- 1University of Bremen, FB02, UFT, General and Theoretical Ecology, Bremen, Germany
- 2University of Bremen, FB04, UFT, Sustainable Chemistry, Bremen, Germany
- 3University of Bremen, FB02, UFT, Soil Microbial Ecology, Bremen, Germany
Silver nanoparticles (AgNP) are integrated into various products due to their antimicrobial characteristics and hence, the application of AgNP is increasing. During production, use and disposal AgNP are emitted and enter the environment via several pathways. Soils are considered a major sink of AgNP. The aim of the present study was to determine the toxic effect of AgNP on Folsomia candida reproduction to illustrate potential impact on terrestrial ecosystems. The AgNP-dependent reduction of F. candida reproduction was studied in RefeSol 01-A, LUFA 2.2 and OECD soil at 0.3 μg–50 mg Ag kg−1. To simulate realistic exposure pathways, effects on F. candida reproduction after the application of AgNP via sewage sludge and after aging this treatment in the soil for up to 140 days were studied using environmentally relevant concentrations. The OECD representative AgNP, NM-300K, and AgNO3, as a metal salt reference, were used in all experiments. The generated data demonstrate that the presence of AgNP in the soil in the low mg Ag kg−1 concentration range results in significant, but concentration independent inhibition of F. candida reproduction in RefeSol 01-A and LUFA 2.2. Significant inhibition of F. candida reproduction due to AgNP was also observed for soil amended with AgNP treated sludge. An increase in inhibition with aging of the AgNP in the soil was evident. In conclusion, our results demonstrate that, at environmentally relevant concentrations, AgNP adsorption to sludge and subsequent aging in soil lead to a toxic effect on soil invertebrates.
Introduction
The use and application of silver nanoparticles (AgNP) is steadily rising and with it the need to assess the potential impacts on the environment. AgNP are integrated into various products due to their antimicrobial characteristics; these range from food packaging (de Moura et al., 2012), paints, sportswear coatings to health care and medical applications (Benn and Westerhoff, 2008; Rai et al., 2009). During production, use and disposal AgNP can be emitted and enter the environment via several pathways; soils are considered a major sink of NP (Keller and Lazareva, 2014). AgNP accumulate in sewage sludge of waste water treatment plants (WWTP) which is applied to agricultural soils as fertilizer (Tourinho et al., 2012; Batley et al., 2013; Schlich et al., 2013b). Some plant protection products and fertilizers also include AgNP and enter terrestrial ecosystems via application to crops (Mishra and Singh, 2015).
Several studies (e.g., Hänsch and Emmerling, 2010; Feng et al., 2013; Schlich et al., 2013b; Simonin and Richaume, 2015) have shown that AgNP at environmentally relevant concentrations below 1 mg Ag kg−1 cause adverse effects to soil organisms of various trophic levels (McKee and Filser, 2016). Predicted environmental concentrations of AgNP in soil and sludge-amended soil differ between studies, but all lay in the ng to μg Ag kg−1 soil range. Massarsky et al. (2014) modeled a yearly global increase of 1.407–6.36 μg Ag kg−1 sewage sludge amended soil. Sun et al. (2014) predicted incremental increase in concentrations in soil and sludge amended soil of 1.2 and 110 ng Ag kg−1 per year, respectively, in the EU. In Denmark the environmental concentration of AgNP is predicted to be 350 ng Ag kg−1 in sludge amended soil by 2020 through accumulation since the year 2000 (Kjølholt et al., 2015). Gottschalk et al. (2009) expect a yearly increase of 526–2,380 ng Ag kg−1 in sludge amended soil in the United States.
The focus in ecotoxicological testing of AgNP in soil has, thus far, been more on microorganisms (Schlich et al., 2013b; Engelke et al., 2014; McKee and Filser, 2016), earthworms (Heckmann et al., 2011; Shoults-Wilson et al., 2011a; Schlich et al., 2013a; van der Ploeg et al., 2014; Novo et al., 2015), enchytraeids (Gomes et al., 2013, 2015; Ribeiro et al., 2015; Bicho et al., 2016) and nematodes (Roh et al., 2009; Meyer et al., 2010). Collembola have received less attention. They are a large and highly abundant group of arthropods which are major contributors to decomposition processes and play important ecological roles in soil (e.g., Filser, 2002). One of the few studies on effects of AgNP on Collembola (Waalewijn-Kool et al., 2014) did not detect toxic effects of paraffin-coated AgNP on Folsomia candida survival and reproduction at very high concentrations (673 mg Ag kg−1) (Waalewijn-Kool et al., 2014). These tests were conducted in a loamy sand soil (LUFA 2.2). In a different study F. candida reproduction had an EC50 of >1,792 mg Ag kg−1 for PVP-coated AgNP in a Canadian agricultural sandy loam soil. In an agricultural silt loam soil the EC50 was estimated >1,855 mg Ag kg−1 for PVP-coated AgNP (Velicogna et al., 2016). With AgNP and LUFA 2.2 soil a dose-response pattern was detected at the concentration range of 64–640 mg Ag kg−1 for F. candida reproduction and the EC50 was at 540 mg kg−1 (Mendes et al., 2015).
The differences in results between studies are in part based on differences in particle characteristics and coatings. Additionally ionic strength and composition, pH and natural organic content of soils affect aggregation or stabilization of AgNP in the environment (Fabrega et al., 2011). This has effects on the fate and bioavailability of AgNP in soil (Handy et al., 2012) and strongly influences AgNP toxicity to earthworms (Shoults-Wilson et al., 2011a) and nitrifying bacteria (Simonin et al., 2015). When performing toxicity tests with AgNP most studies apply as-manufactured nanoparticles to the soil. During the WWTP processing transformations and modifications of the pristine AgNP take place (Kim et al., 2010). Hence, WWTP simulations allow the nanoparticles and ions to be exposed to possible transformation reactions, thus providing maximum environmental relevance (Schlich et al., 2013b).
Based on predicted environmental concentrations for the soil compartment the present study examined concentrations of AgNP in the μg Ag kg−1 and low mg Ag kg−1 range. We used the representative OECD standard silver nanomaterial NM-300K in all our experiments to enable a comparison of effects of dose and soil on Collembola reproduction. In addition to testing pristine NM-300K and AgNO3 as a salt reference, sewage sludge aged with NM-300K in soil for up to 140 days was tested for effects on F. candida reproduction. We generally hypothesized that toxicity should vary (a) between NM-300K and AgNO3, (b) depending on soil type, (c) with respect to exposure duration of NM-300K in soil and (d) after the application of NM-300K treated sewage sludge to the soil.
Materials and Methods
Chemicals
NM-300K was used for all studies. NM-300K is a sterically stabilized nanosilver dispersion containing nanoparticles smaller than 20 nm. It originates from a single batch of commercially manufactured representative nanosilver which is used for the measurement and testing for hazard identification, risk and exposure assessment studies by the Joint Research Center (Klein et al., 2011).
The NM-300K sample dispersion is yellow-brown; it is an aqueous dispersion of nanosilver containing 10.16% w/w Ag and the stabilizing agents polyoxyethylene glycerol trioleate and polyoxyethylene (20) sorbitan mono-laurat (Tween 20), each 4% w/w, which prevents particle aggregation because of sterical repulsion (Hoppe et al., 2015). A 2% w/w stock of solution in deionized water was prepared. Before preparing the solutions for the toxicity test with demineralized, filtered water the vial containing the stock was sonicated (Bandelin, Sonorex AK100H) for 15 min. The NM-300K sample dispersion as well as the stock solution were stored in the dark at room temperature.
AgNO3 powder (≥99.0% purity) for the salt control was purchased from Sigma-Aldrich (Munich, Germany) and immediately before application to the soils a solution with demineralized, filtered water was prepared.
Chemical Analyses
NM-300K characterization revealed silver particles of about 15 nm size, with 99% of the silver particles of a size below 20 nm (Klein et al., 2011). The average specific surface area of 38.1 m2 g−1 (Klein et al., 2011) was calculated from the average diameter of 15 nm and the density of 10.5 g cm−3 (Haynes, 2012). For TEM analyses of NM-300K refer to Klein et al. (2011). Köser et al. performed dynamic light scattering and zeta-potential measurements for the particle characterization in water: NM-300K particles in demineralized H2O (10 mg Ag L−1) exhibited an average hydrodynamic diameter of about 40 nm and zeta-potential of −15 mV (Köser et al., 2013).
The NM-300K dispersion was colloidally stable for 14 days with constant particle size distribution but an increase in zeta potential to −24 mV on day 14 (Köser et al., 2013).
The recovery of NM-300K and AgNO3 in RefeSol 01-A was determined using Atomic Absorption Spectrometry (AAS). The silver content of soil samples was quantified using aqua regia digestion (HCl:HNO3, 4:1, v:v) and subsequent measurement by graphite furnace atomic absorption spectrometry (GF-AAS) using an Unicam 989 QZ AA Spectrometer (Unicam, Cambridge, UK) with a GF-90 plus furnace and FS-90 plus autosampler. Approximately 1 g aliquots of the dried soil samples were digested in 20 mL digestion solution consisting of 12 mL conc. HCl, 3 mL conc. HNO3 and 5 mL double distilled water (ddH2O) at 65°C for 2 h. In case the silver content was higher than the range of the silver AAS method (0.5–20 μg Ag/L) the digestion solution was diluted prior AAS measurement using diluted aqua regia (100 mL consisting of 11 mL conc. HNO3, 44 mL conc. HCl and approximately 45 mL ddH2O). The average recovery for AgNO3 was 99.5 ± 0.5% (2 preparations, 10 samples each) and 81.4 ± 9.5% for NM-300K (5 preparations, 10 samples each). For reasons of consistency nominal concentrations will be used in the following.
A pore water extraction from 5 g of OECD soil spiked with 0.6, 15, and 375 μg Ag kg−1 was performed by vacuum extraction at 300 mbar (membrane pump VCZ 324, IMLVAG GmbH, Ilmenau, Germany). The soil was exposed either to NM-300K or AgNO3 for 28 days before the extraction. For the extraction the water content was adjusted to the total pore volume of soil packed in rings for 24 h and the pore water was then extracted from the packed soil matrix over a 0.45 μm filter (SteriFlip-HV, Merck Millipore, Darmstadt, Germany) for 5 min. The effluent was analyzed for silver by GF-AAS (Unicam 989 QZ) with a detection limit of 0.9 μg Ag L−1.
Soil Types and Characterization
OECD soil is an artificial soil used for standardized testing of chemicals in the Collembola reproduction test (Organisation for Economic Co-operation Development, 2009). It is composed of 74% sand, 20% kaolin clay, 5% peat, and CaCO3 to adjust the pH (Table 1). RefeSol 01-A and LUFA 2.2 are commonly occurring soil types from selected areas arable land in Germany. These standard soils are especially recommended for soil biological tests, because they are sufficiently characterized and validated and the variability in soil properties is reflected. The soils are normally sampled from 0 to 20 cm depth, prepared and sieved to 2 mm. The total carbon content, organic carbon content, CaCO3 and pH of the OECD soil and RefeSol 01-A were measured in 100 g subsamples. The electric conductivity, soil texture, cation exchange capacity of the artificial OECD were also determined (Table 1). The corresponding data for RefeSol 01-A and LUFA 2.2 were provided by the Fraunhofer Institute IME and LUFA-Speyer, if available (Table 1).
Soil texture was determined via combined pipette and sieving method after removal of organic matter and aggregate dispersion. The total carbon content was determined via elemental analyzer (Euro EA, Hekatech) and to measure the CaCO3 content the DIN ISO 10693.5 was applied. From these two measurements the organic carbon content was calculated. The cation exchange capacity was determined by preparing an extraction solution that was centrifuged and filtered and the cations in the supernatant were measured by atomic absorption spectroscopy (AAS 5100, Perkin Elmer).
Collembola Reproduction Test
The F. candida culture was originally obtained from the working group of Prof. Achazi at the Freie Universität Berlin in the early 1990s. The miniaturized form (Filser et al., 2014) of the OECD reproduction test with F. candida (Organisation for Economic Co-operation Development, 2009) was used in all experiments of this study. Four 9 to 12 day old Collembola were introduced to 10 g of dry soil. In all tests each treatment was replicated five times and the test ran under standardized conditions (20°C, 12:12 light: dark) for 28 days. Once a week the moisture content was checked gravimetrically and adjusted to 50% WHC max if needed. At the end of the test a floatation with 100 mL water and a few drops of black ink per test vessel was performed and pictures were taken (Canon EOS 600D). Juveniles and adults were counted with help of the program ImageJ (National Institute of Health United States of America, 2015) and the cell counter plugin. The results are given as mean value and standard deviation, when the same test was repeated several times the standard error was calculated.
In all the experiments described in the following the same type of Collembola reproduction test was performed. The differences between these experiments lay in soil, concentrations and application form of NM-300K (described below in pristine and aged experiments) and are summarized in Table 2.
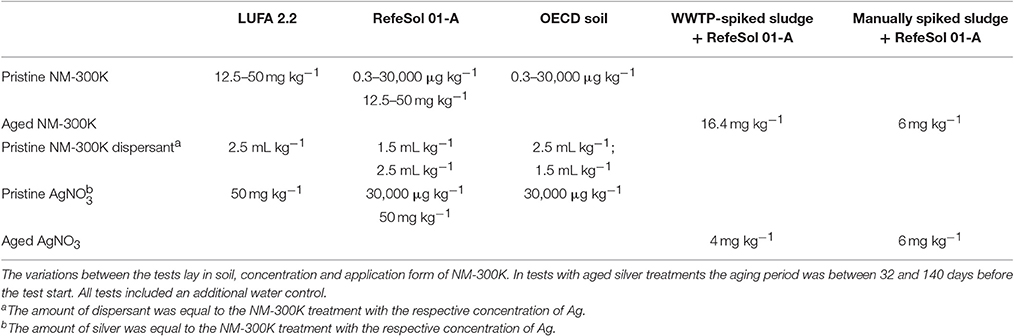
Table 2. Summary of the treatments applied in 28- day Collembola reproduction tests (50% WHC max, 20°C, n = 5).
Pristine NM-300K Experiments
Pristine NM-300K were added to different soils in various concentrations, the basic set up of the reproduction tests was however the same. Both experiments included a negative control with demineralized water. The stock solutions with NM-300K and AgNO3 were always freshly prepared with demineralized, filtered water before being thoroughly mixed in the soil to ensure homogenous distribution.
a) Concentration range of 0.3 to 30,000 μg Ag kg−1 in OECD soil and RefeSol 01-A
Two types of soils were used: OECD soil and RefeSol 01-A. 0.3, 3, 30, 300, 3,000, and 30,000 μg Ag kg−1 dry soil in form of NM-300K; 30,000 μg Ag kg−1 in form of AgNO3 and a dispersant control (NM-300K dispersant) were added to each soil. The dispersant control included the same amount of dispersant as the highest NM-300K treatment. The pH of the soil was measured (Jürgens, WTW, Weiheim, Germany) at the start and the end of the experiments.
b) Concentration range of 12.5–50 mg Ag kg−1 in RefeSol 01-A and LUFA 2.2
This experiment was conducted with RefeSol 01-A and LUFA 2.2 soils. The uncontaminated soils were sieved (2 mm), aliquoted to 15 g in 50 mL disposable centrifuge tubes, autoclaved at 120°C and stored at room temperature before use. 12.5, 25, and 50 mg Ag kg−1 in the form of NM-300K and 50 mg Ag kg−1 in the ionic form of AgNO3 were applied to the soils. NM-300K dispersant at the same concentration as in the highest NM-300K concentration served as a dispersant control. The test with each soil was repeated twice.
Aged NM-300K Experiments
The long-term effects of NM-300K and silver nitrate added to RefeSol 01-A via sewage sludge was studied on F. candida. The treated soil samples were prepared and stored at the Fraunhofer Institute IME. The soil had been mixed with either (a) manually spiked sludge or (b) after NP-dosage in a sewage treatment plant simulation. Both procedures for the incorporation of NM-300K and silver nitrate into the sewage sludge are described in detail by Schlich et al. (2013b). This is briefly summarized below.
a) Manually spiked sludge
Sewage sludge was freshly gathered from the municipal sewage treatment plant of Schmallenberg, Germany. NM-300K and silver nitrate were added to the sludge under aeration and stirring. The procedure was divided into three steps to avoid the inhibition of sludge microorganisms. The sludge was added to RefeSol 01-A in accordance with the German sewage sludge ordinance, which states that 5 tons per hectare over 3 years can be deployed onto agricultural areas (German Federal Minstry of Justice and Comsumer Protection)1. A silver concentration of 6.0 mg Ag kg−1 dry soil was intended for both NM-300K and AgNO3, which corresponds roughly to 3,500 mg kg−1 dry matter sludge.
b) NP-dosage via sewage treatment plant simulation (WWTP-spiked sludge)
Simulated sewage treatment plants were conducted according to the OECD Guideline 303A (Organisation for Economic Co-operation Development, 2001). One negative control, NM-300K (1.6 mg L−1) and silver nitrate (0.4 mg L−1) were dosed into the denitrification section of the WWTP continuously over 10 days. The influent concentrations led to a final silver concentrations in the sludge of about 9.6 mg Ag kg−1 dry sludge according to Schlich et al. (2013b). The sewage sludge was homogenously mixed into RefeSol 01-A. For the NM-300K-dosage via sewage treatment plant simulation the targeted concentrations were approximately 16.0 mg Ag kg−1 dry soil for NM-300K and 4.0 mg Ag kg−1 dry soil for silver nitrate.
In both experiments with aged NM-300K the soil was mixed well and stored in 20-L polyethylene containers at 20°C. Collembola tests were performed 32, 60, 100, and 140 days after NM-300K application via sewage sludge. The test at 32 days was only performed for the manually spiked sludge because the test system with WWTP-spiked sludge was unintentionally disturbed.
Statistical Analysis
The program R version 3.1.2 (R Core Team, 2014) was used to analyses the data statistically. The number of juveniles per adult per treatment were tested for normal error distribution with Shapiro-Wilk tests (p > 0.05) and if necessary transformations (square root, log,) were applied. Then a one-way ANOVA (p < 0.05) was run and a post-hoc Dunnett-test (p < 0.05) was performed to compare each treatment to the respective control.
When comparing the effects of one treatment in two soils Student t-tests (p < 0.05) were applied.
If a normal error distribution was not achieved through transformation a non-parametric Wilcoxon test (p < 0.05) was performed.
In addition to the above described statistical analyses a post-hoc Dunnett test (p < 0.05) was performed to compare each treatment to the highest NM-300K concentration in RefeSol 01-A. Also Student-t-tests were done to compare the number of juveniles per adult in the same treatment but different soils.
Results
All results shown in the following meet the OECD guideline validity criteria (Organisation for Economic Co-operation Development, 2009). Adult Collembola survival was not significantly affected in any of the tested conditions, therefore only the reproduction data is shown.
Pristine NM-300K Experiments
In the pore water extraction only in the sample treated with 375 μg Ag kg−1 1 μg Ag L−1 was detected (Supplementary Material).
a) Concentration range of 0.3 to 30,000 μg Ag kg−1 in OECD soil and RefeSol 01-A
The pH of the test soils did not differ significantly between treatments and remained stable during the course of the experiment (Supplementary Material). In OECD soil no significant effect on the reproduction of F. candida in any of the tested treatments was observed (Figure 1). In RefeSol 01-A the treatment had a significant influence on the number of juveniles per adult only at the highest concentration (ANOVA p < 0.01), but no dose-response relationship was detected. No significant effect of NM-300K dispersant or AgNO3 on the reproduction was found in either soil (Figure 1). The reproductive output differs significantly between OECD soil and RefeSol 01-A in the highest NM-300K treatment. The results indicate that NM-300K and AgNO3 inhibit F. candida reproduction at concentrations in the mg kg−1 range, but not at lower concentrations (μg kg−1 range). Since no effects were observed in OECD soil after application of AgNP in the μg range, the Collembola reproduction test was repeated in two well characterized agricultural soils in the low mg concentration range.
b) Concentration range of 12.5–50 mg Ag kg−1 in RefeSol 01-A and LUFA 2.2
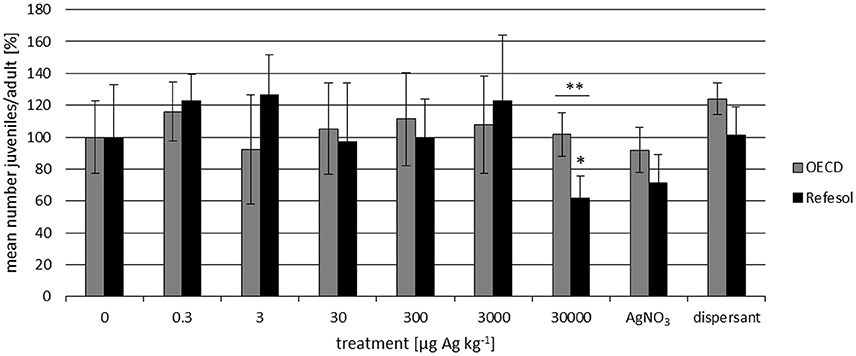
Figure 1. Number of F. candida juveniles per adult normalized to the control (mean ± SD) after a 28-day reproduction test in OECD soil and RefeSol. The nominal concentrations refer to NM-300K in μg Ag kg−1 and the AgNO3 treatment contained the same amount of silver as the 30,000 μg Ag kg−1 NM-300K treatment. The dispersant control contained the same amount of dispersant as the highest NM-300K treatment. Statistically significant difference between the two soils: **p ≤ 0.01. Significant difference between the treatment and the respective control: *p ≤ 0.05.
In both RefeSol 01-A and LUFA 2.2 the presence of AgNO3 and NM-300K at concentrations from 12.5 to 50 mg Ag kg−1 dry soil induced a decrease in reproduction to 60–70% of the untreated control, but again no concentration-effect relationships were found. No significant effect of NM-300K dispersant on the reproduction in LUFA 2.2 and RefeSol 01-A was observed. Comparable results were obtained for both soils (Figure 2).
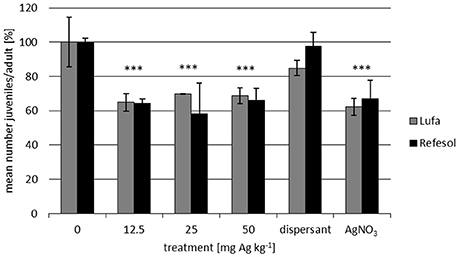
Figure 2. Number of F. candida juveniles per adult normalized to the control (mean ± SE) after 28-day reproduction tests in LUFA 2.2 and RefeSol 01-A. The test was repeated twice and was performed with 5 replicates each. The nominal concentrations refer to mg Ag kg−1 and the AgNO3 treatment contained the same amount of silver as 50 mg Ag kg−1 NM-300K treatment. The dispersant control contained as much dispersant as the highest NM-300K treatment. Statistical significances to the respective control: ***p ≤ 0.001.
Aged NM-300K Experiments
Comparing the controls with and without sludge revealed small but not time related variability (data not shown). Hence, the data obtained for NM-300K and AgNO3 performed with both manually spiked sludge or WWTP-spiked sludge were always related to the sludge control (without Ag). Significant inhibition of the F. candida reproduction due to NM-300K and AgNO3 was observed for both WWTP and manually spiked sludge (Figures 3, 4). Toxicity was similar in the treatments aged for 60, 100, and 140 days in the WWTP-spiked sludge (Figure 3). An increase in inhibition with incubation time was evident for manually spiked sludge (Figure 4). A distinct time dependence was not observed for AgNO3-spiked sludge (Figure 4).
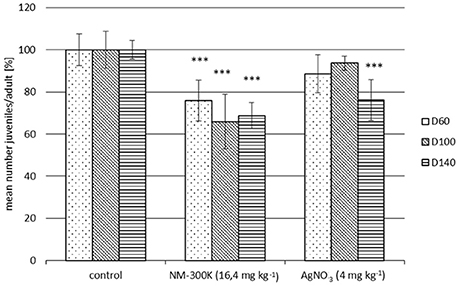
Figure 3. Inhibition of F. candida reproduction in a 28-day test with WWTP-spiked sludge compared to the corresponding sludge control. The sewage sludge was spiked with NM-300K or AgNO3 which were incorporated into the sludge through a simulated waste water treatment plant. The sewage sludge was applied to soil and incubated for 60, 100, or 140 days before the Collembola reproduction tests were performed. Reproduction per adult is normalized to the corresponding sludge control (100%). Each column represents mean ± SD of 5 replicates. Statistical significances to the respective control: ***p ≤ 0.001.
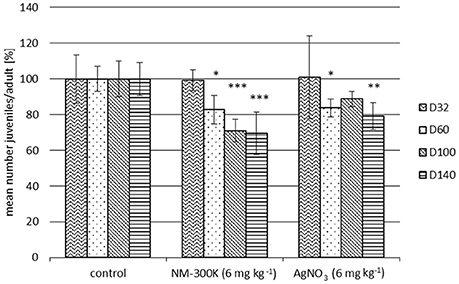
Figure 4. Inhibition of F. candida reproduction in a 28-day test with manually spiked sludge compared to the corresponding sludge control. NM-300K or AgNO3 were applied to the sewage sludge by manual spiking. The sewage sludge was applied to soil and incubated for 32, 60, 100, or 140 days before the tests were performed. Reproduction per adult is normalized to the corresponding sludge control (100%). Each column represents mean ± SD of 5 replicates. Statistical significances to the respective control: *p ≤ 0.05, **p ≤ 0.01, ***p ≤ 0.001.
Discussion
Pristine NM-300K Experiments
a) Concentration range of 0.3 to 30,000 μg Ag kg−1 in OECD soil and RefeSol 01-A
We detected a significant inhibition of F. candida reproduction in Refesol 01-A at 30,000 μg kg−1 while in OECD soil no toxicity was found within the tested concentration range (Figure 1).
The difference in observed effects in OECD and RefeSol 01-A may be caused by the properties of the soils, which play an important role in the speciation of the AgNP, as shown by Shoults-Wilson et al. (2011a). For example, the oxidation rate of AgNP depends on the soil type (Shoults-Wilson et al., 2011a,b). In soils with low organic matter content and acidic soils Ag ions are more available for biological uptake (Shoults-Wilson et al., 2011a; Cornelis et al., 2012; Benoit et al., 2013). The uptake of Ag by the earthworm E. fetida from natural sandy loam soil spiked with PVP-coated AgNP is significantly higher than from an artificial soil (70% sand, 20% kaolin clay, 10% peat). This natural soil has a low cation exchange capacity, organic matter content, clay content and pH (Shoults-Wilson et al., 2011a). OECD soil has a pH of 6.53 and contains five times more organic matter and a three times higher clay content than RefeSol 01-A soil (Table 1). These soil characteristics might result in less free Ag ions in the soil pore water and less bioavailable Ag in OECD soil.
This is in line with our results from the pore water extraction in OECD soil where silver was only detected in a marginal amount in the treatment with 375 μg Ag kg−1. This indicates that most of the silver is bound to particles in OECD soil and is not bioavailable in the pore water. These concentrations of silver are lower than those detected by Waalewijn-Kool et al. (2014) who recovered about 1.5% Ag in the pore water of LUFA 2.2 soil spiked with 168 mg Ag kg−1 paraffin-coated AgNP and 30–60 mg Ag kg−1 AgNO3. Column leaching experiments revealed that only a small amount of Ag was released from RefeSol 01-A after incubation with NM-300K for 3 days (Hoppe et al., 2015). The detected Ag release corresponded to ≤ 1% of the total amount of Ag in the soil column (Hoppe et al., 2015). These results suggest that NM-300K are retained in this soil. Retained AgNP might release considerable amounts of toxic Ag ions into the soils. However, even if released to soils, relatively large amounts of Ag ions may be readily sorbed in the soils (Hoppe et al., 2015). It is also known that Ag is sulfidized in soil reducing the bioavailable fraction of silver (Levard et al., 2012).
b) Concentration range of 12.5–50 mg Ag kg−1 in RefeSol 01-A and LUFA 2.2
The similar significant inhibition of F. candida reproduction in both RefeSol 01-A and LUFA 2.2 is probably due to similar pH values and content of clay and organic matter in these soils (Figure 2).
The decrease in reproduction irrespective of the applied concentration of NM-300K or AgNO3 may be related to the strong adsorption to soil and complexation with organic matter. This results in a marginal increase of free Ag ions in solution with increasing nominal AgNP or AgNO3 quantity. The high percentage of soil adsorption and complexation by dissolved organic matter of added AgNP was also reported by Hoppe et al. (2015) who investigated the sorption of NM-300K and silver ions in 25 German arable soils with varying properties in 24 h batch retention experiments. The adsorption of Ag ions was consistent with the Freundlich model with high KF values, which suggested a high retention of Ag ions. The Freundlich model is an adsorption isotherm. It is a graphical representation of the relationship between the amount adsorbed by a unit weight of adsorbent (e.g., soil) and the amount of adsorbate remaining in the medium at equilibrium. It maps the distribution of adsorbable solute between the liquid and solid phases at various equilibrium concentrations. The adsorption isotherm is based on data that are specific for each system, and the isotherm must be determined for every application. KF is the empirically obtained constant.
Research on the partitioning of silver and chemical speciation of free Ag in agricultural soils amended with polyacrylate stabilized AgNP (Benoit et al., 2013) has revealed that most of the free Ag is adsorbed onto the mineral surface of the soil straight after application of AgNP as well as AgNO3. Soil and water were mixed in a 1:2 ratio and Ag was measured in both compartments. Measurements of Ag and total solution AgNP following the addition of AgNP and AgNO3 in soil showed that <1% of the applied silver was found in solution while most is bound to the soil compartment and hence, is not bioavailable. Addition of AgNP or AgNO3 in the range of 1–100 mg Ag kg−1 soil always revealed saturation curves when the Ag in solution was plotted vs. Ag concentration measured in the soil (Benoit et al., 2013). Hence, we hypothesize that also in the present study further increase in the nominal NM-300K concentrations resulted only in marginal increase of Ag ions and NM-300K in solution and their bioavailability remained constant in the concentration range investigated. In consequence, the effective Ag ions or NM-300K concentration remained constant, leading to an inhibition of the collembolan reproduction above a certain threshold, irrespective of the applied Ag concentration. Concentration dependent toxic effects may be detected at NM-300K concentrations which exceed the sorption capacity of the soil.
Aged NM-300K Experiments
When simulating a realistic exposure pathway by applying NM-300K and AgNO3 via sewage sludge the reproduction of F. candida was significantly reduced. This effect appeared after 60 days of aging (Figures 3, 4).
Benoit et al. (2013) showed that the addition of 25–100 mg Ag kg−1 dry soil, as either AgNO3 or AgNP, resulted in similar concentrations of free Ag ions in solution after about a month of equilibration. They speculate that Ag was rapidly bound to the available ligands in the soil solution or to soil surfaces during the first hours of exposure. Subsequently, as the system equilibrates, Ag dissociation from the sorption sites may have resulted in higher free Ag ions. Hence, in our experiments the increase in toxicity on F. candida reproduction with NM-300K aging in the soil is likely driven by Ag dissociation as well.
It has been shown in various studies that AgNP is partially transformed to silver sulfide when passing through WWTP (Kaegi, 2011; Lombi et al., 2013). This can reduce the bioavailable fraction of silver in sewage sludge and the toxicity of AgNP in short-term studies (Levard et al., 2012). In our studies with long-term aged NM-300K in sewage sludge we however detected an increase in toxicity with aging.
The influence of soil organic matter content on the binding strength of AgNO3, electrostatically (citrate) stabilized and uncoated AgNP has been investigated by Coutris et al. (2012). In their experiments soil samples were spiked with the different silver forms and left to age for 2 h, 2 days, 5 or 10 weeks before they were submitted to sequential extraction. All types of silver were more mobile in the mineral soil than in the soil rich in organic matter, although the fractionation patterns were very different for the three silver forms in both soils. In contrast to AgNO3 and citrate stabilized AgNP, 80% of the uncoated sterically stabilized AgNP was not extractable at all. The results also showed that in contrast to soils treated with AgNO3 and citrate stabilized AgNP, the bioaccessible fraction of silver from uncoated AgNP increased over time, and by day 70 was between 8 and 9 times greater than that seen in the other two treatments. These findings demonstrate that uncoated AgNP can act as a continuous source of bioaccessible Ag ions (Coutris et al., 2012). These results were confirmed in the present study by the time dependent increase in inhibition of collembolan reproduction (Figures 3, 4).
Our results also correspond to the findings of Schlich et al. (2013b): at environmentally relevant concentrations, NM-300K adsorption to sludge and aging in soil has a toxic effect on soil microorganisms. Other authors have also seen a rise in toxicity of AgNP to soil invertebrates with time when aged in soil. van der Ploeg et al. (2014) detected lower juvenile hatchability and survival of Lumbricus rubellus for NM-300K than AgNO3 at 15 mg Ag kg−1 in a long-term study. They suspect that NM-300K have longer lasting toxic effects, because of Ag ion dissolution, than AgNO3 which on the short-term is more toxic (van der Ploeg et al., 2014). For Eisenia fetida toxicity of uncoated AgNP also increased with aging time and the authors suspect the same mechanism behind this (Diez-Ortiz et al., 2015).
Conclusions
Our study shows that the presence AgNP in the concentration range of 12.5–50 mg Ag kg−1 soil results in significant, but concentration independent inhibition of F. candida reproduction in RefeSol 01-A and LUFA 2.2., but not in the OECD soil. Hence, observed effects vary depending on soil type.
Significant inhibition of F. candida reproduction due to AgNP was also observed for soil amended with AgNP treated sludge. An increase in inhibition with aging of the AgNP in the soil was evident, and indicates that toxic effects depend on exposure duration of NM-300K in soil.
In conclusion, our results demonstrate that, at environmentally relevant concentrations, AgNP adsorption to sludge and subsequent aging in soil lead to toxic effects on soil invertebrates at low concentration. The consideration of transformations and the implementation of aging tests when performing environmental risk assessment of AgNP (and other nanoparticles) are essential.
Author Contributions
The funding was secured by JF. MM, ME, XZ, and JF designed the toxicological tests which were performed and analyzed by MM, ME, XZ, and EL. JK designed, conducted and analyzed the data of the chemical analyses. The soil characterization performed by TE and the pore water extraction was planned and executed by MM and TE. The paper was written by MM and ME and all other authors reviewed the manuscript.
Conflict of Interest Statement
The authors declare that the research was conducted in the absence of any commercial or financial relationships that could be construed as a potential conflict of interest.
Acknowledgments
The pristine NM-300K studies with concentrations of 12.5–50 mg Ag kg−1 and the studies with aged NM-300K were supported by the German Federal Ministry for Education and Research (project BMBF 03X0091).
Supplementary Material
The Supplementary Material for this article can be found online at: http://journal.frontiersin.org/article/10.3389/fenvs.2017.00019/full#supplementary-material
Footnotes
1. ^German Federal Minstry of Justice and Comsumer Protection German Sewage Sludge Regulation. Available online at: http://www.gesetze-im-internet.de/abfkl_rv_1992/ (Accessed July 25, 2016).
References
Batley, G. E., Kriby, J. K., and McLaughlin, M. J. (2013). Fate and risks of nanomaterials in aquatic and terrestrial environments. Acc. Chem. Res. 46, 854–862. doi: 10.1021/ar2003368
Benn, T. M., and Westerhoff, P. (2008). Nanoparticle silver released into water from commercially available sock fabrics. Environ. Sci. Technol. 42, 4133–4139. doi: 10.1021/es7032718
Benoit, R., Wilkinson, K. J., and Sauvé, S. (2013). Partitioning of silver and chemical speciation of free Ag in soils amended with nanoparticles. Chem. Cent. J. 7:75. doi: 10.1186/1752-153x-7-75
Bicho, R. C., Ribeiro, T., Rodrigues, N. P., Scott-Fordsmand, J. J., and Amorim, M. J. (2016). Effects of Ag nanomaterials (NM300K) and Ag salt (AgNO3) can be discriminated in a full life cycle long term test with Enchytraeus crypticus. J. Hazard. Mater. 318, 608–614. doi: 10.1016/j.jhazmat.2016.07.040
Cornelis, G., Thomas, C. D. M., McLaughlin, M. J., Kirby, J. K., Beak, D. G., and Chittleborough, D. (2012). Retention and dissolution of engineered silver nanoparticles in natural soils. Soil Sci. Soc. Am. J. 76, 891–902. doi: 10.2136/sssaj2011.0360
Coutris, C., Joner, E. J., and Oughton, D. H. (2012). Aging and soil organic matter content affect the fate of silver nanoparticles in soil. Sci. Total Environ. 420, 327–333. doi: 10.1016/j.scitotenv.2012.01.027
de Moura, M. R., Mattoso, L. H. C., and Zucolotto, V. (2012). Development of cellulose-based bactericidal nanocomposites containing silver nanoparticles and their use as active food packaging. J. Food Eng. 109, 520–524. doi: 10.1016/j.jfoodeng.2011.10.030
Diez-Ortiz, M., Lahive, E., George, S., Ter Schure, A., Van Gestel, C. A. M., Jurkschat, K., et al. (2015). Short-term soil bioassays may not reveal the full toxicity potential for nanomaterials; Bioavailability and toxicity of silver ions (AgNO3) and silver nanoparticles to earthworm Eisenia fetida in long-term aged soils. Environ. Pollut. 203, 191–198. doi: 10.1016/j.envpol.2015.03.033
Engelke, M., Köser, J., Hackmann, S., Zhang, H., Mädler, L., and Filser, J. (2014). A miniaturized solid contact test with Arthrobacter globiformis for the assessment of the environmental impact of silver nanoparticles. Environ. Toxicol. Chem. 33, 1142–1147. doi: 10.1002/etc.2542
Fabrega, J., Luoma, S. N., Tyler, C. R., Galloway, T. S., and Lead, J. R. (2011). Silver nanoparticles: behaviour and effects in the aquatic environment. Environ. Int. 37, 517–531. doi: 10.1016/j.envint.2010.10.012
Feng, Y., Cui, X., He, S., Dong, G., Chen, M., Wang, J., et al. (2013). The role of metal nanoparticles in influencing arbuscular mycorrhizal fungi effects on plant growth. Environ. Sci. Technol. 47, 9496–9504. doi: 10.1021/es402109n
Filser, J. (2002). The role of Collembola in carbon and nitrogen cycling in soil. Pedobiologia 46, 234–245. doi: 10.1078/0031-4056-00130
Filser, J., Wiegmann, S., and Schröder, B. (2014). Collembola in ecotoxicology-Any news or just boring routine? Appl. Soil Ecol. 83, 193–199. doi: 10.1016/j.apsoil.2013.07.007
Gomes, S. I., Scott-Fordsmand, J. J., and Amorim, M. J. (2015). Cellular energy allocation to assess the impact of nanomaterials on soil invertebrates (Enchytraeids): the effect of Cu and Ag. Int. J. Environ. Res. Public Health 12, 6858–6878. doi: 10.3390/ijerph120606858
Gomes, S. I., Soares, A. M., Scott-Fordsmand, J. J., and Amorim, M. J. (2013). Mechanisms of response to silver nanoparticles on Enchytraeus albidus (Oligochaeta): survival, reproduction and gene expression profile. J. Hazard. Mater. 254–255, 336–344. doi: 10.1016/j.jhazmat.2013.04.005
Gottschalk, F., Sonderer, T., Scholz, R. W., and Nowack, B. (2009). Modeled environmental concentrations of engineered nanomaterials (TiO2, ZnO, Ag, CNT, Fullerenes) for different regions. Environ. Sci. Technol43, 9216–9222. doi: 10.1021/es9015553
Handy, R. D., Cornelis, G., Fernandes, T., Tsyusko, O., Decho, A., Sabo-Attwood, T., et al. (2012). Ecotoxicity test methods for engineered nanomaterials: practical experiences and recommendations from the bench. Environ. Toxicol. Chem. 31, 15–31. doi: 10.1002/etc.706
Hänsch, M., and Emmerling, C. (2010). Effects of silver nanoparticles on the microbiota and enzyme activity in soil. J. Plant Nutr. Soil Sci. 173, 554–558. doi: 10.1002/jpln.200900358
Heckmann, L. H., Hovgaard, M. B., Sutherland, D. S., Autrup, H., Besenbacher, F., and Scott-Fordsmand, J. J. (2011). Limit-test toxicity screening of selected inorganic nanoparticles to the earthworm Eisenia fetida. Ecotoxicology 20, 226–233. doi: 10.1007/s10646-010-0574-0
Hoppe, M., Mikutta, R., Utermann, J., Duijnisveld, W., and Guggenberger, G. (2015). Remobilization of sterically stabilized silver nanoparticles from farmland soils determined by column leaching. Eur. J. Soil Sci. 66, 898–909. doi: 10.1111/ejss.12270
Kaegi, R. (2011). Behavior of silver nanoparticles in a pilot wastewater treatment plant SI. Environ. Sci. Technol. 45, 9113–9114. doi: 10.1021/es1041892
Keller, A. A., and Lazareva, A. (2014). Predicted releases of engineered nanomaterials: from global to regional to local. Environ. Sci. Technol. Lett. 1, 65–70. doi: 10.1021/ez400106t
Kim, B., Park, C. S., Murayama, M., and Hochella, M. F. (2010). Discovery and characterization of silver sulfide nanoparticles in final sewage sludge products. Environ. Sci. Technol. 44, 7509–7514. doi: 10.1021/es101565j
Kjølholt, J., Gottschalk, F., Brinch, A., Lützhøft, H.-C. H., Hartmann, N. B., Nowack, B., et al. (2015). Environmental Assessment of Nanomaterial Use in Denmark. Copenhagen: The Danish Environmental Protection Agency.
Klein, C., Comero, S., Stahlmecke, B., Romazanov, J., Kuhlbusch, T., Van Doren, E., et al. (2011). NM-Series of Representative Manufactured Nanomaterials: NM-300 Silver Characterisation, Stability, Homogeneity. Luxembourg; Publications Office of the European Union.
Köser, J., Engelke, M., Kück, A., Lesnikov, E., Arning, J., Thöming, J., et al. (2013). Abschätzung der Umweltgefährdung durch Silber-Nanomaterialien: vom chemischen Partikel zum technischen Produkt, UMSICHT. Abschlussbericht, 34.
Levard, C., Hotze, E. M., Lowry, G. V., and Brown, G. E. (2012). Environmental transformations of silver nanoparticles: impact on stability and toxicity. Environ. Sci. Technol. 46, 6900–6914. doi: 10.1021/es2037405
Lombi, E., Donner, E., Taheri, S., Tavakkoli, E., Jämting, Å. K., McClure, S., et al. (2013). Transformation of four silver/silver chloride nanoparticles during anaerobic treatment of wastewater and post-processing of sewage sludge. Environ. Pollut. 176, 193–197. doi: 10.1016/j.envpol.2013.01.029
Massarsky, A., Trudeau, V. L., and Moon, T. W. (2014). Predicting the environmental impact of nanosilver. Environ. Toxicol. Pharmacol. 38, 861–873. doi: 10.1016/j.etap.2014.10.006
McKee, M. S., and Filser, J. (2016). Impacts of metal-based engineered nanomaterials on soil communities. Environ. Sci. Nano 3, 506–533. doi: 10.1039/C6EN00007J
Mendes, L. A., Maria, V. L., Scott-Fordsmand, J. J., and Amorim, M. J. (2015). Ag nanoparticles (Ag NM300K) in the terrestrial environment: effects at population and cellular level in Folsomia candida (Collembola). Int. J. Environ. Res. Public Health 12, 12530–12542. doi: 10.3390/ijerph121012530
Meyer, J. N., Lord, C. A., Yang, X. Y., Turner, E. A., Badireddy, A. R., Marinakos, S. M., et al. (2010). Intracellular uptake and associated toxicity of silver nanoparticles in Caenorhabditis elegans. Aquat. Toxicol. 100, 140–150. doi: 10.1016/j.aquatox.2010.07.016
Mishra, S., and Singh, H. B. (2015). Biosynthesized silver nanoparticles as a nanoweapon against phytopathogens: exploring their scope and potential in agriculture. Appl. Microbiol. Biotechnol. 99, 1097–1107. doi: 10.1007/s00253-014-6296-0
National Institute of Health United States of America (2015). ImageJ. Available online at: http://rsb.info.nih.gov/ij/ (Accessed November 23, 2015).
Novo, M., Lahive, E., Díez-Ortiz, M., Matzke, M., Morgan, A. J., Spurgeon, D. J., et al. (2015). Different routes, same pathways: molecular mechanisms under silver ion and nanoparticle exposures in the soil sentinel Eisenia fetida. Environ. Pollut. 205, 385–393. doi: 10.1016/j.envpol.2015.07.010
Organisation for Economic Co-operation and Development (2001). OECD Guideline for the Testing of Chemicals 303. Simulation Test-Aerobic Sewage Treatment: 303 A: Activated Sludge Units-303 B: Biofilms. Paris: OECD.
Organisation for Economic Co-operation and Development (2009). OECD Guidelines for the testing of Chemicals 232. Collembolan Reproduction Test in Soil. Paris: OECD.
Rai, M., Yadav, A., and Gade, A. (2009). Silver nanoparticles as a new generation of antimicrobials. Biotechnol. Adv. 27, 76–83. doi: 10.1016/j.biotechadv.2008.09.002
R Core Team (2014). R: A Language and Environment for Statistical Computing. Vienna: R Foundation for Statistical Computing.
Ribeiro, M., Maria, V., Scott-Fordsmand, J., and Amorim, M. (2015). Oxidative sress mechanisms caused by Ag nanoparticles (NM300K) are different from those of AgNO3: effects in the soil invertebrate Enchytraeus crypticus. Int. J. Environ. Res. Public Health 12, 9589–9602. doi: 10.3390/ijerph120809589
Roh, J. Y., Sim, S. J., Yi, J., Park, K., Chung, K. H., Ryu, D. Y., et al. (2009). Ecotoxicity of silver nanoparticles on the soil nematode Caenorhabditis elegans using functional ecotoxicogenomics. Environ. Sci. Technol. 43, 3933–3940. doi: 10.1021/es803477u
Schlich, K., Klawonn, T., Terytze, K., and Hund-Rinke, K. (2013a). Effects of silver nanoparticles and silver nitrate in the earthworm reproduction test. Environ. Toxicol. Chem. 32, 181–188. doi: 10.1002/etc.2030
Schlich, K., Klawonn, T., Terytze, K., and Hund-Rinke, K. (2013b). Hazard assessment of a silver nanoparticle in soil applied via sewage sludge. Environ. Sci. Eur. 25:17. doi: 10.1186/2190-4715-25-17
Shoults-Wilson, W. A., Reinsch, B. C., Tsyusko, O. V., Bertsch, P. M., Lowry, G. V., and Unrine, J. M. (2011a). Role of particle size and soil type in toxicity of silver nanoparticles to earthworms. Soil Sci. Soc. Am. J. 75, 365–377. doi: 10.2136/sssaj2010.0127nps
Shoults-Wilson, W. A., Zhurbich, O. I., McNear, D. H., Tsyusko, O. V., Bertsch, P. M., and Unrine, J. M. (2011b). Evidence for avoidance of Ag nanoparticles by earthworms (Eisenia fetida). Ecotoxicology 20, 385–396. doi: 10.1007/s10646-010-0590-0
Simonin, M., Guyonnet, J. P., Martins, J. M. F., Ginot, M., and Richaume, A. (2015). Influence of soil properties on the toxicity of TiO2 nanoparticles on carbon mineralization and bacterial abundance. J. Hazard. Mater. 283, 529–535. doi: 10.1016/j.jhazmat.2014.10.004
Simonin, M., and Richaume, A. (2015). Impact of engineered nanoparticles on the activity, abundance, and diversity of soil microbial communities: a review. Environ. Sci. Pollut. Res. Int. 22, 13710–13723. doi: 10.1007/s11356-015-4171-x
Sun, T. Y., Gottschalk, F., Hungerbühler, K., and Nowack, B. (2014). Comprehensive probabilistic modelling of environmental emissions of engineered nanomaterials. Environ. Pollut. 185, 69–76. doi: 10.1016/j.envpol.2013.10.004
Tourinho, P. S., van Gestel, C. A., Lofts, S., Svendsen, C., Soares, A. M., and Loureiro, S. (2012). Metal-based nanoparticles in soil: fate, behavior, and effects on soil invertebrates. Environ. Toxicol. Chem. 31, 1679–1692. doi: 10.1002/etc.1880
van der Ploeg, M. J., Handy, R. D., Waalewijn-Kool, P. L., van den Berg, J. H., Herrera Rivera, Z. E., Bovenschen, J., et al. (2014). Effects of silver nanoparticles (NM-300K) on Lumbricus rubellus earthworms and particle characterization in relevant test matrices including soil. Environ. Toxicol. Chem. 33, 743–752. doi: 10.1002/etc.2487
Velicogna, J. R., Ritchie, E. E., Scroggins, R. P., and Princz, J. I. (2016). A comparison of the effects of silver nanoparticles and silver nitrate on a suite of soil dwelling organisms in two field soils. Nanotoxicology 10, 1144–1151. doi: 10.1080/17435390.2016.1181807
Keywords: silver nanoparticles, NM-300K, standard soil, Folsomia candida, aging, sewage sludge
Citation: McKee MS, Engelke M, Zhang X, Lesnikov E, Köser J, Eickhorst T and Filser J (2017) Collembola Reproduction Decreases with Aging of Silver Nanoparticles in a Sewage Sludge-Treated Soil. Front. Environ. Sci. 5:19. doi: 10.3389/fenvs.2017.00019
Received: 19 January 2017; Accepted: 24 April 2017;
Published: 10 May 2017.
Edited by:
Marcelo Bispo de Jesus, Universidade Estadual de Campinas, BrazilReviewed by:
Claudia Cascio, Bundesinstitut für Risikobewertung, GermanySara Cristina Antunes, University of Porto, Portugal
Copyright © 2017 McKee, Engelke, Zhang, Lesnikov, Köser, Eickhorst and Filser. This is an open-access article distributed under the terms of the Creative Commons Attribution License (CC BY). The use, distribution or reproduction in other forums is permitted, provided the original author(s) or licensor are credited and that the original publication in this journal is cited, in accordance with accepted academic practice. No use, distribution or reproduction is permitted which does not comply with these terms.
*Correspondence: Moira S. McKee, bW9pcmEubWNrZWVAdW5pLWJyZW1lbi5kZQ==