- Department of Civil, Construction and Environmental Engineering, Marquette University, Milwaukee, WI, United States
Conventional petroleum-derived plastics are recalcitrant to biodegradation and can be problematic as they accumulate in the environment. In contrast, it may be possible to add novel, biodegradable bioplastics to anaerobic digesters at municipal water resource recovery facilities along with primary sludge to produce more biomethane. In this study, thermal and chemical bioplastic pretreatments were first investigated to increase the rate and extent of anaerobic digestion. Subsequently, replicate, bench-scale anaerobic co-digesters fed synthetic primary sludge with and without PHB bioplastic were maintained for over 170 days. Two polyhydroxybutyrate (PHB), one poly(3-hydroxybutyrate-co-4-hydroxybutyrate) and one polylactic acid (PLA) bioplastic were investigated. Biochemical methane potential (BMP) assays were performed using both untreated bioplastic as well as bioplastic pretreated at elevated temperature (35–90°C) under alkaline conditions (8<pH<12) for 3–48 h. PHB and PLA pretreatment increased average BMP values up to over 100%. Average PHB lag time before methane production started decreased when pretreatment was performed. Bench-scale anaerobic co-digesters fed synthetic primary sludge with PHB bioplastic resulted in 80–98% conversion of two PHB bioplastics to biomethane and a 5% biomethane production increase at the organic loadings employed (sludge OLR = 3.6 g COD per L of reactor volume per day [g COD/LR-d]; bioplastic OLR = 0.75 g theoretical oxygen demand per L of reactor volume per day [ThOD/LR-d]) compared to digesters not fed bioplastics. Anaerobic digestion or co-digestion is a feasible management option for biodegradable plastics.
Introduction
Conventional plastics derived from petroleum are not biodegradable to a significant extent and result in accumulation of plastic waste in landfills or natural environments (Rostkowski et al., 2012). Conventional plastics accumulate most notably in oceans where they have been shown to disintegrate, forming microplastic particles that adsorb pollutants such as polychlorinated biphenyls (PCBs), pesticides, and phthalates (Andrady, 2011). Microplastic particles with sorbed pollutants can be consumed by marine organisms and enter the human food chain (Mato et al., 2001; Hammer et al., 2012).
To be considered biodegradable, bioplastics must exceed 90% carbon conversion to carbon dioxide during aerobic composting within 180 days (Brodhagen et al., 2017). Polyhydroxybutyrate (PHB) bioplastic is biodegraded in aerobic and anaerobic engineered processes as well as natural environments; however anaerobic co-digestion of PHB for the express purpose of waste management and renewable energy has not been investigated (Abou-Zeid et al., 2004; Volova et al., 2010; Gómez and Michel, 2013; Deroiné et al., 2014). Budwill et al. (1996) reported that PHB is anaerobically biodegradable in various scenarios but suggested that municipal anaerobic sewage sludge digesters were suitable PHB degrading environment to generate biomethane. PHB was shown to anaerobically biodegrade over 90% in 10 days at mesophilic conditions, whereas polylactic acid (PLA) only biodegraded 7% in 90 days even though it is considered to be industrially compostable under aerobic thermophilic conditions (Yagi et al., 2014). Despite lesser biodegradability, PLA is more readily available on the market today due to more efficient production at full scale (Kolstad et al., 2012; Gómez and Michel, 2013; Yagi et al., 2013, 2014).
To help mitigate the environmental concerns of conventional plastics, a more efficient coupling of bioplastic production and waste management should be developed (Gironi and Piemonte, 2011). According to cradle-to-gate lifecycle assessments (LCA), the biodegradable bioplastic PHB has potentially lower ecological impacts and global warming potential than conventional plastics if feedstocks are biobased and originate as by-products or wastes (Narodoslawsky et al., 2015). Other LCA researchers investigated PHB in a more holistic cradle-to-cradle scenario profiling an optimized process scheme with the assumption of complete biomethane recovery using anaerobic biodegradation and concluded that PHB was superior to conventional plastic in terms of global warming potential (Rostkowski et al., 2012). The assumption for complete biomethane recovery was described as an end of life option in which PHB was converted to biogas at an anaerobic digestion facility. Direct evidence supporting anaerobic digestion of bioplastics such as PHB to biomethane in a waste management scenario is limited. Anaerobic digestion feasibility is often assumed with results from anaerobic batch tests that may not accurately reflect operation of continuously fed digesters at quasi steady state.
Waste management and renewable energy generation from some biodegradable bioplastics could be achieved through anaerobic co-digestion using existing infrastructure and minimal process modification. With co-digestion, two or more feed materials, such as biodegradable plastic and municipal primary sludge, are fed to an anaerobic digester concomitantly. Co-digestion is implemented at some existing municipal water resource recovery facilities that often have excess capacity as well as boilers and electricity-generating equipment that employ biometahene (Navaneethan et al., 2011). Onsite storage of bioplastics, like PHB, could supplement anaerobic digestion by providing a dense source of carbon that may be utilized to blend with other influent waste streams. PHB has a bulk theoretical oxygen demand (ThOD) of 2,200 g ThOD/L, whereas synthetic municipal primary sludge contains approximately 50 g COD/L. In addition, Stroot et al. (2001) suggested a C:N ratio for anaerobic digestion in the range of 20:1–30:1, but municipal sewage sludge for digestion was found to have C:N ratios ranging from 6:1 to 16:1, whereas the bioplastics contain C, but no N; thus co-digestion of bioplastics can increase C:N ratio to suggested values as well as result in increased biomethane production for renewable energy generation.
Bioplastics, like PHB and PLA encountered in the consumer market, are water insoluble, hydrophobic polyesters that can be hydrolyzed by water soluble endogenous carboxylesterase enzymes secreted by microbes. Carboxylesterases, like PHA depolymerase or lipase, disrupt the ester linkages between bioplastic monomers and release them from bioplastic as water soluble molecules becoming bioavailable for microbial metabolism (Yoshie et al., 2002). An obligate anaerobic bacterium, Ilyobacter polytropus, was evaluated in pure culture and was found to ferment 3-hydroxybutyrate to acetate and butyrate (Stieb and Schink, 1984). In order to facilitate more rapid bioplastic transformation to biomethane on the time scale of municipal anaerobic digestion, the surface area could be increased through chemical and thermal processing and pretreatment. Abiotic hydrolysis or depolymerization of PHA bioplastics into monomeric constituents and intermediate breakdown products was demonstrated at a pH of 13 in 0.1 M sodium hydroxide aqueous solution at temperatures ranging from 60 to 70°C and various incubation periods (Yu et al., 2005). Over 70% abiotic degradation of PHB was demonstrated at 70°C in 4 M sodium hydroxide after 4 h of treatment. Treatment of PHB in acidic solutions of sulfuric acid (0.05–2 M) at 70°C for up to 14 h did not result in abiotic degradation (Yu et al., 2005). Near complete abiotic degradation of poly(3-hydroxybutyrate-co-3-hydroxyvalerate) (PHBV) was shown at 60°C in 0.1 M sodium hydroxide after 18 h of treatment (Myung et al., 2014). Thus, pretreatment in alkaline media at elevated temperatures induced polyester backbone hydrolysis resulting in release of water soluble breakdown products such as 3-hydroxybutyrate and crotonate, which have both been shown to support growth of strictly anaerobic microbes (Dörner and Schink, 1990; Janssen and Harfoot, 1990).
In this study, bioplastic thermal and chemical pretreatments were employed to increase the rate and extent of anaerobic digestion and co-digestion of commercially available PHB and PLA bioplastics. In order to elucidate the applicability of bioplastic pretreatments for anaerobic digestion and co-digestion, biochemical methane potential (BMP) assays were performed and methane yields were compared. Bench-scale anaerobic co-digestion of two PHB bioplastics, both pretreated and untreated, at quasi steady state with synthetic municipal primary sludge was then performed.
Materials and Methods
Bioplastics
Bioplastics tested include four PHB varieties including one poly(3-hydroxybutyrate-co-4-hydroxybutyrate) as well as one PLA (see Table 1). ENMAT™ Y3000 powder and Mirel™ F1006 bioplastics were produced through fermentation of D-glucose. The PHB copolymer Mirel™ M2100 (4.4% 4-hydroxybutyrate) was produced through fermentation of D-glucose and 1,4-butanediol. PHB produced by Mango Materials, Inc. was made from biomethane made from an anaerobic digester. The PLA Ingeo™ 2003D was obtained from a commercial, cold drink cup and may have contained other proprietary additives not reported by the manufacturer; this bioplastic was produced by fermentation of corn derived dextrose followed by polymerization.
Bioplastic Processing and Pretreatment
Bioplastics were processed using methods similar to those reported by others (Witt et al., 2001; Yagi et al., 2013). Briefly, pelletized or thermoformed bioplastic samples were immersed in a liquid nitrogen bath for approximately 5 min to make them brittle and easier to grind, mechanically ground in a laboratory blender with a stainless steel canister (Waring 700G Commercial Blender), and sieved to less than 0.15 mm particle size. All bioplastics evaluated, apart from methane-derived PHB manufactured by Mango Materials, were commercially available at the time of testing. The Mango Materials plastic was obtained from the manufacturer as a prototype sample that was not yet commercially available. The commercially available bioplastics contain additives such as plasticizers and inks that may have influenced anaerobic digestion results.
Processed bioplastics were pretreated to increase surface area or initiate depolymerization to facilitate increased biomethane evolution during anaerobic digestion and co-digestion. Pretreatments were performed for each bioplastic using two methods. The first method involved only thermal pretreatment. This was done at 35, 55, and 90°C for 3, 24, and 48 h at each temperature (9 different time-temperature conditions). The second method involved exposing the plastics to alkaline conditions with thermal pretreatment. Temperatures that resulted in the greatest 40-day BMP values using the first method were selected for subsequent alkaline-thermal testing at pH values of 8, 10, and 12 and incubation durations of 3, 24, and 48 h (3 pH values at 3 different holding times and 2 different temperatures yielded 18 different pretreatments for each bioplastic).
For pretreatment, a bioplastic suspension (25 g/L) in deionized water was placed into a 50 mL glass vial or 500 mL glass Erlenmeyer flask. The suspension was mixed with a magnetic stir bar and the pH was increased by sodium hydroxide addition. Thermal pretreatment was done in a water bath continuously mixed at 150 rpm on an orbital shaker (Stuart–Bibby Scientific SBS40 Shaking Water Bath). After thermal pretreatment, the slurry was allowed to cool to ambient temperature and the pH was adjusted to approximately 7 using hydrochloric acid. Pretreated, neutralized bioplastic suspensions were then dried with a laboratory air blowdown evaporator to facilitate more accurate substrate distribution on a mass basis for anaerobic digestion evaluation.
Untreated and pretreated PHB2 samples were observed by scanning electron microscope (SEM) imaging to visualize the physical effect of thermal alkaline pretreatment. Surface morphology was captured via JEOL JSM-6510LV SEM imaging (JEOL Ltd., Akishima, Tokyo, Japan) under high vacuum at an accelerating voltage of 20 kV and magnifications of x500 and x5,000. PHB particles were mounted to SEM specimen mounts with carbon tape and sputter-coated with gold and palladium to a thickness of approximately 200 Å (20 nm).
Biochemical Methane Potential (BMP) Assays
BMP assays were employed to evaluate biomethane yields from untreated and pretreated bioplastics and reported at 40-day test duration unless otherwise noted at 15 or 60 days. BMP assays were performed in triplicate as described elsewhere (Owen et al., 1979). Briefly, serum bottles (160 mL) were seeded with 50 mL of biomass and 5 mL of bioplastic slurry (25 g/L) containing either pretreated bioplastic, untreated bioplastic as negative control (NC), 5 mL of de-ionized water as blank control (BC), or 5 mL of glucose solution (13 g/L) as positive control (PC). Serum bottles were capped with butyl rubber stoppers (Geo-Microbial Technologies, Ochelata, OK) and crimped with aluminum seals. Setup was performed within a vinyl anaerobic glove box (Coy Laboratory Products, Grass Lake, MI) purged with nitrogen (N2) gas and less than one percent hydrogen (H2) gas. BMP assays were incubated (35°C) with constant orbital mixing at 150 rpm (New Brunswick Scientific—Model C25KC, Edison, NJ). Serum bottle biogas volume was measured intermittently with wetted glass barrel syringes at ambient pressure and 35°C, whereas serum bottle headspace methane concentration was determined by gas chromatography. All BMP values were calculated by subtracting the blank control biomethane production value from the BMP gross test value. Lag time was defined as the period between initiation of the BMP assay and the time when the biomethane production rate exceeded that of the blank control. Seed biomass was a mesophilic (35°C) laboratory-maintained methanogenic, anaerobic biomass (15.5 ± 0.2 g/L total solids [TS], 7.1 ± 0.2 g/L volatile solids [VS]) fed dry milk substrate (3.5 g/LR-day) and basal nutrient media (Supplementary Table 1) every day with a 15 day solids retention time (SRT) and continuous mixing. Biomass was stored for an average of approximately 1 week at 35°C in 1 L amber glass jars with loose-fitted lids to allow for gas evolution prior to BMP analyses.
Anaerobic Co-digesters
Synthetic municipal primary sludge (SMWS) was digested alone or was co-digested with either untreated or pretreated PHB1 and PHB2 (see Table 1 for bioplastic abbreviations) in duplicate anaerobic co-digesters (eight digesters total). Co-digesters were 2.5 L bench-scale, continuously stirred-tank reactors (CSTR) operated with a 15-day SRT and 15-day hydraulic residence time for 175 days. Conditions were maintained at 35.7°C ± 2.1% and a constant mixing rate of 350 rpm using a magnetic stir bar. Co-digesters were seeded with mesophilic municipal anaerobic biomass (VS = 3.5%) from the South Shore Water Reclamation Facility (Oak Creek, WI). SMWS was composed of basal nutrient media, alkalinity (Supplementary Table 1) and particulate substrate provided by ground dog food (1.21 ± 0.12 g COD/g dog food) sieved to less than 0.8 mm particle size having approximately 21% protein and 13% fat (Nutro Natural Choice, Franklin, TN, USA). However, dry dog food may not be a good substitute for waste activated sludge which includes biomass from the activated sludge process. SMWS was fed at an organic loading rate (OLR) of 3.6 g COD/LR-day which was equivalent to 7.5 g dog food/day (Carey et al., 2016). The bioplastic OLR was 0.75 g theoretical oxygen demand (ThOD) per liter of reactor per day (ThOD/LR-d) which was approxiumately 20% of the COD OLR from SMWS alone. Control digesters were fed SMWS and untreated PHB bioplastic as a codigestate.
SMWS was fed to all co-digesters without bioplastic from days 1 to 115; subsequently bioplastic was co-fed with SMWS from days 116 to 175. Digester performance was assessed by daily monitoring of temperature, pH, and biogas production as well as weekly biogas methane content, volatile fatty acids (VFA) concentrations, and solids analysis. Daily biogas volume produced was collected in gas sampling bags (Cole Parmer Kynar PVDF 20.3 L) and subsequently measured with a wet test meter (Precision Scientific). Bench scale anaerobic digestion lag time was defined as the period between day 115 when PHB co-digestion was initiated and the time when the rate of co-digester biomethane production exceeded that of the digester fed SMWS alone. Quasi steady state operation was defined as occurring after all digesters were operated under consistent conditions for at least three SRTs (i.e., 45 days) and all daily effluent COD concentrations and biogas production rate values did not vary more than 10%.
Analyses
Biogas was analyzed for methane content by gas chromatography with thermal conductivity detection (GC-TCD) (GC System 7890A, Agilent Technologies, Irving, TX, USA) and data were reported at 35°C and 1 atm. Total solids and VS concentration and COD were measured by standard methods (APHA, AWWA, WEF, 1999). VFA concentrations were determined by gas chromatography flame ionization detection (GC-FID) after samples were centrifuged, supernatant filtered through 0.45 μm syringe-tip filter, and acidified with phosphoric acid (Schauer-Gimenez et al., 2010). Since accurate bioplastic COD analysis was not achievable, the bioplastics ThOD values were calculated based on the bioplastic mass and molecular structure, with ratios of 1.67 g ThOD/g PHB and 1.33 g ThOD/g PLA. Bioplastics theoretical maximum methane production values (35°C, 1 atm) were calculated using the Buswell Equation (Buswell and Mueller, 1952) and were 0.66 L CH4/g PHB and 0.53 L CH4/g PLA. Statiscical analyses were performed in R Studio version 3.4.1. Normal distributions were not assumed, and significant differences among mean BMP values were determined using the non-parametric Mann-Whitney-Wilcoxon test with a confidence level of 0.95 and one-sided alternative hypothesis.
Results and Discussion
Bioplastic Pretreatment and BMP Assays
Pretreatment of PHB1 qualitatively resulted in visible surface erosion, increased porosity, and increased surface area compared to untreated (Figure 1). Increasing PHB surface area and porosity increases the available binding sites for biological enzymatic degradation and may therefore increase hydrolysis rates (Shang et al., 2012). Hydrolysis of recalcitrant substrates can be the rate-limiting step in methanogensis, thus pretreatments that can facilitate increased rates of hydrolysis may increase the rate of methanogenesis (Venkiteshwaran et al., 2015). Thermal alkaline pretreatment of PHB and PLA bioplastics increased anaerobic biodegradability in terms of increased BMP values and reduced lag time compared to untreated controls as described below.
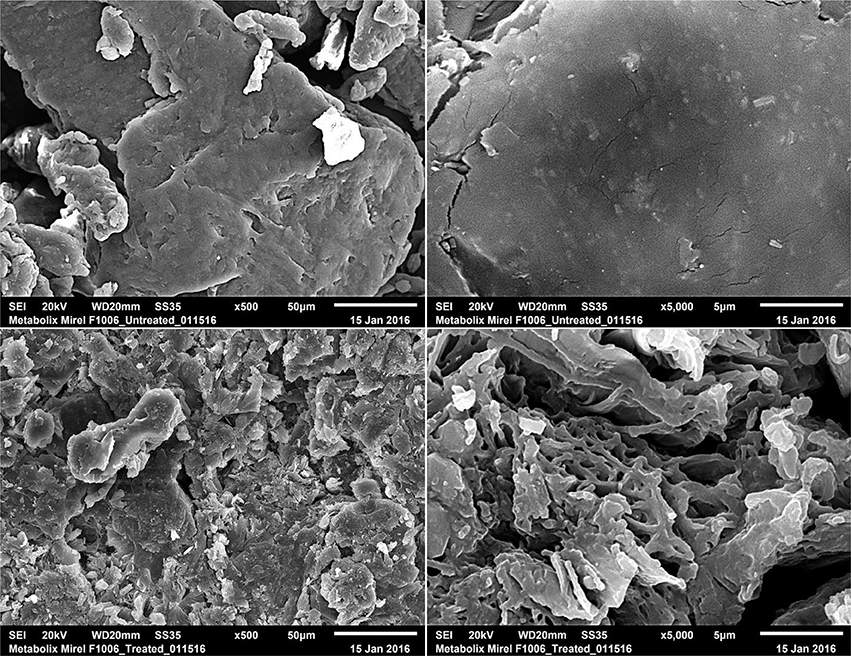
Figure 1. Scanning electron micrographs of untreated and pretreated PHB (Mirel™ F1006) after processing. Untreated PHB at magnification x500 (top, left) and x5,000 (top, right). Pretreated PHB at 500x (bottom, left) and 5,000x (bottom, right), pretreatment conditions were 90°C and pH 12 for 48 h.
BMP values and lag times resulting from 27 different pretreatment conditions (i.e., three temperatures at three pH values and three different contact times) for each bioplastic were determined and provided an initial assessment of biomethane production changes due to pretreatments for each bioplastic (see Supplementary Tables 2–6). Percent conversion values for PHB and PLA to biomethane were calculated as the quotient of BMP value divided by the theoretical maximum methane production value determined from the bioplastic ThOD loading. Compared to untreated bioplastics, pretreated PHB and PLA resulted in increased average BMP values. The pretreatment conditions resulting in the maximum increases in methane production are presented in Figure 2. Maximum percent conversion to biomethane for PHB was 101 ± 6% and 22 ± 6% for PLA after 40 days. Lag times of pretreated PHBs and PLA compared to untreated control digesters were reduced up to 60 and 98%, respectively.
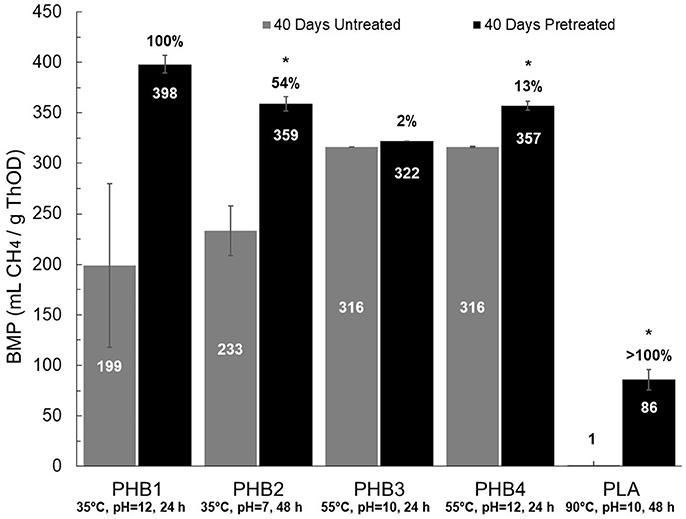
Figure 2. BMP values for untreated (gray) and pretreated (black) bioplastics under conditions resulting in the greatest biomethane increase. The specific conditions are written under each bar in the graph (temperature, pH, duration). BMP values, shown within each bar, with 40 days duration are reported at 35°C and ambient pressure. Percentages above black bars indicate relative increase from untreated to pretreated, with statistically significant differences at 95% confidence denoted by an asterisk (*). Error bars are relative standard deviation (n = 3); some error bars are small and not visible.
BMP values for pretreated PHBs averaged 360 ± 18 mL CH4/g ThOD (35°C, 1 atm) representing 91 ± 4% conversion to biomethane, whereas untreated PHBs averaged 270 ± 71 mL CH4/g ThOD and converted 67 ± 19% to biomethane (Figure 2). An additional 20 days of BMP analysis yielded averages of 101 ± 4% and 76 ± 17% conversion for pretreated and untreated PHBs, respectively. Pretreatment led to statistically significant increased BMP values for PHB2 and PHB4, but not for PHB1 and PHB3 (see Supplementary Tables 2–5). Although the average BMP value of pretreated PHB1 increased by 100% compared to that of the untreated PHB1, the difference was not statistically significant due to high variance in the untreated BMP measurements (RSD ± 81%).
PHB3 that was derived from methane exhibited rapid conversion to biomethane at 60 ± 1% after 15 days despite a negligible response to pretreatment. Other reports described untreated PHB conversion to biomethane at 39% in 5 days, 87% in 21 days, 92.5% in 22 days, and 100% in 98 days (Budwill et al., 1992, 1996; Yagi et al., 2014). Individual BMP results from each pretreated PHB vary, but the largest increase in BMP relative to untreated PHB were generally demonstrated at pretreatment conditions of 55°C, pH value of 12, and 24 or 48 h pretreatment duration, which agrees with reports concluding that abiotic pretreatment of PHB at elevated temperature and pH produced degradation products (Yu et al., 2005).
Compared to untreated PLA, pretreatment of PLA resulted in the largest increase in BMP of the bioplastics studied (Supplementary Table 6). Untreated PLA did not anaerobically degrade to biomethane, whereas pretreatment at 90°C, pH value at or above 7 for 48 significantly increased BMP to an average of 79 ± 8 mL CH4/g ThOD and equivalent to as much as 22 ± 6% conversion to biomethane. Extending the BMP analysis another 20 days resulted in an additional 5% conversion to biomethane for PLA. Low PLA conversion to biomethane under mesophilic conditions has been reported by others. Kolstad et al. (2012) observed no biomethane evolution in mesophilic anaerobic digesters after 170 days, whereas others reported low conversion to biomethane from 12% at 77 days, 23% at 182 days, and up to 49% after 277 days (Yagi et al., 2009, 2014). In contrast, thermophilic anaerobic digestion of PLA was reported to yield higher rates of digestion with nearly 25% conversion to biomethane in 30 days and up to 75% in 75 days (Yagi et al., 2013). One study attempted pretreatment of PLA at 70°C for 1 h with no pH control, but this resulted in less biomethane than untreated PLA (Endres and Siebert-Raths, 2011). Results from previous studies are in close accordance with the results herein. However, many of the previous investigations acclimated their seed inocula to enrich for bioplastic fermenting bacteria, whereas the work described herein did not. Therefore, the BMPs reported herein are for unacclimated biomass that may result in longer lag time and lesser biomethane production within 40 days.
Thermal alkaline pretreatment of bioplastics generally resulted in reduced lag time compared to untreated bioplastics. Average lag time for untreated PHBs was greater than that for pretreated PHB. Untreated PLA did not yield biomethane after 60 days, but pretreated PLA demonstrated no detectable lag time (Figure 3). Lag times of untreated PHB3 were longer than those for treated PHB2 and highlighted that some commercial PHBs may not anaerobically degrade quickly, especially when using unacclimated biomass. The PHB3 was notable in that pretreatment did not result in a decreased lag time, whereas lag times for all other PHBs and PLA were reduced. In the case of PLA, lag time was inversely correlated to pretreatment duration, with pretreatment times of 3, 24, and 48 h resulting in sequentially decreasing lag time of >3 weeks, 2 weeks, and no lag time, respectively (Figure 3E). Similarly, Yagi et al. (2009) reported a 55 day lag time for untreated PLA and others reported no anaerobic degradation for untreated PLA (Kolstad et al., 2012; Criddle and Billington, 2014). Yagi et al. (2014) suggested that mesophilic anaerobic microbial consortia may only have the ability to degrade low molecular weight PLA, and based on the BMP tests conducted here, it is possible that substantial methane production only occurred from low molecular weight PLA produced by thermal hydrolysis during pretreatments at 90°C and 48 h. Longer pretreatment duration of PLA correlated to decreased lag time to the point when 48 h of pretreatment eliminated lag time altogether. PLA pretreatment at alkaline pH at 90°C for durations longer than 48 h may result in increased BMP and potentially complete conversion to biomethane during anaerobic digestion.
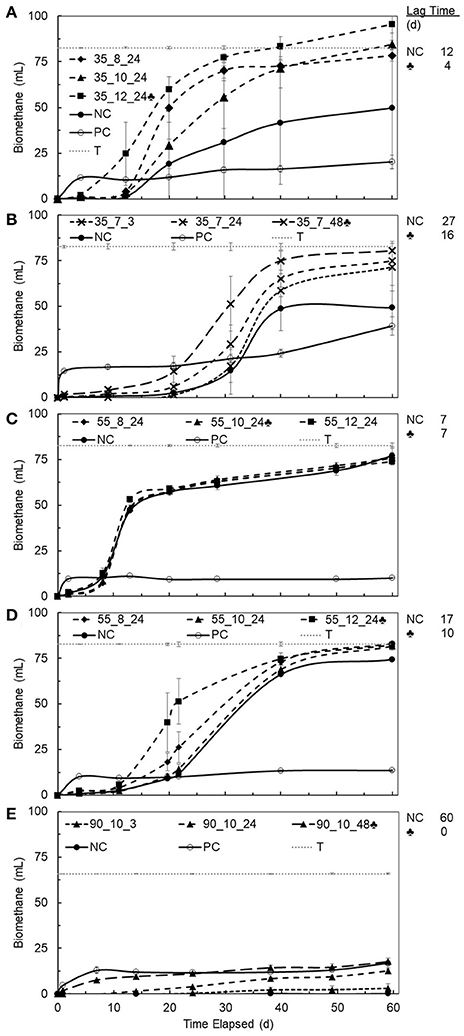
Figure 3. Average cumulative biomethane produced during BMP assays (n = 3, error bars and one standard deviation, 35°C, ambient pressure) vs. time elapsed for PHB1 (A), PHB2 (B), PHB3 (C), PHB4 (D), PLA (E) after pretreatment. Conditions of pretreatment are denoted on each chart as temperature, °C _ pH _ incubation time, h. Dashed lines show incubation times and pH 7 (x), pH 8 (♦), pH 10 (▴), pH 12 (■), and highest biomethane production (♣). Solid lines show controls; negative control (NC •) was untreated bioplastic, positive control (PC °) was glucose, dotted line denotes theoretical maximum (T) biomethane production, and lag time shown to the right of each chart.
Bench Scale Co-digestion
Co-digestion of SMWS and PHB was feasible at bench scale as evidenced by efficient biotransformation to biomethane, while pH, temperature, VFAs, and VS removal remained stable (Table 2, Supplementary Figures 1–3). When bioplastics were co-digested, biomethane production increased 17% over that from digesting SMWS alone. Quasi steady state co-digestion of SMWS and PHB, after 45 days exhibited approximately 80–98% conversion of PHB to biomethane (Table 2). Calculations for conversion percentage of bioplastic to biomethane relied upon theoretical biomethane yield.
Average pH of digester effluent fed SMWS alone was 7.30 ± 0.02, while pH in all digesters dropped slightly after PHB was fed to the digesters the pH difference was statistically significant during quasi steady state co-digestion with PHB at an average value 7.24 ± 0.02 (Supplementary Figure 1). VFA concentrations of digester effluent expressed as acetic acid equivalents were 48 ± 4 mg/L and 46 ± 3 mg/L before and during co-digestion at quasi steady state for all digesters, respectively, and were not statistically different (Supplementary Figure 2). The VS as a percent of TS in digester effluent deviated only 2% for all digesters and ranged between 57 and 59% (Supplementary Figure 3). The VS reduction (VSR) values increased for all digesters when PHB was co-digested and the average increased from as low as 75 ± 1% during SMWS digestion alone to as much as 81 ± 1% when bioplastic was co-digested. Solids initially increased in response to PHB addition but attained a quasi-steady state value after 15 days or one SRT. Average percent biomethane in biogas decreased from 2 to 3% when PHB was co-digested (Table 2), but the differences were not statistically significant.
In contrast to co-digestion of untreated PHB, co-digestion of pretreated PHB increased biomethane production by 5% and reduced lag time by approximately 4 days for both PHB1 and PHB2 (Figure 4). Lag time for bench scale co-digestion of PHB2 was 6 days for untreated and 3 days for pretreated bioplastic.
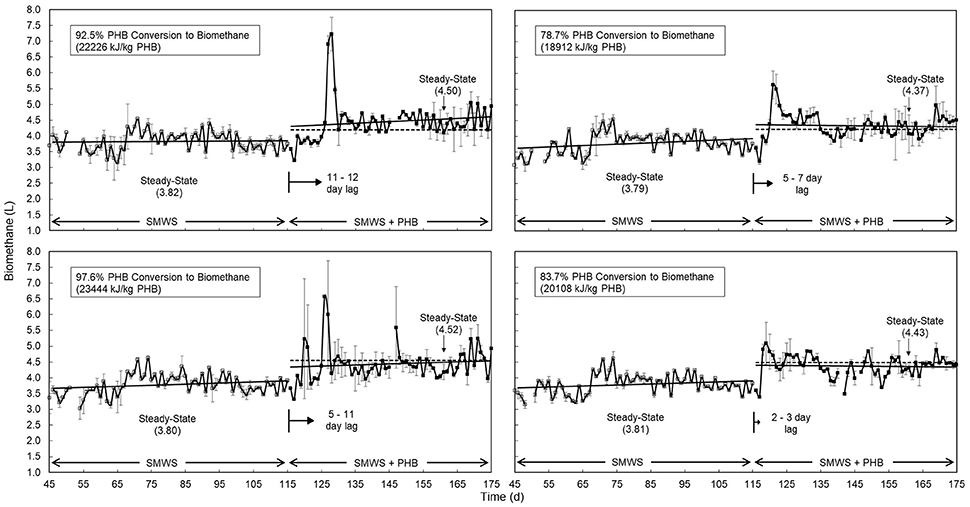
Figure 4. Anaerobic digester daily biomethane production (n = 2, error bars show standard deviation) comparing (top, left) untreated PHB1, (bottom, left) pretreated PHB1 (treatment: 55° C, pH = 12, 24 h) and (top, right) untreated PHB2, (bottom, right) pretreated PHB2 (55°C, pH = 12, 48 h). Quasi steady state was assumed after 45 days with average biomethane production (L/d) at quasi steady state presented in parentheses. Solid lines depict gas production rates before and after PHB co-digestion, dotted lines show theoretical co-digestion production based on 40 days BMPs. Solid arrows proportionately illustrate average lag period (d) between PHB addition and increased biomethane production. Steady state conversion of PHB to biomethane (%) and higher heating value of methane per kg PHB was based on an expected 21% increase in biomethane yield.
PHB co-digestion with synthetic primary sludge increased both the overall rate and extent of biomethane production compared to anaerobic digestion of synthetic primary sludge alone (Figure 4).
Conclusions
Biodegradable bioplastic can be co-digested under stable conditions at municipal water resource recovery facilities to generate renewable energy. Bioplastic pretreatment (≥55°C, pH ≥ 10, ≥24 h) resulted in more rapid and complete anaerobic bioplastic co-digestion. With pretreatment, partial anaerobic digestion of PLA was accomplished. In addition, thermal alkaline bioplastic pretreatment reduced lag time before biomethane production occurred and increased bioplastic conversion to biomethane. Pretreatment of PHB bioplastic under quasi steady state co-digestion conditions resulted in approximately 6% greater biomethane production compared to untreated PHB. Bioplastic co-digestion at the loadings used increased biomethane production by 17%.
Author Contributions
NB: performed experiments, performed data analysis and drafted the manuscript; DZ: planned the research, supervised the research and revised the manuscript.
Funding
This work was supported by the National Science Foundation under Award No. 1540010 to Marquette University for the Water Equipment and Policy Industry/University Cooperative Research Center.
Conflict of Interest Statement
The authors declare that the research was conducted in the absence of any commercial or financial relationships that could be construed as a potential conflict of interest.
Acknowledgments
The authors thank Mango Materials for providing PHB1, PHB2, and PHB3 and the Virginia Institute of Marine Science for providing PHB4.
Supplementary Material
The Supplementary Material for this article can be found online at: https://www.frontiersin.org/articles/10.3389/fenvs.2017.00093/full#supplementary-material
References
Abou-Zeid, D. M., Mulller, R. J., and Deckwer, W. D. (2004). Biodegradation of aliphatic homopolyesters and aliphatic-aromatic copolyesters by anaerobic microorganisms. Biomacromolecules 5, 1687–1697. doi: 10.1021/bm0499334
Andrady, A. L. (2011). Microplastics in the marine environment. Mar. Pollut. Bull. 62, 1596–1605. doi: 10.1016/j.marpolbul.2011.05.030
APHA, AWWA, WEF. (1999). Standard Methods for the Examination of Water and Wastewater, 20th Edn. Washington, DC: American Public Health Association Publications.
Brodhagen, M., Goldberger, J. R., Hayes, D. G., Inglis, D. A., Marsh, T. L., and Miles, C. (2017). Policy considerations for limiting unintended residual plastic in agricultural soils. Environ. Sci. Policy 69, 81–84. doi: 10.1016/j.envsci.2016.12.014
Budwill, K., Fedorak, P. M., and Page, W. J. (1992). Methanogenic degradation of poly(3-hydroxyalkanoates). Appl. Environ. Microbiol. 58, 1398–1401.
Budwill, K., Fedorak, P. M., and Page, W. J. (1996). Anaerobic microbial degradation of poly (3-hydroxyalkanoates) with various terminal electron acceptors. J. Environ. Polym. Degrad. 4, 91–102. doi: 10.1007/BF02074870
Buswell, A. M., and Mueller, H. F. (1952). Mechanism of methane fermentation. Ind. Eng. Chem. 44, 550–552. doi: 10.1021/ie50507a033
Carey, D. E., Zitomer, D. H., Hristova, K. R., Kappell, A. D., and McNamara, P. J. (2016). Triclocarban influences antibiotic resistance and alters anaerobic digester microbial community structure. Environ. Sci. Technol. 50, 126–134. doi: 10.1021/acs.est.5b03080
Criddle, C. S., and Billington, S. L. (2014). Renewable Bioplastics and Biocomposites from Biogas Methane and Waste Derived Feedstock: Development of Enabling Technology, Life Cycle Assessment, Analysis of Costs. Sacramento, CA: California Department of Resource Recycling and Recovery.
Deroiné, M., Le Duigou, A., Corre, Y. M., Le Gac, P. Y., Davies, P., César, G., et al. (2014). Seawater accelerated ageing of poly(3-hydroxybutyrate-co-3-hydroxyvalerate). Polym. Degrad. Stab. 105, 237–247. doi: 10.1016/j.polymdegradstab.2014.04.026
Dörner, C., and Schink, B. (1990). Clostridium homopropionicum sp. nov., a new strict anaerobe growing with 2-, 3-, or 4-hydroxybutyrate. Arch. Microbiol. 154, 342–348. doi: 10.1007/BF00276529
Endres, H.-J., and Siebert-Raths, A. (eds.). (2011). “End-of-Life options for biopolymers,” in Engineering Biopolymers (Munich: Carl Hanser Verlag GmbH & Co. KG), 225–243.
Gironi, F., and Piemonte, V. (2011). Bioplastics and petroleum-based plastics: strengths and weaknesses. Energy Sources Part A Recover. Util. Environ. Eff. 33, 1949–1959. doi: 10.1080/15567030903436830
Gómez, E. F., and Michel, F. C. (2013). Biodegradability of conventional and bio-based plastics and natural fiber composites during composting, anaerobic digestion and long-term soil incubation. Polym. Degrad. Stab. 98, 2583–2591. doi: 10.1016/j.polymdegradstab.2013.09.018
Hammer, J., Kraak, M. H. S., and Parsons, J. R. (2012). “Plastics in the marine environment: the dark side of a modern gift,” in Reviews of Environmental Contamination and Toxicology, ed D. M. Whitacre (New York, NY: Springer), 1–44.
Janssen, P. H., and Harfoot, C. G. (1990). Ilyobacter delafieldii sp. nov., a metabolically restricted anaerobic bacterium fermenting PHB. Arch. Microbiol. 154, 253–259. doi: 10.1007/BF00248964
Kolstad, J. J., Vink, E. T. H., De Wilde, B., and Debeer, L. (2012). Assessment of anaerobic degradation of IngeoTM polylactides under accelerated landfill conditions. Polym. Degrad. Stab. 97, 1131–1141. doi: 10.1016/j.polymdegradstab.2012.04.003
Mato, Y., Isobe, T., Takada, H., Kanehiro, H., Ohtake, C., and Kaminuma, T. (2001). Plastic resin pellets as a transport medium for toxic chemicals in the marine environment. Environ. Sci. Technol. 35, 318–324. doi: 10.1021/es0010498
Myung, J., Strong, N. I., Galega, W. M., Sundstrom, E. R., Flanagan, J. C. A., Woo, S. G., et al. (2014). Disassembly and reassembly of polyhydroxyalkanoates: recycling through abiotic depolymerization and biotic repolymerization. Bioresour. Technol. 170, 167–174. doi: 10.1016/j.biortech.2014.07.105
Narodoslawsky, M., Shazad, K., Kollmann, R., and Schnitzer, H. (2015). LCA of PHA production - identifying the ecological potential of bio-plastic. Chem. Biochem. Eng. Q. 29, 299–305. doi: 10.15255/CABEQ.2014.2262
Navaneethan, N., Topczewski, P., Royer, S., and Zitomer, D. (2011). Blending anaerobic co-digestates: synergism and economics. Water Sci. Technol. 63:2916. doi: 10.2166/wst.2011.557
Owen, W. F., Stuckey, D. C., Healy, J. B., Young, L. Y., and McCarty, P. L. (1979). Bioassay for monitoring biochemical methane potential and anaerobic toxicity assay. Water Res. 13, 485–492. doi: 10.1016/0043-1354(79)90043-5
Rostkowski, K. H., Criddle, C. S., and Lepech, M. D. (2012). Cradle-to-gate life cycle assessment for a cradle-to-cradle cycle: biogas-to-bioplastic (and back). Environ. Sci. Technol. 46, 9822–9829. doi: 10.1021/es204541w
Schauer-Gimenez, A. E., Zitomer, D. H., Maki, J. S., and Struble, C. A. (2010). Bioaugmentation for improved recovery of anaerobic digesters after toxicant exposure. Water Res. 44, 3555–3564. doi: 10.1016/j.watres.2010.03.037
Shang, L., Fei, Q., Zhang, Y. H., Wang, X. Z., Fan, D., and Di Chang, H. N. (2012). Thermal properties and biodegradability studies of Poly(3-hydroxybutyrate-co-3-hydroxyvalerate). J. Polym. Environ. 20, 23–28. doi: 10.1007/s10924-011-0362-9
Speece, R. E. (2008). Anaerobic Biotechnology and Odor/Corrosion Control for Municipalities and Industries. Nashville, TN: Archae Press
Stieb, M., and Schink, B. (1984). A new 3-hydroxybutyrate fermenting anaerobe, Ilyobacter polytropus, gen. nov. sp. nov., possessing various fermentation pathways. Arch. Microbiol. 140, 139–146. doi: 10.1007/BF00454916
Stroot, P. G., McMahon, K. D., Mackie, R. I., and Raskin, L. (2001). Anaerobic codigestion of municipal solid waste and biosolids under various mixing conditions—I. digester performance. Wat. Res 35, 1804–1816. doi: 10.1016/S0043-1354(00)00439-5
Venkiteshwaran, K., Bocher, B., Maki, J., and Zitomer, D. (2015). Relating anaerobic digestion microbial community and process function. microbiol. Insights 8, 37–44. doi: 10.4137/MBI.S33593
Volova, T. G., Boyandin, A. N., Vasiliev, A. D., Karpov, V. A., Prudnikova, S. V., Mishukova, O. V., et al. (2010). Biodegradation of polyhydroxyalkanoates (PHAs) in tropical coastal waters and identification of PHA-degrading bacteria. Polym. Degrad. Stab. 95, 2350–2359. doi: 10.1016/j.polymdegradstab.2010.08.023
Witt, U., Einig, T., Yamamoto, M., Kleeberg, I., Deckwer, W.-D., and Müller, R.-J. (2001). Biodegradation of aliphatic–aromatic copolyesters: evaluation of the final biodegradability and ecotoxicological impact of degradation intermediates. Chemosphere 44, 289–299. doi: 10.1016/S0045-6535(00)00162-4
Yagi, H., Ninomiya, F., Funabashi, M., and Kunioka, M. (2009). Anaerobic biodegradation tests of poly(lactic acid) under mesophilic and thermophilic conditions using a new evaluation system for methane fermentation in anaerobic sludge. Int. J. Mol. Sci. 10, 3824–3835. doi: 10.3390/ijms10093824
Yagi, H., Ninomiya, F., Funabashi, M., and Kunioka, M. (2013). Thermophilic anaerobic biodegradation test and analysis of eubacteria involved in anaerobic biodegradation of four specified biodegradable polyesters. Polym. Degrad. Stab. 98, 1182–1187. doi: 10.1016/j.polymdegradstab.2013.03.010
Yagi, H., Ninomiya, F., Funabashi, M., and Kunioka, M. (2014). Mesophilic anaerobic biodegradation test and analysis of eubacteria and archaea involved in anaerobic biodegradation of four specified biodegradable polyesters. Polym. Degrad. Stab. 110, 278–283. doi: 10.1016/j.polymdegradstab.2014.08.031
Yoshie, N., Oike, Y., Kasuya, K. I., Doi, Y., and Inoue, Y. (2002). Change of surface structure of poly(3-hydroxybutyrate) film upon enzymatic hydrolysis by PHB depolymerase. Biomacromolecules 3, 1320–1326. doi: 10.1021/bm020077a
Keywords: methanogen, polyhydroxybutyrate, poly(3-hydroxybutyrate-co-4-hydroxybutyrate, polylactic acid, plastics
Citation: Benn N and Zitomer D (2018) Pretreatment and Anaerobic Co-digestion of Selected PHB and PLA Bioplastics. Front. Environ. Sci. 5:93. doi: 10.3389/fenvs.2017.00093
Received: 01 August 2017; Accepted: 15 December 2017;
Published: 04 January 2018.
Edited by:
Sabine Kleinsteuber, Helmholtz-Zentrum für Umweltforschung (UFZ), GermanyReviewed by:
Jo De Vrieze, Ghent University, BelgiumStefan Junne, Technische Universität Berlin, Germany
Copyright © 2018 Benn and Zitomer. This is an open-access article distributed under the terms of the Creative Commons Attribution License (CC BY). The use, distribution or reproduction in other forums is permitted, provided the original author(s) or licensor are credited and that the original publication in this journal is cited, in accordance with accepted academic practice. No use, distribution or reproduction is permitted which does not comply with these terms.
*Correspondence: Daniel Zitomer, ZGFuaWVsLnppdG9tZXJAbXUuZWR1