- 1Ecology and Genetics, University of Oulu, Oulu, Finland
- 2Department of Biological and Environmental Science, University of Jyväskylä, Jyvaskyla, Finland
- 3Taras Shevchenko National University of Kyiv, Kyiv, Ukraine
- 4Ecologie Systématique Evolution, Université Paris-Sud, CNRS, AgroParisTech, Université Paris-Saclay, Orsay, France
- 5Department of Biological Sciences, University of South Carolina, Columbia, SC, United States
Exposure to ionizing radiation (IR) from radionuclides released into the environment can damage DNA. An expected response to exposure to environmental radionuclides, therefore, is initiation of DNA damage response (DDR) pathways. Increased DNA damage is a characteristic of many organisms exposed to radionuclides but expression of DDR genes of wildlife inhabiting an area contaminated by radionuclides is poorly understood. We quantified expression of five central DDR genes Atm, Mre11, p53, Brca1, and p21 in the livers of the bank vole Myodes glareolus that inhabited areas within the Chernobyl Exclusion Zone (CEZ) that differed in levels of ambient radioactivity, and also from control areas outside the CEZ (i.e., sites with no detectable environmental radionuclides) in Ukraine. Expression of these DDR genes did not significantly differ between male and female bank voles, nor among sites within the CEZ. We found a near two-fold upregulation in the DDR initiators Mre11 and Atm in animals collected from the CEZ compared with samples from control sites. As Atm is an important regulator of oxidative stress, our data suggest that antioxidant activity may be a key component of the defense against exposure to environmental radioactivity.
Introduction
Accidental release of radionuclides into the environment presents a potential health risk to humans and wildlife (Møller and Mousseau, 2006; Lourenço et al., 2016). On 26 April 1986, reactor 4 of the Chernobyl Nuclear Power Plant (NPP) exploded, releasing an estimated 9 × 103 to 1 × 104 petabecquerels (Pbq) of radionuclides over much of Eastern Europe, Russia, and Fennoscandia (Dreicer et al., 1996). This accident, together with the accident at the Fukushima Daiichi NPP in 2011, stimulated public and scientific interest in the impacts of environmental radionuclides on natural ecosystems (Wheatley et al., 2016). To limit human exposure to radionuclides, the Chernobyl Exclusion Zone (CEZ) was established at ~30 km radius around the accident site. The CEZ still contains elevated levels of isotopes with long half-lives, notably strontium-90, caesium-137, and plutonium-239 (about 29, 30, and 24,100 years, respectively). The wildlife inhabiting the CEZ provide the best-studied model of the biological impact of exposure to radionuclides (Møller and Mousseau, 2006).
One harmful effect of exposure to ionizing radiation (IR) from radionuclides is elevated DNA damage, either directly or by generating reactive oxygen species (ROS) through radiolysis of intracellular water (Ward, 1988; Einor et al., 2016). Elevated DNA damage, for example as chromosomal aberrations (Dzyubenko and Gudkov, 2009) or DNA breaks (Bonisoli-Alquati et al., 2010; Fujita et al., 2014), has been observed in wildlife and humans inhabiting areas affected by the accidents at Chernobyl and Fukushima (reviewed by Lourenço et al., 2016). That not all studies find elevated DNA damage in areas with increased radioactivity (e.g., Bonisoli-Alquati et al., 2015) points to interspecific differences in response to environmental radionuclides (Møller and Mousseau, 2015). Somewhat surprisingly, despite the many (>200) studies of DNA damage, few studies have quantified activity of DNA repair pathway genes on organisms exposed to environmental radionuclides (Lourenço et al., 2016).
In eukaryotes, DNA repair is activated and regulated by the DNA damage response (DDR) pathway, which is a multi-branched signaling cascade initiated by the serine/threonine kinases ATR and ATM upon detection of single-strand DNA breaks (SSBs) and double-strand DNA breaks (DSBs), respectively (Giglia-Mari et al., 2011). DSBs typically result from exposure to highly genotoxic agents and IR (Ward, 1990) and pose a serious problem for genomic integrity as they cannot always be repaired without incorporating mutations, thus increasing the risk of cancer (Cannan and Pederson, 2016). DSBs are detected by the MRE11-RAD50-NBS1 complex, which activates ATM. Pathways activated by ATM include p53-mediated cell cycle checkpoint and apoptosis, increased antioxidant production, and DNA repair, where the choice between non-homologous end joining (NHEJ) and homologous recombination (HR) repair is regulated by BRCA1 (Daley and Sung, 2014).
Just three studies, all on plants, have quantified DNA repair activity in wildlife affected by the Chernobyl and Fukushima accidents. After exposure to high doses (750 Gy) of IR, DNA repair activity in pollen and seed from two species of plant depended upon the composition of radionuclides in the soil in which the parental plants had grown (Boubriak et al., 2008). Progeny of Arabidopsis inhabiting areas around Chernobyl exhibited low recombination rates, despite X-ray induced upregulation of homologous recombination repair-related Rad54-like in samples from a contaminated area (Kovalchuk et al., 2014). Unfortunately these studies within the CEZ did not use replicate samples from contaminated and uncontaminated sites, making it challenging to determine whether radiation or a third variable accounts for these effects. In rice (Oryza sativa) seedlings, exposure to low-dose IR affected expression of stress response and DNA repair associated genes (Hayashi et al., 2014), with some single-strand break repair genes initially upregulated but suppressed after 24 h of IR exposure. Ultimately, plants have been found to be sensitive to environmental radiation, and it has been suggested that they could serve as bioindicator species for assessing radiation risk (Nikitaki et al., 2017). The DDR of wild vertebrates exposed to environmental radionuclides has rarely been studied, although, for example, upregulation in the p53 gene has been observed in wood mice (Apodemus sylvaticus) inhabiting a former uranium mine area (Lourenço et al., 2013).
The subject of the physiological and ecological effects of low-dose IR is highly controversial, as evidenced by several conflicting studies concerning species diversity and abundance in the CEZ. Populations of large mammals, such as the wild boar (Sus scrofa), appear especially abundant (Deryabina et al., 2015), but it is difficult to quantify the effect of human absence on these populations. Here, we quantify mRNA transcription in five DDR and repair genes in livers of the bank vole Myodes glareolus, a small rodent that inhabits areas within and outside the CEZ. Multiple populations from both experimental and control regions were studied to ensure spatial replication of study sites that differ in contamination levels. The bank vole is abundant (typically 10–80 animals per hectare) in forest habitats in much of Europe and Asia (Hutterer et al., 2016) and was one of the first mammals to re-colonize the CEZ after the nuclear accident (Baker et al., 1996). Bank voles inhabiting the CEZ show an increased frequency of chromosomal aberrations (Goncharova and Ryabokon, 1995) and increased oxidative stress in the form of cataracts (Lehmann et al., 2016), although estimates of DNA damage have returned conflicting results (Cristaldi et al., 1991; Rodgers and Baker, 2000). We hypothesize that exposure to low-dose IR stimulates the expression of key genes in the DDR pathway.
Materials and Methods
Sample Collection
Animals were caught using Ugglan Special live traps (Grahnab, Sweden), with sunflower seeds and potato as bait, during 6th−11th May 2015, at 14 locations within the CEZ that differed in levels of soil radionuclides (Figure 1). Bank voles were also caught during 16th−27th August 2015 at three separate regions outside the CEZ, where elevated levels of soil radionuclides have not been detected: Brody (50°0594 N, 25°10752 E), Lubny (50°05564 N, 32°98566 E), and Korostyshev (50°34422 N, 29°23673 E) in order to control for habitat effects. At each trapping location, 20 traps were placed in a line, with each trap separated by about 10 m and with trapping locations separated by at least 500 m. Procedures were performed in accordance with relevant guidelines and regulations, approved by the Finnish Animal Experiment Board and the Finnish Ministry of the Environment (under the authorization ESAVI/3834/04.10.03/2011 and ESAVI/7256/04.10.07/2014). Ambient radiation levels at the trapping locations were measured at 1 cm above the ground with a hand-held GM dosimeter (Inspector, International Medcom INC, Sebastopol, CA, USA) calibrated to measure Sieverts (Sv); such measurements of radiation are repeatable among days and even years (Møller and Mousseau, 2013). Mean ambient radiation levels varied among trapping locations from 0.1 to 12.3 μSv/h within the CEZ (Figure 1) and was 0.135 μSv/h at the three areas outside the CEZ. To estimate lifetime external doses, animals were allocated to two age groups based on head width, which is often used as a proxy of age in small mammals (Kallio et al., 2014): juveniles (<12.0 mm) and adults (≥12.1 mm). Accumulated external doses were calculated for 1 month (juveniles, ranging from 0.07 to 9.01 mGy) and for the range of 2–5 months (adults, ranging from 0.20 to 28.64 mGy; Datasheet 1).
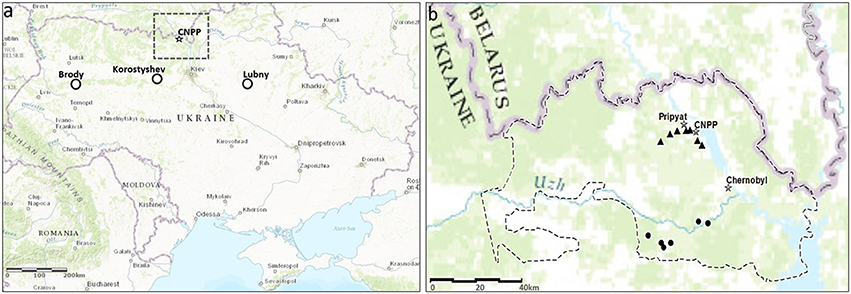
Figure 1. (a) Location of control population trapping sites in Ukraine. (b) Bank vole trapping sites in the Chernobyl Exclusion Zone (CEZ). Background radiation intensity is shown as circles (<1.0 μGy/h) and as triangles (up to 12.3 μGy/h). Created using ESRI ArcGIS 10.5. Satellite imagery©.
After measuring head width (to 0.1 mm) and body mass (to 0.1 g), animals were euthanized by cervical dislocation and liver tissue samples transferred to Allprotect Tissue Reagent (Qiagen). We selected bank voles with 10–14 mm head width to reduce variation associated with maturation. Samples were stored at −80°C until processing.
Quantitative PCR (qPCR)
Intron-exon boundaries in five DSB damage response genes Atm, Mre11, Brca1, p53, and p21 were identified using sequences from a draft bank vole genome (Genbank accession no. GCA_001305785). Bank vole gene sequences were aligned using online BLAST (Altschul et al., 1990) with default parameters against their putative mRNA homologs in the prairie vole (Microtus ochrogaster) available in Genbank: p53 (XM_005349777), Brca1 (XM_013354463), Atm (XM_013346263), Mre11a (XM_013355380), and p21 (XM_005360336). For normalization of gene expression, we amplified two reference genes: beta-actin (Actb) and retention in endoplasmic reticulum sorting receptor 1 (Rer1). Beta-actin primers are based on a sequence from mouse (Mus musculus) and primers for Rer1 were based on the putative gene sequence derived from bank vole genome. Primers were designed using Primer3 (Rozen and Skaletsky, 2000; Table 1). The mouse nucleotide database in Genbank was used to identify common transcripts and avoid potentially rare splice variants.
Up to 30 mg liver tissue per sample was homogenized using TissueLyser II (Qiagen) bead mill (2 × 2 min at 25 Hz), and total RNA was extracted with RNeasy Mini Kit (Qiagen) that incorporated a DNase digestion step according to the manufacturer's protocol. Four hundred nanograms of total RNA per sample was used for reverse transcription in 20 μl reaction volumes using iScript cDNA synthesis kit (Bio-Rad) according to the manufacturer's protocol.
Quantitative PCRs were completed for each individual sample in 16 μl final reaction volumes that contained 4 ng cDNA template, 400 nM both forward and reverse primers and 8 μl LightCycler 480 SYBR Green I Master (Roche). Thermal cycling profiles were: 95°C for 5 min followed by, 95°C for 10 s, 60°C for 15 s (for all primers), and 72°C for 10 s (with recording), using a LightCycler 480 Real-Time PCR System (Roche). Primer specificity was determined by melt curve analysis and PCR efficiencies were calculated from standard curves using five-fold serial dilutions of mixed-sample liver cDNA (Table 1). All qPCRs were run as three technical replicates and a sample was re-analyzed if the standard deviation among replicates was >0.4. Actb showed slightly greater variation in expression (SD = 0.97 cycles, n = 25) than Rer1 (SD = 0.65 cycles, n = 25) across samples.
Data Analysis
Raw data were imported into GenEx v.6.1 to allow PCR efficiency correction (, where CqE is the uncorrected Cq value and E is the PCR efficiency). Each sample was normalized against the geometric mean expression of two internal reference genes Actb and Rer1 to control for outlying values (Vandesompele et al., 2002). Cq values were converted to relative linear scale with average expression of the control groups set as the reference level, after which all data were converted to log2 scale (Datasheet 2). Subsequent statistical analyses were performed in SPSS v.24.0 (IBM Corp. 2016).
First, we examined differences in gene expression between three groups of samples: (1) animals within the CEZ caught from areas with elevated ambient radiation dose rates (>1.0 μGy/h, mean 4.8 μGy/h, conferring a yearly external radiation dose of between ~10 and 110 mGy at the most irradiated location), (2) animals from within the CEZ where ambient radiation was not dramatically elevated (<1.0 μGy/h, mean 0.20 μGy/h) and (3) animals from outside the CEZ (mean 0.13 μGy/h). While the levels of soil radiation do not differ significantly between the latter two groups, bank voles inhabiting the CEZ may have moved among areas prior to capture. Variation in gene expression among the three groups and possible sex interaction was analyzed using two-way analysis of variance (ANOVA), followed by a Tukey's post-hoc test. Second, as no significant differences in gene expression could be attributed to an animal's sex or among samples within the CEZ (see Results), we made a comparison in gene expression between animals collected (1) from within the CEZ and (2) the three control areas, using a Student's t-test.
Results
We quantified gene expression in five DDR genes Atm, Mre11, p53, Brca1, and p21 in bank voles populating the CEZ. We chose these genes for their positions and signaling roles at the top of the DDR cascade to investigate possible activation of DDR response in a natural low-dose radiation environment. Our analyses were based on data from 57 animals: 30 (14 males, 16 females) from the CEZ, and 27 (14 m, 13 f) from Brody, Lubny, and, Korostyshev. Samples from the CEZ were further divided into two groups of “elevated” (7 m, 5 f) and “near-background” (7 m, 11 f) levels of environmental radioactivity as described above. Lifetime external doses within the “elevated” group were estimated to range between 1.14 and 9.01 mGy for juveniles and 2.82 to 28.64 mGy for adults, while in “near-background group” ranges were 0.07–0.34 mGy for juveniles and 0.13–1.68 mGy for adults. Gene expression levels were found to be similar between the three external control locations (Datasheet 2), and thus were considered as a single control group. Liver was selected as the tissue of interest as it is radiosensitive in a clinical context (Christiansen et al., 2007; Stryker, 2007); moreover, liver tissue appears sensitive to DNA damage, with liver tissue from bank voles exposed to environmental radionuclides having shorter telomeres compared with samples from control areas (Kesäniemi et al. unpublished).
We found significant differential expression in Mre11 and Atm, which could be explained by radioactivity [2-way ANOVA, Atm F(2, 51) = 9.52, P < 0.001; Mre11 F(2, 51) = 10,18, P < 0.001; p53 F(2, 51) = 0.34, P > 0.05; Brca1 F(2, 51) = 0.04, P > 0.05; p21 F(2, 51) = 0.62, P > 0.05]. No significant differences in the expression of any of the five genes could be explained by sex [Atm F(2, 51) = 0.19, P > 0.05; Mre11 F(2, 51) = 0.60, P > 0.05; p53 F(2, 51) = 0.07, P > 0.05; Brca1 F(2, 51) = 1.82, P > 0.05; p21 F(2, 51) = 0.03, P > 0.05]. Significant upregulation (compared with samples from control areas) of Atm and Mre11 was observed in both “elevated” (PAtm < 0.01, PMre11 < 0.05) and “near-background” (PAtm < 0.001, PMre11 < 0.001) groups of samples from the CEZ, despite the significantly lower amount of environmental radiation in the latter group of samples (Figure 2); upregulation of Mre11 was in fact slightly stronger (but non-significantly so) in the “near-background” treatment.
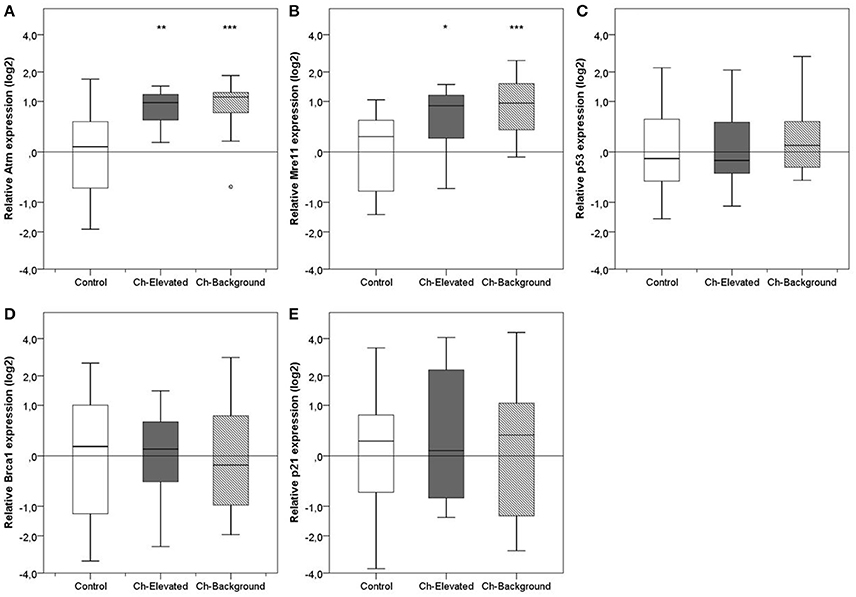
Figure 2. Relative expression of five central DNA damage response (DDR) genes in livers of bank voles collected from the Chernobyl Exclusion Zone (CEZ) (Ch-Background = <1.0 μGy/h, Ch-Elevated = up to 12.3 μGy/h) and control locations outside the CEZ. Gene expression was normalized against two internal reference genes (Actb and Rer1) with the average expression in the control group set as the reference level. (A) Atm, (B) Mre11, (C) p53, (D) Brca1, and (E) p21. *p < 0.05; **p < 0.005; ***p < 0.001.
Hence, relative quantification of five DDR genes among samples (males and females) from (1) the CEZ and (2) the control areas outside the CEZ revealed significant upregulation in Atm and Mre11 in bank voles inhabiting the CEZ compared with bank voles taken from control areas: transcription of mRNA increased almost two-fold in Atm (1.93-fold increase, t(55) = 4.51, P < 0.001) and Mre11 (1.87-fold increase, t(55) = 4.56, P < 0.001), whereas no significant differences in mRNA levels of p53, Brca1, or p21 were detected (Figure 2).
Discussion
Exposure to IR causes DNA damage, and elevated DNA damage is characteristic of wildlife inhabiting areas affected by nuclear accident sites. An appropriate response from DNA repair pathways is an expected response to exposure to environmental radioactivity. We identified a significant, almost two-fold, upregulation in expression of Atm and Mre11 in the livers of bank voles living within the CEZ, which is the first evidence that expression of DNA repair-related genes is stimulated in vertebrates exposed to low-dose environmental radioactivity.
A notable feature of these data is the similar gene expression in animals caught from within the CEZ, irrespective of the level of soil radionuclides at the trapping location. The CEZ comprises a mosaic of radionuclide contamination (Chesser et al., 2004) where the variation in radionuclide levels in soil and vegetation introduce small scale (few hundred meters) heterogeneity in the overall received doses for bank voles (Chesser et al., 2000). One explanation for the common upregulation effect is that bank voles can readily disperse over 1 km during the breeding season (Kozakiewicz et al., 2007) and thus individuals trapped from the areas with little or no soil radionuclides within the CEZ could have been exposed to substantial levels of radionuclides prior to capture. Bank voles inhabit burrows and have an opportunistic diet (Butet and Delettre, 2011), and are thus exposed to a wide variety of ingested radionuclides including the common fission product caesium-137. Caesium-137 is particularly problematic for ecosystems due to its capacity to form various water-soluble salts. Bank voles can carry potentially high amounts of internal radiation sources (Baker et al., 2017). In this scenario, the common upregulation effect between contaminated and non-contaminated areas represents an average expression for inhabiting the CEZ especially if there are carry forward effects from exposure to very high amounts of radionuclides in prior generations. Such effects of past exposures have been reported with respect to mitochondrial mutation rates in these same voles (Baker et al., 2017) and would have the effect of averaging effects over broader geographic scales. Another possibility is that observed patterns reflect local adaptations that have spread from populations at contaminated regions to adjacent areas via gene flow (Møller et al., 2006; Fedorka et al., 2012) which could also account for a “higher than expected” level of expression in less contaminated areas within the CEZ.
Upregulation of DDR pathways of animals residing within the CEZ may seem intuitive, but these pathways have not been studied in wildlife inhabiting areas contaminated by radioactivity. That Atm is upregulated is relevant as this gene is positioned at the top of the DBS repair pathway. ATM is primarily regulated at the protein level when subjected to IR (Bakkenist and Kastan, 2003), but Atm promoter activity, and protein abundance, is stimulated by exposure to IR in mice tissues, including liver (Gueven et al., 2006); moreover, silencing of ATM decreases radioresistance of glioma in vitro and in vivo (Li et al., 2016). Perhaps crucially, ATM is an important regulator of cellular oxidative stress (Barzilai et al., 2002), promoting the production of NADPH (Cosentino et al., 2011). Moreover, ATM can be activated by oxidative stress independently from DBS-related activation by the MRN complex (Guo et al., 2010). Antioxidant production likely constitutes a major part of the adaptive response against environmental radioactivity in birds within and around the CEZ (Galván et al., 2014) and in cell lines of fibroblasts from bank voles (Mustonen et al., submitted). Moreover, a change in fur color of bank voles within the CEZ was attributed to downregulation of pheomelanin to reallocate antioxidants to ROS defense (Boratynski et al., 2014). Indeed, upregulation of oxidative stress response genes Cat and Fsd3 in Arabidopsis (Kovalchuk et al., 2014) indicate that increased ROS defense could be a key coping strategy against exposure to environmental radionuclides.
MRE11 is a constituent protein of the MRE11-RAD50-NBS1 complex, a multi-purpose maintainer of genomic stability whose tasks include DSB detection and subsequent activation of ATM protein (Lee and Paull, 2005) as well as DNA repair functions and telomeric maintenance (Lamarche et al., 2010). Reduced expression of the Mre11 gene often leads to genomic instability, and increased protein levels of ATM and MRE11 in some tumors are associated with resistance against radiotherapy (Tribius et al., 2001; Deng et al., 2011). An apparently low frequency of micronuclei in bank voles from the CEZ might indicate that this species has some degree of radioresistance (Rodgers and Baker, 2000). Given our results, Atm and Mre11 represent important candidate genes that regulate genomic stability in bank voles exposed to environmental radionuclides.
Our observation of no significant variation in expression of p53 may be explained by this gene being primarily regulated through post-translational modification of cellular protein (Kruse and Gu, 2009), although transcriptional upregulation has previously been detected in the livers of European wood mice inhabiting an abandoned uranium mine area (Lourenço et al., 2013). Significant enrichment of heavy metals (uranium and cadmium) were also detected in these mice, contributing to toxicity alongside radiation. Thus, radionuclide levels comparable to studied areas in the CEZ are not severe enough to induce transcription in p53. P21 is under transcriptional regulation by p53 and is required for DNA damage related cell cycle inhibition (Bunz, 1998). That p21 expression is not altered in animals inhabiting the CEZ implies cell cycle control pathways are not activated in response to increased Atm expression. Brca1 is upregulated in response to genotoxic stress (De Siervi et al., 2010). A lack of change in expression of Brca1 in bank voles from the CEZ indicates that the homologous recombination repair pathway is not a key component of the response to environmental radionuclides, consistent with a recent study of expression of DBS repair pathways during exposure to IR (Liu et al., 2016). Arabidopsis from the CEZ show reduced recombination, which might prevent gross chromosomal rearrangements (Kovalchuk et al., 2014).
As the expression of p21 and Brca1 is not stimulated it is plausible that the low-dose IR environment of Chernobyl does not cause sufficient DNA damage to warrant cell cycle arrest and activation of DSB repair. Nonetheless, some genomic impact is derived from exposure to radionuclides as bank voles from the CEZ have shorter telomeres than bank voles from control areas (Kesäniemi et al., unpublished), likely reflecting an increase in oxidative stress that is widely associated with telomere shortening (von Zglinicki, 2002). Hence is it interesting that ATM can be activated by oxidation independently from MRN interaction. Altered telomere homeostasis may affect the expression of Mre11 as a telomere maintainer and the role of this gene warrants further investigation.
Author Contributions
TJ and PW: designed the outline of the study; AL, ET, AM, TM, TAM, and GM: were involved in setup of infrastructure in the field and animal sample collection, and TJ and JK: conducted molecular analyses and performed calculations; TJ: wrote the manuscript with input from all other authors.
Conflict of Interest Statement
The authors declare that the research was conducted in the absence of any commercial or financial relationships that could be construed as a potential conflict of interest.
Acknowledgments
We are grateful to GM, Igor Chizhevsky, Serhii Kireyev, Anatoly Nosovsky, Viktor Krasnov, and Maksym Ivanenko for logistic support during fieldwork. The project was funded by Academy of Finland grants awarded to PW (project number 287153) and to TM (project number 268670) and by Kuopio Naturalists' Society (to TJ). Funding to TAM and AM included grants from the Samuel Freeman Charitable Trust and the American Council of Learned Societies.
Supplementary Material
The Supplementary Material for this article can be found online at: https://www.frontiersin.org/articles/10.3389/fenvs.2017.00095/full#supplementary-material
Datasheet 1. Accumulated external dose estimations by age groups.
Datasheet 2. qPCR raw and normalized quantification cycle data.
References
Altschul, S. F., Gish, W., Miller, W., Myers, E. W., and Lipman, D. J. (1990). Basic local alignment search tool. J. Mol. Biol. 215, 403–410. doi: 10.1016/S0022-2836(05)80360-2
Baker, R. J., Dickins, B., Wickliffe, J. K., Khan, F. A., Gaschak, S., Makova, K., et al. (2017). Elevated mitochondrial genome variation after 50 generations of radiation exposure in a wild rodent. Evol. Appl. 10, 784–791. doi: 10.1111/eva.12475
Baker, R. J., Hamilton, M. J., Van Den Bussche, R. A., Wiggins, L. E., Sugg, D. W., Smith, M. H., et al. (1996). Small mammals from the most radioactive sites near the chornobyl nuclear power plant. J. Mammal. 77, 155–170. doi: 10.2307/1382717
Bakkenist, C. J., and Kastan, M. B. (2003). DNA damage activates ATM through intermolecular autophosphorylation and dimer dissociation. Nature 421, 499–506. doi: 10.1038/nature01368
Barzilai, A., Rotman, G., and Shiloh, Y. (2002). ATM deficiency and oxidative stress: a new dimension of defective response to DNA damage. DNA Repair 1, 3–25. doi: 10.1016/S1568-7864(01)00007-6
Bonisoli-Alquati, A., Voris, A., Mousseau, T. A., Møller, A. P., Saino, N., and Wyatt, M. D. (2010). DNA damage in barn swallows (Hirundo rustica) from the Chernobyl region detected by use of the comet assay. Comp. Biochem. Physiol. C Toxicol. Pharmacol. 151, 271–277. doi: 10.1016/j.cbpc.2009.11.006
Bonisoli-Alquati, A., Koyama, K., Tedeschi, D. J., Kitamura, W., Sukuzi, H., Ostermiller, S., et al. (2015). Abundance and genetic damage of barn swallows from Fukushima. Sci. Rep. 5:9432. doi: 10.1038/srep09432
Boratynski, Z., Lehmann, P., Mappes, T., Mousseau, T. A., and Møller, A. P. (2014). Increased radiation from Chernobyl decreases the expression of red colouration in natural populations of bank voles (Myodes glareolus). Sci. Rep. 4:7141. doi: 10.1038/srep07141
Boubriak, I. I., Grodzinsky, D. M., Polischuk, V. P., Naumenko, V. D., Gushcha, N. P., Micheev, A. N., et al. (2008). Adaptation and impairment of DNA repair function in pollen of Betula verrucosa and seeds of Oenothera biennis from differently radionuclide- contaminated sites of Chernobyl. Ann. Bot. 101, 267–276. doi: 10.1093/aob/mcm276
Bunz, F. (1998). Requirement for p53 and p21 to sustain G2 arrest after DNA damage. Science 282, 1497–1501. doi: 10.1126/science.282.5393.1497
Butet, A., and Delettre, Y. R. (2011). Diet differentiation between European Arvicoline and Murine rodents. Acta Theriol. 56, 297–304. doi: 10.1007/s13364-011-0049-6
Cannan, W. J., and Pederson, D. S. (2016). Mechanisms and consequences of double-strand DNA break formation in chromatin. J. Cell. Physiol. 231, 3–14. doi: 10.1002/jcp.25048
Chesser, R. K., Bondarkov, M., Baker, R. J., Wickliffe, J. K., and Rodgers, B. E. (2004). Reconstruction of radioactive plume characteristics along Chernobyl's Western Trace. J. Environ. Radioact. 71, 147–157. doi: 10.1016/S0265-931X(03)00165-6
Chesser, R. K., Sugg, D. W., Lomakin, M. D., van den Bussche, R. A., DeWoody, J. A., Jagoe, C. H., et al. (2000). Concentrations and dose rate estimates of 134137cesium and 90strontium in small mammals at chornobyl, Ukraine. Environ. Toxicol. Chem. 19, 305–312. doi: 10.1002/etc.5620190209
Christiansen, H., Sheikh, N., Saile, B., Reuter, F., Rave-Fränk, M., Hermann, R. M., et al. (2007). x-Irradiation in rat liver: consequent upregulation of hepcidin and downregulation of hemojuvelin and ferroportin-1 gene expression. Radiology 242, 189–197. doi: 10.1148/radiol.2421060083
Cosentino, C., Grieco, D., and Costanzo, V. (2011). ATM activates the pentose phosphate pathway promoting anti-oxidant defence and DNA repair. EMBO J. 30, 546–555. doi: 10.1038/emboj.2010.330
Cristaldi, M., Ieradi, L. A., Mascanzoni, D., and Mattei, T. (1991). Environmental impact of the Chernobyl accident: mutagenesis in bank voles from Sweden. Int. J. Radiat. Biol. 59, 31-40. doi: 10.1080/09553009114550031
Daley, J. M., and Sung, P. (2014). 53BP1, BRCA1, and the choice between recombination and end joining at DNA double-strand breaks. Mol. Cell. Biol. 34, 1380–1388. doi: 10.1128/MCB.01639-13
Deng, R., Tang, J., Ma, J.-G., Chen, S.-P., Xia, L.-P., Zhou, W.-J., et al. (2011). PKB/Akt promotes DSB repair in cancer cells through upregulating Mre11 expression following ionizing radiation. Oncogene 30, 944–955. doi: 10.1038/onc.2010.467
Deryabina, T. G., Kuchmel, S. V., Nagorskaya, L. L., Hinton, T. G., Beasley, J. C., Lerebours, A., et al. (2015). Long-term census data reveal abundant wildlife populations at Chernobyl. Curr. Biol. 25, R824–R826. doi: 10.1016/j.cub.2015.08.017
De Siervi, A., De Luca, P., Byun, J. S., Di, L. J., Fufa, T., Haggerty, C. M., et al. (2010). Transcriptional autoregulation by BRCA1. Cancer Res. 70:532. doi: 10.1158/0008-5472.CAN-09-1477
Dreicer, M., Aarkrog, A., Alexakhin, R., Anspaugh, L., Arkhipov, N. P., and Johansson, K.-J. (1996). “Consequences of the Chernobyl accident for the natural and human environments,” in International Conference on “One Decade after Chernobyl: Summing up the Consequences of the Accident” (Vienna) (IAEA), 319–361.
Dzyubenko, E. V., and Gudkov, D. I. (2009). Cytogenetical and haematological effects of long-term irradiation on freshwater gastropod snails in the Chernobyl accident exclusion zone. Radioprotection 44, 933–936. doi: 10.1051/radiopro/20095166
Einor, D., Bonisoli-Alquati, A., Costantini, D., Mousseau, T. A., and Møller, A. P. (2016). Ionizing radiation, antioxidant response and oxidative damage: a meta-analysis. Sci. Total Environ. 548–549, 463–471. doi: 10.1016/j.scitotenv,.2016.01.027
Fedorka, K. M., Winterhalter, W. E., Shaw, K. L., Brogan, W. R., and Mousseau, T. A. (2012). The role of gene flow asymmetry along an environmental gradient in constraining local adaptation and range expansion. J. Evol. Biol. 25, 1676–1685. doi: 10.1111/j.1420-9101.2012.02552.x
Fujita, Y., Yoshihara, Y., Sato, I., and Sato, S. (2014). Environmental radioactivity damages the DNA of earthworms of Fukushima Prefecture, Japan. Eur. J. Wildl. Res. 60, 145–148. doi: 10.1007/s10344-013-0767-y
Galván, I., Bonisoli-Alquati, A., Jenkinson, S., Ghanem, G., Wakamatsu, K., Mousseau, T. A., et al. (2014). Chronic exposure to low-dose radiation at Chernobyl favours adaptation to oxidative stress in birds. Funct. Ecol. 28, 1387–1403. doi: 10.1111/1365-2435.12283
Giglia-Mari, G., Zotter, A., and Vermeulen, W. (2011). DNA damage response. Cold Spring Harb. Perspect. Biol. 3, 1–19. doi: 10.1101/cshperspect.a000745
Goncharova, R. I., and Ryabokon, N. I. (1995). Dynamics of cytogenetic injuries in natural populations of bank vole in the Republic of Belarus. Radiat. Prot. Dosimetry 62, 37–40. doi: 10.1093/oxfordjournals.rpd.a082816
Gueven, N., Fukao, T., Luff, J., Paterson, C., Kay, G., Kondo, N., et al. (2006). Regulation of the Atm promoter in vivo. Genes Chromosomes Cancer 45, 61–71. doi: 10.1002/gcc.20267
Guo, Z., Kozlov, S., Lavin, M. F., Person, M. D., and Paull, T. T. (2010). ATM activation by oxidative stress. Science 330, 517–521. doi: 10.1126/science.1192912
Hayashi, G., Shibato, J., Imanaka, T., Cho, K., Kubo, A., Kikuchi, S., et al. (2014). Unraveling low-level gamma radiation-responsive changes in expression of early and late genes in leaves of rice seedlings at litate Village, Fukushima. J. Hered. 105, 723–738. doi: 10.1093/jhered/esu025
Hutterer, R., Kryštufek, B., Yigit, N., Mitsain, G., Palomo, L. J., Henttonen, H., et al. (2016). Myodes glareolus. IUCN Red List Threat. Species 2016.
Kallio, E. R., Begon, M., Birtles, R. J., Bown, K. J., Koskela, E., Mappes, T., et al. (2014). First report of Anaplasma phagocytophilum and Babesia microti in rodents in Finland. Vector Borne Zoonotic Dis. 14, 389–393. doi: 10.1089/vbz.2013.1383
Kovalchuk, I., Abramov, V., Pogribny, I., Kovalchuk, O., Physiology, S. P., and May, N. (2014). Molecular aspects of plant adaptation to life in the chernobyl zone. Plant Physiol. 135, 357–363. doi: 10.1104/pp.104.040477
Kozakiewicz, M., Chołuj, A., and Kozakiewicz, A. (2007). Long-distance movements of individuals in a free-living bank vole population: an important element of male breeding strategy. Acta Theriol. 52, 339–348. doi: 10.1007/BF03194231
Kruse, J.-P., and Gu, W. (2009). Modes of p53 regulation. Cell 137, 609–622. doi: 10.1016/j.cell.2009.04.050
Lamarche, B. J., Orazio, N. I., and Weitzman, M. D. (2010). The MRN complex in double-strand break repair and telomere maintenance. FEBS Lett. 584, 3682–3695. doi: 10.1016/j.febslet.2010.07.029
Lee, J.-H., and Paull, T. T. (2005). ATM activation by DNA double-strand breaks through the Mre11-Rad50-Nbs1 complex. Science 308, 551–554. doi: 10.1126/science.1108297
Lehmann, P., Boratynski, Z., Mappes, T., Mousseau, T. A., and Møller, A. P. (2016). Fitness costs of increased cataract frequency and cumulative radiation dose in natural mammalian populations from Chernobyl. Sci. Rep. 6:19974. doi: 10.1038/srep19974
Li, Y., Li, L., Li, B., Wu, Z., Wu, Y., Wang, Y., et al. (2016). Silencing of ataxia-telangiectasia mutated by siRNA enhances the in vitro and in vivo radiosensitivity of glioma. Oncol. Rep. 35, 3303-3312. doi: 10.3892/or.2016.4754
Liu, M., Wang, H., Lee, S., Liu, B., Dong, L., and Wang, Y. (2016). DNA repair pathway choice at various conditions immediately post irradiation. Int. J. Radiat. Biol. 92, 819–822. doi: 10.1080/09553002.2016.1230243
Lourenço, J., Mendo, S., and Pereira, R. (2016). Radioactively contaminated areas: bioindicator species and biomarkers of effect in an early warning scheme for a preliminary risk assessment. J. Hazard. Mater. 317, 503–542. doi: 10.1016/j.jhazmat.2016.06.020
Lourenço, J., Pereira, R., Gonçalves, F., and Mendo, S. (2013). Metal bioaccumulation, genotoxicity and gene expression in the European wood mouse (Apodemus sylvaticus) inhabiting an abandoned uranium mining area. Sci. Total Environ. 443, 673–680. doi: 10.1016/j.scitotenv.2012.10.105
Møller, A. P., Hobson, K. A., Mousseau, T. A., and Peklo, A. M. (2006). Chernobyl as a population sink for barn swallows: tracking dispersal using stable-isotope profiles. Ecol. Appl. 16, 1696–1705. doi: 10.1890/1051-0761(2006)016[1696:CAAPSF]2.0.CO;2
Møller, A. P., and Mousseau, T. A. (2013). Assessing effects of radiation on abundance of mammals and predator-prey interactions in Chernobyl using tracks in the snow. Ecol. Indic. 26, 112–116. doi: 10.1016/j.ecolind.2012.10.025
Møller, A. P., and Mousseau, T. A. (2015). Strong effects of ionizing radiation from Chernobyl on mutation rates. Sci. Rep. 5:8363. doi: 10.1038/srep08363
Møller, A. P., and Mousseau, T. A. (2006). Biological consequences of Chernobyl: 20 years on. Trends Ecol. Evol. 21, 200–207. doi: 10.1016/j.tree.2006.01.008
Nikitaki, Z., Pavlopoulou, A., Holá, M., Donà, M., Michalopoulos, I., Balestrazzi, A., et al. (2017). Bridging plant and human radiation response and DNA repair through an in silico approach. Cancers 9:E65. doi: 10.3390/cancers9060065
Rodgers, B. E., and Baker, R. J. (2000). Frequencies of micronuclei in bank voles from zones of high radiation at chornobyl, Ukraine. Environ. Toxicol. Chem. 19, 1644–1648. doi: 10.1002/etc.5620190623
Rozen, S., and Skaletsky, H. (2000). Primer3 on the WWW for general users and for biologist programmers. Methods Mol. Biol. 132, 365–386. doi: 10.1385/1-59259-192-2:365
Stryker, J. A. (2007). Science to practice: why is the liver a radiosensitive organ? Radiology 242, 1–2. doi: 10.1148/radiol.2421061103
Tribius, S., Pidel, A., and Casper, D. (2001). ATM protein expression correlates with radioresistance in primary glioblastoma cells in culture. Int. J. Radiat. Oncol. Biol. Phys. 50, 511–523. doi: 10.1016/S0360-3016(01)01489-4
Vandesompele, J., De Preter, K., Pattyn, F., Poppe, B., Van Roy, N., De Paepe, A., et al. (2002). Accurate normalization of real-time quantitative RT-PCR data by geometric averaging of multiple internal control genes. Genome Biol. 3:RESEARCH0034. doi: 10.1186/gb-2002-3-7-research0034
von Zglinicki, T. (2002). Oxidative stress shortens telomeres. Trends Biochem. Sci. 27, 339–344. doi: 10.1016/S0968-0004(02)02110-2
Ward, J. F. (1988). DNA damage produced by ionizing radiation in mammalian cells: identities, mechanisms of formation, and reparability, Prog. Nucleic Acid Res. Mol. Biol. 35, 95–125.
Ward, J. F. (1990). The yield of DNA double-strand breaks produced intracellularly by ionizing radiation: a review. Int. J. Radiat. Biol. 57, 1141–1150. doi: 10.1080/09553009014551251
Keywords: chernobyl, ionizing radiation, DNA damage, DNA repair, oxidative stress, Atm, Mre11
Citation: Jernfors T, Kesäniemi J, Lavrinienko A, Mappes T, Milinevsky G, Møller AP, Mousseau TA, Tukalenko E and Watts PC (2018) Transcriptional Upregulation of DNA Damage Response Genes in Bank Voles (Myodes glareolus) Inhabiting the Chernobyl Exclusion Zone. Front. Environ. Sci. 5:95. doi: 10.3389/fenvs.2017.00095
Received: 22 September 2017; Accepted: 20 December 2017;
Published: 09 January 2018.
Edited by:
Rajeshwar P. Sinha, Banaras Hindu University, IndiaReviewed by:
Alexandros G. Georgakilas, National Technical University of Athens, GreeceGiovanni Cenci, Sapienza Università di Roma, Italy
Alma Balestrazzi, University of Pavia, Italy
Copyright © 2018 Jernfors, Kesäniemi, Lavrinienko, Mappes, Milinevsky, Møller, Mousseau, Tukalenko and Watts. This is an open-access article distributed under the terms of the Creative Commons Attribution License (CC BY). The use, distribution or reproduction in other forums is permitted, provided the original author(s) or licensor are credited and that the original publication in this journal is cited, in accordance with accepted academic practice. No use, distribution or reproduction is permitted which does not comply with these terms.
*Correspondence: Toni Jernfors, dG9uaS5qZXJuZm9yc0BvdWx1LmZp