- 1Department of Crop and Soil Sciences, Washington State University, Pullman, WA, United States
- 2Department of Crop and Soil Sciences, Dryland Research Station, Washington State University, Lind, WA, United States
- 3Center for Sustaining Agriculture and Natural Resources, Washington State Uninversity, Mt. Vernon, WA, United States
- 4NE-SARE, Pennsylvania State University, State College, PA, United States
- 5Department of Soil and Water Systems, University of Idaho, Moscow, ID, United States
- 6School of Economic Sciences, Washington State University, Pullman, WA, United States
- 7Columbia Basin Agricultural Research Center, Oregon State University, Pendleton, OR, United States
- 8Boundary County Idaho Extension, University of Idaho, Bonners Ferrry, ID, United States
- 9Northwest Sustainable Agroecosystems Research Unit, Agricultural Research Service (USDA), Washington State University, Pullman, WA, United States
- 10Adams County Extension, Washington State University, Ritzville, WA, United States
- 11Grassland, Soil and Water Research Laboratory, Agricultural Research Service (USDA), Temple, TX, United States
- 12Biological Systems Engineering, Washington State University, Pullman, Washington, DC, United States
- 13Department of Entomology, Plant Pathology and Nematology, University of Idaho, Moscow, ID, United States
Climate-friendly best management practices for mitigating and adapting to climate change (cfBMPs) include changes in crop rotation, soil management and resource use. Determined largely by precipitation gradients, specific agroecological systems in the inland Pacific Northwestern U.S. (iPNW) feature different practices across the region. Historically, these farming systems have been economically productive, but at the cost of high soil erosion rates and organic matter depletion, making them win-lose situations. Agronomic, sociological, political and economic drivers all influence cropping system innovations. Integrated, holistic conservation systems also need to be identified to address climate change by integrating cfBMPs that provide win-win benefits for farmer and environment. We conclude that systems featuring short-term improvements in farm economics, market diversification, resource efficiency and soil health will be most readily adopted by farmers, thereby simultaneously addressing longer term challenges including climate change. Specific “win-win scenarios” are designed for different iPNW production zones delineated by water availability. The cfBMPs include reduced tillage and residue management, organic carbon (C) recycling, precision nitrogen (N) management and crop rotation diversification and intensification. Current plant breeding technologies have provided new cultivars of canola and pea that can diversify system agronomics and markets. These agronomic improvements require associated shifts in prescriptive, precision N and weed management. The integrated cfBMP systems we describe have the potential for reducing system-wide greenhouse gas (GHG) emissions by increasing soil C storage, N use efficiency (NUE) and by production of biofuels. Novel systems, even if they are economically competitive, can come with increased financial risk to producers, necessitating government support (e.g., subsidized crop insurance) to promote adoption. Other conservation- and climate change-targeted farm policies can also improve adoption. Ultimately, farmers must meet their economic and legacy goals to assure longer-term adoption of mature cfBMP for iPNW production systems.
Introduction
Agriculture is an important player in climate change. The contributions of global agriculture to greenhouse gas (GHG) emissions and climate change are well recognized (Reicosky et al., 2000; Snyder et al., 2009), setting the stage for agriculture to play a positive role in GHG mitigation through implementation of numerous agronomic practices (Smith et al., 2007; USDA-ERS, 2016). Furthermore, innovations are needed to increase resilience of agricultural systems to climate change and to exploit the opportunities that climate changes present (IPCC (International Panel on Climate Change), 2007a,b). The United States Department of Agriculture (USDA) established goals for climate change mitigation and adaptation in its 2010–2014 and 2014–2018 strategic plans (USDA, 2010, 2014). Performance measures included tracking crop production, fertilizer use and conservation practices. The U.S. General Accounting Office (GAO) requires USDA to provide these performance measures to inform Congress and the general public about the USDA program progress. It has determined that USDA programs face major challenges in encouraging farmers to modify farming practices geared toward climate change adaptation and mitigation. A report to the Energy and Commerce Committee of the U.S. House of Representatives made the following recommendations: (i) translate, distill and deliver climate science research information in user-friendly formats and tools, (ii) provide financial incentives to encourage farmer adoption of what we will refer to as “climate-friendly Best Management Practices” (cfBMPs), and (iii) provide crop producers with farm level economic enterprise costs and returns of adapting cfBMPs, with the guiding principle of identifying systems that provide economically attractive pathways to advancing farm level climate change adaptation and mitigation (GAO, 2014). Addressing these recommendations has required detailed characterization and modeling of biophysical drivers associated with crop growth and production across different agroecological cropping zones. It has also required an understanding of the principle drivers of whole cropping patterns linked to changes in weather (Stöckle et al., 2017) and other drivers that influence farmer decision making.
The recommendation of the Energy and Commerce Committee will have different implications for specific crops and growing regions. This review focuses on wheat (Triticum aestivum)-based cropping systems of the Inland Pacific Northwest of the USA (iPNW), and has three objectives: (i) describe the historical evolution of iPNW wheat (Triticum aestivum) based cropping systems and efforts to achieve economic and environmental goals, (ii) review farm-level biophysical, socio-economic and agronomic decision drivers that include cfBMPs and potentially shape win-win scenarios across iPNW agroecological zones, and (iii) describe integrated cfBMPs' potential abilities to improve adaptability and flexibility of cropping systems that also contribute to system-wide GHG reductions. Its overall goal is to use historical lessons of multidisciplinary and integrated research, extension and stakeholder engagement to define pathways toward simultaneously achieving farm economic, legacy and climate change goals.
Win-win scenarios are defined herein as mature cropping systems with integrated cfBMPs that achieve shorter-term goals by improving farm profitability and building a stable farm legacy, while enabling longer-term GHG mitigation and flexible adaptability to annual weather and long term climate change. Synergistic impacts occur when multiple management strategies are integrated into whole cropping systems (Zentner et al., 2002), potentially creating these win-win cropping systems.
Developing and sustaining win-win scenarios relies on progressive farmers who are economically motivated to change and adapt new cfBMP integrated systems. These new systems must align with climate friendly iPNW farming goals (Kruger, 2004) by integrating agronomic management variables: conservation tillage and residue management, organic carbon (C) and nutrient recycling, refined N management, and crop diversification and intensification. All are well-recognized conservation management strategies and now they are also recognized for their potential to help dryland farmers achieve climate change adaptation and GHG mitigation (CGIAR, 2012).
Historical Lessons on Moving Toward Win-Win Cropping Systems
The history of iPNW wheat production is marked by a recurring theme of tradeoffs between farm profitability and the deterioration of soil health, air and water quality, organic matter and nutrient depletion (Table 1). The region has been dominated by soil-erosive but profitable cereal-fallow farming with little crop diversification (Schillinger and Papendick, 2008), despite recognition of soil deterioration during the first generation of regional wheat farming (Spillman, 1906; Sievers and Holtz, 1922). A similar history of wheat farming and soil degradation has also been documented in the Canadian western prairies (Janzen, 2001). Balancing farm profits while reducing environmental degradation is a main challenge in identifying effective win-win strategies for the iPNW and similar wheat growing regions.
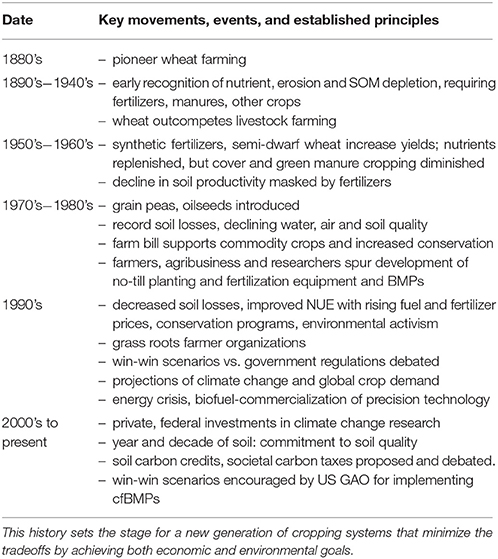
Table 1. Historical timeline of movements, events and principles established in the inland Pacific Northwestern US (iPNW) wheat-based agriculture, and resulting tradeoffs between short-term economics and long-term impacts on soil, air and water quality.
Recurring History of Economic and Environmental Tradeoffs
The history of soil management in the iPNW mirrors that of the rest of the U.S. Individual land ownership and management on American farms forced pioneer farmers to choose between economic survival and soil stewardship, since soil rebuilding was slow, costly and sometimes not achievable with the available resources. Early government programs only addressed farmers' economic concerns without prioritizing land stewardship (Gray et al., 1938). Further compounding the problem, the iPNW evolved toward the segregation of animal and cropping systems soon after settlement, with wheat farming displacing sheep, cattle and hog ranching (McGregor, 1982). This regional trend of crop-animal segregation reflected a broader global disruption of organic nutrient cycling and C inputs (Magdoff et al., 1997), which was further accelerated by the advent of inexpensive fertilizers and global grain marketing that made exclusive crop farming more profitable regionally (McGregor, 1982) and globally (Kirkegaard et al., 2011). Lack of organic inputs combined with accelerated soil erosion contributed to the rapid decline of soil organic matter (SOM), native fertility and overall soil health and productivity (Spillman, 1906; Albrecht, 1938). Early conservation principles were primarily focused on using fertilizers and lime to replace depleted soil nutrients that were removed from the crop-soil system by grain harvest (Fulmer and Heileman, 1899). Soil restorative practices such as integration of grass pastures and animal manure, and rotating with grain legumes and mustards were recognized (Spillman, 1906), but not widely adopted. As a result, SOM continued to decline in the iPNW, with soil erosion occurring at annual rates of 10 to more than 67 MT/ha (Kaiser et al., 1954; Schillinger and Papendick, 2008). Conventional tillage and fallowing have been the primary contributors to SOM decline in the iPNW (Machado, 2011). Gray et al. (1938) attributed the lack of significant progress in adopting soil conserving practices to a failure to address short-term economic needs of farmers.
In 1975, iPNW research and extension programs, Solutions to Economic and Environmental Problems (USDA-funded STEEP, nd1; Oldenstadt et al., 1982) followed by the USDA-funded Columbia Plateau PM10 (CP3) project were established with specific goals to develop and implement economically viable solutions to reduce water and wind erosion. Viable reduced- and no-tillage systems were the major research outcomes of these projects, followed by vigorous extension efforts to translate the research into grower-adaptable individual best management practices, principally outlined in compendiums of soil management guides (STEEP nd1, Papendick et al., 1985). The projects fostered collaborations among researchers, farmers, crop advisors and agribusinesses. Significant gains in conservation farming were documented, but adoption was not universal (Kok et al., 2009).
The Need for Integrated Systems
Research focused on specific practices such as tillage, however successful, failed to address the system-wide changes and farm scale economic implications required to incentivize farmer adoption. In addition, specific economic drivers can distort system-wide management for sustainability. Through the 1980s, commodity-based subsidies and growing global markets had fostered the winter wheat dominated cropping system in the region without regard to pest problems and erosion (Young et al., 1994a). Up to the mid-1980s producers moldboard-plowed their fields to bury residue and weed seeds prior to planting the following year's wheat crop. In addition, rotations with spring pea (Pisum sativum) improved wheat yields, but required eight tillage operations between wheat harvest to post plant spring pea. In 1985, the USDA-ARS initiated a field-size, long-term Integrated Pest Management (IPM) research project for conservation crop production in the PNW (Young et al., 1994a). This was the first farm scale, multi-and interdisciplinary cropping systems research project that focused on weed management integrated with conservation tillage in the iPNW. This project demonstrated that integrated cropping systems research could lead to less risky alternative cropping systems that could motivate farmer adoption (Young D. L. et al., 1994).
Cropping system comparisons of variable costs demonstrated that reduced fuel and number of field operations with direct seeding are somewhat offset by the increased herbicide costs associated with the practice. The cost tradeoffs coupled with direct seed grain yields result in similar economic returns in the intermediate zone of eastern Washington (Esser and Jones, 2013) and the drier grain-fallow regions of eastern Oregon (Machado et al., 2015) when compared to yields obtained with conventional tillage. Comparisons of conservation-till 4-year crop rotation systems and conventional-till 2-year wheat-fallow rotation systems also demonstrated a marked reduction in water runoff and soil erosion in the conservation-till systems (Williams et al., 2013). Similar results obtained in drier traditional iPNW wheat-fallow systems led Janosky et al. (2002) to conclude that since no “short- or long-term economic sacrifice” was required to shift to a minimum tillage system when using existing on-farm equipment, a neutral-win solution to soil erosion could be achieved when reduced-till and conventional-till wheat yields were comparable. Yet, strict no-till systems required fixed-cost investments in expensive, specialized direct seeding equipment, so these systems had only been moderately adopted (Kok et al., 2009). While projects like the USDA IPM project, described above, developed integrated strategies identified as essential for protecting soil and water quality by the USDA (Batie et al., 1993) and encouraged early adopting farmers to try direct seeding, they fell short in economically motivating farmers into universal adoption of these no-till systems that required new equipment. The economic advantages of direct-seeded wheat cropping systems combined with alternative crop sequences, tailored N and weed management were later demonstrated as potential win-win scenarios for direct-seeded dryland wheat systems in western Canada (Smith et al., 2006).
Conservation Farming Enables Climate Change Adaptation and Mitigation
Now, new environmental challenges imposed by climate change require proactive changes in cropping systems, but history dictates that initial adoption may also require economic incentives. Well-recognized best management for protecting soil health, and air and water quality also have the ability to foster climate adaptive and GHG-mitigating systems, often by incorporating elements designed to conserve soil and nutrient resources. Furthermore, the same cropping strategies that focus on building soil, protecting air and water quality, and supplying crop nutrients have the ability to reduce costs associated nutrient inputs and losses. The US Government Accounting Office cited the USDA programming and performance goals on soil and water conservation and quality as examples of needed elements for USDA's current climate change strategic plan (GAO, 2014).
Complex Factors Driving Farmers' Cropping System Decisions
To translate climate change science into adoption of transformative systems, it is important to focus on decision drivers from a farmer's perspective (Reganold et al., 2011). The farmer's decision process involves information gathering from multiple sources (Figure 1). Historically, farmers have been challenged with seasonal fluctuations in weather, farm input prices, as well as local and global competition and markets (Coughenour and Chamala, 2000). They must assimilate massive amounts of information along all of these fronts in order to make appropriate agronomic management decisions with their foremost goals of sustaining a business, raising a family, supporting their community while enjoying farming life and building their farm legacies (Jonovic, 1997; Figure 1). There is a need for integrated efforts of researchers, extension advisors, environmental stakeholders, market end-users and policymakers to develop and drive cropping system solutions (Smit and Skinner, 2002) that are feasible for farmers to adopt at a local level (Caron et al., 2014). Farmers are familiar with the proven conservation strategies and affiliated government support policies. Many of these same practices are now recognized to provide climate change benefits, so current climate change research and extension can focus on improving and integrating these practices, rather than promoting substantial new changes that inherently come with greater risks and uncertainty. A focus on farmers' immediate concerns and priorities, with an emphasis on familiar conservation practices already supported by environmental stakeholders and policymakers, can help drive farm planning that includes agronomic management shifts that also provide tangential benefits to address climate change (Howden et al., 2007).
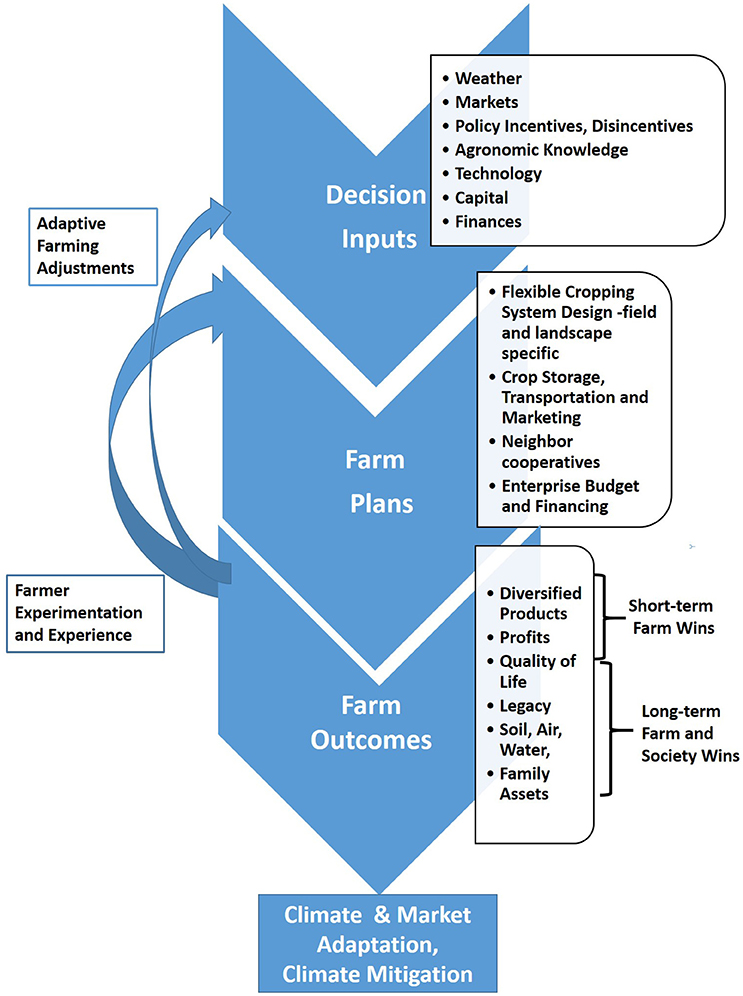
Figure 1. Input drivers of farmer decisions and plans targeting short- and long-term farm outcomes, with government incentives and disincentives targeting long-term societal wins. Successes or failures in accomplishment of farmers' primary goals provide feedback for managing flexible, evolving farming systems. Win-win systems feature improved farm adaptability to markets and climate, while increasing greenhouse gas (GHG) mitigation as co-products.
Biophysical Drivers of iPNW Alternative Cropping Systems
Farmers are very aware of seasonal weather variability and ways to deal with it. Furthermore, they generally acknowledge that weather patterns have recently shifted (Seamon et al., 2016). In support of these recently observed shifts, climate change models project temporal and spatial long-term shifts in temperature and precipitation means and extremes. In general, the iPNW is predicted to experience warmer, wetter winters; more frost-free days; drier, hotter summers; and more variability in temperature and precipitation (Stöckle et al., 2010; Abatzoglou et al., 2014). These projections suggest a need for flexible, diversified systems that enable nimble farm adaptability to climate change, as well as mitigating GHG. For example, future systems that encourage greater water infiltration and soil water storage combined with greater frequency of rotational winter crops that feature earlier flowering and grain development will help farmers adapt to the projected hotter, drier summers (Kaur et al., 2017).
Socioeconomic Drivers of iPNW Alternative Cropping Systems
A prevalent sociological factor that drives farmers' pursuits of improved soil health and conservation relates to their commitment to their farm legacies (Figure 1). This commitment is rooted in the multi-generational family farms prevalent in the iPNW, many extending back four generations to the earliest settlers of the early 1880s (McGregor, 1982). A survey of Washington “centennial farms” was conducted in the late 1980's in which there were 400 applicants and 210 approved 100 year family farms (Lang and Flynn, 1989). These farm families stay committed to maintaining healthy and sustainable farms that can be passed down to the next generation. Farm legacy and economically-driven cropping systems can still feature climate change adaption and mitigation as by-products, since climate change concerns rank low among farmers' priorities and recognized benefits of alternative systems (Seamon et al., 2016). Specifically, most iPNW farmers still see no need to make major changes to farming operations and crop rotations to adapt to climate change or contribute to climate mitigation (Seamon et al., 2016). Yet they are open to adopting cfBMPs such as integrating canola (Brassica napus; Pan et al., 2016b) and legumes (Vandemark et al., 2014) into their rotations for achieving shorter-term economic and agronomic benefits.
Incentives for adopting BMPs in general, can come from policy or market forces. These policies must be sufficiently substantial for farmers to invest time and resources to change practices (Ribaudo et al., 2011). High startup costs and lower initial yields associated with new systems often necessitate government support (Dillman et al., 1987). Yet, subsidy payments provided by conservation compliance programs may be insufficient to pay for high start-up costs of alternative management adoption such as direct seeding, which incurs expensive fixed and variable costs (Young D. L. et al., 1994). Additional cost savings and profitability of alternative crop rotations are now necessary for these systems to be fully implemented (Figure 1).
Win-win cropping systems should also be adjustable to socioeconomic market shifts. Farmers are already challenged to improve sustainability while increasing crop productivity per land base and supporting changing demands for diversified crop products such as food, feed, fuel and fiber products (Graham-Rowe, 2011; Tilman et al., 2011; Figure 1). Currently, the iPNW cereal production largely meets the bulk commodity, export cereal grain markets fostered by regional grain commissions; about 90% of the wheat produced is marketed to Asian countries (Washington Grain Commission, 2016). However, current market trends point toward the expanding demand for diversified, more-sustainably produced, crop-based food protein, healthy grains and oils (Greene et al., 2017).
Farmer networks also help drive adoption of alternative cropping systems. Emerging markets for alternative crop products challenge farmers to develop innovative networks (Klerkx et al., 2010; Reardon et al., 2016). To help farmers navigate these market opportunities, grassroots grower associations have developed certification recognizing sustainable practices, share knowledge amongst farmers and communicate eco-friendly farming practices to consumers. These iPNW organizations are attempting to redesign historical win-lose scenarios associated with conventional wheat farming (Table 1) and move toward win-win approaches. They are responding to environmental pressures and emerging food markets, simultaneously addressing societal concerns for food, soil, water and air quality. Some organizations are also using food labeling to capture market attitudes toward genetically modified crops, and organically and sustainably produced food. These attitudes will continue to shape market driven choices that in turn, shape the diversification of regional cropping systems.
These grower-based organizations push dual C pathways toward emerging grain markets while improving organic C cycling and soil C sequestration (Figure 2). For example, Shepherd's Grain is a farmer-based company that serves regional markets demanding high-quality grain by adhering to sustainable management practices with no-till seeding as a cornerstone. Its grain products are Food Alliance certified (Banks, 2016). The Pacific Northwest Direct Seed Association (PNDSA), a grass-roots, farmer-organization holds annual conferences focused on practical farming practices such as crop rotation, residue management and no-till systems that improve soil quality (Table 2; PNDSA, 2017). The PNDSA has also developed a certificate program, “Farmed Smart” that recognizes sustainable grain production practices and communicates to environmental groups that sustainability BMPs are practiced. Producing organically certified grain is difficult in the dryland iPNW given challenges associated with weed management and soil fertility (Borrelli et al., 2014; Tautges et al., 2016). Yet several farmers across the region (Lorent et al., 2016) have found means to overcome these barriers and are intent on enhancing soil health by storing more soil C (Figure 2) and reducing chemical inputs to meeting growing regional market demands for organic products.
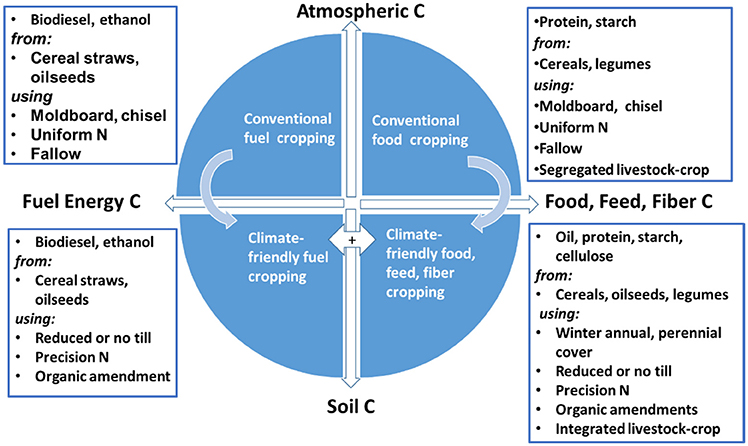
Figure 2. Dominant fates of photosynthetic carbon toward food, fuel, atmosphere and soil of conventional inland Pacific Northwestern US (iPNW) cropping systems (Top) that have focused on food production while emitting greenhouse gas (GHG), moving toward aspirational iPNW cropping systems (Bottom) with integrated cfBMPs that produce food, fuel, feed and fiber while building soil C and reducing system-wide GHG.
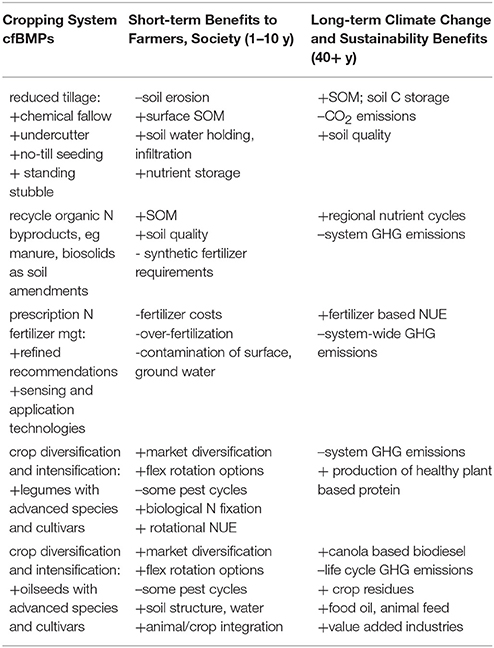
Table 2. Cropping system climate-friendly best management practices (cfBMPs) for integration into win-win scenarios that address farmers' short to medium-term goals, and long-term climate change and sustainability goals.
Policy Drivers of iPNW Alternative Cropping Systems
State and federal policies provide financial and technical support to help initiate farmer adoption of new systems (Figure 1). For example, the USDA Conservation Compliance Program provides guidance, financial support and tools to farmers who participate in the program to control erosion on high risk land. Currently, farmers are required to implement and document a variety of conservation practices in order to qualify for most government programs such as conservation payments and crop insurance.
Some farm support programs have not been as successful at driving conservation adoption. A decade ago, C trading was initiated so that farmers might earn soil C sequestration credits to help balance the economic shortcomings of cfBMP adoption (Branosky, 2006). The relative costs and benefits of GHG emissions caps and N fertilizer taxing were debated (Avi-Yonah and Uhlmann, 2010).
These programs have had mixed success, as some farmers do not consider the financial support sufficient to compensate their costs and efforts of adoption (Conner et al., 2016). Nevertheless, the evolution of win-win cropping systems will potentially lessen farmer reliance on government support for achieving climate change adaptation and mitigation if these outcomes become by-products and integral part of more profitable farming business plans. The full implementation of mitigation practices requires a system-wide accounting of the market and non-market costs and benefits of alternative agricultural practices, which should become the basis of policy and programs (Tilman et al., 2002).
The crop residue management practices promoted by the USDA-NRCS are also supported by farmer-governed state soil and water conservation districts that provide financial and technical knowledge support (Figure 1). Qualified farmers are currently provided incentives through NRCS's Conservation Stewardship Program (USDA-NRCS, nd2). Such activities include cover cropping, integration of big-rooted oilseed crops for improved water management, crop residue management, no-till practices, improved N management such as use of slow-release fertilizers, site-specific N management and riparian zone management. State programs also foster conservation programs that are climate change proactive. Local, state soil and water conservation districts apply for federal and state grant funding to assist farmers in conservation education, planning and implementation of activities designed to enhance soil, water and air quality, manage nutrients, control erosion and increase energy efficiency (Boie, 2016).
The need for win-win scenarios to drive integrated cfBMP adoption is well illustrated by current levels of adoption of precision agriculture practices. The NRCS conservation farming programs currently support farmer use of technologies touted to improve N fertilizer management. These incentives may initially entice progressive, early adopting farmers to try new technologies, such as slow-release N fertilizers and spatial soil test mapping for guiding prescriptive variable rate N applicators, but regional farmer surveys suggested that the incentive levels are often insufficient to sustain practices that might not otherwise demonstrate to be consistently economically profitable (Weddell et al., 2017). In contrast, farmers have rapidly adopted tractor mounted-systems that guide seeding and chemical applications. This technology demonstrates more visible costs savings when field operation overlaps are avoided, resulting in obvious economic and resource use benefits to farmers (Weddell et al., 2017).
Crop insurance is one of the most important farm policy tools that has a potential role to play in incentivizing adoption of cfBMPs by providing risk protection of existing or novel systems. Federal programs have been created to provide a safety net for producers. The Federal Cropping Insurance Program was created in 1938 and expansion in the 1990s. The 2014 Farm Bill shifted compensation away from direct payments to crop insurance-and whole farm revenue based safety nets as the primary mechanism for providing economic stability to the agricultural industry.
With volatile markets and climatic conditions that are projected to become even more extreme, crop insurance will continue to play a vital role in the economic viability and sustainability of agricultural production in the region. The federal crop insurance program has been administered by the USDA-Farm Service Agency (FSA) through a joint public-private partnership with the USDA-Risk Management Agency (RMA). In 2015, roughly 85 percent of major crop area in the US was covered by crop insurance representing a total liability of $102.4 billon (USDA-RMA, 2016). Crop insurance programs have offered yield and revenue based coverage options. From 2011 to 2015 the average annual indemnity payments from these multiple peril cropping insurance policies was $11.1 billion per year. The majority of these payouts were government subsidized with grower premium payments covering only 47% of the costs. Indemnity payments in Whitman County, Washington have been driven by market volatility and weather variability (e.g., heat, drought, flooding) with 82% of the claims focused on unexpected losses in wheat yield or revenue (Figure 3). In 2009 the largest payout was linked to a large decline in wheat prices, whereas excessive rain in 2010–2012, drought in 2014 and 2015, and extreme temperatures in 2016 resulted in large payments.
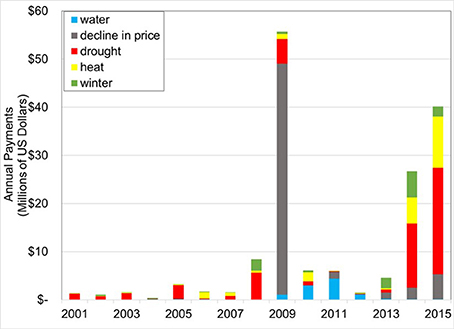
Figure 3. Total indemnity payments broken down by reason in Whitman County, Washington state from 2001 to 2015 (USDA-RMA, 2016).
Government subsidies will continue to grow unless growers are encouraged to adopt more resilient and established win-win systems. Insurance premiums are currently set using a loss-cost method based on average historic losses for a particular county (Woodard et al., 2011). This method has been criticized as it encourages high-risk farming on poor ground with no incentives to minimize the risk of crop failure through building and restoring degraded soils (Wu, 1999; O'Conner, 2013). Insurance programs have been proposed where farmers' premiums are reduced based on measures of soil quality and health (O'Conner, 2013; Woodard, 2016). Such programs provide incentives for farmers to implement practices that build SOM and support a shift in C pathways toward more organic C sequestration and nutrient cycling (Figure 2). Crop diversification and nutrient management strategies that reduce both market and crop loss risks have been further incentivized through reduction of insurance premiums. Management scenarios that may appear to be “break-even” on the short term, such as the adoption of no-tillage practices as described above, may become “win-win” if reduced insurance premiums make this practice more profitable.
Another way that state and federal policies can affect farming systems is through infrastructure support. As an example, the Washington State government supported infrastructure development to build markets for alternative crops (Lang, 2017). In 2006 Washington State created the Energy Freedom Program, which provided low-interest loans and grants to support the construction of oilseed crushers and other bioenergy-related facilities (O'Leary et al., 2013), thus creating a pull market for regionally produced oilseeds. Federal support for this program stemmed from the 2005 National Energy Policy Act (GPO Government Publishing Office, 2005) during a federal energy crisis, linked to turmoil and disruptions in international fuel supplies. State investments in biofuel crop production research and extension coordinated projects were then implemented (Sowers and Pan, 2012), in recognition that rapid and sustained adoption of canola in Australia and Canada occurred at the same time of increased financial investments in research and development (Maaz et al., in press). While these early investments were thought to be economically questionable on a biofuel basis (Young, 2009), today, Washington state has the processing infrastructure in place to supply increasing oilseed-based fuel, food and feed regional markets (Lang, 2017).
Diffusion of iPNW Cropping Systems Innovations
New iPNW agronomic systems have been initiated by innovators and early adopters, followed by early and late majorities and finally the latest adopters, referred to as laggards by the adoption theorists (Rogers, 1995). During historic integration of conservation farming and now cfBMPs for iPNW cereal-based systems, there has been a distinct and influential population of early adopters (Dillman et al., 1987; Figure 4). Early pioneers and adopters of new equipment were mechanically innovative and skilled (Carlson and Dillman, 1988). Farmer innovators and early adopters of no-till seeding drills and fertilizer technologies also developed family businesses around these enterprises (McGregor, 1982), providing added income and motivation. Crop development and seed production enterprises spawn from technological advances in seed development, evaluation, demonstration and sales by international or regional businesses that are sometimes farmer owned (Sowers, 2017). Early adopters of innovative cropping systems and practices have been documented in case studies produced by university extension programs (Mallory et al., 2001; Sowers et al., 2011, 2012; Lorent et al., 2016; Yorgey et al., 2017a). They exhibit natural curiosity, embrace the challenge of trying to do things differently in order to improve their farms and environment, with an overall commitment to “do things right.” They typically have worked closely with researchers, private and government agency crop advisers to implement systems and define how their innovations have changed their systems. Most have taken advantage of farm programs and financing that support conservation practices. The mature, well-vetted win-win scenarios that feature stable, economic profitability of integrated stable cfBMP-based systems, will provide the free-market economic driver necessary to enable late adopters to changeover and to sustain these practices across all adopters with minimal government support and intervention. Late adopters enter when the systems are sufficiently developed and understood; some of the possible risks have been addressed, either through better management strategies or government support (Figure 4).
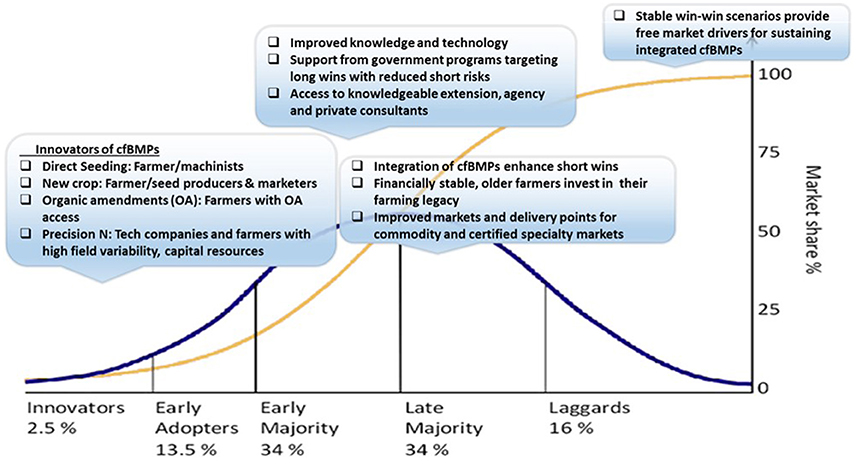
Figure 4. Theoretical adoption curve modified from Rogers (1995) of relative numbers of adopters at different stages (blue line) and cumulative adopters (yellow line), applied to the adopter characteristics of the four major climate friendly best management practices (cfBMPs). Earliest inland Pacific Northwestern US (iPNW) innovators of no-till, organic amendment and new crop use, and precision agriculture technology have been farmers, businessmen, researchers and experimentalists. Early adopters took advantage of early innovators' knowledge and technology and government policy support. Late majority adopters responded to wider availability of knowledge and technology of stacked integrated cfBMPs. Latest adopters, coined “Laggards” by Rogers, convert systems when they are well established and economic and agronomic benefits are clearly demonstrated.
Climate-Friendly, Best Management Practices
Agronomic cfBMPs are reviewed herein: crop residue management, organic recycling, N management, crop diversification and crop intensification (Table 2). Alternative systems that integrate these agronomic strategies across the different iPNW subregions (Figure 5) have promise for achieving climate change mitigation and/or adaptation with integrated win-win strategies (Figure 6).
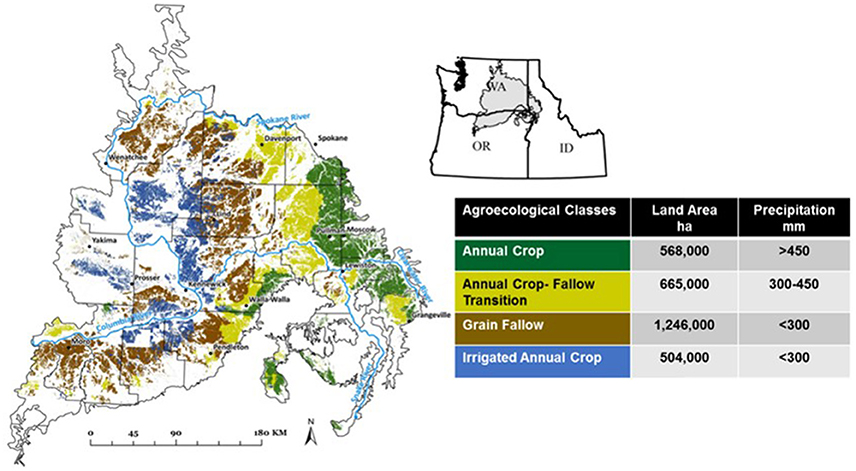
Figure 5. Major agroecological cropping zones, precipitation ranges and crop areas in the wheat-producing inland Pacific Northwestern (iPNW) U.S.
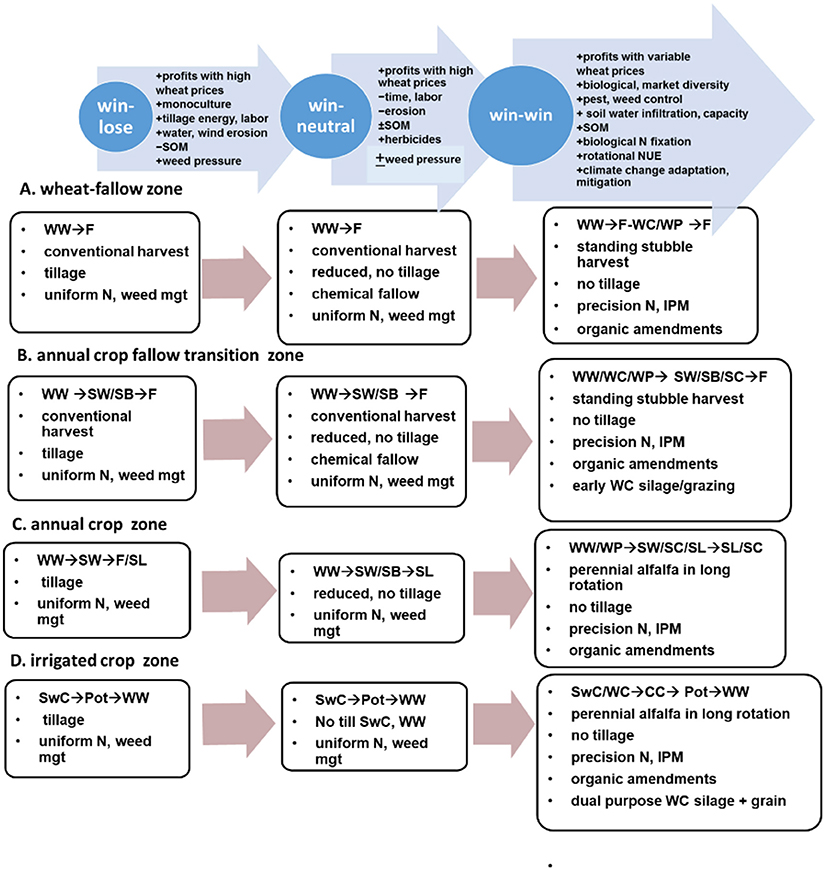
Figure 6. Traditional win-lose (left), transitional win-neutral (middle), and aspirational win-win (right) inland Pacific Northwestern US (iPNW) wheat cropping systems scenarios tailored for the production zones of (A) low precipitation, wheat fallow, (B) intermediate precipitation, annual crop fallow transition, (C) high precipitation, annual cropping, and (D) irrigated annual cropping. Old agronomic management principles combined with new crop options and technologies (climate friendly best management practices (cfBMPs, Table 2) have moved, and will continue to move systems from left to right, from traditional win-lose systems to transitional win-neutral systems to current and aspirational win-win scenarios for achieving short-term economic profitability and long-term solutions to environmental quality, market and climate shifts of the twenty-first century. WW, winter wheat; WC, winter canola; WP, winter pea; WT, winter triticale; SW, spring wheat; SB, spring barley; SC, spring canola; F, summer fallow; SwC, sweet corn; CC, cover crops; POT, potato.
Reduced Tillage and Crop Residue Management
Climate-friendly crop residue management includes replacing plowing with reduced or no-tillage planting and growing alternative crops with greater quantity and quality of crop residue production. These options protect soil against erosional forces, increase SOM and C sequestration, while improving water soil water storage and yield potential, achieving a better balance of C flow toward SOM and food production (Figure 2).
Severe topsoil losses have occurred in fallowed fields seeded to winter wheat, burned stubble fields and wheat-seeded fields following crops such as spring peas (Kaiser et al., 1954). In the iPNW, conservation tillage has reduced soil erosion and sediment loads (Kok et al., 2009; Brooks et al., 2010; Williams et al., 2014). Brown and Huggins (2012) reviewed 131 data sets from the iPNW and found that 75% of native ecosystems converted to agriculture lost at least 0.14–0.70 Mg C ha-1 year−1 and that the conversion from conventional tillage to no-till practices was predicted to increase SOC from 0.12 to 0.21 Mg C ha−1 year−1 for 75% of situations. By reducing erosion, protecting topsoil and maintaining SOM, conservation tillage practices also directly and indirectly affect soil health. In addition, nearly all measurable soil microorganisms, including bacteria, fungi and actinomycetes increase with no-tillage adoption (Stubbs et al., 2004). In addition, Johnson-Maynard et al. (2007) reported significantly higher earthworm densities in conservation tillage treatments as compared to conventional tillage in the annual cropping zone of the iPNW. Earthworms are important biological indicators of soil quality and can increase biodiversity and yields in managed systems (van Groenigen et al., 2014).
Maintaining surface crop residues before late summer planting of winter crops not only protects soil from eroding, but it also enhances snow capture and seedling establishment. No-till winter peas exhibited improved seedling vigor, reduced winter injury and increased grain yield compared to conventionally-tilled peas (Huggins and Pan, 1991). Biomass production is critical for maintaining seed-zone soil moisture in no-till fallow. Chen et al. (2006) demonstrated that tall stubble improved yields of winter lentil (Lens culinaris). Producers normally grow short, semi-dwarf varieties of winter wheat in this area, however, some farmers are starting to grow tricale (× Triticale Wittmack) which produces 50% more crop residue than semi-dwarf wheat in high residue farming systems (Port, 2016).
Brown and Huggins (2012) summarized long-term residue management experiments in iPNW and their conclusions showed a limited ability of reduced tillage and direct seeding of conventional crop rotations to maintain and increase SOM and soil C sequestration. Using these findings, Stöckle et al. (2012) projected that a 10% increase in no-till and reduced tillage in existing cropping systems could reduce GHG by >1,550,000 Mg CO2e/y over the iPNW due to soil C accumulation over a decade, but some site-specific systems would come to equilibrium thereafter. These assessments assume tillage reductions within existing cropping systems. Further research is needed to determine what extent direct seeding in combination with other cfBMPs such as diversified crop rotations with more prolific roots and/or increased stable organic C inputs can more fully replenish and build SOC within the limitations imposed by climatic conditions. Recent developments in monitoring technologies have enabled the ability to assess system C balances between crops and soils while tracking CO2 fluxes of managed fields to assess the effects of diversified crops, soils and fluctuating weather (Waldo et al., 2016; Chi et al., 2017).
Despite benefits of improving crop residue retention as soil C, there are immediate incentives for straw removal that include markets for mushroom and animal bedding, cellulose-based energy and paper-based packaging. Excess straw can cause difficulties in direct seed stand establishment, requiring field burning or straw harvesting. Site-specific straw removal is only recommended from areas of excessive straw production, and nutrient removal from the system needs to be replenished (Huggins et al., 2014).
Organic Resource Recycling
Recycling of available organic resources is considered a cfBMP for two reasons: (i) replacement of commercial fertilizers that have high GHG costs in production (Wood and Cowie, 2004; Brown et al., 2010) and (ii) their unique ability to sequester stable forms of soil C and supply nutrients (Bogner et al., 2007, Table 2). Early recognition of the important role of organic amendments (Spillman, 1906) has been supported by long term trials that consistently demonstrate that animal manure and human biosolids are more effective than crop residues for building SOM, sequestering soil C, improving soil structure, water infiltration and retention, increasing nutrient availability and enhancing microbial activity, while reducing soil bulk density (Yorgey et al., 2017b). These amendments offer a valuable strategy in the winter wheat-fallow region, where reducing or eliminating tillage has been insufficient for re-building soil C levels, due to low productivity and the resulting low levels of C inputs in residues (Machado, 2011; Gollany et al., 2013).
Organic amendments can promote large increases in total soil organic C (SOC) storage (Machado, 2011) with significant buildup of stable C forms (Pan et al., 2017b), reducing CO2 release that would occur if these waste products were otherwise incinerated or left to rot. Yet most of the region is spatially separated from animal and human population centers, so the utilization of many common amendments is presently limited by transportation costs into most of the dryland areas of the iPNW. Nevertheless, opportunities do exist for distribution of biosolids and manures on dryland areas with nearby urban centers and large animal operations.
Biosolids applications can build soil C while producing equivalent or better grain yields than typical applications of inorganic N in tilled and untilled wheat systems (Koenig et al., 2011; Barbarick et al., 2012). These increased yields are often attributed to the phosphorus, sulfur and micronutrients provided by the biosolids (Ippolito et al., 2007). Other possible factors include improved soil physical properties. While biosolids applications generally raise grain protein when applied during the fallow year (Cogger et al., 2013), practical experience suggests that this is generally not great enough to negatively impact prices for soft wheats that can have high protein penalties. Likewise, the risk of N and P losses after biosolids applications is most often relatively low in regional dryland cereal systems, especially for one-time applications (Ippolito et al., 2007; Barbarick et al., 2012; Cogger et al., 2013). These anaerobically digested biosolids were later shown to also build stable and more labile soil organic C and N, while supplying sufficient crop N to a wheat-fallow system (Pan et al., 2017b).
Access and costs of organic amendments is the biggest challenge to dryland farmers of the iPNW. Since biosolids are a by-product that must be managed by wastewater treatment facilities financed by sewage taxes, they are available at no cost or reduced cost to farmers. In some cases, municipalities charge transportation and application fees, or a fee equal to the N value of the biosolids (Sullivan et al., 2015). The question remains of whether a similar system is feasible for manure management of concentrated animal operations. Over-irrigation pushes nitrate through shallow root zones to groundwater, causing water quality problems in the aquifer (Brown et al., 2011). Manure related water quality problems are driving public opinion and litigation, as well as public policy. Central Washington irrigated systems support a large dairy industry that has limited land to upon which to recycle manure.
There is an opportunity to replicate the biosolids success story by transporting manure to dryland wheat farms. Improved on-farm separation and concentration of nutrient-rich solids should increase economically viable hauling distances (Yorgey et al., 2014; Frear et al., in press). In the iPNW, animal production is located in concentrated production facilities, spatially separated from the dryland wheat farms. In south-central Idaho, where dairy farms exist in combination with dryland fields on high plateaus, manure is used as a nutrient source in the production of organically certified wheat sold at prices that are 2–3 times those of commodity wheat and organic alfalfa hay (Lorent et al., 2016). Manures that have solids separated, aged, dried and sometimes composted primarily benefit overall soil health by increasing SOM, rather than serving as a primary source of crop available N. For example, compost improved cereal yields by successfully restoring organic matter on eroded Palouse hilltops, with yield improvements achieved after N immobilization was overcome with additional N fertilizer input (Cox et al., 2001). Likely mechanisms for the yield gains include improvements in nutrient and water holding capacity, soil structure and water infiltration.
Other materials that may recycle nutrients and C include biochar (a charcoal-like material that is generated when organic materials such as forestry wastes are heated in oxygen-limited environments) and black liquor (an organic by-product that results from paper-making). Application of 10 tons/acre or more of alkaline biochar (pH 10) derived from forest wastes had mild soil liming effects and improved wheat yields near Pendleton, Oregon (Machado and Pritchett, 2014). While these results are encouraging, separate analysis suggests that biochar is not economical if only the effects on pH are considered (Granatstein et al., 2009; Galinato et al., 2011). Crop residues from cereal and grass seed systems can be used for paper-making and the first new paper pulp mill to be built in the U.S., and the largest straw pulping mill in the world is now under construction (Erb, 2017). Straw fibers are typically alkali-pulped, producing black liquor, a lignin-rich soil amendment that can be returned to the land to increase soil C, biological activity and wet stable aggregates (Xiao et al., 2007a,b).
Cover crops are grasses or legumes that are used primarily to provide seasonal protection against soil erosion and an organic soil improvement (Unger et al., 2006) Compared to amendments, which need to be transported and spread, cover crops generate organic materials in place. However, water is a major limitation in the iPNW and existing research with single- and multi-species cover crops in eastern Washington and in semiarid eastern Colorado (Nielsen et al., 2015) has not found agronomic and economic benefits (Thompson and Carter, 2014; Roberts et al., 2016).
Nitrogen Management
The improvement of N use efficiency (NUE) of cropping systems is a critical cfBMP of win-win scenarios, since N is an expensive farm input and it greatly affects GHG emissions (Snyder et al., 2009). Wheat farming began in the iPNW in the 1870s. Native soil N declined by 22% during the first generation of farmers and replenishing it was a recognized prerequisite for increasing soil productivity (Fulmer and Heileman, 1899). This situation was greatly helped by the mid-twentieth century development of synthetic N fertilizers and N soil testing. Recent estimates of regional N balances suggest crop N removal is being replenished with N biological and fertilizer inputs, although P and K are still being depleted (Table 3). The International Plant Nutrition Institute (IPNI) recognizes that the region as a whole has achieved N balance with regard to N inputs offsetting N removal by grain harvest, after conducting an extensive data analysis of nutrient balances across each county of the U.S. (IPNI, 2012). Yet, documentation of inefficiencies and N losses in the region suggest that while there may be an overall balance of regional N inputs vs. grain N harvested, there are areas of over- and under-application of N fertilizers within fields, resulting in N leaching below the root zone, N runoff to local waterways (Keller et al., 2007) and N volatilization to the atmosphere (Venterea et al., 2012). Excess N is susceptible to nitrous oxide (N2O) production (Snyder et al., 2009) and the release of this GHG can offset carbon sequestration benefits provided by conversion to reduced- or no-till (Stöckle et al., 2012).
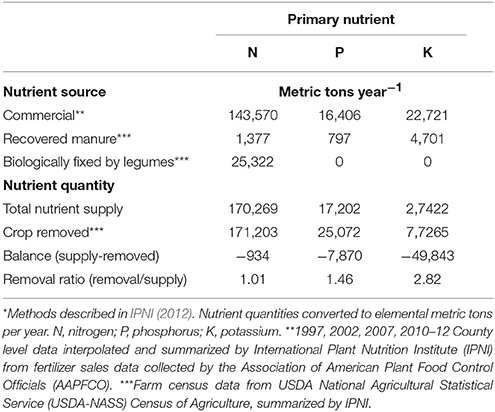
Table 3. Nutrient supply and removal in harvested grain* across the main wheat-producing counties of the inland Pacific Northwestern US (adapted from Borrelli et al., 2017).
Efforts to improve fertilizer N use efficiency in the iPNW (Huggins and Pan, 1993) paralleled the conservation farming movement of the late twentieth century. Several levels of technology adoption hold promise for improving regional NUE and thereby reduce system wide GHG emissions. The baseline opportunity is to increase adoption of soil N test-based N fertilizer recommendations that have existed since the 1950's (Pan et al., 2007). Accurate estimation of N fertilizer rate requirements is based on available water and soil N supplies (Pan et al., 2007, 2016a) with full root zone (0–180 cm) soil testing, but there are opportunities for increasing adoption of routine soil testing by regional farmers since a recent survey revealed that only two-thirds of regional farmers regularly take soil samples (Mahler et al., 2015). Recognition that a majority of crop N uptake comes from non-fertilizer sources (Sowers et al., 1995; Pan et al., 2016a) should provide farmers with ample motivation to take regular soil tests and collect other crop and soil information for optimizing their fertilizer use.
As alternative crops are adapted to the region, crop specific N requirements and recommendations need to be developed and evaluated for specific agroecological subregions. The basic 4R (four “rights”) approach focuses on right fertilizer rate, placement, source and timing, which require adjustments for fertilizer management of alternative crops like canola (Norton, 2013). Canola differs in crop physiology from wheat, which dictates changes in N placement, timing and source (Pan et al., 2017a).
Fertilizer recommendations will need to be integrated with predictions of water supply in a changing climate (Pan et al., 2016a). Overall, adopting farmers need to understand that typical wheat N management principles do not necessarily apply to newly introduced crops.
The potential of site-specific N management for improving within-field NUE was recognized for more than 20 years (Fiez et al., 1994; Pan et al., 1997), but it was also recognized that the N recommendations based on regionally developed algorithms would be insufficient to make landscape level N recommendations since unit N requirements (kg total N supply per kg grain) and NUEs varied across the landscape (Fiez et al., 1994; Hergert et al., 1997). Several biophysical stressors such as limited water availability and compacted soil layers negatively impact NUE across the variable landscapes (Pan and Hopkins, 1991; Fiez et al., 1994; Ibrahim and Huggins, 2011), locations and annual precipitation (Maaz et al., 2016).
Recent technological advances have provided opportunities to more accurately assess landscape specific soil N availability and root stressors that will lead toward better landscape-specific N management within farm fields. These technologies include remote sensor systems, robotics, GIS spatial mapping for prescriptive soil management and new technologies for yield mapping and protein monitoring (Weddell et al., 2017).
As decision support systems become available to help farmers utilize large datasets, there will be potential improvements in the use efficiencies of fertilizers and pesticides, along with improved grain yield and quality that may provide economic advantages of technology adoption without government support. The economic driver of efficient N management is illustrated during times of high N fertilizer prices, when farmers tend to reduce their N fertilizer use and improve their cropping system NUE (Nehring, 2016). Another example is evident in iPNW high protein wheat production, where the ratio of fertilizer price to grain protein price premiums for hard red wheats influence the economically optimal N recommendation and use (Baker et al., 2004). Higher ratios result in lower N input recommendations and improve N use efficiency. A complex of biophysical drivers and crop responses have led to the identification of landscape performance classes for gauging site-specific NUE parameters that link to site-tailored wheat N recommendations (Weddell et al., 2017).
Regional NUE can be improved with the integration of old conservation principles with new fertilizer management technologies, substitution of commercial fertilizer with legume expansion and organic byproduct recycling and adoption of site specific 4R N management utilizing advanced precision technologies. Greenhouse gas production occurs at the fertilizer plant (Wood and Cowie, 2004) and with field applications of N fertilizer (Shcherbak et al., 2014). A 10% overall improvement in regional fertilizer NUE based on annual N fertilizer use (Table 3) would reduce GHG emissions associated with the reduced synthetic N fertilizer use of 13,000 Mg fertilizer N/year.
Crop Diversification
Alternative crop rotation design is another major cfBMP category that has great potential for achieving win-win scenarios (Table 2). Crop diversification changes immediately require adjustments in the other rotation-wide management practices to optimize the cropping system's economic and environmental impacts. Examples of cfBMP integration into alternative cropping systems are described for each iPNW agroecological zone below.
Diversification of cereal systems with broadleaf grains (oilseeds and legumes) or cover crops is useful to optimize pest management, nutrient cycling, N use and water use efficiencies, soil building and potential GHG mitigation (Table 2). Advantages of these “break crops” in rotation with wheat has been shown to result in significant increases in wheat yields worldwide (Kirkegaard et al., 2008). Crop diversification also diversifies marketing options, reducing price risk for farming enterprises. Regional crop diversification opportunities are large, given that the iPNW largely produces to meet the bulk commodity, export cereal grain markets. The iPNW region supports the production of both spring and winter cereals and there are opportunities for integrating both spring and winter oilseeds and pulse crops in each agroecological zone. New crop options need to be integrated into sustainable cropping systems with a new set of cfBMPs (Table 2).
Crop rotation impact on GHG emissions is complex. Some affected variables include amount and source of N inputs and N2O losses, net soil C sequestration, net energy balance of external fossil fuel inputs and biofuel outputs. Diversification with legumes and oilseeds shifts the rotational N cycling, residual N carryover and on-farm production of N through legume-facilitated biological N fixation, all of which will reduce reliance on fertilizer N for subsequent cereal crops (Maaz and Pan, 2017). Important agronomic metrics impacted by alternative crop rotation include rotational N use efficiency, soil C storage, biofuel displacement of fossil fuel use and non-food products that can be credited toward C sequestration.
Weed management is a primary driver of farmer adoption of new crops. While it has no direct impact on climate change mitigation, it has indirect impacts as alternative crop rotations are established and sustained. For example, the persistence of winter annual grassy weeds severely diminishes wheat yield and quality in the iPNW. Diversifying winter wheat sequences with spring crops or fall-seeded broadleaf crops allows in-crop use of grass herbicides to reduce grassy weed populations and modifies the composition of weed populations (Burke et al., 2017). Well-established canola stands are competitive with weeds, providing a major driver in canola adoption (Long et al., 2016). Glyphosate-resistant spring canola provides opportunities for improved control of downy brome (Bromus tectorum), jointed goatgrass (Aegilops cylindrical), feral rye (Secale cereale), and Italian ryegrass (Lolium multiflorum) in annual crop systems (Young et al., 2016) and can reduce Italian ryegrass and broadleaf weed populations when used in place of spring non-glyphosate resistant legumes (Huggins and Painter, 2011). Winter wheat-fallow studies showed that diversifying with winter canola, along with split applications of quizalofop and glyphosate, controlled 90% of feral rye, eliminated seed production of feral rye and increased canola yield more than 40%. Use of glyphosate-resistant winter canola in tandem with glyphosate application can also help control feral rye (Young et al., 2016). Conventional canola cultivars lacking herbicide resistance are highly sensitive to sulfonylurea and imadazolinone herbicides, but new herbicide resistant cultivars are now available for overcoming herbicide carryover.
Additionally, herbicide-tolerant or resistant varieties would allow farmers to plant in fields with a history of imidazolinone and sulfonylurea herbicides and an increased selection of grass herbicides would be available in conventional canola (Young et al., 2016). Because glyphosate is the most important grass herbicide in summer fallow, the various canola scenarios would allow farmers to use other groups of herbicides to control grass weeds, thereby reducing the use of glyphosate in canola and mitigating the development of resistance of grass weeds to glyphosate. It is just as important to rotate herbicides as it is to rotate crops to reduce overall loading rates of any one herbicide. In addition, reduction in herbicide application rates are now enabled by the advent of imaging-based precision herbicide technologies capable of targeting post-emergence weeds in fallow (Riar et al., 2011).
Rotational diversification can also support win-win scenarios by suppressing soilborne fungal pathogens and nematodes that cause wheat and barley (Hordeum vulgare) diseases. In general, the population density of a pathogen increases with the increasing frequency of a host crop in a rotation. Using a rotation to suppress disease is most effective when alternate, non-host crops are available, precipitation is not limiting and conditions promote rapid residue decomposition. For example, planting a non-host broadleaf crop in place of a cereal crop can reduce some pest populations such as Hessian fly, orange wheat blossom midge, mites, Cephalosporium stripe and cereal cyst nematode, while not reducing Rhizoctonia, Pythium, and root-lesion nematode species (Eigenbrode et al., 2017; Kirby et al., 2017a,b).
Integrating legume pulse crops diversifies market opportunities for cereal farmers (Vandemark et al., 2014) but requires market infrastructure. As an example, advancement of chickpea (Cicer arietinum) storage, transportation and marketing in the region has greatly expanded iPNW chickpea production, driven by high prices (Maaz et al., in press). Similarly, the varietal development of edible winter dry peas promises to expand legume acreage into the drier agroecological zones, due to their greater yield potential and ability to survive harsh winters (Schillinger, 2017). Fall-sown dry peas and lentils are well-adapted for direct seeding into standing stubble and increasing demand for cover crop pea seed provides production incentive.
The need for oilseeds in iPNW wheat rotations was first published by Spillman (1906) for renovation of nutrient-mined, eroded soils. Recurring interest in oilseeds for a potential feedstock for biodiesel and jet fuel (Long et al., 2016) and a break crop in wheat rotations has been investigated for the last 50 years (Pan et al., 2016b). Efforts to push the regional adoption of canola based on agronomic benefits were unsuccessful until global markets for food oil improved, federal policies incentivized big rooted rotational crops and state government support for oilseed processing facilities began to pull grain through the supply chain (Lang, 2017).
Oilseed-based biodiesel has been assessed by U.S. EPA LCA to reduce system-wide GHG emissions by 50% when displacing conventional diesel (EPA, 2010) and perhaps higher GHG reductions occur in semiarid systems (Biswas et al., 2011; Kruger et al., 2015). Several factors help to moderate the negative impact on food production when inserting these oilseeds into rotation. Canola produces food oil as well as biofuels and a high protein animal meal by-product that contributes to the food chain (Long et al., 2016). Canola also has early maturing characteristics, its varieties offer unique options for weed control, thereby increasing subsequent wheat yield potential (Sowers et al., 2012; Pan et al., 2016b).
Recurring interests in regional energy production and crop diversification have focused efforts on canola food, feed and fuel production since the 1970s. Recently, over the past decade, there has been a four-fold increase in iPNW canola production due to a convergence of new regional processing facilities, improved global demand and competitive prices for canola, improved diversity of varieties and renewed extension and agronomic research efforts (Pan et al., 2016b). Winter and spring canola are being integrated into the iPNW cropping zones (Figures 5, 6). In addition, interest in developing cellulosic biofuels (Huggins et al., 2014) and paper products (Xiao et al., 2007b; Erb, 2017) can stimulate movement toward higher residue producing crops that could serve conservation compliance and/or emerging markets.
Alfalfa (Medicago sativa) is a viable crop diversification and intensification option for integration with annual crops (Koenig et al., 2009). USDA cropland data layers showed that alfalfa comprised about 5% of the crop acreage in both the annual crop and annual crop-fallow transition zones from 2007 to 2014 (Kirby et al., 2017a). Its potential for addressing climate change is attributed to its capacity to build soil and fix N. Organic alfalfa can be produced economically, stands can be maintained 5-10 years before transitioning back into annual crops, depending on fluctuating markets. This crop provides a transitional land management option prior to organic grain production (Fuerst et al., 2009). Production of organic crops is increasing in the iPNW, primarily in the irrigated zone (Kirby and Granatstein, 2017). Future expansion of organic alfalfa is most likely to occur as a rotational crop in irrigated and high rainfall zones, but markets will depend on the growth of the organic dairy sector in the region.
Crop Intensification
Intensification of crop rotation is another strategy for potentially developing win-win scenarios. It is defined as the increase in rotational land coverage with growing crops. Intensification can be achieved with (i) annual fallow replacement with a crop, (ii) increasing over-winter cropping with cover crops, (iii) substituting winter crops for spring crops, or (iv) replacing annual crops with perennial crops. Crop intensification can increase food production, utilize rising atmospheric CO2 for photosynthesis and sequester more soil C. The major challenge is the need to ensure that crop intensification is coupled with ecologically based management strategies such as conservation farming (Matson et al., 1997), as well as ensure short-term economic viability.
With sufficient available water, annual cropping maximizes biomass production and limits fallow periods between crops when soils are most vulnerable to erosion. Opportunities for intensification vary across the dryland iPNW with temperature and precipitation gradients, terrain and soil characteristics. Research in the annual crop-fallow transition and winter wheat-fallow regions has focused on strategies such as flexible fallow replacement with annual cropping in wetter than average years, or replacing spring crops with fall-sown crops. While these strategies will intensify production, increase C fixation and seasonal soil surface coverage, they can also have negative impacts on farm economics and risk if practiced in drier than average seasons (Young et al., 2015). Profitability of intensive cropping was found to be more variable than for wheat-fallow in the Great Plains, U.S. (DeVuyst and Halvorson, 2004).
Replacing spring crop sequences with fall-sown winter hardy crops intensifies rotations by providing overwinter soil cover with increased yield potential (Schillinger, 2017; Stöckle et al., 2017). Fall-sown crops mature earlier than spring-sown crops, thereby avoiding heat stressors and water deficits that may occur later in the growing season. This advantage of winter over spring cropping may become more critical for future systems to be better adapted to predicted warmer, drier summers (Kaur et al., 2017). For example, replacing spring legumes with a fall-sown pea or lentil provides greater crop biomass, residue and biological N fixation; fall-sown peas can have double the seed yield compared to spring-planted cultivars (McGee, 2016).
Integrated Win-Win Systems by Cropping Zone
The iPNW agroecological zones are largely defined by temperature and moisture gradients. Classification of USDA cropland data layers allows the identification and area estimates of four major iPNW cereal cropping zones: annual crop, annual crop fallow transition, grain fallow and irrigated (Figure 5). The area of these zones are projected to shift with regional climate change (Karimi et al., 2017).
Effective integration of BMPs is production-zone dependent (Zentner et al., 2002). In terms of gauging short term economic “wins,” farmers and economists have historically conducted single crop net return comparisons of substituting alternative crops for traditional crops. In recognition of the potential rotational benefits of alternative crops and management systems, rotational enterprise budgeting tools are being developed to for specific production zones to help farmers understand a more complete economic impacts of system redesigns within production zones (Connolly et al., 2015, 2016).
Crop–Fallow Zone
The crop-fallow zone has insufficient annual precipitation (<300 mm) to economically support annual cereal cropping (Young et al., 2015). This zone occupies the greatest regional area, which is projected to reduce in size with climate change scenarios (Karimi et al., 2017). Tilled summer fallow is practiced in this zone for storing soil water to produce winter wheat every other year despite its erosive impacts (Lindstrom et al., 1974). While minimum tillage or chemical fallow, direct seeded wheat-fallow have reduced wind erosion in win-neutral scenarios (Figure 6), it has done little to build SOM and restore soil health (Gollany et al., 2013; Ghimire et al., 2017). Reduced-tillage of summer fallow using an undercutter cuts weeds without inverting the soil, maintains surface residue cover for reduced wind erodibility and has similar economic costs to summer fallow (Young and Schillinger, 2012). However, with no increases in subsequent wheat yields, only a neutral-win scenario was achievable by protecting soil, but not improving profitability.
Integration of tillage management with different crop rotations have been and will continue to be evaluated. Continuous no-till spring cereal cropping produced more residue which reduced wind erosion on susceptible soils by 95% compared to the traditional winter wheat-fallow system (Thorne et al., 2003). Yet, it also exhibited poor N balances (Pan et al., 2001) and it was found to be economically less viable than conventional wheat-fallow (Young et al., 2015), thus judged to be an overall lose-neutral scenario. These findings have led to evaluations of diversified crop rotations with winter canola and winter peas (Young et al., 2014; Schillinger, 2017) produced in concert with minimum or no-tillage (Figure 6).
Recent introduction of winter canola into this region has met with mixed success, requiring more research to determine residue production and management requirements for more stable plant establishment and winter survival (Young et al., 2014). Tall standing stubble has been shown to increase water use efficiency and grain yield compared to shorter stubble in the Canadian prairie (Cutforth and McConkey, 1997; Cutforth et al., 2011), but seed zone moisture during summer fallow was not examined.
Production of high residue crops such as tall wheats and triticale, in tandem with a stripper header type combine, has resulted in tall standing stubble that can trap more snow and keep surface soils moist and cool during late summer establishment for improved crop establishment of no-till winter canola (Port, 2016). Insertion of alternative crops can have positive or negative effects on subsequent wheat production and rotational enterprise budgets (Connolly et al., 2015). Better weed control, enabled by insertion of herbicide resistant canola into wheat monoculture, has resulted in positive improvements in pervasive weed control, wheat yield and quality (Young et al., 2016).
This is the dryland zone closest to the irrigated central basins of Washington and Oregon (Figure 5) that produces large quantities of manure from concentrated dairy farms. In addition, this zone is closest to dense urban populations in western Washington and Oregon. The close proximity offers opportunities for processed manure and biosolids to be imported into this fallow zone, which will reduce fertilizer nutrient requirements. This would also build up the low SOM levels as has been demonstrated in a long-term manure trials at Pendleton, OR (Machado, 2011) and biosolids trials near Okanogan, WA (Cogger et al., 2013; Pan et al., 2017b).
Annual Crop-Fallow Transition Zone
The annual crop-fallow region (Figure 5) has traditionally supported winter wheat, spring wheat and spring barley in >50% of the zone, fallow in 27% and spring legumes in 10% of the zone with more fallow during drier years (Pan et al., 2016b). In wetter than normal years, continuous crop rotations have been grown. There is potential for crop diversification and intensification with winter and spring oilseeds and peas in this zone. This “flex cropping” in this zone allows farmers to intensify cropping in years with favorable field conditions and markets. Plant-available soil water is the most reliable indicator of potential yield. Farmers may take advantage of ample overwinter precipitation storage in the soil to plant a spring crop, replacing a traditional fallow sequence. Flexible decisions on direct seeding spring broadleaf crops in this zone, to conserve moisture and reduce erosion, should be based on over-winter soil water storage to 120 cm rooting depth and weather predictions for in-season precipitation (Pan et al., 2016a).
More specifically, Lutcher et al. (2009) stated critical levels of over-winter soil water storage required to trigger spring crop plant-back. This intensification strategy in a win-win scenario is further enabled by integrating direct seeding and canola diversification. Such a strategy has demonstrated improved weed control and economically viability while diversifying market opportunities enabled by a nearby canola processing facility in this production zone (Esser and Jones, 2013). Future win-win scenarios for this zone (may include direct seeded winter wheat, canola or peas, followed by direct-seeded spring crops or early planted biennial forage-grain winter canola, Figure 6). Fall-sown direct-seeded facultative spring wheat is another flexible option to normal spring wheat recrop planting. It is more competitive with annual weeds, it is better for erosion control with earlier established ground cover and it can yield better than spring planted cereals in a recrop scenario by enabling earlier plant development for avoiding summer heat stress (Bewick et al., 2008; Sullivan et al., 2013).
Annual Crop Zone
The high precipitation annual crop zone (Figure 5) supports annual cropping, with winter wheat, spring wheat and spring barley grown over 60% of the cropping zone land area and cool season legumes (lentils and dry peas) account for only ~18% (Pan et al., 2016b). This zone is projected to increase with climate change scenarios (Karimi et al., 2017). Wheat-fallow in this region proved to be vulnerable to very high soil erosion rates, so continuous annual cropping of winter wheat, spring cereals and spring legumes was adopted, particularly with the advent of commercial fertilizers (Kaiser et al., 1954). Nevertheless, soil erosion rates were still very high when direct-seeding planters were first introduced into the existing crop rotations of the region (Papendick et al., 1985). A long-term study in this region, south of Moscow, Idaho, has shown that direct-seeded standard winter wheat-spring pea rotation has resulted in comparable yields while reducing erosion potential, compared to the same rotation that was conventionally tilled (Guy and Lauver, 2007).
The IPM project of the 1990s (introduced earlier) more fully integrated an economically viable crop rotation, weed management and residue management while maintaining surface residues for conservation compliance and increasing soil moisture (Young et al., 1994a,b,c). Nevertheless, it has since been determined that direct-seeded winter wheat-spring cereal-spring legume eventually builds up pervasive annual grassy weeds, including Italian ryegrass (Young et al., 2016). Spring canola substitution for spring wheat and more profitable chickpeas in place of peas are options currently being implemented. Typically, there is insufficient soil moisture and growing degree days following crop harvest to establish and grow winter canola in this zone, so the focus has been on adapting spring canola (Pan et al., 2016b). In replacing spring wheat with spring canola, a direct-seeded crop sequence of spring canola-spring pea-winter wheat provides two sequential broadleaf crops that enable better control of pervasive grassy weeds such as Italian rye, that otherwise are difficult to control with a winter wheat-spring cereal sequence. Future direct-seeded cropping systems should have flexibility to have two spring broadleaf crop options (oilseed and legume) in rotation with winter wheat to achieve biological and market diversification for improved rotational economic returns, while sequestering C and controlling grassy weeds and soil erosion. Crop intensification by integration of alfalfa and animal/crop systems also provide potential win-win scenarios in this zone, depending on relative competitiveness of fluctuating animal vs. crop grain markets (Figure 6).
Irrigated Crop Zone
Located in the temperate desert of central Washington and Oregon (Figure 5), the irrigated crop zone supports annual row crop rotations that include potatoes and sweet corn that are considered susceptible to nitrate leaching. Previous studies in the Columbia Basin have shown that cover cropping can reduce leaching and recycle N in shallow-rooted potato crop sequences (Weinert et al., 2002; Collins et al., 2007). More recent research showed that integrated reduced tillage-cover cropping could increase the NUE and N export efficiency (NEE), while increasing season surface coverage, reducing wind erosion potential of a potato-wheat and corn-potato cropping sequence (Madsen, 2017). The NUE of the potato-wheat cropping sequence increased significantly due to reduced tillage. In addition, the potato NEE increased significantly due to cover crop and reduced tillage. Additionally, cover crops reduced the amount of mineral N between 60 and 120 cm. The increase in NUE and NEE, without a decrease in yield demonstrates that integrated cover cropping and reduced tillage can be the basis of win-win irrigated cropping systems (Madsen, 2017). Soil N testing and predicted rapid N mineralization from cover crops in these agroecosystems will provide estimates of total soil N supply that can lead to reduced N fertilizer recommendations (Weinert et al., 2002). Cropping system modeling suggests that reducing tillage while controlling N fertilizer inputs in these irrigated systems has promise for reducing net GHG emissions (Stöckle et al., 2012).
Finally, the proximity of crop acreage in the irrigated and crop-fallow zones to concentrated livestock farming and western Washington and Oregon urban populations opens new opportunities for livestock grazing on crop residues (Yorgey et al., 2017c) and judicious use of manure and biosolids during cropping sequences. In return, these production systems provide food and fuel in completing the cycle with nutrient sources.
Summary and Conclusion
Farm management practices have been adopted if they directly benefited farmers in improving their profitability and farm legacy while diversifying their markets. Historically these practices have often been adopted at the expense of soil, air and water quality. Wheat farmers and farmer networks in the iPNW over the past 40 years have recognized the need to minimize negative impacts on their surrounding air and water quality, while sustaining and building soil health. Now, they are also being challenged to meet climate change mitigation and adaptation goals. If conservation and climate friendly practices do not lead to short term gains in farm profitability, then government incentives have been required to foster adoption, and even these incentives have fallen short of implementing universal changes. Integrated coordination of agronomic research and extension with emerging market opportunities and technological advances, farmer attitudes, and public policy is required to drive cropping system changes (Smit and Skinner, 2002; Robertson and Swinton, 2005) that will meet farmers' priorities as well as address climate change.
Adoption and integration of new crop rotations with alternative management strategies for crop residues, organic recycling and N fertilizers into flexible farming systems promise to produce synergistic impacts on the overall farm economics and agroecological goals. Successful implementation will require the extension and implementation of old conservation principles with new technology. Many of the historically recognized conservation practices are now also recognized to improve soil health and system-wide climate change adaptation and mitigation.
Moving forward, win-win scenarios are being tailored for subregional crop production zones principally defined by available water in the iPNW. Coordinated public and private efforts need to be directed at refining, enabling and integrating best management practices into win-win scenarios to support farmers in their roles as producers, land and environmental stewards and climate change warriors.
Author Contributions
WP: Lead author responsible for writing team coordination, major text outline and writing, figure and table construction. WS: Dryland cropping systems contributions, including fallow management and winter pea production. FY: Dryland cropping and weed management, canola establishment sections. GY: Organic amendment first draft. EK: Crop rotation first draft. KB: Fertility section, overall editing. EB: policy related crop insurance summary. VM: Socioeconomic, policy section concepts, writing and editing. TM: Fertilizer management, NUE concepts, policy for crop adaptation. SM: Soil organic matter long term trends. JLJ-M: Earthworm, soil biota. IM: Irrigated crop zone cfBMPs. LP: Coordinator of overall cropping systems project and data collection. KP: Socioeconomic section writer, editor. DH: Residue management, precision farming concepts, regional SOM summary. AE: Contributor of cropping systems comparisons in transition zone. HC: Contributor of irrigated systems findings and concepts. CS: Contributor of modeling outcomes and references. SE: Overall REACCH project leader and manuscript editor.
Funding
The authors thank the following sources of support: USDA-NIFA Award #2011-68002-30191 from the USDA National Institute of Food and Agriculture. National Science Foundation Award #0903714. Washington Oilseed Cropping Systems. USDA National Institute of Food and Agriculture, Hatch project 1014527
Conflict of Interest Statement
The authors declare that the research was conducted in the absence of any commercial or financial relationships that could be construed as a potential conflict of interest.
Footnotes
1. ^STEEP (Solutions to Environmental and Economic Problems) nd. Advancing sustainable agriculture in the Pacific Northwest. Conservation Tillage Systems Information Resource. Pullman, WA: Washington State University Extension Service. Available at: http://pnwsteep.wsu.edu/tillagehandbook/index.htm (Accessed on April 10, 2017).
2. ^USDA-NRCS. (nd). Conservation stewardship program. Available at: https://www.nrcs.usda.gov/wps/portal/nrcs/main/national/programs/financial/csp/ (Accessed on March 20, 2017).
References
Abatzoglou, J. T., Rupp, D. E., and Mote, P. W. (2014). Seasonal climate variability and change in the Pacific Northwest of the United States. J. Climate 33, 121–131. doi: 10.1175/JCLI-D-13-00218.1
Albrecht, W. A. (1938). “Loss of soil organic matter and its restoration,” in Soils and Men, USDA Yearbook of Agriculture, House Document No. 38, eds H. G. Knight, C. E. Kellog, M. A. McCall, A. L. Patrick, C. R. Enlow, E. N. Munns, C. P. Barnes, B. W. Allen, O. E. Barker, E. N. Bressnan, and G. Hambidge (Washington, DC: United States Government Printing Office), 347–360.
Avi-Yonah, R. S., and Uhlmann, D. M. (2010). Combating global climate change: why a carbon tax is a better response to global warming than cap and trade. Stanford J 28, 3–50.
Baker, D. A., Young, D. L., Huggins, D. R., and Pan, W. L. (2004). Economically optimal nitrogen fertilizer for yield and protein in hard red spring wheat. Agron. J. 96, 116–123. doi: 10.2134/agronj2004.0116
Banks, R. (2016). Shepherd's Grain: Bridges Built, Alliances Forged. myFarmlife.com, Summer 2016. Available online at: https://www.myfarmlife.com/features/shepherds-grain-bridges-built-alliances-forged/ (Accessed on March 01, 2017)
Barbarick, K. A., Ippolito, J. A., McDaniel, J., Hansen, N. C., and Peterson, G. A. (2012). Biosolids application to no-till dryland agroecosystems. Agric. Ecosyst. Environ. 150, 72–81. doi: 10.1016/j.agee.2012.01.012
Batie, S. S., Gilliam, J. W., Groffman, P. M., Hallberg, G. R., Hamilton, N. D., Larson, W. E., et al. (1993). Soil and Water Quality: An Agenda for Agriculture. National Academies Press. Available online at: http://www.nap.edu/catalog/2132.html (Accessed on December 05, 2016)
Bewick, L. S., Young, F. L., Alldredge, J. R., and Young, D. L. (2008). Agronomics and economics of no-till facultative wheat in the Pacific Northwest, USA. Crop Prot. 27, 932–942. doi: 10.1016/j.cropro.2007.11.013
Biswas, W. K., Barton, L., and Carter, D. (2011). Biodiesel production in a semiarid environment: a life cycle assessment approach. Environ. Sci. Technol. 45, 3069–3074. doi: 10.1021/es1031807
Bogner, J., Ahmed, M. A., Diaz, C., Faaij, A., Gao, Q., Hashimoto, S., et al. (2007). “Waste management,” in Climate Change 2007: Mitigation. Contribution of Working Group III to the Fourth Assessment Report of the Intergovernmental Panel on Climate Change, eds B. Metz, O. R. Davidson, P. R. Bosch, R. Dave, L. A. Meyer (Cambridge: Cambridge University Press), 585–618.
Boie, J. (2016). FY2017 Annual Work Plan. Palouse Conservation District. Available online at: http://scc.wa.gov/wp-content/uploads/2016/06/Palouse-Conservation-District-Annual-Plan-FY2017_053116_submitted.pdf. (Accessed on March 30, 2017)
Borrelli, K., Koenig, R., Burke, I., Gallagher, R. S., Pittmann, D., Snyder, A., et al. (2014). Transition cropping system impacts on organic wheat yield and quality. Renew Agr. Food Syst. 30, 461–472. doi: 10.1017/S1742170514000283
Borrelli, K., Maaz, T., Pan, W. L., Carter, P., and Tao, H. (2017). “Soil fertility management,” in Advances in Dryland Farming in the Inland Pacific Northwest, eds G. Yorgey and C. Kruger (Pullman, WA: Washington State Extension Publication EM108–05), 237–282.
Branosky, E. (2006). Agriculture and Climate Change: The Policy Context. WRI Policy Note No. 1. World Resource Institute. Available online at: http://www.wri.org/sites/default/files/pdf/policynote_agriculture_climate.pdf (Accessed on April 02, 2017)
Brooks, E. S., Boll, J., Snyder, A. J., Ostrowski, K. M., Kane, S. L., Wulfhorst, J. D., et al. (2010). Long-term sediment loading trends in the Paradise Creek watershed. J. Soil Water Cons. 65, 331–341. doi: 10.2489/jswc.65.6.331
Brown, K. B., McIntosh, J. C., Rademacher, L. K., and Lohse, K. A. (2011). Impacts of agricultural irrigation recharge on groundwater quality in a basalt aquifer system (Washington, USA): a multi-tracer approach. Hydrogeol. J. 19, 1039–1051. doi: 10.1007/s10040-011-0736-z
Brown, S., Beecher, N., and Carpenter, A. (2010). Calculator tool for determining greenhouse gas emissions from biosolids processing and end use. Environ. Sci. Technol. 44, 9509–9515. doi: 10.1021/es101210k
Brown, T. T., and Huggins, D. R. (2012). Soil carbon sequestration in the dryland cropping region of the Pacific Northwest. J. Soil Water Cons. 67, 406–416. doi: 10.2489/jswc.67.5.406
Burke, I., Kahl, C. K., Tautges, N., and Young, F. L. (2017). “Integrated weed management,” in Advances in Dryland Farming in the Inland Pacific Northwest, eds G. Yorgey and C Kruger (Pullman, WA: Washington State Extension Publication EM108), 353–398.
Carlson, J. E., and Dillman, D. A. (1988). The influence of farmers' mechanical skill on the development and adoption of a new agricultural practice. J. Rural Soc. 53, 235–245.
Caron, P., Bienabe, E., and Hainzelin, E. (2014). Making transition towards ecological intensification of agriculture a reality: the gaps in and the role of scientific knowledge. Curr. Opin. Environ. Sustain. 8, 44–52.
CGIAR (2012). “Strategies for combating climate change in drylands agriculture,” in International Conference on Food Security in Dry Lands (Doha). Available online at: https://assets.publishing.service.gov.uk/media/57a08a89e5274a31e000065c/Strategies_Combating_Climate_Change_Dry_Areas.pdf (Accessed on February 05, 2017)
Chen, C., Miller, P., Muehlbauer, F., Neill, K., Wichman, D., and McPhee, K. (2006). Winter pea and lentil response to seeding date and micro- and macro-environments. Agron. J. 98, 1655–1663. doi: 10.2134/agronj2006.0085
Chi, J., Maureira, F., Waldo, S., Pressley, S. N., Stöckle, C. O., O'Keeffe, P. T., et al. (2017). Carbon and water budgets in multiple wheat-based cropping systems in the Inland Pacific Northwest US: comparison of CropSyst simulations with eddy covariance measurements. Front. Ecol. Environ. 5:50. doi: 10.3389/fevo.2017.00050
Cogger, C. G., Bary, A. I., Kennedy, A. C., and Fortuna, A.-M. (2013). Long-term crop and soil response to biosolids applications in dryland wheat. J. Environ. Qual. 42, 1872–1880. doi: 10.2134/jeq2013.05.0109
Collins, H. P., Delgado, J. A., Alva, A. K., and Follett, R. F. (2007). Use of nitrogen-15 isotopic techniques to estimate nitrogen cycling from a mustard cover crop to potatoes. Agron. J. 99, 27–35. doi: 10.2134/agronj2005.0357
Conner, D., Miller, J., Zia, A., Wang, Q., and Darby, H. (2016). Conjoint analysis of farmers' response to conservation incentives. Sustain 8:684.
Connolly, J. R., McCracken, V. A., and Painter, K. M. (2015). Wheat and Canola Rotations in Eastern Washington Low Rainfall Regions (<12”) (Oilseed Series). Pullman, WA: Washington State University Extension Technical Bulletin TB09E.
Connolly, J. R., McCracken, V. A., and Painter, K. M. (2016). Enterprise Budgets: Wheat and Canola Rotations in Eastern Washington Intermediate Rainfall Regions 12” to 16” (Oilseed Series). Pullman, WA: Washington State University Extension Technical Bulletin TB010E.
Coughenour, M., and Chamala, S. (2000). Conservation Tillage and Cropping Innovation: Constructing the New Culture of Agriculture. Ames, IA: Iowa State University. doi: 10.1002/9780470290149
Cox, D., Bezdicek, D., and Fauci, M. (2001). Effects of compost, coal ash, and straw amendments on restoring the quality of eroded Palouse soil. Biol. Fert. Soils 33, 365–372. doi: 10.1007/s003740000335
Cutforth, H. W., and McConkey, B. G. (1997). Stubble height effects on microclimate, yield and water use efficiency of spring wheat grown in a semiarid climate on the Canadian prairies. Can. J. Plant Sci. 77, 359–366. doi: 10.4141/P96-153
Cutforth, H., McConkey, B., Angadi, S., and Judiesch, D. (2011). Extra-tall stubble can increase crop yield in the semiarid Canadian prairie. Can. J. Plant Sci. 91, 783–785. doi: 10.4141/cjps10168
DeVuyst, E. A., and Halvorson, A. D. (2004). Economics of annual cropping versus crop–fallow in the Northern Great Plains as influenced by tillage and nitrogen. Agron. J. 96, 148–153. doi: 10.2134/agronj2004.0148
Dillman, D. A., Beck, D. M., and Carlson, J. E. (1987). “Factors influencing the diffusion of no-till agriculture in the Pacific Northwest,” in STEEP-Soil Conservation Concepts and Accomplishments, ed L. Elliot (Pullman, WA: Washington State University Press), 343–364.
Eigenbrode, S. D., Bechinski, E. J., Bosque-Pérez, N. A., Crowder, D., Rashed, A., Rondon, S. J., et al. (2017). “Insect management strategies,” in Advances in Dryland Farming in the Inland Pacific Northwest, eds G. Yorgey and C. Kruger (Pullman, WA: Washington State Extension Publication EM108).
EPA (2010). “EPA lifecycle analysis of greenhouse gas emissions from renewable fuels,” in Environmental Protection Agency Office of Transportation and Air Quality EPA-420-F-10-006. Available at: https://www.epa.gov/sites/production/files/2015-08/documents/420f10006.pdf (Accessed on December 02, 2012)
Erb, G. (2017). New Mill Will Turn E. Washington Wheat Straw from Waste to Resource. Seattle Times. Available online at: https://www.seattletimes.com/business/new-mill-will-turn-e-washington-wheat-straw-from-waste-to-resource/ (Accessed on August 28, 2017)
Esser, A. D., and Jones, R. (2013). No-till and conventional tillage fallow winter wheat production comparison in the dryland cropping region of Eastern Washington. J. NACAA 6. Available online at: https://www.nacaa.com/journal/index.php?jid=227
Fiez, T. E., Miller, B. C., and Pan, W. L. (1994). Assessment of spatially variable nitrogen fertilizer management in winter wheat. J. Prod. Ag. 7, 86–93. doi: 10.2134/jpa1994.0086
Frear, C. S., Ma, J., and Yorgey, G. G. (in press). Approaches to Nutrient Recovery from Dairy Manure. Pullman, WA: Washington State University Extension Publication.
Fuerst, E. P., Koenig, R. T., Kugler, J., Painter, K., Stannard, M., and Goldberger, J. (2009). Organic Alfalfa Management Guide. Pullman, WA: Washington State University Extension EB2039E.
Fulmer, E., and Heileman, W. H. (1899). Fertilizers. Part 1. Principles Underlying the Use of Fertilizers. Part II. Sources and Compositions of Fertilizers. Pullman, WA: Agricultural College Experiment Station; State College of Washington.
GPO Government Publishing Office (2005). 109th Congress. Energy policy act of 2005. Public Law 109-58. Available online at: www.gpo.gov/fdsys/pkg/PLAW-109publ58/pdf/PLAW-109publ58.pdf (Accessed on January, 28 2017).
Galinato, S. P., Yoder, J. K., and Granatstein, D. (2011). The economic value of biochar in crop production and carbon sequestration. Energ. Policy 39, 6344–6350. doi: 10.1016/j.enpol.2011.07.035
GAO (2014). USDA's Ongoing Efforts can be Enhanced with Better Metrics and More Relevant Information for Farmers. Washington DC: Government Accounting Office GAO-14–755.
Ghimire, R., Machado, S., and Bista, P. (2017). Soil pH, soil organic matter, and crop yield in wheat-fallow systems. Agron. J. 109, 1–12. doi: 10.2134/agronj2016.08.0462
Gollany, H. T., Fortuna, A.-M., Samuel, M. K., Young, F. L., Pan, W. L., and Pecharko, M. (2013). Estimated soil organic carbon accretion vs. sequestration using chemical and physical fractionation and the CQESTR model. Soil Sci. Soc. Am. J. 77, 618–629. doi: 10.2136/sssaj2012.0303
Graham-Rowe, D. (2011). Agriculture: beyond food versus fuel. Nature 474, S6–S8. doi: 10.1038/474S06a
Granatstein, D., Kruger, C. E., Collins, H., Galinato, S., Garcia-Perez, M., and Yoder, J. (2009). Use of Biochar from the Pyrolysis of Waste Organic Material as a Soil Amendment. Final project report, Center for Sustaining Agriculture and Natural Resources, Washington State University, Wenatchee, WA, 168. Available online at: https://fortress.wa.gov/ecy/publications/publications/0907062.pdf (Accessed on November 21, 2016)
Gray, L. C., Bennett, J. B., Kraemer, E., and Sparhawk, W. N. (1938). “The causes: traditional attitudes and institutions” in Soils and Men: 1938 Yearbook of Agriculture, eds H. G. Knight, C. E. Kellogg, C. P. Barnes, M. A. McCall, B. W. Allin, A. L. Patrick, O. E. Baker, C. R. Enlow, E. N. Bressman, E. N. Munns, and G. Hambidge, House Document No. 38 (Washington, DC: United States Department of Agriculture, United States Government Printing Office), 111–136.
Greene, C., Ferreira, G., Carlson, A., Cooke, B., and Hitaj, C. (2017). Growing Organic Demand Provides High-Value Opportunities for Many Types of Producers. Amber Waves. Washington DC: United States Department of Agriculture Economic Research Service. Available online at: https://www.ers.usda.gov/amber-waves/2017/januaryfebruary/growing-organic-demand-provides-high-value-opportunities-for-many-types-of-producers/ (Accessed on February 27, 2017)
Guy, S. O., and Lauver, M. A. (2007). No-till and conventional-till effects on spring wheat in the Palouse. Crop. Manag. 6. doi: 10.1094/CM-2007-0508-01-RS
Hergert, G. W., Pan, W. L., Huggins, D. R., Grove, J. H., Peck, T. R., and Malzer, G. L. (1997). “The adequacy of current fertilizer recommendations for soil specific management,” in The State of Site-Specific Management for Agriculture, eds F. J. Pierce and E. J. Sadler (Madison, WI: ASA, CSSA, SSSA), 283–300.
Howden, S. M., Soussana, J.-F., Tubiello, F. N., Chhetri, N., Dunlop, M., and Meinke, H. (2007). Adapting agriculture to climate change. Proc. Natl. Acad. Sci. U.S. A. 104, 19691–19696.
Huggins, D. R., Kruger, C. E., Painter, K. M., and Uberuaga, D. P. (2014). Site-specific trade-offs of harvesting cereal residues as biofuel feedstocks in dryland annual cropping systems of the Pacific Northwest, USA. Bioenerg. Res. 7, 598–608. doi: 10.1007/s12155-014-9438-4
Huggins, D. R., and Painter, K. (2011). Spring and Winter Canola Research at Cook Agronomy Farm. Washington Biofuels Cropping Systems Annual Report, Washington State University, Pullman, WA. Available online at: http://css.wsu.edu/oilseeds/production-information/ (Accessed on November 25, 2015)
Huggins, D. R., and Pan, W. L. (1991). Wheat stubble management affects survival, growth and yield of winter legumes. Soil Sci. Soc. Am. J. 55, 823–829. doi: 10.2136/sssaj1991.03615995005500030032x
Huggins, D. R., and Pan, W. L. (1993). Component analysis of cropping system differences in nitrogen use efficiency. Agron. J. 85, 898–905. doi: 10.2134/agronj1993.00021962008500040022x
Ibrahim, H. M., and Huggins, D. R. (2011). Spatio-temporal patterns of soil water storage under dryland agriculture at the watershed scale. J. Hydrol. 404, 186–197. doi: 10.1016/j.jhydrol.2011.04.029
IPCC (International Panel on Climate Change) (2007a). “Climate change 2007: synthesis report,” in Contribution of Working Groups I, II and III to the Fourth Assessment Report of the Intergovernmental Panel on Climate Change, eds R. K. Pachauri and A. Reisinger (Geneva: IPCC).
IPCC (International Panel on Climate Change) (2007b). “Climate change 2007: Impacts, adaptation and vulnerability,” in Working Group II Contribution to the Fourth Assessment Report of the IPCC, eds M. L. Parry, O. F. Canziani, J. P. Palutikof, P. J. van der Linden, and C. E. Hansen (Cambridge: Cambridge University Press).
IPNI (2012). A Nutrient Use Information System (NuGIS) for the U.S. January 12, 2012. Norcross, GA: International Plant Nutrition Institute. Available online at: www.ipni.net/nugis (Accessed on November, 2016).
Ippolito, J. A., Barbarick, K. A., and Norvell, K. L. (2007). Biosolids impact soil phosphorus accountability, fractionation, and potential environmental risk. J. Environ. Q. 36, 764–772. doi: 10.2134/jeq2006.0308
Janosky, J. S., Young, D. L., and Schillinger, W. F. (2002). Economics of conservation tillage in a wheat-fallow rotation. Agron. J. 94, 527–531. doi: 10.2134/agronj2002.5270
Janzen, H. H. (2001). Soil science on the Canadian prairies-peering into the future from a century ago. Can. J. Soil Sci. 81, 489–503. doi: 10.4141/S00-054
Johnson-Maynard, J. L., Umiker, K. J., and Guy, S. O. (2007). Earthworm dynamics and soil physical properties in the first three years of no-till management. Soil Till Res 94, 338–345. doi: 10.1016/j.still.2006.08.011
Jonovic, D. J. (1997). The Ultimate Legacy: How Owners of Family and Closely Held Businesses Can Achieve their Real Purpose. Cleveland, OH: Jamieson Press.
Kaiser, V. G., Pawson, W. W., Groeneveld, M. H. H., and Brough, O. L. Jr. (1954). Soil Losses on Wheat Farms in the Palouse Wheat-Pea Area. Pullman, WA: Washington Agricultural Experiments Station Circular 255, State College of Washington.
Karimi, T., Stöckle, C. O., Higgins, S. S., Nelson, R. L., and Huggins, D. (2017). Projected dryland cropping system shifts in the Pacific Northwest in response to climate change. Front. Ecol. Environ. 5:20. doi: 10.3389/fevo.2017.00020
Kaur, H., Huggins, D. R., Rupp, R. A., Abatzoglou, J. T., Stöckle, C. O., and Reganold, J. P. (2017). Agro-ecological class stability decreases in response to climate change projections for the Pacific Northwest, USA. Front. Ecol. Environ. 5:74. doi: 10.3389/fevo.2017.00074
Keller, C. K., Butchera, C. N., Smith, J. L., and Allen-King, R. M. (2007). Nitrate in tile drainage of the semiarid Palouse basin. J. Environ. Qual. 37, 353–361. doi: 10.2134/jeq2006.0515
Kirby, E. M., Pan, W. L., Huggins, D. R., Painter, K. M., and Bista, P. (2017a). “Rotational diversification and intensification,” in Advances in Dryland Farming in the Inland Pacific Northwest, eds G. Yorgey and C. Kruger (Pullman, WA: Washington State Extension Publication EM108–05), 163–236.
Kirby, E. M., Paulitz, T. C., Murray, T. D., Schroeder, K. L., and Chen, X. M. (2017b). “Disease management for wheat and barley,” in Advances in Dryland Farming in the Inland Pacific Northwest, eds G. Yorgey and C. Kruger (Pullman, WA: Washington State Extension Publication EM108–05), 399–468.
Kirby, E., and Granatstein, D. (2017). Certified Organic Acreage and Sales in Washington State: 2007-2015. Pullman, WA: Washington State Extension Publication TB43E.
Kirkegaard, J. A., Peoples, M. B., Angus, J. F., and Unkovich, M. J. (2011). “Diversity and evolution of rainfed farming systems in Southern Australia,” in Rainfed Farming Systems, eds P. Tow, I. Cooper, I. Partridge, and C. Birch (Dordrecht: Springer). doi: 10.1007/978-1-4020-9132-2_26
Kirkegaard, J., Christen, O., Krupinsky, J., and Layzelld, D. (2008). Break crop benefits in temperate wheat production. Field Crops Res. 107, 185–195. doi: 10.1016/j.fcr.2008.02.010
Klerkx, L., Aarts, N., and Leeuwis, C. (2010). Adaptive management in agricultural innovation systems: The interactions between innovation networks and their environment. Agric. Syst. 103, 390–400. doi: 10.1016/j.agsy.2010.03.012
Koenig, R. T., Cogger, C. G., and Bary, A. I. (2011). Dryland winter wheat yield, grain protein, and soil nitrogen responses to fertilizer and biosolids applications. Appl. Environ. Soil Sci. 2011:925462. doi: 10.1155/2011/925462
Koenig, R. T., Horneck, D. A., Platt, T., Petersen, P., Stevens, R., Fransen, S., et al. (2009). Nutrient Management Guide for Dryland and Irrigated Alfalfa in the Inland Northwest. Pullman, WA: Washington State Pacific Northwest Extension Publication.
Kok, H., Papendick, R. I., and Saxton, K. E. (2009). STEEP: impact of long-term conservation farming research and education in Pacific Northwest wheatlands. J. Soil Water Cons. 64, 253–264. doi: 10.2489/jswc.64.4.253
Kruger, C., Stockle, C., Shrestha, D., Painter, K., and Pan, B. (2015). “Life cycle assessment of Pacific Northwest canola-based biodiesel,” in Regional Approaches to Climate Change for Northwest Agriculture, Climate Science Northwest Farmers Can Use, eds D. D. Laursen, S. Eigenbrode, B. Mahler, and B. Stokes (Moscow, ID: University of Idaho), 26–28.
Lang, M. B. (2017). Oilseeds and biofuels in Washington state. Washington Oilseeds Cropping Systems. Washington Department of Agriculture. Available online at: http://css.wsu.edu/oilseeds/files/2017/03/WOCS-Lang-2017-Presentation-Handout-1.pdf (Accessed on April 03, 2017).
Lang, M. B., and Flynn, V. (1989). Washington's Centennial Farms. Yesterday and Today. Olympia, WA: Washington State Department of Agriculture.
Lindstrom, M. J., Koehler, F. E., and Papendick, R. I. (1974). Tillage effects on fallow water storage in eastern Washington dryland region. Agron. J. 66, 312–316. doi: 10.2134/agronj1974.00021962006600020036x
Long, D., Young, F., Schillinger, W., Reardon, C., Williams, J., Allen, B., et al. (2016). Ongoing development of dryland oilseed production systems in northwestern region of the United States. Bioenergy 9, 412–429. doi: 10.1007/s12155-016-9719-1
Lorent, L., Roberts, D., and Burke, I. (2016). Organic Small Grain Production in The Inland Pacific Northwest: A Collection of Case Studies. Pacific Northwest Publication 683. Pullman, WA: Washington State University.
Lutcher, L. K., Wysocki, D. J., Corp, M. K., and Horneck, D. A. (2009). Agronomic Guidelines for Flexible Cropping Systems in Dryland Areas of Oregon. Corvallis, OR: Oregon State University Extension Service EM 8999–E.
Maaz, T. M., Wulfhorst, J. D., McCracken, V., Kirkegaard, J., Huggins, D. R., Roth, I., et al. (in press). Economic, policy, social challenges of introducing oilseed pulse crops into dryland wheat rotations. Agric. Ecosyst. Environ. doi: 10.1016/j.agee.2017.03.018
Maaz, T., and Pan, W. L. (2017). Residual fertilizer, crop sequence, and water availability impact rotational nitrogen balances. Agron. J. 109, 2839–2862. doi: 10.2134/agronj2016.08.0457
Maaz, T., Pan, W. L., and Hammac, A. H. (2016). Influence of soil nitrogen and water supply on canola nitrogen use efficiency of canola. Agron. J. 108, 2099–2109. doi: 10.2134/agronj2016.01.0008
Machado, S. (2011). Soil organic carbon dynamics in the Pendleton long-term experiments: implications for biofuel production in Pacific Northwest. Agron. J. 103, 253–260. doi: 10.2134/agronj2010.0205s
Machado, S., and Pritchett, L. (2014). “Biochar effects on wheat productivity in chemical fallow,” in Poster Presentation Presented at the Regional Approaches to Climate Change for Pacific Northwest Agriculture Annual Meeting (Richland, WA).
Machado, S., Pritchett, L., and Petrie, S. (2015). No-tillage cropping systems can replace traditional summer fallow in north-central Oregon. Agron. J. 107, 1863–1877. doi: 10.2134/agronj14.0511
Madsen, I. (2017). Nitrogen-Plant-Soil-Interactions at the Root-Rhizosphere, Cropping System, and National Scales. Ph.D. dissertation, Washington State University, Pullman, WA.
Magdoff, F., Lanyon, L., and Liebhardt, B. (1997). Nutrient cycling, transformations, and flows: implications for a more sustainable agriculture. Adv. Agron. 60, 1–73. doi: 10.1016/S0065-2113(08)60600-8
Mahler, B., Pan, B., and Wysocki, D. (2015). “The importance of soil fertility in crop production,” in Regional Approaches to Climate Change for Northwest Agriculture, Climate Science Northwest Farmers Can Use, eds K. Borrelli, D. Daley Laursen, S. Eigenbrode, B. Mahler, and R. Pepper (Moscow, ID: University of Idaho).
Mallory, E. B., Fiez, T., Veseth, V. J., Roe, R. D., and Wysocki, D. J. (2001). Direct Seeding Case Study Series for the Inland Northwest. Pullman WA: Pacific Northwest Extension Publications, Washington State University Cooperative Extension, Oregon State University Extension Service, University of Idaho Cooperative Extension System, and the U. S. Department of Agriculture.
Matson, P. A., Parton, W. J., Power, A. G., and Swift, M. J. (1997). Agricultural intensification and ecosystem properties. Science 277, 504–509. doi: 10.1126/science.277.5325.504
McGee, R. J. (2016). “Breeding fall planted pulse crops,” in Pacific Northwest Direct Seed Association Annual Conference. Available online at: http://www.directseed.org/files/9514/5335/1350/Breeding_Fall_Planted_Pulse_Crops_Rebecca_McGee.pdf (Accessed on April 15, 2017).
McGregor, A. C. (1982). Counting Sheep. From Open Range to Agribusiness on the Columbia Plateau. Seattle, WA: University of Washington Press.
Nehring, R. (2016). Fertilizer Use & Markets. United States Department of Agriculture Economic Research Service, Amber Waves. Available online at: https://www.ers.usda.gov/topics/farm-practices-management/chemical-inputs/fertilizer-use-markets/ (Accessed on April 08, 2017)
Nielsen, D. C., Lyon, D. W., Hergert, G. W., Higgins, R. K., Calderón, F. J., and Vigil, M. F. (2015). Cover crop mixtures do not use water differently than single-species plantings. Agron. J. 107, 1025–1038. doi: 10.2134/agronj14.0504
Norton, R. (2013). “4R Canola nutrition guide, Canola Technology Update: Nutrient Management V1,” in International Plant Nutrition Institute. Available online at: anz.ipni.net/beagle/ANZ-3176&f=Canola%204R%20Guide.pdf (Accessed on May 1, 2017).
O'Conner, C. (2013). Soil Matters: How the Federal Crop Insurance Program Should be Reformed to Encourage Low-Risk Farming Methods with High-Reward Environmental Outcomes. NRDC Issue Paper, ip:13-04-a. Available online at: https://www.nrdc.org/sites/default/files/soil-matters-IP.pdf (Accessed on March 25, 2017)
Oldenstadt, D. L., Allan, R. E., Bruehl, G. W., Dillman, D. A., Michalson, E. L., Papendick, R. I., et al. (1982). Solutions to Environmental and Economic Problems (STEEP). Science 217, 904–909. doi: 10.1126/science.217.4563.904
O'Leary, M., Usibelli, T., and Moulton, P. (2013). 2012 Energy Freedom Status Report: Funding Bioenergy and Clean Energy Development in Washington State. Olympia, WA: Washington State Department of Commerce. Available online at: https://www.digitalarchives.wa.gov/do/739075E9DE5F1E93161EEE1F88FBFF5A.pdf (Accessed on January 28, 2017).
Pan, W., Schillinger, W., Huggins, D., Koenig, R., and Burns, J. (2007). “Fifty years of predicting wheat nitrogen requirements based on soil water, yield, protein and nitrogen efficiencies,” in Managing Crop Nitrogen for Weather, ed T. Bruulsema (Norcross, GA: International Plant Nutrition Institute), 10-1–10-8.
Pan, W. L., and Hopkins, A. G. (1991). Winter barley development, N and P use: responses to no-till and N management at slope positions of eroded toposequences. Plant Soil 135, 21–29.
Pan, W. L., Huggins, D. R., Malzer, G. L., and Smith, J. L. (1997). “Variable soil-plant relationships: implications for site-specific nitrogen management,” in The State of Site-Specific Management for Agriculture, eds F. J. Pierce and E. J. Sadler (Madison, WI: ASA, CSSA, SSSA), 81–100.
Pan, W. L., Maaz, T. M., Hammac, W. A., McCracken, V. A., and Koenig, R. T. (2016a). Mitscherlich-modeled, semi-arid canola nitrogen requirements influenced by soil nitrogen and water. Agron. J. 108, 884–894. doi: 10.2134/agronj2015.0378
Pan, W. L., Maaz, T. M., Madsen, I. J., Reese, M., Hammac, W. A., Wysocki, D., et al. (2017a). 4R nitrogen management when integrating canola into semi-arid wheat. Crops Soils 50, 16–66. doi: 10.2134/cs2017.50.0309
Pan, W. L., Port, L. E., Xiao, Y., Bary, A. I., and Cogger, C. G. (2017b). Soil carbon and nitrogen fraction accumulation with long-term biosolids applications. Soil Sci. Soc. Am. J. 0. doi: 10.2136/sssaj2017.03.0075. [Epub ahead of print].
Pan, W. L., Young, F. L., Hulbert, S. C., Huggins, D. R., and Maaz, T. M. (2016b). Canola Integration into semi-arid wheat cropping systems of the inland Pacific Northwestern USA. Crop Pasture Sci. 67, 253–265.
Pan, W. L., Young, F. L., and Kidwell, K. K. (2001). “Carbon and nitrogen cycling in direct-seeded spring cereal alternatives to summer fallow,” in Proceedings of the Western Nutrient Management Conference, Vol. 4, ed B. Brown (Brookings, SD: Potash and Phosphate Institute), 202–209.
Papendick, R. I., Young, D. L., McCool, D. K., and Krauss, H. A. (1985). “Regional effects of soil erosion on crop productivity: the Palouse area of the Pacific Northwest (USA),” in Soil Erosion and Crop Productivity, eds R. F. Follett and B. A. Stewart (Madison, WI: American Society of Agronomy), 305–320.
PNDSA (2017). Pacific Northwest Direct Seed Association. Farming for the Future. Available online at: http://www.directseed.org/ (Accessed on March 01, 2017).
Port, L. (2016). A Diversified High-Residue No-Till Cropping System for the Low Rainfall Zone. MS thesis, Washington State University, Pullman,WA.
Reardon, T., Wesseler, J., and Zilberman, D. (2016). Strategic Perspectives on Innovation in Agrifood Business In Emerging Versus Developed Markets. April 19-21, 2016 University of California Berkeley International & Executive Programs Workshop Report. Available online at: https://iep.berkeley.edu/content/strategic-perspectives-innovation-agrifood-supply-chains-2016. (Accessed on March 01, 2017).
Reganold, J. P., Jackson-Smith, D., Batie, S. S., Harwood, R. R., Kornegay, J. L., Bucks, D., et al. (2011). Transforming U.S. Agriculture. Science 332, 670–671. doi: 10.1126/science.1202462
Reicosky, D. C., Hatfield, J. L., and Sass, R. L. (2000). “Agricultural contributions to greenhouse gas emissions,” in Climate Change and Global Security, eds K. R. Reddy and H. F. Hodges (Wallingford: CAB International), 37–56. doi: 10.1079/9780851994390.0037
Riar, D. S., Ball, D. A., Yenish, J. P., and Burke, I. C. (2011). Light-activated sensor-controlled sprayer provides effective postemergence control of broadleaf weeds in fallow. Weed. Technol. 25, 447–453. doi: 10.1614/WT-D-10-00013.1
Ribaudo, M., Delgado, J., Hansen, L., Livingston, M., Mosheim, R., and Williamson, J. (2011). Nitrogen in Agricultural Systems: Implications for Conservation Policy. ERR-127. U.S. Department of Agriculture Economic Research Service September 2011. Available online at: https://www.ers.usda.gov/publications/pub-details/?pubid=44919 (Accessed on March 01, 2017).
Roberts, D., Fleming, F. J., Gross, C., Rush, T., Warner, E., Laney, C., et al. (2016). “Cover cropping for the intermediate precipitation zone of dryland eastern Washington,” in 2016 Dryland Field Day Abstracts (Moscow ID: University of Idaho, Oregon State University and Washington State University).
Robertson, G. P., and Swinton, S. M. (2005). Reconciling agricultural productivity and environmental integrity: a grand challenge for agriculture. Front. Ecol. Environ. 3, 38–45.
Schillinger, W. F. (2017). Winter pea: promising new crop for Washington's dryland wheat-fallow region. Front. Ecol. Environ. 5:43.
Schillinger, W. F., and Papendick, R. I. (2008). Then and now: 125 years of dryland wheat farming in the Inland Pacific Northwest. Agron. J. 100(Suppl. 3), S166–S182. doi: 10.2134/agronj2007.0027c
Seamon, E., Roesch-McNally, G., McNamee, L., Roth, I., Wulfhorst, J. D., Eigenbrode, S. D., et al. (2016). Producer Perceptions on Climate Change and Agriculture: A Statistical Atlas. Moscow, ID: University of Idaho Agricultural Economics Extension Series 17–01.
Shcherbak, I., Millar, N., and Robertson, G. P. (2014). Global metaanalysis of the nonlinear response of soil nitrous oxide (N2O) emissions to fertilizer nitrogen. Proc. Natl. Acad. Sci. U.S.A. 25, 9199–9204. doi: 10.1073/pnas.1322434111
Sievers, F. J., and Holtz, H. F. (1922). The Silt Loam Soils of Eastern Washington and Their Management. Pullman, WA: State College of Washington Agricultural Experiment Station.
Smit, B., and Skinner, M. W. (2002). Adaptation options in agriculture to climate change: a typology. Mitig. Adapt. Strat. Glob. Chang. 7, 85–114. Available online at: http://citeseerx.ist.psu.edu/viewdoc/download?doi=10.1.1.408.997&rep=rep1&type=pdf
Smith, E. G., Upadhyay, B. M., Blackshaw, R. E., Beckie, H. J., Harker, K. N., and Clayton, G. W. (2006). Economic benefits of integrated weed management systems in field crops in the Dark Brown and Black soil zones of western Canada. Can. J. Plant Sci. 86, 1273–1279. doi: 10.4141/P05-181
Smith, P., Martino, D., Cai, Z., Gwary, D., Janzen, H., Kumar, P., et al. (2007). “Agriculture,” in: Climate Change 2007: Mitigation. Contribution of Working Group III to the Fourth Assessment Report of the Intergovernmental Panel on Climate Change, eds B. Metz, O. R. Davidson, P. R. Bosch, R. Dave, and L. A. Meyer (Cambridge: Cambridge University Press).
Snyder, C. S., Bruulsema, T. W., Jensen, T. L., and Fixen, P. E. (2009). Review of greenhouse gas emissions from crop production systems and fertilizer management effects. Agri. Ecosyst. Environ. 133, 247–266.
Sowers, K. E. (2017). 2017 Spring Oilseed Suppliers in the PNW. Washington Oilseed Cropping Systems. Pullman, WA: Washington State University. Available online at: http://css.wsu.edu/oilseeds//files/2012/09/2017SpringOilseedSupplyfinal-1.pdf (Accessed on April 25, 2017).
Sowers, K. E., and Pan, B. (2012). Executive Summary. Biofuels cropping system project 2012 annual progress report. Pullman, WA: Washington State University.
Sowers, K. E., Pan, W. L., Miller, B. C., and Smith, J. L. (1995). Nitrogen use efficiency of split applications in soft white winter wheat. Agron. J. 86, 942–948. doi: 10.2134/agronj1994.00021962008600060004x
Sowers, K. E., Roe, R. D., and Pan, W. L. (2011). Oilseed Production Case Studies in the Eastern Washington High Rainfall Zone. Pullman, WA: Washington State Extension Manual EM037E.
Sowers, K. E., Roe, R. D., and Pan, W. L. (2012). Oilseed Production Case Studies in the Eastern Washington Low to Intermediate High Rainfall Zone. Pullman, WA: Washington State Extension Manual EM 048E.
Spillman, W. J. (1906). Renovation of Worn Out Soils. Farmers Bulletin No. 245. USDA, Washington, DC: Washington Government Printing Office.
Stöckle, C. S., Higgins, S., Kemanian, A., Nelson, R., Huggins, D., Marcos, J., et al. (2012). Carbon storage and nitrous oxide emissions of cropping systems in Washington: a simulation study. J. Soil Water Cons. 67, 365–377. doi: 10.2489/jswc.67.5.365
Stöckle, C. O., Higgins, S., Nelson, R., Abatzoglou, J., Huggins, D., Pan, W., et al. (2017). Evaluating opportunities for an increased role of winter crops as adaptation to climate change in dryland cropping systems of the U.S. Inland Pacific Northwest. Climate Change. doi: 10.1007/s10584-017-1950-z. [Epub ahead of print].
Stöckle, C. S., Nelson, R. L., Higgins, S., Brunner, J., Grove, G., Boydston, R., et al. (2010). Assessment of climate change impact on eastern Washington agriculture. Clim. Change 2010, 77–102. doi: 10.1007/s10584-010-9851-4
Stubbs, T. L., Kennedy, A. C., and Schillinger, W. F. (2004). Soil ecosystem changes during the transition to no-till cropping. J. Crop Improv. 11, 105–135. doi: 10.1300/J411v11n01_06
Sullivan, D. M., Cogger, C. G., and Bary, A. I. (2015). Fertilizing with Biosolids. Corvallis, OR: Oregon State University Extension Service. A Pacific Northwest Extension Publication. PNW 508.
Sullivan, L. S., Young, F. L., Smiley, R. W., and Alldredge, J. R. (2013). Weed and disease incidence in no-till, facultative wheat in the Pacific Northwest, USA. Crop Prot. 53, 132–138. doi: 10.1016/j.cropro.2013.06.013
Tautges, N. E., Burke, I. C., Borrelli, K., and Fuerst, E. P. (2016). Competitive ability of rotational crops with weeds in dryland organic wheat production systems. Renew. Ag. Food Syst. 32, 57–68. doi: 10.1017/S1742170516000028
Thompson, W. H., and Carter, P. G. (2014). “Cover crop water consumption in southeastern Washington palouse,” in Poster in ASA, CSSA and SSSA International Meeting. Available online at: https://scisoc.confex.com/scisoc/2014am/webprogram/Paper84949.html (Accessed on September 01, 2017).
Thorne, M. E., Young, F. L., Pan, W. L., Bafus, R., and Alldredge, J. R. (2003). No-till spring cereal cropping systems reduce wind erosion susceptibility in the wheat/fallow region of the Pacific Northwest. J. Soil Water Conserv. 58, 250–257. Available online at: https://naldc.nal.usda.gov/download/48775/PDF
Tilman, D., Balzer, C., Hill, J., and Befort, B. L. (2011). Global food demand and the sustainable intensification of agriculture. Proc. Nat. Acad. Sci. U.S.A. 108, 20260–20264. doi: 10.1073/pnas.1116437108
Tilman, D., Cassman, K. G., Matson, P. A., Naylor, R., and Polasky, S. (2002). Agricultural sustainability and intensive production practices. Nature 418, 671–677. doi: 10.1038/nature01014
Unger, P. W., Fryrear, D. W., and Lindstrom, M. J. (2006). “Soil conservation,” in Dryland Agriculture, Agronomy Monograph 23, eds G. A. Peterson, P. W. Unger, and W. A. Payne (Madison, WI: ASA-CSSA-SSSA), 87–112.
USDA (2010). U.S. Department of Agriculture Strategic Plan FY 2010-2015. Available online at: www.ocfo.usda.gov/usdasp/sp2010/sp2010.pdf (Accessed on March 01, 2017)
USDA (2014). U.S. Department of Agriculture Climate Change Adaptation Plan. Available online at: www.ocfo.usda.gov/usdasp/usdasp.htm (Accessed on March 01, 2017)
USDA-ERS (2016). Agricultural Production and Mitigation. Available online at: https://www.ers.usda.gov/topics/natural-resources-environment/climate-change/agricultural-production-and-mitigation/ (Accessed on March 01, 2017)
USDA-RMA (2016). United State Department of Agriculture Risk Management Agency. Available online at: https://www.rma.usda.gov/ (Accessed on February 15, 2017)
van Groenigen, J. W., Lubbers, I. M., Vos, H. M., Brown, G. G., De Deyn, G. B., and van Groenigen, K. J. (2014). Earthworms increase plant production: a meta-analysis. Sci. Rep. 4:6365. doi: 10.1038/srep06365
Vandemark, G. J., Brick, M. A., Osorno, J. M., Kelly, J. D., and Urrea, C. A. (2014). “Edible grain legumes,” in Yield Gains in Major U.S. Field Crops, CSSA special publication 33, eds S. Smith, B. Diers, J. Specht, and B. Carver (Madison, WI: ASA, CSSA, and SSSA), 87–124.
Venterea, R. T., Halvorson, A. D., Kitchen, N., Liebig, M. A., Cavigelli, M. A., Del Grosso, S. J., et al. (2012). Challenges and opportunities for mitigating nitrous oxide emissions from fertilized cropping systems. Front. Ecol. Environ. 10, 562–570. doi: 10.1890/120062
Waldo, S., Chi, J., Pressley, S. N., O'Keeffe, P., Pan, W. L., Brooks, E., et al. (2016). Assessing carbon dynamics at high and low rainfall agricultural sites in the inland Pacific Northwest US using the eddy covariance method. Agr. Forest Meteorol. 218–219, 25–36. doi: 10.1016/j.agrformet.2015.11.018
Washington Grain Commission (2016). All About Grains. Available online at: http://wagrains.org/all-about-wheat/varieties-of-wheat/ (Accessed on March 29, 2017)
Weddell, B., Brown, T., and Borrelli, K. (2017). “Precision agriculture,” in Advances in Dryland Farming in the Inland Pacific Northwest, eds G. Yorgey and C. Kruger (Pullman, WA: Washington State Extension Publication EM108–05), 319–352.
Weinert, T., Pan, W. L., Moneymaker, M. R., Santo, G. S., and Stevens, R. G. (2002). Nitrogen recycling by non-leguminous winter cover crops to reduce leaching in potato rotations. Agron. J. 94, 365–372.
Williams, J. D., Gollany, H. T., Siemens, M. C., Wuest, S. B., and Long, D. S. (2013). Comparison of runoff, soil erosion, and winter wheat yields from no-till and inversion tillage production systems in northeastern Oregon. J. Soil Water Cons. 64, 43–52. doi: 10.2489/jswc.64.1.43
Williams, J. D., Wuest, S. B., and Long, D. S. (2014). Soil and water conservation in the Pacific Northwest through no-tillage and intensified crop rotations. J. Soil Water Cons. 69, 495–504. doi: 10.2489/jswc.69.6.495
Wood, S., and Cowie, A. (2004). A Review of Greenhouse Gas Emission Factors for Fertiliser Production. IEA Bioenergy Task 38. Available online at: http://www.sciencetheearth.com/uploads/2/4/6/5/24658156/2004_wood_a_review_of_greenhouse_gas_emission_factors.pdf (Accessed on February 01, 2012).
Woodard, J. D. (2016). Integrating high resolution soil data into federal crop insurance policy: implications for policy and conservation. Envir. Sci. Policy 66, 93–100. doi: 10.1016/j.envsci.2016.08.011
Woodard, J. D., Sherrick, B. J., and Schnitkey, G. D. (2011). Actuarial impacts of loss cost ratio ratemaking in U.S. crop insurance programs. J. Agric. Res. Econ. 36, 211–228. Available online at: https://core.ac.uk/download/pdf/6546457.pdf
Wu, J. J. (1999). Crop insurance, acreage decisions, and nonpoint-source pollution. Am. J. Agr. Econ. 81, 305–320. doi: 10.2307/1244583
Xiao, C., Bolton, R., and Pan, W. L. (2007a). Lignin from rice straw kraft pulping: effects on soil aggregation and chemical properties. Biores. Technol. 98, 1482–1488. doi: 10.1016/j.biortech.2005.11.014
Xiao, C., Stevens, R., Fauci, M., Bolton, R., Lewis, M., McKean, W. T., et al. (2007b). Soil microbial activity, aggregation and nutrient responses to straw pulping liquor in corn. Biol. Fert. Soils 43, 709–719. doi: 10.1007/s00374-006-0153-y
Yorgey, G. G., Frear, C. S., Kruger, C. E., and Zimmerman, T. J. (2014). The Rationale for Recovery of Phosphorus and Nitrogen from Dairy Manure. Washington State Extension Publication FS136E. Pullman, WA: Washington State University.
Yorgey, G., Pan, W. L., Awale, R., Machado, S., and Bary, A. (2017b). “Soil amendments,” in Advances in Dryland Farming in the Inland Pacific Northwest, eds G. Yorgey and C. Kruger (Pullman, WA: Washington State Extension Publication EM 108–07), 319–352.
Yorgey, G., Borrelli, K. A., and McGuire Painter, K. (2017c). Strip Tillage of Vegetables with Livestock Integration. Pullman, WA: Washington State University, CSANR. Available online at: https://www.youtube.com/watch?v=KU_9ikqtDVw&list=PLajA3BBVyv1z8zOtVWmznqGJ7ZCP-Xooa&index=14 (Accessed on May 01, 2017).
Yorgey, G., Painter, K., Borrelli, K., Kantor, S., Davis, H., Bernacchi, L., et al. (2017a). Farmer-to-Farmer Case Study Series: Increasing Resilience among farmers in the Pacific Northwest. Moscow, ID: University of Idaho.
Young, D. L. (2009). Biofuels: political/economic boondoggle or energy salvation for Western States? J. Agr. Res. Econ. 34, 383–394. Available online at: http://citeseerx.ist.psu.edu/viewdoc/download?doi=10.1.1.468.5301&rep=rep1&type=pdf
Young, D. L., Kwon, T. J., and Young, F. L. (1994). Profit and risk for integrated conservation farming systems in the Palouse. J. Soil Water Cons. 6, 601–606.
Young, D. L., and Schillinger, W. F. (2012). Wheat farmers adopt the undercutter fallow method to reduce wind erosion and sustain profitability. Soil Till Res. 124, 240–244. doi: 10.1016/j.still.2012.07.001
Young, F. L., Alldredge, A. R., Pan, W. L., and Henning, C. (2015). Comparisons of annual no-till spring cereal cropping systems in the Pacific Northwest. Crop Forage Turf. Manage. doi: 10.2134/cftm2014.0089
Young, F. L., Ogg, A. G. Jr., Boerboom, C. M., and Alldredge, J. R. (1994a). Integration of weed management and tillage practices in spring dry pea production. Agron. J. 86, 868–874.
Young, F. L., Ogg, A. G. Jr., and Papendick, R. I. (1994b). Case studies of integrated-whole farm systems designs: field scale replicated IPM trials. Am. J. Altern. Agr. 9, 52–56.
Young, F. L., Ogg, A. G. Jr., Papendick, R. I., Thill, D. C., and Alldredge, J. R. (1994c). Tillage and weed management affects winter wheat yield in an Integrated Pest Management System. Agron. J. 86, 149–154.
Young, F. L., Whaley, D. K., Lawrence, D. W., and Burke, I. C. (2016). Feral rye (Secale cereale) control in winter canola in the Pacific Northwest. Weed Tech. 30, 163–170. doi: 10.1614/WT-D-15-00109.1
Young, F. L., Whaley, D. K., Pan, W. L., Roe, R. D., and Alldredge, J. R. (2014). Introducing winter canola to the winter wheat-fallow region of the Pacific Northwest. Crop Manage. doi: 10.2134/CM-2013-0023-RS
Keywords: adaptation, mitigation, diversification, intensification, socioeconomic, policy, socioeconomic, win-win
Citation: Pan WL, Schillinger WF, Young FL, Kirby EM, Yorgey GG, Borrelli KA, Brooks ES, McCracken VA, Maaz TM, Machado S, Madsen IJ, Johnson-Maynard JL, Port LE, Painter K, Huggins DR, Esser AD, Collins HP, Stockle CO and Eigenbrode SD (2017) Integrating Historic Agronomic and Policy Lessons with New Technologies to Drive Farmer Decisions for Farm and Climate: The Case of Inland Pacific Northwestern U.S. Front. Environ. Sci. 5:76. doi: 10.3389/fenvs.2017.00076
Received: 04 May 2017; Accepted: 30 October 2017;
Published: 28 November 2017.
Edited by:
Urs Feller, University of Bern, SwitzerlandReviewed by:
Sileshi Gudeta Weldesemayat, EliSil Environmental Consultants, ZambiaEdmundo Acevedo, Universidad de Chile, Chile
Copyright © 2017 Pan, Schillinger, Young, Kirby, Yorgey, Borrelli, Brooks, McCracken, Maaz, Machado, Madsen, Johnson-Maynard, Port, Painter, Huggins, Esser, Collins, Stockle and Eigenbrode. This is an open-access article distributed under the terms of the Creative Commons Attribution License (CC BY). The use, distribution or reproduction in other forums is permitted, provided the original author(s) or licensor are credited and that the original publication in this journal is cited, in accordance with accepted academic practice. No use, distribution or reproduction is permitted which does not comply with these terms.
*Correspondence: William L. Pan, d2xwYW5Ad3N1LmVkdQ==