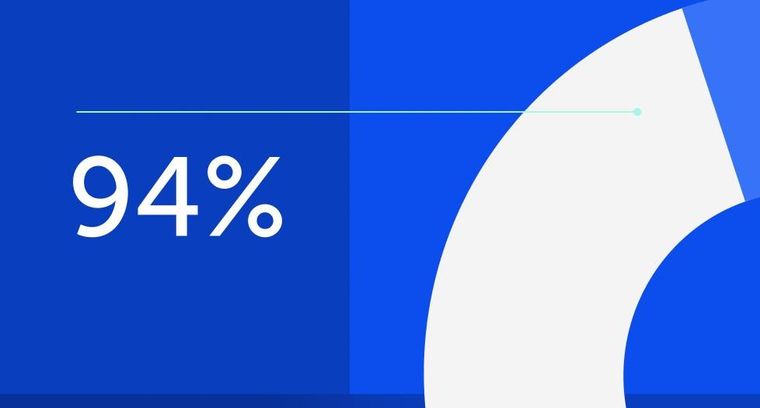
94% of researchers rate our articles as excellent or good
Learn more about the work of our research integrity team to safeguard the quality of each article we publish.
Find out more
REVIEW article
Front. Environ. Sci., 07 May 2018
Sec. Microbiotechnology
Volume 6 - 2018 | https://doi.org/10.3389/fenvs.2018.00024
Arsenic (As) contamination is a serious issue throughout the world. The scale of problem is being realized to be even greater with the discovery of new As contaminated regions with time. Rice is a staple crop across the world with approximately half of the world population dependent on rice for their daily dietary intake especially in Southeast Asian countries. It is not only the consumption of rice grains but also food products based on rice, which contribute toward As exposure to humans. Plant growth promoting microorganisms (PGPMs) constitute a diverse group of microorganisms including bacteria, fungi and microalgae. These are associated with the rhizospheric zone of plants. They improve plant growth through different mechanisms like increase of nutrients level in plants, improved soil quality, siderophore and hormone production, changes in biochemical properties of plants etc. Another important assistance imparted by PGPMs is the altered speciation of As in the soil through methylation and subsequent change in the bioavailability of As to the plants. Further, a change in As speciation also affects As uptake and transport in plants. The purpose of this review is to discuss importance of PGPM association in As toxicity amelioration in plants along with favorably reducing As concentrations in crop plants or increasing As accumulation in phytoremediator plants. This review also presents mechanisms of action of PGPMs and describes both laboratory- and field-studies on the application of PGPMs for tackling As-contamination. The future prospects of successful utilization of PGPMs are also discussed.
Arsenic (As) contamination in soil and groundwater has become a serious health and environmental concern worldwide especially in south and Southeast Asia. Natural biogeochemical processes are considered to be primarily responsible for As contamination of groundwater in South and Southeast Asia (Srivastava et al., 2012; Rodríguez-Lado et al., 2013; Podgorski et al., 2017). Millions of people are at risk of As poisoning through food especially rice and rice based products (Meharg and Rahman, 2003; Awasthi et al., 2017). Rice is renowned for more efficient As accumulation in comparison to other crops. This is due to the presence of As predominantly in the form of arsenite [As(III)] in anaerobic rice field conditions and transport of As(III) via highly expressed silicic acid transporters in rice (Srivastava et al., 2012). Other crops like wheat, maize, Indian mustard are grown aerobically leading to abundance arsenate [As(V)] in field. And, the uptake and transport of As(V) occurs through phosphate transporters that is subjected to strong competition with phosphate. Nevertheless, other crop plants (wheat, maize) and vegetables (tuber, leaf, fruit) also act as sources of As. Humans exposed to As for prolonged durations, ranging up to the lifetime, can have severe effects on proper functioning of various tissues and organs including gastrointestinal tract, liver, skin, kidney, neurological system etc. The most prominent visible signs of chronic toxicity of As (known as arsenicosis) are skin related symptoms viz., hyperkeratosis, hyperpigmentation and skin cancers. This is because skin has high keratin levels that has sulfhydryl groups (-SH) and reduced form of As, arsenite [As(III)], binds strongly to—SH groups (Duker et al., 2005). Arsenic toxicity can also cause epigenetic changes and induce cancers of other organs e.g., liver, kidney, and bladder (Abdul et al., 2015). Animals too can be affected by As through ingestion via water and fodder and can in turn act as source of As to subsequent species in the food chain. Cow milk, poultry, fish, etc. have been found to be contaminated with As (Datta et al., 2012). Arsenic exposure to plants for long time inhibits their growth and development, leading to either death of plants or to poor yield and quality of crops. Various tissue systems and physiological functions of plants are influenced by As including metabolism of major elements (e.g., nitrogen, carbon, sulfur; Jha and Dubey, 2004; Pathare et al., 2013) energy and redox homeostasis (Srivastava et al., 2013b), photosynthesis and respiration (Chen et al., 2014), water uptake, and transport (Srivastava et al., 2013a) etc. The biochemical and molecular basis of As toxicity in both plants and humans include phosphate replacement via As(V) in biomolecules, reaction of —SH groups in proteins with As(III) (Rosen et al., 2011), increase in production of reactive oxygen species (ROS) (Srivastava et al., 2007), changes in expression and activity profile of several proteins and enzymes (Requejo and Tena, 2006; Norton et al., 2008; Srivastava et al., 2015). The sources of As to plants and humans and toxicity responses are depicted in Figure 1.
Figure 1. The figure represents the presence of As in soil, water, and air and the entry routes of As to plants, animals and humans via irrigation, volcanic eruptions, mining and industrial activities, microbial processes and rain. Once inside plants and humans, the toxicity mechanisms include elemental interactions, reactive oxygen species (ROS) production, oxidative stress induction, redox and energy imbalance, and epigenetic, transcriptional and proteomic changes. Prolonged exposure to As leads to diminished growth, and yield in plants and to various medical problems, including cancers in humans. (As values for soil, inland water, ocean, and atmosphere and irrigation water taken from Neumann et al. (2011) and Sohn (2014).
Arsenic occurs in the environment in inorganic [arsine (As−3), elemental arsenic (As0), As(III), and As(V)] and organic forms [dimethylarsinic acid (DMA), monomethylarsonic acid (MMA), trimethylarsine oxide (TMAO), arsenobetaine etc.]. It has been documented that bacteria, fungi, algae and even humans can methylate arsenite [As(III)] to methylated species. Arsenic methylation is catalyzed by homologs of As(III) S-adenosylmethionine (SAM) methyltransferases genes (Yang and Rosen, 2016). Nearly all microbes show resistance to As(III) and arsenate [As(V)] and exhibit the potential to transform As into volatile arsine gases, namely arsine (AsH3), monomethylarsine (MeAsH2), dimethylarsine (Me2AsH), and trimethylarsine (TMA) (Páez-Espino et al., 2009). Fungi including Aspergillus, Candida, Scopulariopsis, Penicillium, Fusarium, and Trichoderma can also methylate inorganic As species to organic ones (Cullen and Reimer, 1989; Bentley and Chasteen, 2002). Arsines are toxic As species. However, it must be noted the potential of microbes for As methylation and volatilization depends on soil chemistry, As level and organic matter (Mestrot et al., 2011). The As biovolatilization from paddy fields has been found to range from 0.002 to 0.13% of the total As in a year with As volatilization rate of about 4 μg kg−1 yr−1 (Mestrot et al., 2011).
The situation demands development of affordable, environment friendly and sustainable options for farmers to grow low grain As containing rice plants (Olmeta-Schult et al., 2018). At the same time, to harness phytoremediation prospects, a cost-effective solar driven technology, the efficiency of plants for removing the contaminant needs to be enhanced. In this regard, As-resistant plant growth promoting microorganisms (PGPMs) might be envisioned as safe, low-cost, promising, and sustainable biological tools for mitigating As toxicity in plants and for regulating As accumulation in crop and/or phytoremediator plants (Vejan et al., 2016). If suitable PGPM based strategies become successful, this would provide additional benefits in terms of reduced chemical fertilizers consumption, cost minimization, and environmental protection.
Rhizospheric interactions between plants and microorganisms play a crucial role in growth of plants, and in nutrient uptake and transport. Several studies have shown that plant's adaptation to local environmental stress is closely related to microbiota present in their surroundings (Vacheron et al., 2013). Roots secrete secondary metabolites, which not only activate the movement of microbes toward itself but also nourish them (Lugtenberg and Kamilova, 2009). The organic metabolites include amino acids, fatty acids, nucleotides, organic acid, phenolics, putrescene, sterols, sugars, and vitamins. PGPMs have many plant growth promoting traits and minimize the toxic effects of abiotic and biotic stress including heavy metals (Ma et al., 2016). Microbes possessing both As resistance and plant growth promoting properties provide tolerance to the plants through several mechanisms that can be direct or indirect (Figure 2). Indirect mechanisms include prevention of phytopathogens to promote the plant growth. Direct mechanisms include effect on the bioavailability of As to plants (through secretion of protons, organic acids, redox reaction, metabolic reactions) and chemical form of As (reduction, oxidation, methylation, demethylation), effect on As interactions with other elements like Fe, Si, etc., alteration of plant growth (by indole-3-acetic acid (IAA) and 1-aminocyclopropane-1-carboxylate (ACC) deaminase production, extracellular enzymes, nitrogen fixation, extracellular polysaccharides) (Khan et al., 2010; Vacheron et al., 2013; Rashid et al., 2016; Karthik et al., 2017; Kong and Glick, 2017; Olanrewaju et al., 2017; Gouda et al., 2018). Phosphate is a crucial element for achieving optimal growth of plants and the bioavailability of phosphate in most soils is very low. Similarly, great amount of nitrogen inputs are required to maintain good growth of plants. Another detrimental factor to plant growth is low soil organic carbon. PGPMs have been found to improve N, P, and K nutrition of plants and improve soil quality through enhancement of soil organic matter as well as by promoting aggregation of soil particles and improving soil properties (Khan et al., 2010; Rashid et al., 2016).
Figure 2. Schematic representation of mechanisms of growth promotion and arsenic tolerance imparted to plants by plant growth promoting microorganisms (PGPMs).
Phytohormones such as auxin, ethylene, gibberellin, and cytokinins are important for improved plant growth (Dimkpa et al., 2008; Kong and Glick, 2017). Indole-3-acetic acid (IAA) is the most studied, and the most common natural auxin found in plants. It stimulates root and xylem development; controls vegetative growth, initiates formation of both lateral and adventitious roots and also affects pigment formation, photosynthesis, and resistance to stressful conditions (Spaepen and Vanderleyden, 2011). Ethylene regulates nutrient and water uptake, promotes root initiation, and stimulates seed germination and synthesis of other plant hormones (Thao et al., 2015). Cytokinins and gibberellins produced by PGPRs also help in promotion of plant growth (Etesami, 2018).
Siderophores are the low molecular weight high-affinity iron (Fe)-chelating ligands (stability constants 1012 to 1052) with side chains and functional groups (Crosa and Walsh, 2002). They are grouped into three families: hydroxamates, catecholates, and carboxylates. These compounds are basically produced under Fe-limiting conditions and enhance Fe-uptake in form of ferric ions (Fe3+). The research on siderophore production has gained momentum in the last decade due to their unique characteristic to extract Fe in form of Fe3+ (Saha et al., 2015). Among all the siderophores, hydroxamate, desferrioxamine B (DFOB) is easily available and therefore, the most researched one. The microbial siderophores are one of the PGP attributes that are used by plants in form of Fe3+-siderophores complex (Johnstone and Nolan, 2015). As-bioavailability especially in rice fields is related to water management and Fe-As cycling by microbial colonies inhabiting the rhizospheric zone of the rice plants. Further, Fe forms specific iron plaque on rice roots that can bind As strongly on root surface itself and modulate As bioavailability (Garnier et al., 2010). The siderophore produced by heavy metal tolerant bacteria can also reduce the bioavailability and toxicity of heavy metals like As, Pb, Cd, and Hg by precipitating free metal ions with siderophores (Etesami, 2018).
Soil contains a plethora of diverse class of microorganisms, including bacteria, fungi, actinomycetes, protozoa, and algae. PGPMs comprise of a variety of microorganisms like bacteria, cyanobacteria, fungi including arbuscular mycorrhizal (AM) fungi (Mishra et al., 2017). Microorganisms play a crucial role in biogeochemical cycle of As through bioaccumulation and biotransformation (Yang and Rosen, 2016). Bacteria (Rhizobium, Frankia, Klebsiella, Clostridium, Bacillus, Pseudomonas, and Arthrobacter) constitute important PGPMs and impart several benefits including crucial processes like nitrogen fixation by rhizobia (Checcucci et al., 2017). Algae are a diverse group of eukaryotic organisms having the ability for oxygenic photosynthesis. Anabena, Nostoc, Chlorella, Dunaliella, Desmodesmus are some of best examples of microalgae under PGPMs. Generally microalgae can adsorb As on their surface and with time As penetrates the cell membrane and enters into cell. The mechanism of As metabolism in marine algae is reduction and oxidation of As species, As methylation and transformation into arsenosugars (Duncan et al., 2015; Wang et al., 2015). Fungi are saprophytic and ubiquitous microorganisms that can decompose organic matter and help in nutrient cycling in soil. A number of fungi have been employed to remediate As. Some of them include Aspergillus flavus (Maheswari and Murugesan, 2011), Alcaligenes sp. (Yoon et al., 2009), Thiomonas sp. (Duquesne et al., 2007), and Trichoderma sp. (Tripathi et al., 2017). Among them Trichoderma is most extensively studied fungus. Fungi transform As species from inorganic to organic forms and may finally transform As to volatile species. Apart from rhizospheric fungi, arbuscular mycorrhizal fungi (AMF) constitute an important group. AM fungi viz., Rhizophagus, Glomus exist in symbiotic association with plants and this associated is based on mutual benefits (Li et al., 2016). The AM fungi take photosynthetic sugar products from plants and provide in return mineral nutrients and water (Poonam et al., 2017). The PGPM to be used for the purpose of amelioration of As stress and regulation of As accumulation must be itself tolerant to As stress (Armendariz et al., 2015). Further, plant–microbes interaction can be even more fruitful if a combination of different microorganisms can be utilized (Shukla and Srivastava, 2017).
A number of studies showcasing successful utilization of PGPMs for regulating the accumulation of As in plants and for improving the tolerance and growth of plants are performed (Table 1). This section discusses a few recent studies.
Table 1. A summary of recent reports on the utilization of PGPMs for the amelioration of As stress and for regulating As accumulation in plants.
Mallick et al. (2018) isolated two As resistant bacteria from rhizosphere of mangrove plants in Sunderban area, Kocuria flava and Bacillus vietnamensis. Both strains improved growth and decreased As in rice plants. As tolerant microbes like B. licheniformis, Micrococcus luteus, Pseudomonas fluorescens were found to possess siderophore producing, phosphate solubilizing as well as nitrogen fixing properties in a study by Ivan et al. (2017). Out of these, M. luteus inoculation imparted As tolerance to grapevine with increased biomass and antioxidant potential (Ivan et al., 2017). Zhang et al. (2017) genetically engineered Rhizobium leguminosarum with As(III) S-adenosylmethionine methyltrasnferase gene from Chlamydomonas reinhardtii (CrarsM). They found legume symbiont to be able to methylate As(III) to various As species. The red clover plants grown in symbiotic association with recombinant R. leguminosarum showed presence of up to 42.4% of methylated As species along with volatilization of 0.01–0.02% of total As. In a study, Pteris vittata plants were grown on Fe-free medium containing a catecholate type siderophore from Pseudomonas PG12 strain. Fe and As were supplied in the form of mineral, FeAsO4. It was found that Fe dissolution was effectively performed in presence of PG12 siderophore and resulted in increase in Fe and As (14.3–78.5 mg kg−1 in fronds) and this was accompanied with increase in biomass of plants (Liu et al., 2015).
Mesa et al. (2017) explored the possibilities of enhancing phytoremediation efficiency of Betula celtiberica trees at a contaminated site in Spain. They isolated, cultured and evaluated 54 rhizobacteria and 41 root endophytes associated with B. celtiberica and selected total four strains for detailed lab and field studies (Mesa et al., 2017). It was found that endophytic strains (Variovorax paradoxus, Phyllobacterium myrsinacearum) caused an increase in As accumulation in B. celtiberica, while the rhizospheric strain Ensifer adhaerens promoted plant growth. It has been reported that Brevundimonas diminuta NBRI012 is an As resistant IAA producing rhizobacteria, which can alleviate the negative effects of As in rice and improve the growth of the rice (Singh et al., 2016). Singh et al. (2015) analyzed As resistant bacterial strains from paddy soil as Bacillus altitudinis, B. megaterium, and Lysinibacillus sp. strain SS11. Lysinibacillus strain was found to tolerate up to 3,256 mg L−1 As(V) and 1,136 mg L−1 As(III). Further, it was found that As accumulation potential of P. vittata plants increased in presence of microbial inoculants as compared to that in their absence (Singh et al., 2015).
Lampis et al. (2015) demonstrated an increase in biomass of P. vittata plants (up to 45%) as well as total As removal (from 13 to 35%) when inoculated with siderophore and IAA-producing bacterial strains viz., Pseudomonas sp., Delftia sp., Bacillus sp., Variovorax sp., and Pseudoxanthomonas sp. Das et al. (2014) identified 12 potential As resistant bacterial isolates from agricultural soils of Taiwan. Out of these As(III) oxidizing ability was found in bacteria belonging to Pseudomonas, Acinetobacter, Klebsiella, and Comamonas. Various strains of Pseudomonas sp., Geobacillus sp., Bacillus sp., Paenibacillus sp., Enterobacter sp. and Comamonas sp. also possessed PGP properties. Shagol et al. (2014) have also identified potential As tolerant and plant growth promoting bacterial strains from a metal contaminated site in South Korea and found that three strains (Rhodococcus aetherivorans JS2210, Pseudomonas oreensis JS2214, and Pseudomonas sp. JS238) could induce growth of roots of maize in response to As(V) stress. Wang et al. (2012) isolated As tolerant bacterial strains from the rhizosphere of P. vittata that included a bacterium capable of As(III) oxidation (Acinetobacter) while four bacterial strains having potential both for As(V) reduction and As(III) oxidation (Comamonas, Flavobacterium, Pseudomonas, and Staphylococcus). Likewise, Agrobacterium radiobacter D14 was used with Populus deltoids and it was found that plants could tolerate even 300 mg Kg−1 As in soil and showed 54% As removal that was more than that observed in absence of bacterium (43%). In addition, the translocation of As to shoots was also increased (Wang et al., 2011).
Arbuscular mycorrhizal fungi (AMF) are found in approximately 80% of all plant species (Chen et al., 2017). AM fungi facilitate mainly the phosphate uptake in plants, but their role in imparting stress tolerance is also well-demonstrated. Sharma et al. (2017) compared the potential of AM fungi Rhizoglomus intraradices and Glomus etunicatum for amelioration of As stress in wheat (Triticum aestivum). As stress affected the percentage of root colonization of both fungal inoculants still both mycorrhiza inoculated wheat plants exhibited better growth as compared to NM plants. Mycorrzhiza inoculation also assisted in fighting the As-induced P deficiency and thus maintained the P:As ratio and in decreasing As translocation in low As treatment (25 mg kg−1 soil). Spagnoletti et al. (2016) studied the responses of AMF (R. intraradices)-soybean plants system in As-contaminated soils and found increase in biomass of plants along with decline in As concentration. Molecular investigation suggested upregulation of RiPT, a high-affinity phosphate transporter of AMF, and RiArsA, a putative As efflux pump. Li et al. (2016) inoculated the R. intraradices to six varieties of rice to evaluate the response of AMF colonization. AMF colonization showed decline in inorganic/organic As ratio in grains of all six rice varieties. In study conducted by Wu et al. (2015) on upland rice variety (Zhonghan 221), three strains of Glomus fungi (Glomus geosporum, Glomus versiforme, and Glomus mosseae) were utilized for As stress amelioration. This study also advocated the positive effect of AMF colonization on rice crop including higher grain yield without increasing As in grain. Another aspect of AMF-plant symbiosis is the involvement of 14-3-3 proteins, which affect several functions by interactions with crucial proteins. The 14-3-3 proteins can also affect phosphate uptake and transport processes in the plants. A recent study on rice plants inoculated with AM fungi (R. irregularis) showed an upregulation of 14-3-3 genes under AMF colonization (Pathare et al., 2016) indicating their possible involvement in AMF-mediated As toxicity amelioration in rice plants.
Chan et al. (2013) tested a combination approach utilizing three Glomus species (G. geosporum, G. mosseae, G. versiforme) for modulating As stress in rice. They found AMF combination to enhance phosphate uptake and to reduce As translocation in rice grains. Garg and Singla (2012) assessed the effect of As stress on Pisum sativum under the symbiotic interaction with G. mosseae. A concentration dependent increase in As uptake and accumulation and a decline in dry biomass of plants was observed. The AMF inoculation was found to tackle As stress with decrease in As(V) uptake, increase in plant growth and N, P, K levels.
Trichoderma is a filamentous fungi belonging to class Ascomycetes and is an extensively studied important PGPM (Waghunde et al., 2016). Trichoderma sp. are excellent plant growth promoter, which improve soil fertility and has capacity to impart stress tolerance, possibly due to its rhizospheric competence with other organisms. It can induce hormone production, nutrients release from soil, and enhance development of root system (de Souza et al., 2015). They have a variety of functional groups on surface to bind with metals (Congeevarama et al., 2007; Tripathi et al., 2017).
Tripathi et al. (2017) compared As tolerant and sensitive strains of Trichoderma sp. viz., M-35 and PPLF-28, respectively, for As(V) toxicity amelioration in chickpea plants. Although total As was not affected by two strains, induced transformation of iAs to organic As was noticed upon inoculation with tolerant strains as compared to sensitive ones and this effect was correlated to improved growth and nutrient content in plants. Other anatomical and molecular analyses also suggested grater As stress ameliorative potential of tolerant strain. Another strain of Trichoderma, T. reesei NBRI0716 was found to alter As speciation (66% decline in inorganic As and more DMA and MMA) and improve grain yield and quality (amino acids and mineral content) of chick pea plants when grown in soil amended with As (100 mg kg−1) (Tripathi et al., 2013). It was also found that this strain could also restore other growth deformities like reduced trichome density and turgidity, nodule formation, chlorophyll content, and also up-regulated the expression of stress responsive genes and proline (Tripathi et al., 2013). Su et al. (2017) used chlamydospores of Trichoderma asperellum for improved stability of fungus application in contaminated sites. They tested these chlamydospores for As toxicity amelioration in Ipomoea aquatic and found promising results in terms of improved growth and increased As content of plants. Hence, they suggested potential application of such chlamydospores for enhancing phytoremediation potential of plants. Earlier, Caporale et al. (2014) also studied two Trichoderama strains, T. harzianum and T. atroviride for As stress amelioration in lettuce plants and found decline in As level and growth improvement along with improved P status.
Srivastava et al. (2011) isolated 15 fungal strains from As contaminated (9.45–15.65 mg kg−1) soils of West Bengal, which belonged to Aspergillus, Trichoderma, Neocosmospora, Rhizopus, Sordaria, Penicillium and sterile mycelial strain. Fungal biomass of ten strains could remove As (10.92–65.81%) from the medium containing 10 mg L−1 As; of this about 3.71–29.86% was calculated to be biovolatilized As. Later, they used 4 As tolerant strains for As tolerance of rice and pea (Srivastava et al., 2011). It was found that plants grown in fungal inoculated soils had improved growth. Westerdykella and Trichoderma were the better performing isolates than Rhizopus and Lasiodiplodia. Plant growth increase varied from 16 to 293% in inoculated soil.
Endophytic fungi, Piriformospora indica protects rice plants from As toxicity by not only reducing the As availability in the plant environment but also by restricting As in colonized roots through immobilization of into insoluble particulate matter. This fungus also modulates antioxidant responses of plants to ameliorate As stress (Mohd et al., 2017). Verma et al. (2016) isolated an arsenic methyltransferase (WaarsM) gene from a soil fungus, Westerdykella aurantiaca and expressed the gene in S. cerevisiae (Δacr2). The WaarsM expressing yeast cells (Δacr2) cells showed increased As methylation potential with 2.2 ppm and 0.58 ppm volatile arsenicals upon exposure to 20 ppm As(V) and 2 ppm As(III), respectively. Further laboratory studies also demonstrated an increase in As tolerance of rice plants, when WaarsM expressing yeast cells were co-cultured with rice plants.
Use of microalgae or algal biomass for bioremediation of heavy metal is an efficient, eco-friendly, and cost effective tool. Algal species are able to minimize the toxicity of heavy metals by biosorption. Biosorption potential of algae depends on the presence of various functional groups (e.g., imidazole, carboxyl, phosphoryl, sulphuryl, hydroxyl, amine, sulfate, etc.) on cell walls (Kaplan, 2013). The production of PCs is an important process of As detoxification through complexation of As (Munoz et al., 2014). Reduced and oxidized PCs act as redox buffer to prevent oxidative damage inside the cell.
The inoculation of two alga, Chlorella vulgaris and Nannochloropsis sp. was recently compared in rice plants against As toxicity by Upadhyay et al. (2016). The toxic effects of As on rice plant growth were ameliorated by algal inoculation while at the same time significantly reducing As concentration in both roots and shoots. Other than As metabolism, algae also show involvement of GSH and PCs in As detoxification processes. It has been noted that As exposure induces the production of PCs in algal cells and a variety of As-SH complexes have been reported in alga like Stichococcus bacillaris (Pawlik-Skowronska et al., 2004). Tang et al. (2016) developed transgenic plants of A. thaliana with expression of As(III)-S-adenosylmethyltransferase (arsM) gene from C. reinhardtii. The transgenic plants showed ability to transform most of the inorganic As into DMA(V) in shoots and even into volatile As species. However, algal utilization in As stress amelioration in plants is yet largely unexplored.
Sustainable technologies need to be developed in future both for safe agricultural production in As contaminated environments and for remediation of the contaminated sites. Arsenic resistant and PGPMs offer a great hope in this regard. The work performed in this area suggests immense potential for PGPMs for safe rice cultivation with low As accumulation in grains. At the same time, there are PGPMs that can increase As accumulation in phytoremeidator plants like P. vittata. Hence, it would not be an exaggeration that the future belongs to PGPMs mediated regulation of As concentrations and As toxicity amelioration in plants. However, extensive researches would be required to advance this technology viz., (1) to standardize PGPM based strategy for different environments, (2) to identify potential combinations of PGPMs of a particular group of organisms (e.g., bacteria), (3) to hunt for PGPMs combinations of diverse groups (e.g., bacteria-fungi, fungi-algae, bacteria-AMF, bacteria-fungi-algae, etc.) and (4) to gain deeper insights into the mechanisms of actions of PGPMs. The developed strategy should also be economically lucrative so that public and farmers' participation may be ensured.
SS conceptualized the review; MU, PY, AS, and SS wrote the review; SS did final editing of the MS.
The authors declare that the research was conducted in the absence of any commercial or financial relationships that could be construed as a potential conflict of interest.
The reviewer GS and handling editor declared their shared affiliation.
This research was supported by a grant from Science & Engineering Research Board (SERB), India (YSS/2014/000080). PY is thankful to SERB for project fellowship. MU and AS are thankful to Banaras Hindu University for providing fellowship.
Abdul, K. S., Jayasinghe, S. S., Chandana, E. P., Jayasumana, C., and De Silva, P. M. (2015). Arsenic and human health effects: a review. Environ. Toxicol. Pharmacol. 40, 828–846. doi: 10.1016/j.etap.2015.09.016
Alcántara-Martínez, N., Figueroa-Martínez, F., Rivera-Cabrera, F., Gutiérrez-Sánchez, G., and Volke-Sepúlveda, T. (2018). An endophytic strain of Methylobacterium sp. increases arsenate tolerance in Acacia farnesiana (L.) Willd: a proteomic approach. Sci. Total Environ. 625, 762–774. doi: 10.1016/j.scitotenv.2017.12.314
Armendariz, A. L., Talano, M. A., Oller, A. L., Medina, M. I., and Agostini, E. (2015). Effect of arsenic on tolerance mechanisms of two plant growth-promoting bacteria used as biological inoculants. J. Environ. Sci. 33, 203–210. doi: 10.1016/j.jes.2014.12.024
Awasthi, S., Chauhan, R., Dwivedi, S., Srivastava, S., Srivastava, S., and Tripathi, R. D. (2018). A consortium of alga (Chlorella vulgaris) and bacterium (Pseudomonas putida) for amelioration of arsenic toxicity in rice: a promising and feasible approach. Environ. Exp. Bot. 150, 115–126. doi: 10.1016/j.envexpbot.2018.03.001
Awasthi, S., Chauhan, R., Srivastava, S., and Tripathi, R. D. (2017). The journey of arsenic from soil to grain in rice. Front. Plant Sci. 8:1007. doi: 10.3389/fpls.2017.01007
Bentley, R., and Chasteen, T. G. (2002). Microbial methylation of metalloids: arsenic, antimony, and bismuth. Microbiol. Mol. Biol. Rev. 66, 250–271. doi: 10.1128/MMBR.66.2.250-271.2002
Caporale, A. G., Sommella, A., Lorito, M., Lombardi, N., Azam, S. M., Pigna, M., et al. (2014). Trichoderma spp. alleviate phytotoxicity in lettuce plants (Lactuca sativa L.) irrigated with arsenic-contaminated water. J. Plant Physiol. 171, 1378–1384. doi: 10.1016/j.jplph.2014.05.011
Chan, W. F., Li, H., Wu, F. Y., Wu, S. C., and Wong, M. H. (2013). Arsenic uptake in upland rice inoculated with a combination or single arbuscular mycorrhizal fungi. J. Hazard. Mater. 262, 1116–1122. doi: 10.1016/j.jhazmat.2012.08.020
Checcucci, A., DiCenzo, G. C., Bazzicalupo, M., and Mengoni, A. (2017). Trade, diplomacy, and warfare: the quest for elite rhizobia inoculant strains. Front. Microbiol. 8:2207. doi: 10.3389/fmicb.2017.02207
Chen, W., Taylor, N. L., Chi, Y., Millar, A. H., Lambers, H., and Finnegan, P. M. (2014). The metabolic acclimation of Arabidopsis thaliana to arsenate is sensitized by the loss of mitochondrial Lipoamide Dehydrogenase2, a key enzyme in oxidative metabolism. Plant Cell Environ. 37, 684–695. doi: 10.1111/pce.12187
Chen, X. W., Wu, F. Y., Li, H., Chan, W. F., Wu, S. C., and Wong, M. H. (2017). Mycorrhizal colonization status of lowland rice (Oryza sativa L.) in the southeastern region of China. Environ. Sci. Pollut. Res. 24, 5268–5276. doi: 10.1007/s11356-016-8287-4
Congeevarama, S., Dhanarani, S., Park, J., Dexilin, M., and Thamaraiselui, K. (2007). Biosorption of chromium and nickel by heavy metal resistant fungal and bacterial isolates. J. Hazard. Mater. 146, 270–277. doi: 10.1016/j.jhazmat.2006.12.017
Crosa, J. H., and Walsh, C. T. (2002). Genetics and assembly line enzymology of siderophore biosynthesis in bacteria. Microbiol. Mol. Biol. Rev. 66, 223–249. doi: 10.1128/MMBR.66.2.223-249.2002
Cullen, W. R., and Reimer, K. J. (1989). Arsenic speciation in the environment. Chem. Rev. 89, 713–764. doi: 10.1021/cr00094a002
Das, J., and Sarkar, P. (2018). Remediation of arsenic in mung bean (Vigna radiata) with growth enhancement by unique arsenic-resistant bacterium Acinetobacter lwoffii. Sci. Total Environ. 624, 1106–1118. doi: 10.1016/j.scitotenv.2017.12.157
Das, S., Jean, J. S., Chou, M. L., Rathod, J., and Liu, C. C. (2016). Arsenite-oxidizing bacteria exhibiting plant growth promoting traits isolated from the rhizosphere of Oryza sativa L.: implications for mitigation of arsenic contamination in paddies. J. Hazard. Mater. 302, 10–18. doi: 10.1016/j.jhazmat.2015.09.044
Das, S., Jean, J. S., Kar, S., Chou, M. L., and Chen, C. Y. (2014). Screening of plant growth-promoting traits in arsenic-resistant bacteria isolated from agricultural soil and their potential implication for arsenic bioremediation. J. Hazard. Mater. 272, 112–120. doi: 10.1016/j.jhazmat.2014.03.012
Datta, B. K., Bhar, M. K., Patra, P. H., Majumdar, D., Dey, R. R., Sarkar, S., et al. (2012). Effect of environmental exposure of arsenic on cattle and poultry in Nadia district, West Bengal, India. Toxicol. Int. 19, 59–62. doi: 10.4103/0971-6580.94511
de Souza, R., Ambrosini, A., and Passaglia, L. M. (2015). Plant growth-promoting bacteria as inoculants in agricultural soils. Genet. Mol. Biol. 38, 401–419. doi: 10.1590/S1415-475738420150053
Dimkpa, C., Svatoš, A., Merten, D., Büchel, G., and Kothe, E. (2008). Hydroxamate siderophores produced by Streptomyces acidiscabies E13 bind nickel and promote growth in cowpea (Vigna unguiculata L.) under nickel stress. Can J. Microbiol. 54, 163–172. doi: 10.1139/w07-130
Duker, A. A., Carranza, E. J., and Hale, M. (2005). Arsenic geochemistry and health. Environ. Int. 31, 631–641. doi: 10.1016/j.envint.2004.10.020
Duncan, E. G., Maher, W. A., and Foster, S. D. (2015). Contribution of arsenic species in unicellular algae to the cycling of arsenic in marine ecosystems. Environ. Sci. Technol. 49, 33–50. doi: 10.1021/es504074z
Duquesne, K., Lieutaud, A., Ratouchniak, J., Yarzabal, A., and Bonnefoy, V. (2007). Mechanisms of arsenite elimination by Thiomonas sp. isolated from Carnoules acid mine drainage. Eur. J. Soil Biol. 43, 351–355. doi: 10.1016/j.ejsobi.2007.03.010
Etesami, H. (2018). Bacterial mediated alleviation of heavy metal stress and decreased accumulation of metals in plant tissues: mechanisms and future prospects. Ecotoxicol. Environ. Saf. 147, 175–191. doi: 10.1016/j.ecoenv.2017.08.032
Garg, N., and Singla, P. (2012). The role of Glomus mosseae on key physiological and biochemical parameters of pea plants grown in arsenic contaminated soil. Sci. Hort. 143, 92–101. doi: 10.1016/j.scienta.2012.06.010
Garnier, J. M., Travassac, F., Lenoble, V., Rose, J., Zheng, Y., Hossain, M. S., et al. (2010). Temporal variations in arsenic uptake by rice plants in Bangladesh: the role of iron plaque in paddy fields irrigated with groundwater. Sci. Total Environ. 408, 4185–4193. doi: 10.1016/j.scitotenv.2010.05.019
Gouda, S., Kerry, R. G., Das, G., Paramithiotis, S., Shin, H. S., and Patra, J. K. (2018). Revitalization of plant growth promoting rhizobacteria for sustainable development in agriculture. Microbiol. Res. 206, 131–140. doi: 10.1016/j.micres.2017.08.016
Govarthanan, M., Mythili, R., Selvankumar, T., Kamala-Kannan, S., and Kim, H. (2018). Myco-phytoremediation of arsenic- and lead-contaminated soils by Helianthus annuus and wood rot fungi, Trichoderma sp. isolated from decayed wood. Ecotoxicol. Environ. Saf. 151, 279–284. doi: 10.1016/j.ecoenv.2018.01.020
Ivan, F. P., Salomon, M. V., Berli, F., Bottini, R., and Piccoli, P. (2017). Characterization of the As(III) tolerance conferred by plant growth promoting rhizobacteria to in vitro-grown grapevine. Appl. Soil Ecol. 109, 60–68. doi: 10.1016/j.apsoil.2016.10.003
Jha, A. B., and Dubey, R. S. (2004). Carbohydrate metabolism in growing rice seedlings under arsenic toxicity. J. Plant Physiol. 161, 867–872. doi: 10.1016/j.jplph.2004.01.004
Johnstone, T. C., and Nolan, E. M. (2015). Beyond iron: non-classical biological functions of bacterial siderophores. Dalton Trans. 44, 6320–6329. doi: 10.1039/C4DT03559C
Kaplan, D. (2013). “Absorption and adsorption of heavy metals by microalgae,” in Handbook of Microalgal Culture: Applied Phycology and Biotechnology, 2nd Edn, eds A. Richmond, and Q. Hu (Oxford: John Wiley & Sons Ltd), 602–611.
Karthik, C., Elangovan, N., Kumar, T. S., Govindharaju, S., Barathi, S., Oves, M., et al. (2017). Characterization of multifarious plant growth promoting traits of rhizobacterial strain AR6 under chromium (VI) stress. Microbiol. Res. 204, 65–71. doi: 10.1016/j.micres.2017.07.008
Khan, M. S., Zaidi, A., Ahemad, M., Oves, M., and Wani, P. A. (2010). Plant growth promotion by phosphate solubilizing fungi – current perspective. Arch. Agron. Soil Sci. 56, 73–98. doi: 10.1080/03650340902806469
Kong, Z., and Glick, B. R. (2017). The role of plant growth-promoting bacteria in metal phytoremediation. Adv. Microbiol. Physiol. 71, 97–132. doi: 10.1016/bs.ampbs.2017.04.001
Lampis, S., Santi, C., Ciurli, A., Andreolli, M., and Vallini, G. (2015). Promotion of arsenic phytoextraction efficiency in the fern Pteris vittata by the inoculation of As-resistant bacteria: a soil bioremediation perspective. Front. Plant Sci. 6:80. doi: 10.3389/fpls.2015.00080
Li, H., Chen, X., and Wong, M. (2016). Arbuscular mycorrhizal fungi reduced the ratios of inorganic/organic arsenic in rice grains. Chemosphere 145, 224–230. doi: 10.1016/j.chemosphere.2015.10.067
Liu, X., Yang, G. M., Guan, D. X., Ghosh, P., and Ma, L. Q. (2015). Catecholate-siderophore produced by As-resistant bacterium effectively dissolved FeAsO4 and promoted Pteris vittata growth. Environ. Pollut. 206, 376–381. doi: 10.1016/j.envpol.2015.07.034
Lugtenberg, B. J., and Kamilova, F. (2009). Plant-growth-promoting rhizobacteria. Annu. Rev. Microbiol. 63, 541–556. doi: 10.1146/annurev.micro.62.081307.162918
Ma, Y., Oliveira, R. S., Freitas, H., and Zhang, C. (2016). Biochemical and molecular mechanisms of plant-microbe-metal interactions: relevance for phytoremediation. Front. Plant Sci. 7:918. doi: 10.3389/fpls.2016.00918
Maheswari, S., and Murugesan, A. G. (2011). Removal of arsenic(III) ions from aqueous solution using Aspergillus flavus isolated from arsenic contaminated site. Ind. J. Chem. Technol. 18, 45–52.
Mallick, I., Bhattacharyya, C., Mukherji, S., Dey, D., Sarkar, S. C., Mukhopadhyay, U. K., et al. (2018). Effective rhizoinoculation and biofilmformation by arsenic immobilizing halophilic plant growth promoting bacteria (PGPB) isolated from mangrove rhizosphere: a step towards arsenic rhizoremediation. Sci. Total Environ. 610–611, 1239–1250. doi: 10.1016/j.scitotenv.2017.07.234
Meharg, A. A., and Rahman, M. M. (2003). Arsenic contamination of Bangladesh paddy field soils: implications for rice contribution to arsenic consumption. Environ. Sci. Technol. 37, 229–234. doi: 10.1021/es0259842
Mesa, V., Navazas, A., González-Gil, R., González, A., Weyens, N., Lauga, B., et al. (2017). Use of endophytic and rhizosphere bacteria to improve phytoremediation of arsenic-contaminated industrial soils by autochthonous Betula celtiberica. Appl. Environ. Microbiol. 83, e03411–e03416. doi: 10.1128/AEM.03411-16
Mestrot, A., Feldmann, J., Krupp, E. M., Hossain, M. S., Roman-Ross, G., and Meharg, A. A. (2011). Field fluxes and speciation of arsines emanating from soils. Environ. Sci. Technol. 45, 1798–1804. doi: 10.1021/es103463d
Mishra, J., Singh, R., and Arora, N. K. (2017). Alleviation of heavy metal stress in plants and remediation of soil by rhizosphere microorganisms. Front. Microbiol. 8:1706. doi: 10.3389/fmicb.2017.01706
Mohd, S., Shukla, J., Kushwaha, A. S., Mandrah, K., Shankar, J., Arjaria, N., et al. (2017). Endophytic fungi Piriformospora indica mediated protection of host from arsenic toxicity. Front. Microbiol. 8:754. doi: 10.3389/fmicb.2017.00754
Munoz, L. P., Purchase, D., Jones, H., Feldmann, J., and Garelick, H. (2014). Enhanced determination of As-phytochelatin complexes in Chlorella vulgaris using focused sonication for extraction of water-soluble species. Anal. Methods 6, 791–797. doi: 10.1039/C3AY41629A
Neumann, R. B., St. Vincent, A. P., Roberts, L. C., Badruzzaman, A. B. M., Ali, M. A., and Harvey, C. F. (2011). Rice field geochemistry and hydrology: an explanation for why groundwater irrigated fields in Bangladesh are net sinks of arsenic from groundwater. Environ. Sci. Technol. 45, 2072–2078. doi: 10.1021/es102635d
Norton, G. J., Lou-Hing, D. E., Meharg, A. A., and Price, A. H. (2008). Rice-arsenate interactions in hydroponics: whole genome transcriptional analysis. J. Exp. Bot. 59, 2267–2276. doi: 10.1093/jxb/ern097
Olanrewaju, O. S., Glick, B. R., and Babalola, O. O. (2017). Mechanisms of action of plant growth promoting bacteria. World J. Microbiol. Biotechol. 33:197. doi: 10.1007/s11274-017-2364-9
Olmeta-Schult, F., Segal, L. M., Tyner, S., Moon, T. A., Chow, R. D. W., Chakrabarty, P., et al. (2018). NextGen Voices: research resolutions. Science 359:1008. doi: 10.1126/science.358.6366.1008-c
Páez-Espino, D., Tamames, J., de Lorenzo, V., and Cánovas, D. (2009). Microbial responses to environmental arsenic. Biometals 22, 117–130. doi: 10.1007/s10534-008-9195-y
Pathare, V., Sonawane, B. V., Srivastava, S., and Suprasanna, S. (2016). Arsenic stress affects the expression profile of genes of 14-3-3 proteins in the shoot of mycorrhiza colonized rice. Physiol. Mol. Biol. Plant 22, 515–522. doi: 10.1007/s12298-016-0382-y
Pathare, V., Srivastava, S., and Suprasanna, P. (2013). Evaluation of effects of arsenic on carbon, nitrogen and sulphur metabolism in two contrasting varieties of Brassica juncea. Acta Physiol. Plant. 35, 3377–3389. doi: 10.1007/s11738-013-1370-2
Pawlik-Skowronska, B., Pirszel, J., Kalinowska, R., and Skowronski, T. (2004). Arsenic availabiilty, toxicity and direct role of GSH and phytochelatins in As detoxification in the green alga Stichococcus bacillaris. Aquat. Toxicol. 70, 201–212. doi: 10.1016/j.aquatox.2004.09.003
Podgorski, J. E., Eqani, S. A. M. A. S., Khanam, T., Ullah, R., Shen, H., and Berg, M. (2017). Extensive arsenic contamination in high-pH unconfined aquifers in the Indus Valley. Sci. Adv. 3:e1700935. doi: 10.1126/sciadv.1700935
Poonam, S. S., Pathare, V., and Suprasanna, P. (2017). Physiological and molecular insights into rice-arbuscular mycorrhizal interactions under arsenic stress. Plant Gene 11, 232–237. doi: 10.1016/j.plgene.2017.03.004
Ranjan, R., Kumar, N., Dubey, A. K., Gautam, A., Pandey, S. N., and Mallick, S. (2018). Diminution of arsenic accumulation in rice seedlings co-cultured with Anabaena sp.: modulation in the expression of lower silicon transporters, two nitrogen dependent genes and lowering of antioxidants activity. Ecotox. Environ. Saf. 151, 109–117. doi: 10.1016/j.ecoenv.2017.12.056
Rashid, M. I., Mujawar, L. H., Shahzad, T., Almeelbi, T., Ismail, I. M., and Oves, M. (2016). Bacteria and fungi can contribute to nutrients bioavailability and aggregate formation in degraded soils. Microbiol. Res. 183, 26–41. doi: 10.1016/j.micres.2015.11.007
Requejo, R., and Tena, M. (2006). Maize responses to acute arsenic toxicity as revealed by proteome analysis of plant shoots. Proteomics 6, S156–S162. doi: 10.1002/pmic.200500381
Rodríguez-Lado, L., Sun, G., Berg, M., Zhang, Q., Xue, H., Zheng, Q., et al. (2013). Groundwater arsenic contamination throughout China. Science 341, 866–868. doi: 10.1126/science.1237484
Rosen, B. P., Ajees, A. A., and McDermott, T. R. (2011). Life and death with arsenic. Bioessays 33, 350–357. doi: 10.1002/bies.201100012
Saha, M., Sarkar, S., Sarkar, B., Sharma, B. K., Bhattacharjee, S., and Tribedi, P. (2015). Microbial siderophores and their potential applications: a review. Environ. Sci. Pollut. Res. 23, 3984–3999. doi: 10.1007/s11356-015-4294-0
Shagol, C. C., Krishnamoorthy, R., Kim, K., Sundaram, S., and Sa, T. (2014). Arsenic-tolerant plant-growth-promoting bacteria isolated from arsenic-polluted soils in South Korea. Environ. Sci. Pollut. Res. 21, 9356–9365. doi: 10.1007/s11356-014-2852-5
Sharma, S., Anand, G., Singh, N., and Kapoor, R. (2017). Arbuscular mycorrhizal augments arsenic tolerance in wheat (Triticum aestivum L.) by strengthening antioxidant defense system and thiol metabolism. Front. Plant Sci. 8:906. doi: 10.3389/fpls.2017.00906
Shukla, A., and Srivastava, S. (2017). “Emerging aspects of bioremediation of arsenic,” in Green Technologies and Environmental Sustainability, eds R. Singh and S. Kumar (Cham: Springer International Publishing AG), 395–407.
Singh, N., Marwa, N., Mishra, S. K., Mishra, J., Verma, P. C., Rathaur, S., et al. (2016). Brevundimonas diminuta mediated alleviation of arsenic toxicity and plant growth promotion in Oryza sativa L. Ecotoxicol. Environ. Saf. 125, 25–34. doi: 10.1016/j.ecoenv.2015.11.020
Singh, S., Shrivastava, A., Barla, A., and Bose, S. (2015). Isolation of arsenic-resistant bacteria from Bengal delta sediments and their efficacy in arsenic removal from soil in association with Pteris vittata. Geomicrobiol. J. 32, 712–723. doi: 10.1080/01490451.2015.1004141
Spaepen, S., and Vanderleyden, J. (2011). Auxin and plant-microbe interactions. Cold Spring Harb. Perspect. Biol. 3:a001438. doi: 10.1101/cshperspect.a001438
Spagnoletti, F. N., Balestrasse, K., Lavado, R. S., and Giacometti, R. (2016). Arbuscular mycorrhiza detoxifying responses against arsenic and pathogenic fungus in soybean. Ecotoxicol. Environ. Saf. 133, 47–56. doi: 10.1016/j.ecoenv.2016.06.012
Spagnoletti, F. N., and Lavado, R. S. (2015). The arbuscular mycorrhiza Rhizophagus intraradices reduces the negative effects of arsenic on soybean plant. J. Agron. 5, 188–199. doi: 10.3390/agronomy5020188
Srivastava, P. K., Vaish, A., Dwivedi, S., Chakrabarty, D., Singh, N., and Tripathi, R. D. (2011). Biological removal of arsenic pollution by fungi. Sci. Tot. Environ. 409, 2430–2442. doi: 10.1016/j.scitotenv.2011.03.002
Srivastava, S., Mishra, S., Tripathi, R. D., Dwivedi, S., Trivedi, P. K., and Tandon, P. K. (2007). Phytochelatins and antioxidant systems respond differentially during arsenite and arsenate stress in Hydrilla verticillata (L.f.) Royle. Environ. Sci. Technol. 41, 2930–2936. doi: 10.1021/es062167j
Srivastava, S., Srivastava, A. K., Sablok, G., Deshpande, T. U., and Suprasanna, P. (2015). Transcriptomics profiling of Indian mustard (Brassica juncea) under arsenate stress identifies key candidate genes and regulatory pathways. Front. Plant Sci. 6:646. doi: 10.3389/fpls.2015.00646
Srivastava, S., Srivastava, A. K., Suprasanna, P., and D'Souza, S. F. (2013a). Quantitative real-time expression profiling of aquaporin-isoforms and growth response of Brassica juncea under arsenic stress. Mol. Biol. Rep. 40, 2879–2886. doi: 10.1007/s11033-012-2303-7
Srivastava, S., Suprasanna, P., and D'Souza, S. F. (2012). Mechanisms of arsenic tolerance and detoxification in plants and their application in transgenic technology: a critical appraisal. Int. J. Phytorem. 14, 506–517. doi: 10.1080/15226514.2011.604690
Srivastava, S., Suprasanna, P., and D'Souza, S. F. (2013b). Redox state and energetic equilibrium determine the magnitude of stress in Hydrilla verticillata upon exposure to arsenate. Protoplasma 248, 805–815. doi: 10.1007/s00709-010-0256-z
Su, S., Zeng, X., Bai, L., Williams, P. N., Wang, Y., Zhang, L., et al. (2017). Inoculating chlamydospores of Trichoderma asperellum SM-12F1 changes arsenic availability and enzyme activity in soils and improves water spinach growth. Chemosphere 175, 497–504. doi: 10.1016/j.chemosphere.2017.02.048
Tang, Z., Lv, Y., Chen, F., Zhang, W., Rosen, B. P., and Zhao, F. J. (2016). Arsenic methylation in Arabidopsis thaliana expressing an algal arsenite methyltransferase gene increases arsenic phytotoxicity. J. Agric. Food Chem. 64, 2674–2681. doi: 10.1021/acs.jafc.6b00462
Thao, N. P., Khan, M. I. R., Thu, N. B. A., Hoang, X. L. T., Asgher, M., Khan, N. A., et al. (2015). Role of ethylene and its cross talk with other signalling molecules in plant responses to heavy metal stress. Plant Physiol. 169, 73–84. doi: 10.1104/pp.15.00663
Tripathi, P., Singh, P. C., Mishra, A., Chaudhry, V., Mishra, S., Tripathi, R. D., et al. (2013). Trichoderma inoculation ameliorates arsenic induced phytotoxic changes in gene expression and stem anatomy of chickpea (Cicer arietinum). Ecotoxicol. Environ. Safety 89, 8–14. doi: 10.1016/j.ecoenv.2012.10.017
Tripathi, P., Singh, P. C., Mishra, A., Srivastava, S., Chauhan, R., Awasthi, S., et al. (2017). Arsenic tolerant Trichoderma sp. reduces arsenic induced stress in chickpea (Cicer arietinum). Environ. Pollut. 223, 137–145. doi: 10.1016/j.envpol.2016.12.073
Upadhyay, A. K., Singh, N. K., Singh, R., and Rai, U. N. (2016). Amelioration of arsenic toxicity in rice: comparative effect of inoculation of Chlorella vulgaris and Nannochloropsis sp. on growth, biochemical changes and arsenic uptake. Ecotoxicol. Environ. Safety 124, 68–73. doi: 10.1016/j.ecoenv.2015.10.002
Vacheron, J., Desbrosses, G., Bouffaud, M. L., Touraine, B., Moënne-Loccoz, Y., Muller, D., et al. (2013). Plant growth-promoting rhizobacteria and root system functioning. Front. Plant Sci. 4:356. doi: 10.3389/fpls.2013.00356
Vejan, P., Abdullah, R., Khadiran, T., Ismail, S., and Boyce, A. N. (2016). Role of plant growth promoting rhizobacteria in agricultural sustainability-A review. Molecules 21:573. doi: 10.3390/molecules21050573
Verma, S., Verma, P. K., Meher, A. K., Dwivedi, S., Bansiwal, A. K., Pande, V., et al. (2016). A novel arsenic methyltransferase gene of Westerdykella aurantiaca isolated from arsenic contaminated soil: phylogenetic, physiological, and biochemical studies and its role in arsenic bioremediation. Metallomics 8:344. doi: 10.1039/C5MT00277J
Waghunde, R. R., Shelake, R. M., and Sabalpara, A. N. (2016). Trichoderma: a significant fungus for agriculture and environment. Afr. J. Agric. Res. 11, 1952–1965. doi: 10.5897/AJAR2015.10584
Wang, Q., Xiong, D., Zhao, P., Yu, X., Tu, B., and Wang, G. (2011). Effect of applying an arsenic-resistant and plant growth-promoting rhizobacterium to enhance soil arsenic phytoremediation by Populus deltoides LH05-17. J. Appl. Microbiol. 111, 1065–1074. doi: 10.1111/j.1365-2672.2011.05142.x
Wang, X., Nie, Z., He, L., Wang, Q., and Sheng, X. (2017). Isolation of As-tolerant bacteria and their potentials of reducing As and Cd accumulation of edible tissues of vegetables in metal(loid)-contaminated soils. Sci. Total Environ. 579, 179–189. doi: 10.1016/j.scitotenv.2016.10.239
Wang, X., Rathinasabapathi, B., Oliveira, L. M. D., Guilherme, L. R., and Ma, L. Q. (2012). Bacteria-mediated arsenic oxidation and reduction in the growth media of arsenic hyperaccumulator Pteris vittata. Environ. Sci. Technol. 46, 11259–11266. doi: 10.1021/es300454b
Wang, Y., Wang, S., Xu, P., Liu, C., Liu, M., Wang, Y., et al. (2015). Review of arsenic speciation, toxicity and metabolism in microalgae. Rev. Environ. Sci. BioTech. 14, 427–451. doi: 10.1007/s11157-015-9371-9
Wu, F., Hu, J., Wu, S., and Wong, M. H. (2015). Grain yield and arsenic uptake of upland rice inoculated with arbuscular mycorrhizal fungi in As-spiked soils. Environ. Sci. Pollut. Res. 22, 8919–8926. doi: 10.1007/s11356-012-1440-9
Yang, H. C., and Rosen, B. P. (2016). New mechanisms of bacterial arsenic resistance. Biomed. J. 39, 5–13. doi: 10.1016/j.bj.2015.08.003
Yoon, I. H., Chang, J. S., Lee, J. H., and Kim, K. W. (2009). Arsenite oxidation by Alcaligenes sp. strain RS-19 isolated from arsenic-contaminated mines in the Republic of Korea. Environ. Geochem. Health 31, 109–117. doi: 10.1007/s10653-008-9170-0
Keywords: arsenic, bioremediation, crop plants, plant growth-promoting microbes, toxicity
Citation: Upadhyay MK, Yadav P, Shukla A and Srivastava S (2018) Utilizing the Potential of Microorganisms for Managing Arsenic Contamination: A Feasible and Sustainable Approach. Front. Environ. Sci. 6:24. doi: 10.3389/fenvs.2018.00024
Received: 08 January 2018; Accepted: 18 April 2018;
Published: 07 May 2018.
Edited by:
Pankaj Kumar Arora, Babasaheb Bhimrao Ambedkar University, IndiaReviewed by:
M. Oves, King Abdulaziz University, Saudi ArabiaCopyright © 2018 Upadhyay, Yadav, Shukla and Srivastava. This is an open-access article distributed under the terms of the Creative Commons Attribution License (CC BY). The use, distribution or reproduction in other forums is permitted, provided the original author(s) and the copyright owner are credited and that the original publication in this journal is cited, in accordance with accepted academic practice. No use, distribution or reproduction is permitted which does not comply with these terms.
*Correspondence: Sudhakar Srivastava, c3VkaGFrYXIuc3JpdmFzdGF2YUBnbWFpbC5jb20=; c3VkaGFrYXIuaWVzZEBiaHUuYWMuaW4=
Disclaimer: All claims expressed in this article are solely those of the authors and do not necessarily represent those of their affiliated organizations, or those of the publisher, the editors and the reviewers. Any product that may be evaluated in this article or claim that may be made by its manufacturer is not guaranteed or endorsed by the publisher.
Research integrity at Frontiers
Learn more about the work of our research integrity team to safeguard the quality of each article we publish.