and biotic DFE were stored at −20°C until use for the experiments. Then, it was thawed to room temperature (25°C) prior to feeding to the methanogenic biofilm reactor. In this way, the composition of the feed for the methanogenic reactors was constant throughout the experimental period.
Start-Up Process and Operation of the Methanogenic Biofilm Reactors
Prior to feeding the DFE to the methanogenic biofilm reactors, synthetic carbohydrate rich wastewater was used to start-up both reactors. The latter was composed of a mixture of acetate, butyrate, and ethanol at a volumetric ratio of 0.5: 0.3: 0.1, respectively (Yeshanew et al., 2016b). Ammonium chloride (NH4Cl) served as nitrogen source based on a carbon to nitrogen (COD/N) ratio of 20 (Karadag et al., 2015). In addition, the feed was supplemented with nutrients and trace elements as indicated elsewhere (Yeshanew et al., 2016b). A similar start-up strategy as described by Cresson et al. (2007) was followed for the start-up of both methanogenic biofilm reactors: the organic loading rate (OLR) was increased stepwise while keeping a relatively shorter hydraulic retention time (HRT). When nearly 80% COD removal efficiency was attained, the subsequent OLR was increased by 20%. In this study, the OLR of both methanogenic biofilm reactors was gradually increased starting from 0.5 gCOD/L.day to the final designated OLR of 9.0 gCOD/L.day. A shorter and constant HRT of 1 day was maintained throughout the operation of both reactors (Cresson et al., 2007).
After finalizing the start-up process within 25 days, i.e., reaching the desired OLR (9.0 gCOD/L.day) by achieving a satisfying COD removal efficiency (≥ 80%) and stable methane production rate, the two reactors were fed the different DFE: Rabiotic was fed with the abiotic DFE, while Rbiotic was fed with the biotic DFE. This was performed in order to assess the biotic and abiotic role of the DFE on the subsequent methanogenic biofilm reactors.
The responses of the reactors performance toward shock loads were assessed by imposing an increase in OLR (18, 27, and 36 gCOD/L.day), through increasing the influent concentration, while keeping the HRT constant at 1 day. Changes in the reactor performance in response to the shock load were monitored during the operational phases. The OLR was restored to the pre-shock level (9 gCOD/L.day) after the maximum shock load (36 gCOD/L.day) was applied.
Microbiological Analysis
The relative abundance of different groups of the archaeal and bacterial community in the two methanogenic biofilm reactors was quantified using quantitative PCR (qPCR). Initially, DNA was extracted and purified with the fast DNA SPIN kit for soil according to the manufacturer's instruction (MP Biomedicals). An aliquot of 1 ml of sample was first centrifuged for 10 min, and then the pellet was resuspended by vortexing in 115 μl of 10% (wt/vol) N-lauroyl-sarcosine (N-LS) and 385 μl of 4 M guanidine thiocyanate −0.1 M Tris-HCl (pH 7.5). The homogeneous sample (500 μl) was stored at −20°C prior to DNA extraction. DNA quantity and purity were assessed using spectrophotometry (Infinite NanoQuant M200, Tecan). The V3 region of the 16S rRNA gene was amplified to analyse the structure of the archaeal and bacterial community. The primers W49F and W104R were used for the bacterial (29), and W274R and W275F were used for the archaeal (Braun et al., 2011). PCR amplifications were carried out using Mastercycler thermocycler (Eppendorf, Hamburg, Germany).
The PCR mixture used for the amplification of the bacterial sequences contained 5 μl of 10 × Pfu Turbo buffer, 0.2 mM deoxynucleoside triphosphates (dNTPs), 8 nM each primer, 1.25 U of Pfu Turbo DNA polymerase (Stratagene, La Jolla, CA), 1 μl of genomic DNA, and water added to obtain a final volume of 50 μl. The PCR conditions were an initial denaturing step of 2 min at 94°C; 25 cycles of a three-stage program of 30 s at 94°C, 30 s at 61°C, and 30 s at 72°C; and a final elongation for 10 min at 72°C (Braun et al., 2011).
The PCR mixtures used for the amplification of archaeal sequences contained 2.5 μl of 10 × Pfu Turbo buffer, 0.2 mM dNTPs, 10 nM each primer, 0.625 U of Pfu Turbo DNA polymerase (Stratagene, La Jolla, CA), 0.5 μl of genomic DNA, and water added to obtain a final volume of 25 μl. The PCR conditions were an initial denaturing step of 2 min at 94°C; 30 cycles of a three-stage program of 30 s at 94°C, 30 s at 65°C, and 30 s at 72°C; and a final elongation for 10 min at 72°C. All reactions were stopped by cooling the mixture to 4°C (Braun et al., 2011).
The obtained community profiles were aligned based on the ROX internal size standards and normalized with the package Statfingerprints (Michelland et al., 2009) in R 2.12 (R Development Core Team, 2010). Statistical analyses such as dissimilarity indices and analysis of variance were performed with the R package vegan 2.0-0 (Braun et al., 2011) using functions vegdist and anosim, respectively.
Analytical Methods
The total solids (TS) and volatile solids (VS) concentrations were measured according to the Standard Methods for examination of water and wastewater (APHA, 2005). The pH was determined with a Mettler Toledo 1100 Calimatic pH meter and electrical conductivity was measured by an electrical conductivity meter (WTW BIOBLOCK, LF325). The chemical oxygen demand (COD) analysis was determined using analytical test kits for high chloride content waters 146 (MERCK 117059.0001). The total alkalinity concentration was quantified by titration with a strong acid (HCl) (Yeshanew et al., 2016b). Volatile fatty acids (VFAs), lactic acid and alcohols were measured in triplicates with a high performance liquid chromatograph (HPLC) as described elsewhere (Carrillo-Reyes et al., 2016). Biogas composition (CH4, CO2 and H2) was analyzed by a gas chromatograph (Clarus 580, Perkin Elmer) equipped with a thermal conductivity detector as described by Carrillo-Reyes et al. (2016). All tests were carried out in triplicate and results are given as average values of the tests.
Result and Discussion
Pilot Scale Batch DF Process
Biohydrogen Yield
Figure 2A shows the cumulative hydrogen production curve and operational pH during the batch pilot scale DF process. The cumulative hydrogen production curve showed an increasing trend and then reached a plateau. The pH steadily decreased along with the increased biohydrogen production from 8.8 to 5.3 (± 0.1) (Figure 2A). During the maximum hydrogen production rate (day 1–3), the pH was between 5.5 and 6.5. This pH value has been indicated as an optimal value for an efficient hydrogen production during the DF process using mixed cultures (Faloye et al., 2014; Cavinato et al., 2016; Yeshanew et al., 2016c). The average hydrogen gas yield from the pilot scale DF process was 41.7 (± 2.3) ml H2/gVSadded. These results are comparable with the work of Liu et al. (2006), who obtained a hydrogen yield of 43 ml H2/gVSadded from the OFMSW using a mixed culture DF process at mesophilic (37°C) temperature. Besides, the hydrogen yield obtained in the current study was in line with the hydrogen potential range of OFMSW (between 26.3 and 96 ml H2/gVSadded) reported by Okamoto et al. (2000) during lab scale DF under mesophilic conditions. Studies have shown that the hydrogen production by mixed cultures could be directly correlated to the sugar content in the feed substrates (Monlau et al., 2015; Yeshanew et al., 2016c). OFMSW is one of the suitable substrates for the fermentative hydrogen production process due to its high carbohydrate fraction and various other organic compounds, which are easily accessible for the fermentative bacteria (Dong et al., 2009; Guo et al., 2014).
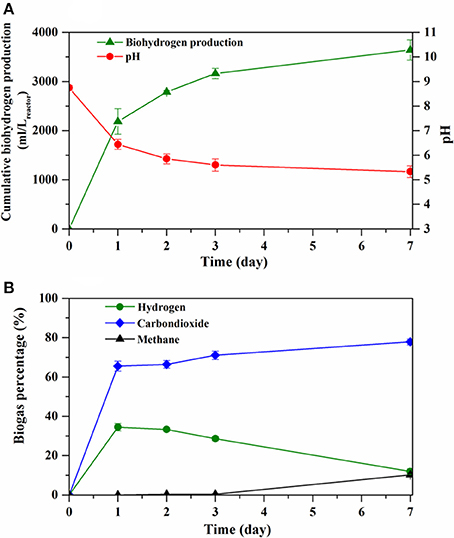
Figure 2. Cumulative hydrogen production curve and pH (A) and biogas composition (B) during the DF process.
As depicted in Figure 2B, the produced biogas during the DF process mainly consisted of hydrogen and carbon dioxide gas with a maximum hydrogen concentration of around 34.4% on day 2. However, the hydrogen concentration declined to 28% on day 3 and 17% at the end of the process (Figure 2B). The decreased hydrogen concentration could be associated to hydrogen consumption by homoacetogens and/or hydrogenotrophic methanogens, as methane gas was detected from day 3 onwards (Figure 2B). This could be attributed to the long fermentation time allowed for the DF process (more than 3 days). Hence, the hydrogen-consuming microbial groups started to develop gradually after day 3 of the DF operation (Figure 2B). Similar to this study, Pasupuleti and Venkata Mohan (2015) observed a drop in the hydrogen concentration and subsequent increase in methane concentration after 12 h during biohydrogen production from distillery wastewater in a batch reactor system, which was due to the dominance of methanogenic fermentation over acidogenic fermentation through time. Another possible reason for the drop in the hydrogen concentration could be the insufficient heat pretreatment of the mixed culture. Heat pretreatment of a mixed culture at 100°C has been regarded as an efficient method to inactivate the hydrogen-consuming microbial population and enrich hydrogen producing spore-forming bacteria, such as Clostridium species (Zahedi et al., 2016). Conversely, a heat pretreatment at 100°C for 1 h has been found to not completely inhibit the homoacetogenic and methanogenic groups (Alibardi et al., 2012) and our result is consistent with this observation.
The results from the current work suggest that during the pilot scale operation of the DF process, the mode of inoculum heat pretreatment has a great relevance and needs to be carefully monitored in order to effectively suppress the hydrogen-consuming anaerobic microbial populations, taking into account the large inoculum volume used for the large reactor size. For instance, applying a proper mixing during the course of the inoculum heat pretreatment results a homogeneous heat applied and hence could inhibit more efficiently the hydrogen-consuming anaerobic groups.
The majority of the previous work on the DF process, although promising, has been conducted at the laboratory scale with a typical reactor volume up to 0.5 L (Dong et al., 2009; Ghimire et al., 2015b; Zahedi et al., 2016). As a consequence, limited information is available on the efficiency of biohydrogen production using the DF process at a larger scale system, i.e., pilot and/or industrial scale (Lee et al., 2010; Cavinato et al., 2012; Faloye et al., 2014). The pilot scale operation carried out in this study may provide experience with upgrading such a process from laboratory to large scale operation and hence promote the intensified practice of the DF process. Cavinato et al. (2012) carried out a two-stage pilot scale thermophilic DF and AD of food waste for the simultaneous production of biohydrogen and methane. The hydrogen yield was 66.7 L H2/kg TVS (total volatile solids) at an OLR of 16.3 kgTVS/m3.day and HRT of 3.3 days. In addition, Lin et al. (2011) reported the long term pilot-scale operation of a high rate reactor for biohydrogen production, fed with sucrose as the substrate at an OLR between 40 and 240 kg COD/m3.day. The authors achieved a maximum hydrogen yield of 1.04 mol H2/mol sucrose at an OLR of 240 kg COD/m3.day.
Chemical Characteristics of the DFE
The DF process not only produces hydrogen gas, but also a liquid supernatant rich in VFAs and alcohols, the DFE (Yeshanew et al., 2016c). Table 3 presents the average chemical characteristics of the DFE produced from the pilot scale DF process duplicate experiments. The DFE was characterized by a high soluble COD content, indicating the efficient hydrolysis of the particulate fraction of the OFMSW to the liquid phase during the DF process (Yeshanew et al., 2016a). The distribution of intermediate metabolites in the DFE is considered as an indicator for monitoring the hydrogen production performance during the DF (Ghimire et al., 2015b).
In this study, the main metabolites were comprised of VFAs and alcohols, with acetic and butyric acid as the dominant VFAs from the process (Table 3). This suggested that the metabolic activity involved in the hydrogen-producing pathways was of an acetate-butyrate fermentation type (Ghimire et al., 2015b; Yeshanew et al., 2016c). Likewise, Guo et al. (2014) observed the prevalence of acetic and butyric acid during the batch fermentative biohydrogen production from the OFMSW. The result also suggests the mixed culture used in this study contains dominantly a Clostridium species, as evidenced by the typical hydrogen and acid producing DF process (Dong et al., 2009; Carrillo-Reyes et al., 2016). Acetic and butyric acid are the primary soluble metabolites generated by Clostridium species at 37°C and pH 5.5 (Guo et al., 2014). Moreover, the DFE had a high total alkalinity concentration, representing its high buffering capacity. This high alkalinity concentration can be ascribed to the presence of a high concentration of total alkalinity in the mixed inoculum used for the DF experiments (Table 1).
Continuous Operation of Methanogenic Biofilm Reactors
Start-Up Process
Figure 3 illustrates the reactor performance in terms of COD removal efficiency, methane yield and effluent pH during the start-up process of both methanogenic biofilm reactors. The objective of the start-up period of the methanogenic biofilm reactors was to enhance the biofilm formation on the carrier materials within a possible shorter operational time by washing out the suspended biomass, meanwhile reaching the desired higher OLR with satisfying substrate removal efficiency (Escudié et al., 2011). According to the start-up strategy suggested by Cresson et al. (2007), the COD removal efficiency should exceed 80% before the subsequent raise of the OLR (Wahab et al., 2015). In this study, both reactors were initially launched at an OLR of 0.5 gCOD/L.day and a shorter HRT of 1 day. Afterwards, the OLR was gradually increased by 20% on a daily basis. For both methanogenic biofilm reactors, the observed COD removal efficiency at each OLR step was nearly 80 (± 5.4)% (Figure 3A). At the end of the start-up process, the maximum OLR (9 gCOD/L.day) was kept constant for 10 consecutive days (more than 3 HRTs) in order to ensure the stable operation of the reactors at a higher OLR. During these days, both reactors achieved a stable COD removal efficiency ranging between 81 and 86%.
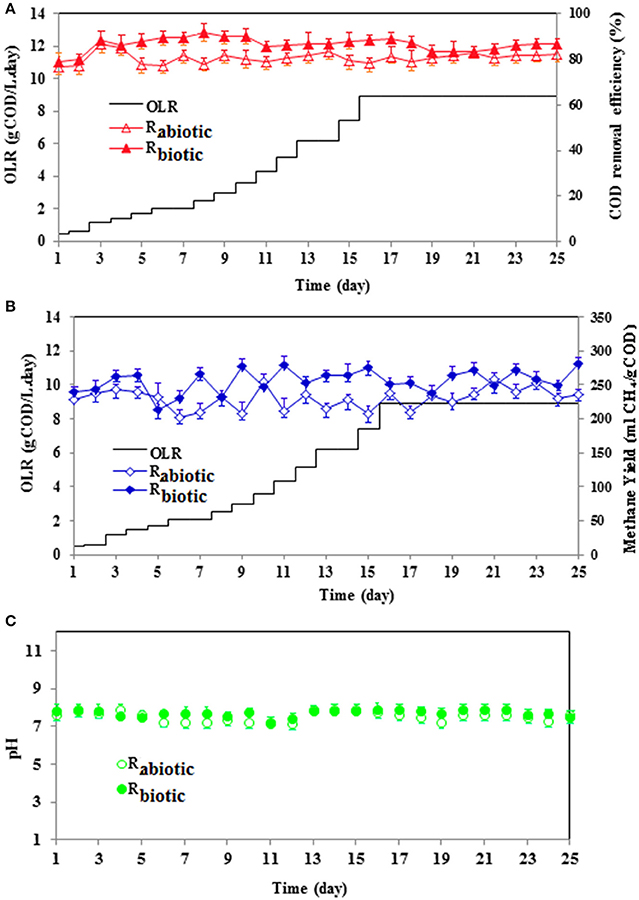
Figure 3. Performance of the methanogenic biofilm reactors during the start-up process: (A) COD removal efficiency, (B) methane yield at each OLR increase, and (C) pH as a function of operational time.
The methane yield of both reactors during the start-up process was between 200 and 270 (± 5.3) ml/gCODremoved (Figure 3B). This methane yield is quite far from the theoretical amount for organic substrate, i.e., 350 ml/gCODremoved. However, it should be noted that during the start-up period of anaerobic biofilm reactors, the AD biomass utilizes some fractions of the incoming carbon to build the biopolymer matrix for the formation of a matured biofilm (Michaud et al., 2002). Hence, the methane yield during the start-up process is often less than the theoretical amount (Cresson et al., 2007; Wahab et al., 2015). Nonetheless, a stable methane yield was observed in both of the methanogenic biofilm reactors, irrespective of the increasing OLR, which shows the high activity of the methanogenic archaea during the process. Besides, the pH of both reactors was kept at a desirable range for AD reactors throughout the start-up period, i.e., between 7.3 and 7.9 (± 0.2), reflecting a stable AD process (Figure 3C).
The start-up process of both methanogenic biofilm reactors was established within 25 days, i.e., the designated OLR of 9.0 gCOD/L.day and a COD removal efficiency exceeding 80% were achieved. This start-up period (25 days) was considered relatively short compared to previous studies, where the start-up period amounted to 150 days (Yeshanew et al., 2016b). This might be attributed to the adopted start-up strategy and the type of wastewater used (mixture of acetic and butyric acid and ethanol), which is readily biodegradable by acetogenic and methanogenic anaerobic biomass. A long start-up period, which might take from 2 to 9 months, is one of the major drawbacks of anaerobic biofilm reactors (Yeshanew et al., 2016b). This is mainly due to the sensitive nature of the slow growing anaerobic biomass to operational conditions, in addition to their vulnerability to easy washout from the reactor (Van Lier et al., 2015). Accordingly, several start-up approaches have been reported in the literature (Escudié et al., 2011; Yeshanew et al., 2016b). The applied start-up strategy constituting of a progressive increase of the OLR at a shorter HRT has favored the biofilm formation, through facilitating the quick wash out of the suspended biomass from the reactor by creating a high competition between suspended and attached biomass for substrates (Cresson et al., 2007; Mattei et al., 2015; Wahab et al., 2015). The result obtained in the current study (i.e., higher COD removal efficiency and stable operation) also confirmed the effectiveness of this strategy in retaining the slow growing methanogenic biomass in the methanogenic biofilm reactor within a short period of time, i.e., 25 days (Figure 3).
Performance of Methanogenic Biofilm Reactors Treating the DFE
After the start-up process was completed (Figure 3), the performance of both methanogenic biofilm reactors was evaluated, while feeding the DFE under abiotic and biotic conditions (Figures 4, 5). Based on the observed performance of both reactors, the operational period can be classified into three phases: stable (from day 1–31), shock load (from day 32–34) and recovery (from day 35–46) phase.
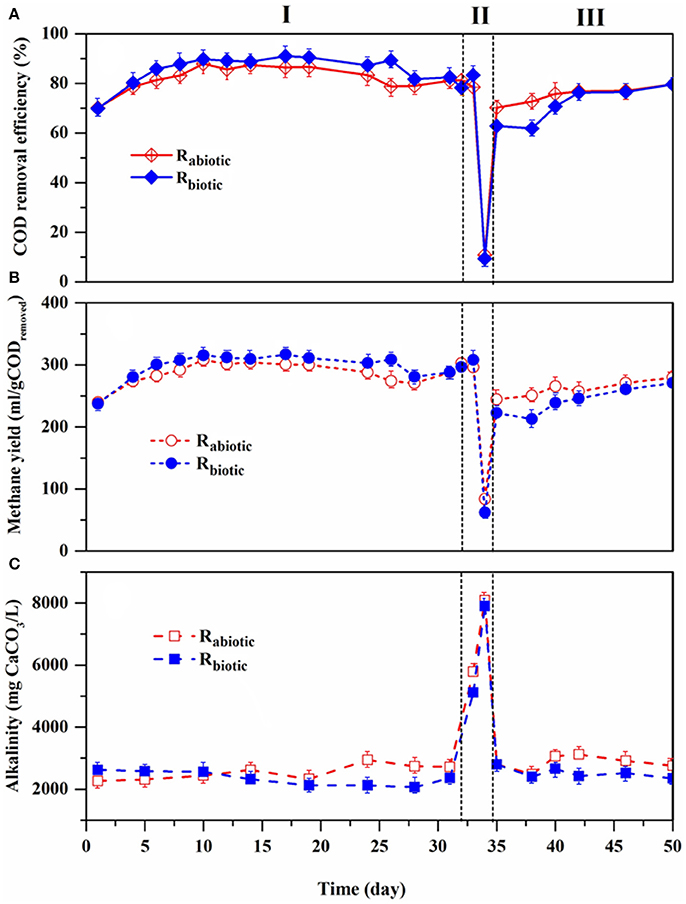
Figure 4. Profiles of the performance of Rabiotic and Rbiotic: (A) COD removal efficiency, (B) methane yield, and (C) total alkalinity.
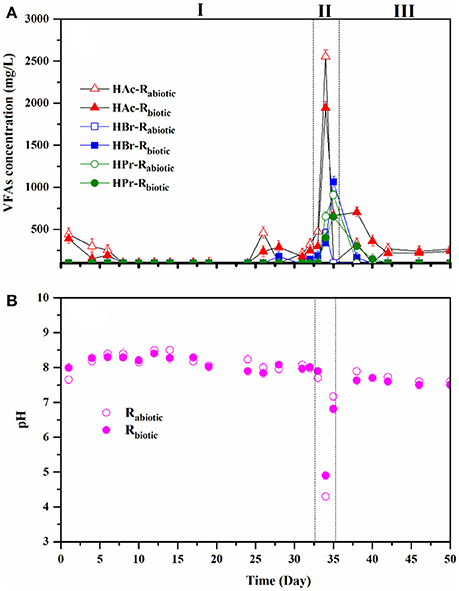
Figure 5. VFAs concentration (A) and pH (B) during the operation of Rabiotic and Rbiotic fed with DFE.
Phase I: stable process operation (day 1–31)
In this operational phase, the reactors were operated at an OLR and HRT of 9 gCOD/L.day and 1 day, respectively, while Rabiotic and Rbiotic were fed with the abiotic and biotic DFE, respectively. Both reactors demonstrated a stable performance in terms of methane yield, COD removal efficiency, and pH (Figures 4A–C). No significant variation of the performance pattern was noted between the two reactors, regardless of the different DFE feeding (abiotic and biotic). The observed methane yield and COD removal efficiency in both reactors were between 280–300 (±4.7) ml CH4/gCODremoved and 80–90 (± 2.6)%, respectively. The pH remained between 7.5 and 8.5 (± 0.2) throughout the process (Figure 5B), showing the optimal ranges for the methanogenic biomass. In addition, the VFAs concentrations in the effluent were low, <200 mg/L (Figure 5A), indicating the capability of the methanogenic archaea to efficiently utilize the VFAs in the DFE and convert them into methane gas.
The reactor operating conditions, i.e., an OLR of 9 gCOD/L.day and 1 day HRT, are regarded as relatively high loading rates compared to the studies carried out by the conventional suspended biomass AD systems, such as a CSTR (Kinnunen et al., 2014; Regueiro et al., 2015; Khemkhao et al., 2016). Operating at a short HRT and high OLR is one of the important features of anaerobic biofilm reactors, since the majority of the methanogenic biomass is retained in the reactors by the biofilm system. Such a system possesses several advantages, including high metabolic activity rates and stable operation at higher loading rates compared to the conventional typologies (Van Lier et al., 2015; Yeshanew et al., 2016c). As a result, biofilm-based AD systems have been widely applied for the treatment of several types of industrial and municipal wastewater (Van Lier et al., 2015).
Our results are consistent with previous studies that have been carried out using two-stage biofilm AD systems from various organic wastes. Chu et al. (2008) observed a high methane yield and stable performance of methanogenic biofilm reactors while treating OFMSW in two-stage systems. The authors obtained a methane yield of 464 ml CH4/g VSadded at an OLR of 16.3 gCOD/L.day and HRT of 5 days. Similarly, Yeshanew et al. (2016c) reported steady state operation of a methanogenic biofilm reactor treating food waste using a two-stage AD system at an OLR of 6.1 gCOD/L.day and HRT of 1.5 days, with a methane yield of around 334.7 ml CH4/gCOD.day. Moreover, Lee et al. (2010) emphasized the stable operation of a biofilm based methanogenic reactor treating OFMSW in a thermophilic two-stage AD system, and achieved 250 ml CH4/gCOD at an OLR of 8.4 gCOD/L.day and a HRT of 7.7 days.
Phase II: shock load phase (day 32-34)
The reactors were then exposed to a transient organic shock load on operational days 32–34, aiming to assess the limiting organic load conditions of the methanogenic biofilm reactors. Accordingly, on day 32, both reactors were imposed to an increased OLR from 9 to 18 gCOD/L.day (first high OLR), by increasing the influent COD concentration from 9 to 18 g/L, while keeping a constant HRT of 1 day. The reactor performance was, however, not affected by the raised organic load as shown by the stable methane yield, COD removal efficiency, pH, and VFAs concentration (Figures 4, 5). Hence, the OLR of both reactors was further increased to 27 gCOD/L.day on day 33. Surprisingly, no signs of process disturbance and instability were noticed in both reactors at this elevated OLR as well.
This high tolerance toward the increased organic load can be attributed to the substrate characteristics (i.e., high alkalinity content of the DFE, Table 3) and the reactor configuration, i.e., methanogenic biofilm reactors are generally less susceptible to high organic loads due to the presence of well attached and active biomass (Yeshanew et al., 2016c). Figure 4C confirmed the presence of a high alkalinity concentration inside the reactor, as the total alkalinity increased along with the OLR. This study showed the benefits of a coupled two-stage AD system to operate stably even at a transient change of OLR. The DFE served as a suitable substrate for the subsequent methanogenic biofilm reactor as it offered not only VFAs to produce methane, but also a high alkalinity to maintain the stability of the process at the higher OLR. This finding highlights the positive and synergistic correlation of the coupled system for an efficient operation of the two-stage AD process.
On day 34, the OLR was increased to 36 gCOD/L.day (i.e., 3-fold increment), targeting to reach the maximum organic shock load. It was performed through raising the influent COD concentration to 36 g/L. In addition, 10 ml of diluted acid (0.1 N HCl) was added together with the influent of both reactors in order to lower the pH and disturb the methanogenic process, since the presence of high alkalinity in the DFE maintains the pH in the range for the methanogenic process (6.5–8.5). Subsequently, on this operational day, process deterioration occurred in both reactors: the methane yield dropped from 300 (± 5.7) to 40 (± 2.1) ml/gCOD along with the COD removal efficiency down to 10% (Figures 4A,B). It was accompanied by a drop of pH to around 4.3. A maximum peak in the VFAs concentration, in particular acetic acid (up to 2555 ± 10.3 mg/L), was recorded on this day (Figure 5A), indicating the system was overloaded. Besides, propionic acid was detected, showing the imbalance of the AD process and the poor reactor performance. In practical applications of the AD process, e.g., in anaerobic wastewater treatment plants, the reactor stability upon a hydraulic and/or organic shock load is one of the most important aspects of the reactor design, owing to the variable nature of domestic wastewater in terms of flow and organic load (Gopala Krishna et al., 2008).
Similar to our study, Gopala Krishna et al. (2008) investigated the stability of an anaerobic baffled reactor (ABR) upon a short term organic shock load when treating low strength wastewater (COD concentration 500 mg/L). The organic shock load was performed by increasing the influent COD concentration 2 and 3 times (i.e., 100 and 200% increase, respectively) each for a period of 4 and 8 h, while maintaining a constant HRT of 8 h. The authors reported that the ABR could sustain the organic shock load during a first organic load rise due to the presence of active biomass in the system. However, high COD and VFAs concentrations were recorded during a second shock load (200% increase of the initial COD).
Phase III. process recovery (day 35-50)
After the organic shock load event, the OLR was reverted to the previous condition, i.e., an OLR of 9 gCOD/L.day and a HRT of 1 day. A fast recovery of the process performance was observed for both reactors (Figure 4). Besides, it was noticed that Rabiotic had a higher performance than Rbiotic in this phase (Figure 4), indicating the abiotic role of the DFE for a rapid restoration of the AD process. From day 35 onwards, the methane yield and COD removal efficiency were notably increased. The accumulated VFAs, in particular the acetic acid concentration, were rapidly depleted and the pH maintained within the range of 7.5–8.0 (± 0.1) (Figure 5A). Afterwards, both reactors operated stably at the designated operational condition. The fast recovery was due to the high alkalinity of the DFE, providing enough buffering capacity to the methanogenic biofilm reactors and hence potentially maintained the process stability.
Microbial Communities in the Methanogenic Biofilm Reactor
The microbial community present in the two methanogenic biofilm reactors was monitored during the stable operation and recovery phase to further study the role of the biotic part of the DFE on the distribution of the microbial diversity in the second stage methanogenic biofilm reactors. The relative abundance of the main microbial groups is given in Table 4. Methanobacteriales constituted the major group of methanogens (64–69% during the stable operational period), followed by Methanosaeta, Methanospirillum, and Methanosarcina. Similar to the reactor performance, no significant variations of the archaeal microbial community were observed between the two methanogenic biofilm reactors, regardless of the different DFE fed to both reactors.
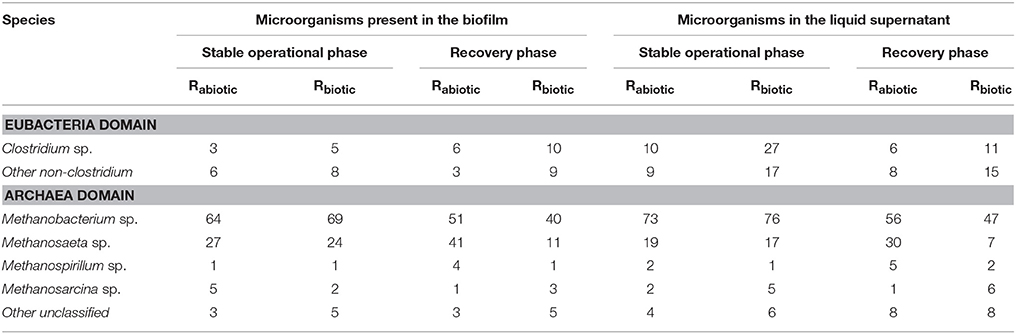
Table 4. Relative abundance of the microorganisms in the two methanogenic biofilm reactors during stable operation and the recovery phase (%).
The larger Methanomicrobiales population was attributed to the high loading rates of both methanogenic biofilm reactors (OLR ≥ 9 gCOD/L/day) compared to previous studies (Yeshanew et al., 2016c). Methanomicrobiales are the most resistant methanogens to higher OLRs (Zahedi et al., 2016). It is important to note that methanogenic reactors should be operated under suitable conditions for syntrophic bacteria (Si et al., 2016). Hence, the increased amount of Methanobacteriaceae would enhance the methane production and COD removal efficiency. Our result is supported by Si et al. (2016), who reported a higher amount of Methanobacteriales during biohythane production in a two-stage system. Similarly, Zahedi et al. (2016) studied the microbial processes involved in the two-phase AD during hydrogen and methane production from biowaste. Methanobacteriales constituted the major group of methanogens in their second stage methanogenic reactor relative to the other methanogenic groups. The authors also emphasized that Methanobacteriales are more tolerant to high organic load conditions compared to Methanosaeta species.
Regarding the fermentative bacterial community in the two methanogenic biofilm reactors, a relatively larger population of bacterial, such as Clostridium sp. was identified in Rbiotic than in Rabiotic (Table 4). This was due to the elimination of the majority of the microorganisms present in the DFE fed to Rabiotic, as described in section Preparation of the DFE (Methanogenic Biofilm Reactor Influent). Clostridium sp. was the dominant microbial species in the DF for the production of hydrogen and VFAs in mixed culture with a pH between 5.0and 6.0 from organic substrates (Guo et al., 2010; Si et al., 2016). This observation confirmed that the presence of fermentative bacteria in the DFE did not have a significant impact on the distribution of the methanogenic biofilm community in both second stage reactors. It should be noted that as there are no substrates for the fermentative bacteria in the second stage biofilm reactor, the bacterial species present in the raw DF effluent do not impact the performance and microbial dynamics of the methanogenic biofilm reactor. Turon et al. (2015) reported a major influence of the fermentative bacteria present in the DFE on the downstream process during heterotrophic cultivation of microalgae using DFE. This was due the competition between microalgae and bacteria, which limited the availability of carbon, nitrogen, phosphate, and oxygen to the microalgae (Turon et al., 2015). Hence, during the coupled process of DF and heterotrophic cultivation of microalgae, the DFE should be sterilized. In contrast, our result showed that the DFE can be used directly in the AD system without further treatment. This is a notable feature of the coupled DF-AD system, making the process cost-effective (Lee et al., 2014).
Overall Energy Recovery of the Coupled DF-AD System
This study demonstrated the potential co-production of hydrogen and methane (biohythane) from OFMSW via the pilot scale DF followed by a methanogenic biofilm reactor. The methanogenic biofilm reactors demonstrated an excellent performance and stability in terms of operating at a higher OLR, robustness against organic load change, and faster process recovery. Furthermore, this study is the first to report the role of fermentative bacteria from the DF step on the subsequent methanogenic biofilm reactor, showing minor performance differences during stable operation, but a noticeable effect during the recovery phase. Accordingly, prolonged operation of such a two stage system is recommended for taking advantage of the high operational stability for commercial application.
Based on the obtained result, the energy recovery from the overall system was computed, considering the total specific gas production and composition as well as the initial COD content of the OFMSW (Table 1). The estimated energy recovery from the OFMSW initial COD as biohythane in terms of hydrogen and methane gas was 28 and 72%, respectively. Si et al. (2016) reported an energy recovery of 19 and 81% from glucose in terms of hydrogen and methane, respectively, using a packed bed two-stage reactor system. Table 5 compares literature data performed on co-production of biohydrogen and methane from various organic substrates and the energy recovery from the substrates.
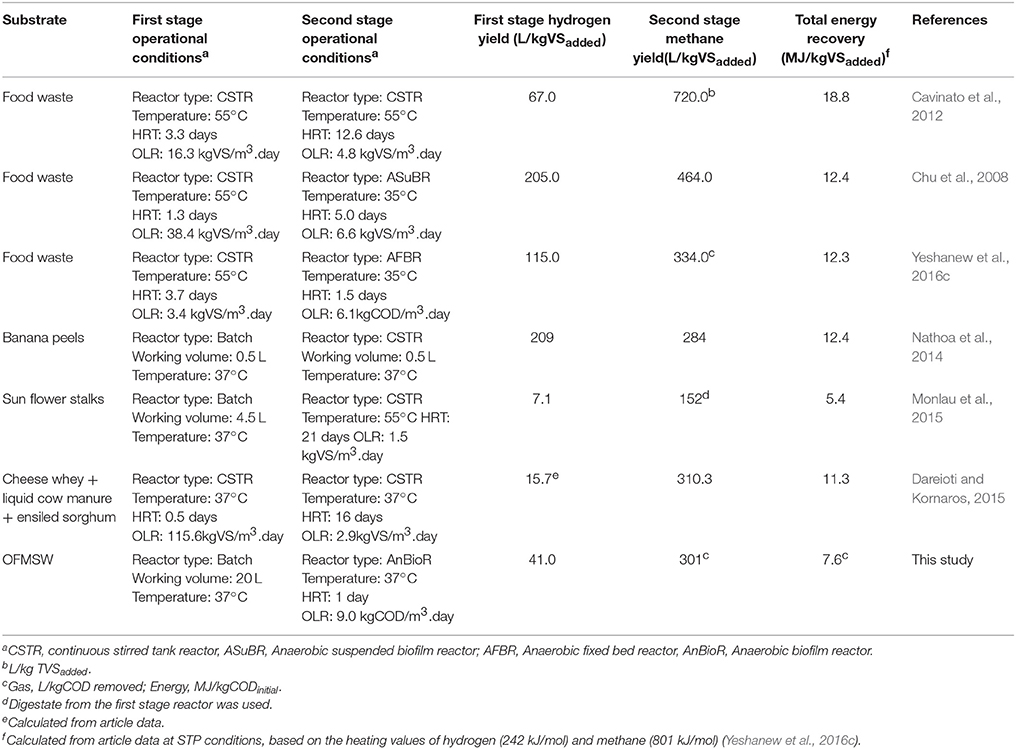
Table 5. Comparison of literature studies performed on the biohythane production potential from various organic wastes using two-stage AD systems under different conditions.
The energy yields of hydrogen and methane from the DF and methanogenic reactor were calculated based on the heating values of H2 (242 kJ/mol) and CH4 (801 kJ/mol). This study indicated OFMSW can be valorized by biohythane production with a good operational performance at high OLRs. The overall energy recovery obtained in this study was comparable to other studies (Table 5). However, a post treatment of the solid residue left after the mild separation of the DFE is suggested in order to accomplish a full conversion and valorization of the OFMSW, by a composting process.
Conclusions
This study showed the potential of OFMSW for simultaneously producing biohydrogen and methane using a pilot scale dark fermenter and lab scale methanogenic biofilm reactor, respectively. The pilot scale DF produced hydrogen gas [with a yield of 41.7 (± 2.3) ml H2/gVSadded] and DFE. The latter had a high total alkalinity (8.5 gCaCO3/L) and VFAs concentration, mainly acetic (8.6 g/L), and butyric acid (7.1 g/L). In the second stage methanogenic biofilm reactor, the observed methane yield and COD removal efficiency were between 280 and 300 ml/gCODremoved and 80 and 90%, respectively, during stable process operation. The most abundant methanogenic populations in the methanogenic biofilm reactors were Methanobacteriales species. The methanogenic biofilm reactor performance was not affected by the fermentative microorganisms present in the DFE. In addition, the methanogenic biofilm reactors showed a high resistance toward organic shock loads and fast recovery after a shock load episode.
Author Contributions
MY designed the study, performed the experiments, interpreted the data, wrote the manuscript and revised it until its final version. FP participated in doing the experiment and revising the manuscript critically. CB participated during the experiment. LF participated in designing the study, writing the manuscript and revising it critically. PL participated in writing the manuscript and revising it critically. GE participated in designing the study, writing the manuscript, and revising it critically. RE participated in designing the study, interpreted the data, writing the manuscript, and revising it critically. ET participated in designing the study, interpreted the data, writing the manuscript and revising it critically.
Conflict of Interest Statement
The authors declare that the research was conducted in the absence of any commercial or financial relationships that could be construed as a potential conflict of interest.
Acknowledgments
The authors would like to acknowledge the European Commission for the financial support through the Erasmus Mundus Joint Doctorate programme ETeCoS3 (Environmental Technologies for Contaminated Solids, Soils, and Sediments) under the grant agreement FPA n° 2010-0009.
References
Alibardi, L., Favaro, L., Lavagnolo, M. C., Basaglia, M., and Casella, S. (2012). Effects of heat treatment on microbial communities of granular sludge for biological hydrogen production. Water Sci. Technol. 66, 1483–1490. doi: 10.2166/wst.2012.336
APHA (2005). Standard Methods for the Examination of Water and Wastewater, 21st Edn. Washington, DC: American Public Health Association; American Water Works Association; Water Environment Federation.
Bonk, F., Bastidas-Oyanedel, J. R., and Schmidt, J. E. (2015). Converting the organic fraction of solid waste from the city of Abu Dhabi to valuable products via dark fermentation-economic and energy assessment. Waste Manage. 40, 82–91. doi: 10.1016/j.wasman.2015.03.008
Braun, F., Hamelin, J., Gévaudan, G., and Patureau, D. (2011). Development and application of an enzymatic and cell flotation treatment for the recovery of viable microbial cells from environmental matrices such as anaerobic sludge. Appl. Environ. Microbiol. 77, 8487–8493. doi: 10.1128/AEM.05549-11
Capson-Tojo, G., Rouez, M., Crest, M., Steyer, J.-P., Delgenès, J.-P., and Escudié, R. (2016). Food waste valorization via anaerobic processes: a review. Rev. Environ. Sci. BioTechnol. 15, 499–547. doi: 10.1007/s11157-016-9405-y
Carrillo-Reyes, J., Trably, E., Bernet, N., Latrille, E., and Razo-Flores, E. (2016). High robustness of a simplified microbial consortium producing hydrogen in long term operation of a biofilm fermentative reactor. Int. J. Hydrogen Energy 41, 2367–2376. doi: 10.1016/j.ijhydene.2015.11.131
Cavinato, C., Bolzonella, D., Pavan, P., and Cecchi, F. (2016). “Two-phase anaerobic digestion of food wastes for hydrogen and methane production,” in Enriched Methane. Green Energy and Technology, eds M. De Falco and A. Basile (Cham: Springer), 75–90.
Cavinato, C., Giuliano, A., Bolzonella, D., Pavan, P., and Cecchi, F. (2012). Bio-hythane production from food waste by dark fermentation coupled with anaerobic digestion process: a long-term pilot scale experience. Int. J. Hydrogen Energy 37, 11549–11555. doi: 10.1016/j.ijhydene.2012.03.065
Chu, C.-F., Li, Y.-Y., Xu, K.-Q., Ebie, Y., Inamori, Y., and Kong, H.-N. (2008). A pH- and temperature-phased two-stage process for hydrogen and methane production from food waste. Int. J. Hydrogen Energy, 33, 4739–4746. doi: 10.1016/j.ijhydene.2008.06.060
Cresson, R., Escudie, R., Carrere, H., Delgenes, J. P., and Bernet, N. (2007). Influence of hydrodynamic conditions on the start-up of methanogenic inverse turbulent bed reactors. Water Res. 41, 603–612. doi: 10.1016/j.watres.2006.10.037
Dareioti, M. A., and Kornaros, M. (2015). Anaerobic mesophilic co-digestion of ensiled sorghum, cheese whey and liquid cow manure in a two-stage CSTR system: effect of hydraulic retention time. Bioresour. Technol. 175, 553–562. doi: 10.1016/j.biortech.2014.10.102
Dong, L., Zhenhong, Y., Yongming, S., Xiaoying, K., and Yu, Z. (2009). Hydrogen production characteristics of the organic fraction of municipal solid wastes by anaerobic mixed culture fermentation. Int. J. Hydrogen Energy 34, 812–820. doi: 10.1016/j.ijhydene.2008.11.031
Escudié, R., Cresson, R., Delgenes, J. P., and Bernet, N. (2011). Control of start-up and operation of anaerobic biofilm reactors: an overview of 15 years of research. Water Res. 45, 1–10. doi: 10.1016/j.watres.2010.07.081
Faloye, F. D., Kana, E. B. G., and Schmidt, S. (2014). Optimization of biohydrogen inoculum development via a hybrid pH and microwave treatment technique-semi pilot scale production assessment. Int. J. Hydrogen Energy, 39, 5607–5616. doi: 10.1016/j.ijhydene.2014.01.163
Ghimire, A., Frunzo, L., Trably, E., Escudié, R., Pirozzi, F., Lens, N. L. P., et al. (2015a). A review on dark fermentative biohydrogen production from organic biomass: process parameters and use of by-products. Appl. Energy 144, 73–95. doi: 10.1016/j.apenergy.2015.01.045
Ghimire, A., Sposito, F., Frunzo, L., Trably, E., Escudié, R., Pirozzi, F., et al. (2016). Effects of operational parameters on dark fermentative hydrogen production from biodegradable complex waste biomass. Waste Manage. 50, 55–64. doi: 10.1016/j.wasman.2016.01.044
Ghimire, A., Valentino, S., Frunzo, L., Trably, E., Escudié, R., Pirozzi, F., et al. (2015b). Biohydrogen production from food waste by coupling semi-continuous dark-photofermentation and residue post-treatment to anaerobic digestion: a synergy for energy recovery. Int. J. Hydrogen Energy 40, 16045–16055. doi: 10.1016/j.ijhydene.2015.09.117
Gopala Krishna, G. V., Kumar, P., and Kumar, P. (2008). Treatment of low strength complex wastewater using an anaerobic baffled reactor (ABR). Bioresour. Technol. 99, 8193–8200. doi: 10.1016/j.biortech.2008.03.016
Guo, X. M., Trably, E., Latrille, E., Carre‘re, H., and Steyer, J. P. (2010). Hydrogen production from agricultural waste by dark fermentation: a review. Int. J. Hydrogen Energy 35, 10660–10673. doi: 10.1016/j.ijhydene.2010.03.008
Guo, X. M., Trably, E., Latrille, L., Carrere, H., and Steyer, J.-P. (2014). Predictive and explicative models of fermentative hydrogen production from solid organic waste: role of butyrate and lactate pathways. Int. J. Hydrogen Energy 39, 7476–7485. doi: 10.1016/j.ijhydene.2013.08.079
Karadag, D., Koroglu, O. E., Ozkaya, B., and Cakmakci, M. (2015). A review on anaerobic biofilm reactors for the treatment of dairy industry wastewater. Process Biochem. 50, 262–271. doi: 10.1016/j.procbio.2014.11.005
Khemkhao, M., Techkarnjanaruk, S., and Phalakornkule, C. (2016). Effect of chitosan on reactor performance and population of specific methanogens in a modified CSTR treating raw POME. Biomass Bioenergy 86, 11–20. doi: 10.1016/j.biombioe.2016.01.002
Kinnunen, M., Hilderbrandt, D., Grimberg, S., Rogers, S., and Mondal, S. (2014). Comparative study of methanogens in one- and two-stage anaerobic digester treating food waste. Renewable Agric. Food Syst. 30, 1–9. doi: 10.1017/S1742170514000350
Lee, D. Y., Ebie, Y., Xu, K. Q., Li, Y. Y., and Inamori, Y. (2010). Continuous H2 and CH4 production from high-solid food waste in the two-stage thermophilic fermentation process with the recirculation of digester sludge. Bioresour. Technol. 101, S42–S47. doi: 10.1016/j.biortech.2009.03.037
Lee, W. S., Chua, A. S. M., Yeoh, H. K., and Ngoh, G. C. (2014). A review of the production and applications of waste-derived volatile fatty acids. Chem. Eng. J. 235, 83–99. doi: 10.1016/j.cej.2013.09.002
Lin, C. Y., Wu, S. Y., Lin, P. J., Chang, J. S., Hung, C. H., Lee, K. S., et al. (2011). A pilot-scale high-rate biohydrogen production system with mixed microflora. Int. J. Hydrogen Energy 36, 8758–8794. doi: 10.1016/j.ijhydene.2010.07.115
Liu, D., Liu, D., Zeng, R. J., and Angelidaki, I. (2006). Hydrogen and methane production from household solid waste in the two-stage fermentation process. Water Res. 40, 2230–2236. doi: 10.1016/j.watres.2006.03.029
Liu, Z., Zhang, C., Lu, Y., Wu, X., Wang, L., Wang, L., et al. (2013). States and challenges for high-value biohythane production from waste biomass by dark fermentation technology. Bioresour. Technol. 135, 292–303. doi: 10.1016/j.biortech.2012.10.027
Luongo, V., Ghimire, A., Frunzo, L., Fabbricino, M., D'Antonio, G., Pirozzi, F., et al. (2016). Photofermentative production of hydrogen and poly-β-hydroxybutyrate from dark fermentation products. Bioresour. Technol. 228, 171–175. doi: 10.1016/j.biortech.2016.12.079
Mattei, M. R., D'Acunto, B., Esposito, G., Frunzo, L., and Pirozzi, F. (2015). Mathematical modeling of competition and coexistence of sulfate-reducing bacteria, acetogens, and methanogens in multispecies biofilms. Desalinination Water Treat. 55, 740–748. doi: 10.1080/19443994.2014.937764
Michaud, S., Bernet, N., Buffiere, P., Roustan, M., and Moletta, R. (2002). Methane yield as a monitoring parameter for the start-up of anaerobic fixed film reactors. Water Res. 36, 1385–1391. doi: 10.1016/S0043-1354(01)00338-4
Michelland, R. J., Dejean, S., Combes, S., Fortun-Lamothe, L., and Cauquil, L. (2009). StatFingerprints: a friendly graphical interface program for processing and analysis of microbial fingerprint profiles. Mol. Ecol. Resour. 9, 1359–1363. doi: 10.1111/j.1755-0998.2009.02609.x
Monlau, F., Kaparaju, P., Trably, E., Steyer, J. P., and Carrere, H. (2015). Alkaline pretreatment to enhance one-stage CH4 and two-stage H2/CH4 production from sunflower stalks: mass, energy and economical balances. Chem. Eng. J. 260, 377–385. doi: 10.1016/j.cej.2014.08.108
Nathoa, C., Sirisukpoca, U., and Pisutpaisal, N. (2014). Production of hydrogen and methane from banana peel by two phase anaerobic fermentation. Energy Procedia 50, 702–710. doi: 10.1016/j.egypro.2014.06.086
Okamoto, M., Miyahara, T., Mizuno, O., and Noike, T. (2000). Biological hydrogen potential of materials characteristic of the organic fraction of municipal solid wastes. Water Sci. Technol. 41, 25–32.
Pan, J., Zhang, R., El-Mashad, H. M., Sun, H., and Ying, Y. (2008). Effect of food to microorganism ratio on biohydrogenproduction from food waste via anaerobic fermentation. Int. J. Hydrogen Energy 33, 6968–6975. doi: 10.1016/j.ijhydene.2008.07.130
Pasupuleti, S. B., and Venkata Mohan, S. (2015). Single-stage fermentation process for high-value biohythane production with the treatment of distillery spent-wash. Bioresour. Technol. 189, 177–185. doi: 10.1016/j.biortech.2015.03.128
R Development Core Team (2010). R: A Language and Environment for Statistical Computing. P. Dalgaard (Producer). Vienna: R Foundation for Statistical Computing.
Raheem, A., Hassan, M. Y., and Shakoor, R. (2016). Bioenergy from anaerobic digestion in Pakistan: potential, development and prospects. Renewable Sustain. Energy Rev. 59, 264–275. doi: 10.1016/j.rser.2016.01.010
Regueiro, L., Lema, J. M., and Carballa, M. (2015). Key microbial communities steering the functioning of anaerobic digesters during hydraulic and organic overloading shocks. Bioresour. Technol. 197, 208–216. doi: 10.1016/j.biortech.2015.08.076
Si, B., Liu, Z., Zhang, Y., Li, J., Shen, R., Zhu, Z., et al. (2016). Towards biohythane production from biomass: Influence of operational stage on anaerobic fermentation and microbial community. Int. J. Hydrogen Energy 41, 4429–4438. doi: 10.1016/j.ijhydene.2015.06.045
Turon, V., Trably, E., Fayet, A., Fouilland, E., and Steyer, J.-P. (2015). Raw dark fermentation effluent to support heterotrophic microalgae growth: microalgae successfully outcompete bacteria for acetate. Algal Res. 12, 119–125. doi: 10.1016/j.algal.2015.08.011
Van Lier, J. B., Van der Zee, F. P., Frijters, C. T. M. J., and Ersahin, M. E. (2015). Celebrating 40 years anaerobic sludge bed reactors for industrial wastewater treatment - review paper. Rev. Environ. Sci. Biotechnol. 14, 681–702. doi: 10.1007/s11157-015-9375-5
Wahab, M. A., Habouzit, F., Bernet, N., Jedidi, N., and Escudié, R. (2015). Evaluation of a hybrid anaerobic biofilm reactor treating winery effluents and using grape stalks as biofilm carrier. Environ. Technol. 37, 1676–1682. doi: 10.1080/09593330.2015.1127291
Yeshanew, M. M., Frunzo, L., Lens, P. N., Pirozzi, F., and Esposito, G. (2016a). Mass loss controlled thermal pretreatment system to assess the effects of pretreatment temperature on organic matter solubilization and methane yield from food waste. Front. Environ. Sci. 4:62. doi: 10.3389/fenvs.2016.00062
Yeshanew, M. M., Frunzo, L., Luongo, L., Pirozzi, F., Lens, P. N. L., and Esposito, G. (2016b). Start-up of an anaerobic fluidized bed reactor treating synthetic carbohydrate rich wastewater. J. Environ. Manage. 184(Pt 2), 456–464. doi: 10.1016/j.jenvman.2016.10.001
Yeshanew, M. M., Frunzo, L., Pirozzi, F., Lens, P. N. L., and Esposito, G. (2016c). Production of biohythane from food waste via an integrated system of continuously stirred tank and anaerobic fixed bed reactors. Bioresour. Technol. 220, 312–322. doi: 10.1016/j.biortech.2016.08.078
Keywords: anaerobic biofilm reactor, biohydrogen, dark fermentation, energy recovery, methane, organic fraction of municipal solid waste
Citation: Yeshanew MM, Paillet F, Barrau C, Frunzo L, Lens PNL, Esposito G, Escudie R and Trably E (2018) Co-production of Hydrogen and Methane From the Organic Fraction of Municipal Solid Waste in a Pilot Scale Dark Fermenter and Methanogenic Biofilm Reactor. Front. Environ. Sci. 6:41. doi: 10.3389/fenvs.2018.00041
Received: 28 October 2017; Accepted: 14 May 2018;
Published: 06 June 2018.
Edited by:
Vincenzo Belgiorno, Università degli Studi di Salerno, ItalyReviewed by:
Mohanakrishna Gunda, Flemish Institute for Technological Research (VITO), BelgiumDaniel Puyol, Universidad Rey Juan Carlos, Spain
Copyright © 2018 Yeshanew, Paillet, Barrau, Frunzo, Lens, Esposito, Escudie and Trably. This is an open-access article distributed under the terms of the Creative Commons Attribution License (CC BY). The use, distribution or reproduction in other forums is permitted, provided the original author(s) and the copyright owner are credited and that the original publication in this journal is cited, in accordance with accepted academic practice. No use, distribution or reproduction is permitted which does not comply with these terms.
*Correspondence: Martha M. Yeshanew, martaminale@gmail.com