- 1Department of Ecology and Environmental Science, Umeå University, Umeå, Sweden
- 2Department of Chemistry, Umeå University, Umeå, Sweden
- 3Department of Environmental and Life Sciences, Karlstad University, Karlstad, Sweden
Behavioral assays constitute important research tools when assessing how fish respond to environmental change. However, it is unclear how behavioral modifications recorded in laboratory assays are expressed in natural ecosystems, a limitation that makes it difficult to evaluate the predictive power of laboratory-based measurements. In this study, we hypothesized that exposure to a benzodiazepine (i.e., oxazepam) increases boldness and activity in laboratory assays as well as in field assays—that is, laboratory results can be used to predict field results. Moreover, we expected the modified behavior to affect other important ecological measures such as habitat selection and home range. To test our hypothesis, we exposed European perch (Perca fluviatilis) to oxazepam and measured subsequent changes in behavioral trials both in laboratory assays and in a lake ecosystem populated with a predatory fish species, pike (Esox lucius). In the lake, the positions of both perch and pike were tracked every 3 min for a month using acoustic telemetry. In the laboratory assay, the oxazepam-exposed perch were bolder and more active than the non-exposed perch. In the lake assay, the oxazepam-exposed perch were also more bold and active, had a larger home range, and used pelagic habitats more than the non-exposed perch. We conclude that ecotoxicological behavioral assays are useful for predicting the effects of exposure in natural systems. However, although individual responses to exposure were similar in both the laboratory and field trials, effects were more obvious in the field study, mainly due to reduced variability in the behavioral measures from the lake. Hence, short-term behavioral assays may fail to detect all the effects expressed in natural environments. Nevertheless, our study clearly demonstrates that behavioral modifications observed in laboratory settings can be used to predict how fish perform in aquatic ecosystems.
Introduction
Laboratory behavioral assays where behavioral traits are recognized as sensitive endpoints for assessing how fish respond to changes in water chemistry are becoming important tools in modern ecotoxicology (Jones and Reynolds, 1997; Scott and Sloman, 2004; Melvin and Wilson, 2013; Brodin et al., 2014). Recent studies have shown that ecologically important behavioral traits, such as activity and boldness, are affected by various emerging aquatic contaminants (Reyhanian et al., 2011; Brodin et al., 2013; Dzieweczynski et al., 2016) as well as other contaminants including inorganic mercury (Pereira et al., 2016), persistent organic pollutants (Vignet et al., 2015), and mining waste leachate (Lanctôt et al., 2016). Effects on boldness and activity have also been noted in response to ongoing large-scale changes in carbon dioxide (CO2) concentrations in oceans and freshwaters (Munday et al., 2009, 2010; Welch et al., 2014; Regan et al., 2016). In other words, many laboratory assays imply that fish may change their activity and boldness in response to various environmental changes.
Although subtle behavioral modifications in laboratory studies may be used as early warning signs of potential effects in natural environments, the usefulness of results obtained in such assays for understanding effects in natural environments has been questioned for decades (Sprague, 1971). Therefore, large-scale field studies that verify contamination-induced behavioral modifications are needed (Atchison et al., 1987). Although behavioral traits observed in laboratory settings may be used to predict ecological and evolutionary consequences in natural settings (Réale et al., 2007), studies supporting this theory are largely based on short-term laboratory assays and field verifications are scarce (Conrad et al., 2011). In addition, some field studies using recapture of previously surveyed individuals have largely failed to verify results from laboratory assays, which raises questions about how findings from the laboratory are extrapolated to the field (Adriaenssens and Johnsson, 2011; Zavorka et al., 2015). However, whole-lake experiments using fish of different origins (domestic and wild) have shown that active and bold fish (domestic) occupy more risky habitats and grow faster, although these fish suffer from higher predation losses, findings that are in line with behavioral theory (Biro et al., 2004, 2006).
Increases in activity and boldness of fish exposed to common pharmaceutical contaminants (i.e., benzodiazepines) have also been linked to the compromise of cellular mechanisms—i.e., lowered action potential of the GABA-A receptor in the central nervous system—in fishes exposed to ocean acidification (Nilsson et al., 2012). Changes in water chemistry modulate the GABA system by lowering the action potential in the GABA-A receptor and this results in GABAergic behavioral modification (GBM). These behavioral modifications, as ecological theory predicts, increase predation risk and mortality (Munday et al., 2010; Brodin et al., 2013; Klaminder et al., 2014). Therefore, GBMs as the result of changes in water chemistry could severely decrease marine fish populations in the near future (Munday et al., 2010; Ou et al., 2015).
In this study, we examined to what extent GBMs in European perch (Perca fluviatilis) are expressed in natural environments by comparing results from laboratory assays with a large-scale field study. We used the benzodiazepine oxazepam to generate an inhibitory effect on GABA neurotransmission and quantified its effects on boldness and activity. We hypothesized that the increase in boldness and activity evident in fish exposed to oxazepam in laboratory assays also exists in oxazepam-exposed fish in the field, an implicit assumption in laboratory-based behavioral assays that has not been thoroughly empirically tested. Moreover, based on behavioral data from the field study, we quantified effects on important ecological measures such as fish habitat selection and home range. We quantified these effects because fish live in a “landscape of fear” that restricts their spatial use when predators are present (Laundre et al., 2010). Therefore, GBMs (i.e., increased activity and boldness) could change patterns in spatial use. The pharmacological-specific property of oxazepam enables us to quantify effects solely due to a GBM and excludes other effects that can arise as a result of changing water chemistry, such as pH effects on predator cues or olfactory receptor functioning (Hara, 1976; Havas and Rosseland, 1995), making our results highly relevant for the study of general environmental changes that modify behavior via this specific neurological pathway.
Methods
Exposure, Tagging of Fish, and Laboratory Behavioral Assays
Wild perch were caught using umbrella traps in Lake Brunnsjön (N 63° 74.6706′, E 20° 04.8761′), Lake Stöcksjön (N 63° 76.3976′, E 19° 76.78′), and Tavlefjärden Bay (N 63° 83.1283′, E 20° 49.5682′). In the laboratory, 3- and 4-year-old perch were held in brackish water (pH = 7.9, salinity = 3.3 psu, Temp = 13°C) and were fed chironomid larvae every second day. All fish were tagged with acoustic transmitters (Vemco V4 180 kHz) that later allowed for tracking the position of fish during the field study. Each acoustic transmitter was inserted into the abdominal cavity using a simple surgical procedure [for a review on tagging techniques used in acoustic telemetry see Liedtke and Rub (2012)]. This procedure takes less than 2 min per fish, during which the fish was sedated using MS-222. Every perch was given a minimum of 10 days to recover after surgery, and all fish began to eat normally well before the laboratory-based behavioral trials. To generate GBMs, a group of perch (N = 17; mean length = 112 mm; SD = 10) were prior to the behavior assay exposed to water containing nominal concentrations of 200 ug L−1 of dissolved oxazepam for 5 days. This concentration of oxazepam was used because it is within the range of concentrations previously seen to generate GBMs in perch (Brodin et al., 2013; Klaminder et al., 2014). That is, the exposure was chosen to guarantee an effect that did not induce any direct negative (toxic) effects. A control group (non-exposed fish) was also established (N = 17, mean length = 113 mm, SD = 13).
In the laboratory assay conducted 5 days after exposure, fish boldness and activity was measured in a 200-L aquarium with the bottom covered with gravel to mimic natural conditions (Figure S1). A single perch was released into a compartment one third the size of the aquarium. The compartment also had artificial submerged vegetation that served as shelter for the fish. A hatch, when opened, allowed the fish to explore the rest of the aquarium. After an acclimation time of 6 h, the behavioral experiment began by opening the hatch to give the fish access to the entire aquarium. Boldness was measured as the total time (1200 s) minus the time it took for an individual to leave the shelter and enter the unexplored area. Activity was measured as the total time (seconds) the fish swam in the unexplored area (i.e., the area accessible when the hatch was open). Boldness and activity were measured for both the controls (N = 17) and the exposed (N = 17) individuals. Each behavioral test was run twice for each fish to test for repeatability of the traits. Notably, two transmitters (from the exposed group) stopped functioning during the lab assay.
Lake Behavior
After the behavioral trials fish were returned to their individual aquaria for recovery and within 6 days, released into a natural lake (Lake Tosktjärn, N 64° 50.4178′, E 19° 04.3577′) following a 2 h transport in a 100-L oxygenated water tank. Lake Tosktjärn is a 1-ha large dystrophic (humic) lake with a mean depth of 5 m (max = 10 m) situated in a mature boreal forest close to the village of Ekorrträsk, Sweden (Figure S2). The lake is weakly acidic (pH = 6.2), containing about 19 mg L−1 of dissolved organic carbon, 17 μg L1 of phosphorous, and 609 μg L−1 of nitrogen. Before the experiment, the lake was fishless due to earlier rotenone treatments and as a consequence food was abundant both in the pelagic (daphnid zooplankton) and littoral zones (dominated by Ephemeroptera, Odonata, Chironomidae, and Asellus). Water temperature in the lake at 1-m depth during the experiment was 2.0 ± 0.5°C.
To generate a natural threat of predation and thus a landscape of risk and fear, pike (Esox lucius) caught in the Öre River (N 63° 52.3461′, E 19° 73.1274′) were released into the lake. The pike (N = 4) weighed between 2.2 and 4.4 kg (sufficient size to prey on all perch) and were transported in an oxygenated tank to the lake, where they were acoustically tagged in the same way as the perch. They were released into the lake on October 20 and 23. The density of pike generated in the lake (1 ha) was comparable with that of a typical boreal lake—i.e., 2–3 pike/ha (Persson et al., 1996). For this study, 14 trackable exposed perch and 12 trackable control fish were released into the lake on October 23 and these fish were used when comparing laboratory vs. field behavior. An additional release of six perch on November 11 was conducted with the intention to compensate for possible high predator losses, but as predation turned out to be low these fishes were not included in the study to avoid merging data sets with different temporal and conditional constraints. Because the lake was covered with a thin layer of ice (~1–2 cm), avian and terrestrial predators were unable to prey on the fish. Although snow-cover on ice can affect the behavior of fish (Blanchfield et al., 2009), this thin ice (< 2 cm) and limited snow accumulation (< 1 cm) during the experimental period meant that light was able to penetrate the water. Hence, fluctuations in light conditions during the study period was mainly induced by diurnal and seasonal changes and not by short-term snow events.
Fish behavior in Lake Tosktjärn was tracked using positioning based on 11 submerged omni-directional acoustic receivers (VEMCO VR2W 180 Hz) anchored to the lake bottom and kept approximately 50 cm above the bottom using buoys. A bathygraphic map illustrating the set-up of receivers and a brief description of Lake Tosktjärn is provided as supporting information (Figure S2). The position of the fish was triangulated, based on hyperbolic positioning theory, using the difference in the time of arrival of detected signals within a network of receivers. All fish were positioned in X and Y dimensions every 140 s for 32 days, with a positioning error typically less than 1 m for the pelagic zone and a positioning error ranging between 0.7 and 7 m for the littoral zone (Figure S3). Based on this positional data, we derived four behavioral variables: (1) boldness, (2) activity, (3) habitat preference, and (4) home-range size. Boldness was defined as the average distance a perch moved away from the release site during a 9-day period. The assumption is that bolder fish would move farther from the release site than shy fish. The distance a perch traveled between each position (i.e., during the 140 s) was used as a field measure of activity. Here the assumption is that more active individuals would move greater total distances compared to less active individuals.
Habitat preference was defined as the proportion of time that a perch spend in the pelagic or in the littoral zone based on hourly mean values. We assumed that the pelagic zone constituted a habitat involving more perceived risk as this habitat offered no vegetation for hiding and made fishes more exposed for predation attacks from both above and below. This assumption was supported by observations in our laboratory assays where perch preferred the vegetated part of the tank and largely avoided the open water until exposure to anxiolytics. Pelagic habitats have also been viewed as more risky habitats in other studies due to the risk generated by avian predators (Biro et al., 2004, 2006). Notable, our separation of habitats is based on perceived risk and, thus, may differ from the apparent risk of predation as avian predation was not present. The littoral zone was defined as the lake area within 10 m of the shoreline. Fifty percent core home range for each perch was calculated using a kernel-based Brownian bridge method (Horne et al., 2007), which takes into account the time dependence as well as the animal travel path between successive relocations, allowing for a more dynamic representation of animal spatial use compared to classic Kernel-based methods. Home range size was calculated every 6 h for each fish. Six hours allowed for enough relocations for reliable kernel estimates. Predation was detected by prolonged overlapping positions of pike and one perch (Figure S4). Here, positional data was scanned for inter-dependency in the movement by measuring the dynamic interaction pattern derived using proximity analysis (Bertrand et al., 1996). All data processing and analyses were done using the statistical program R (Team, 2015). All handling and exposure of fish was approved by the Umeå Ethical Committee on Animal Research (application DNR A56-14).
Uptake and Depuration in Perch
When fish enter clean water, oxazepam levels in their tissues start to depurate, eventually removing any effects of the drug. Previous studies concluded that the half-life of oxazepam in perch is around 2.5 days at a water temperature of 21°C (Heynen et al., 2016). However, depuration rates could be temperature dependent. Therefore, to assess uptake and depuration of oxazepam as a function of time in colder (5°C) water and thereby providing a more representative view of the expected therapeutic legacy in the fish released into Lake Tosktjärn, we performed a separate experiment. Perch (N = 52) were exposed to a measured concentration of 1.5 ± 0.1 μg L−1 oxazepam in circular, black aerated tanks (ϕ = 55 cm, 33 cm H) containing 50 L of aged tap water having a temperature of 5°C. We chose a lower concentration than that used in the experiment because the focus was on determining the depuration rates (half-life) rather than simulating absolute concentrations in the lake. Subsets of 3 to 4 perch were anesthetized with MS222 and sampled for muscle tissue after 4 h and after 1, 2, 3, 5, 9, and 14 days of exposure. Muscle tissue samples were stored frozen until analysis. The remaining individuals were transferred to clean water to study the depuration rate by sampling 4 perch after 8 h and after 1, 2, 3, 5, and 14 days following introduction to clean water. Water samples were also taken at each time point and stored frozen until analysis. During the entire experiment (uptake and depuration phase), perch were fed live zooplankton (Daphnia pulex; indoor population) every second day. Analyses of water samples from the aquaria and fish tissue were carried out using a triple quadruple mass spectrometer connected to a liquid chromatograph (Quantum Ultra EMR, Thermo Fisher Scientific, San Jose, CA, USA). For full information of this method see Brodin et al. (2013).
Statistics
Data on boldness and activity measured in the laboratory were analyzed using a general linear mixed-effect model, treating either “time in open area” (Boldness) or “time spent moving” (Activity) as response variables, treatment as a two-level fixed effect (Exposed and Control), and trial as a random effect. Response data were log +1 transformed to obtain normality. Differences between treatments (exposed and control) in mean boldness, activity, and home range in the lake were tested by t-tests, using the average values for individuals during each period as the response data. To test for differences in habitat preferences between treatments, the probability to reside in open (pelagic) water was modeled as a function of treatment using a generalized linear model with quasi-binomial error to account for overdispersion. Correlations between individual boldness and activity in the lab trials (mean value) and similar measures from the lake were tested using Spearman's rank correlation.
Results
In the laboratory assays, which were repeated twice before the release into Lake Tosktjärn, oxazepam-exposed perch displayed a slight increase in activity and boldness compared to non-exposed perch (Figures 1A,B). Notably, the behavioral modifications were only weakly expressed. Even though the general trends of behavioral change were repeated between trials, a two-tailed test showed no significant differences between the exposed and control groups (P > 0.05). However, as it has previously been shown that oxazepam increases boldness and activity in perch (Brodin et al., 2013, 2014; Klaminder et al., 2014), a directional hypothesis, and thus a one-tailed test (i.e., α = 0.1), justified the significance of the induced behavioral modifications. In the first laboratory trial, activity [F(1, 61) = 2.945, P = 0.091] and boldness [F(1, 61) = 3.520; P = 0.065] were significantly higher in the exposed group, according to a one-tailed test.
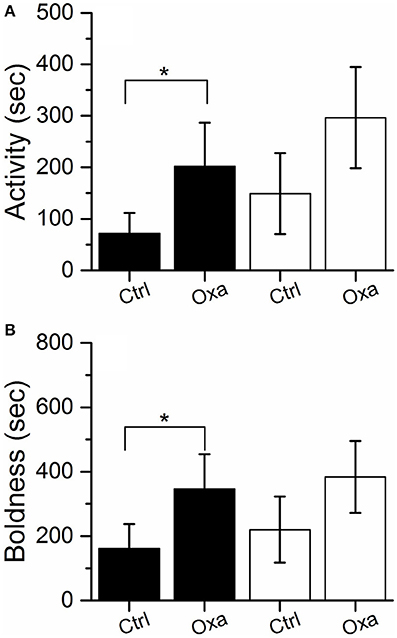
Figure 1. Activity (A) and boldness (B) measured in the laboratory for the control (Ctrl) and exposed (Oxa) group in the first (black) and second (white) trial (white). Significant effects according to a one-tailed test (α = 0.1) are indicated with *. Error bars represent ± 1 SE.
During the first minutes following introduction to the lake, both exposed and control fish remained close to the release site (Figure 2A), followed by an apparent willingness of fish with a GBM to disperse from the release site (Figure 2B). This difference in spatial use became even more pronounced after 24 h (Figure 2C). Contact with two transmitters from exposed perch were lost shortly after introduction into the lake; we attribute this loss to transmitter malfunctioning as avian and terrestrial predation could be excluded due to the ice cover (and two other transmitters stopped working already in the laboratory).
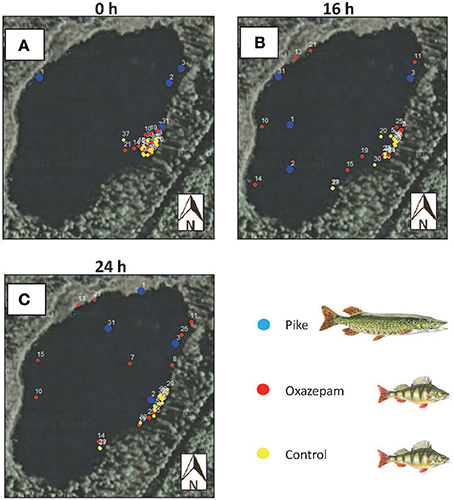
Figure 2. Positions of fish at three time-points after the release. Blue represents a pike, yellow a perch from the control group, and red a perch exposed to oxazepam. (A) is the initial release positions (first detection < 3 min after the release), (B) is position 16 h after the release, and (C) is the position 24 h after the release.
The uptake and depuration experiment indicated that oxazepam depurated slowly in cold water and fish muscle concentrations remained fairly stable during the first 9 days and was gone from the fish after about 18 days in clean water (Figure 3). Therefore, the in-depth statistical analyses of the long-term behavioral data were divided into two periods: (i) the first 9 days, where the therapeutic effect was assumed to be comparable with that generated in the laboratory trials and (ii) 24–32 days after the release, serving as a reference period where all oxazepam had most likely depurated according to a polynomial fit to the uptake and depuration data (corresponding to day 39–47 in Figure 3). The in-depth statistical analyses of the long-term behavioral data revealed that during the first 9 days the fish with GBM were more active (two-tailed t-test, t = 2.7, df = 19, p = 0.01) (Figure 4A) and bolder (two-tailed t-test, t = 2.3, df = 18, p = 0.038) (Figure 4B) in the control fish. In contrast, no such behavioral differences between the groups could be seen after 24 days (p > 0.40), a finding that agrees with the depuration data of oxazepam at low water temperatures. Hence, after 24 days in clean water we expect the behavior of the exposed fish to be unaffected by previous oxazepam exposure and hence to represent their natural behavior. Notably, the insignificant difference between the two groups after 9 days was caused by the increased activity and boldness of the control group, so the control group largely adopted a behavior similar to that of the exposed fish over time. Importantly, the laboratory measures of individual behavior (N = 26) were largely positively correlated to the comparable measures in the lake, where activity was significantly correlated (r = 0.38, P = 0.05) and boldness was marginally significantly correlated between the laboratory and field assays (r = 0.37, P = 0.06).
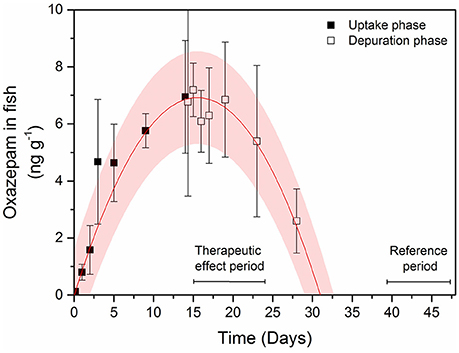
Figure 3. Concentrations of oxazepam in perch muscle tissue during the uptake phase (black squares) and depuration phase (open squares) in 5°C water. Error bars represent ± 1 SD. The pink shaded area is the 95% confidence interval of a polynomial function (r2 = 0.98) fitted to the temporal change in concentrations. Periods with assumed therapeutic effects from the oxazepam treatment (therapeutic effect period) within the whole lake experiment and no effect (reference period) used in the statistical analyzes are also shown.
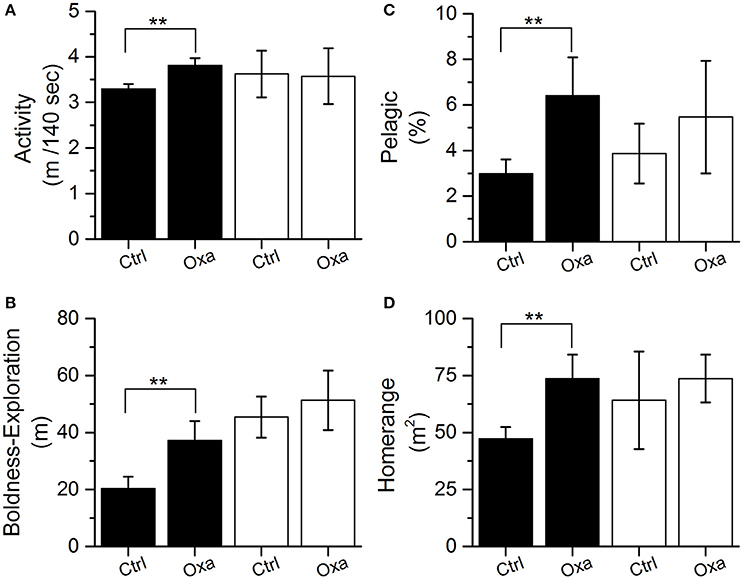
Figure 4. Activity (i.e., distance moved per 140 s) (A), boldness (i.e., average distance traveled from release site) (B), habitat use (proportion of time spend in the pelagic zone) (C), and home range size (50% core area) (D) measured in the lake for the control (Ctrl) and exposed (Oxa) group during day 0–9 (black; therapeutic effect period) and day 24–32 (white; reference period). Significant effects (p < 0.05) are indicated with **. Error bars represent ± 1 SE.
Individuals exposed to GBM also spent more time in the pelagic zone than the control fish during the first 7 days (generalized linear model, df = 24, P < 0.01), but the effect decreased over time and was only marginally significant when analyzing habitat use during the first 9 days (generalized linear model, df = 24, P = 0.067) (Figure 4C). In addition, as a consequence of GBM, the home range of the exposed fish was significantly larger than the home range of the control fish (two-tailed t-test, t = 2.4, df = 16, P = 0.031) (Figure 4D). Home range and habitat selection did not differ between the groups after 24 days when the therapeutic effect of oxazepam had most likely ceased (P > 0.40) and the control fish increased their use of the pelagic habitat and increased the size of their home range. There was no difference between the exposed and the control fish regarding their home range overlap with the home range of the pike during the first 9 days (Figure S6). All predation from pike on perch occurred after the first 9 days when the GBM likely no longer had an effect. In total, only four successful predations (two individuals from each treatment) were detected during the first 2 months.
Discussion
Without field verification, the predictive value of laboratory generated behavioral effects and predictions of subsequent ecological effects are in question. Here, however, we show that the more active and exploratory behavior of fish exposed to oxazepam shown in laboratory experiments (Brodin et al., 2013; Klaminder et al., 2014) also holds true in a natural lake ecosystem; hence, our main hypothesis appears valid as behavioral traits, or even changes in trait expressions, in the wild can indeed be predicted from laboratory assays. The lake fish exposed to oxazepam swam farther away from the release site and thus explored an unknown environment even under the threat of a predator (pike), a behavior considered to be a reliable measure of boldness (Ward et al., 2004). This behavioral effect largely mimics that seen for Atlantic salmon where individuals exposed to a GBM swam longer distances and where first to explore downstream environments, compared to unexposed individuals (Hellström et al., 2016).
In line with our expectations, the GBM affected how the fish moved in the lake and subsequently also even more complex ecological traits such as habitat selection and home range size. Individuals experiencing GBM used the pelagic zone to a greater extent than the control fish. As exposed fish showed bolder behavior both in the laboratory and in the lake, the differences in habitat preference can be explained by the exposed fish exploring more risky habitat according to the landscape of fear theory (Laundre et al., 2010). Here, the olfactory cues from the pike as well as their visual stimuli are expected to generate gradients of perceived risk in the lake and we assume that the lack of vegetation available for protection against predators in the pelagic zone generated increased perceived risk. We interpret the higher predation in the littoral zone as indirect effect of the perceived risk for the open water, i.e., predation occurred in the littoral zone because the predator followed the prey into this part of the lake (Figure S5). That the observed differences between the treatments were only detectable during the few days when behavioral effects of oxazepam were expected to be pronounced suggests that the differences between exposed and non-exposed fish was primarily an effect of the GBM. In other words, differences between treatments during the first 9 days were not the result of confounded initial differences in behavior between fish in the two groups.
We also showed that measures of boldness and activity were positively correlated between lab and field assays. Despite the fairly limited sample size in this study, the behavioral assays used here can be considered good predictors for perch activity and boldness in natural aquatic ecosystems and further indicate that behavior of fish in the lake can be modeled using laboratory assays. However, the large variation in laboratory-assayed behaviors, as seen in our study, often caused by short-term and unpredictable intra-individual variation (Stamps et al., 2012) constitutes a challenge. The importance of short-term variations can be reduced by measuring behavior over long periods, which at least partly explains the generally lower variation in the field behavior measures. In addition, the use of multiple tests incorporating different contexts, which are more robust measures of general boldness (Beckmann and Biro, 2013), might further improve correlations between behavioral measures in artificial settings and behavior in the wild.
Increased boldness implies increased risk of being exposed to predators, and increased predation mortality has been hypothesized to be the major concern when discussing ecological effects of GBM (Munday et al., 2010; Brodin et al., 2013; Klaminder et al., 2014; Ou et al., 2015). However, during the first 9 days of elevated boldness, no successful predation on perch occurred, which made it difficult to evaluate any potential increase in predation risk. The home range of the exposed perch overlapped as much with the pike home range as the control perch (Figure S6), suggesting that the GBM did not strongly affect predation exposure. That the control fish adopted similar behaviors as the exposed individuals over time, rather than the opposite (Figures 4A,C), further stress that the induced GBM was not strongly disadvantageous in the studied environment.
Effects of predation on prey survival and population dynamics are direct and easy to grasp from an ecological perspective as they imply zero fitness, whereas fitness effects of home range size and habitat selection are indirect and more difficult to visualize. Perch that experience GBM more frequently use the pelagic habitat and this will influence their metabolism, food availability, and subsequently growth and body morphology. We hypothesize that effects of GBM can be counterintuitive from a traditional ecotoxicological perspective as the net effect on important fitness correlates (e.g., growth) can be positive on an individual level. This reasoning is in line with previous findings suggesting that bolder individuals (domestically-bred individuals) grow faster and survive better in natural environments with low to moderate levels of predation pressures (Biro et al., 2004, 2006) and that perch inhabiting the non-vegetated pelagic zone grow faster and have a more streamlined body shape than perch living in the benthic zone (Svanback and Eklov, 2003; Olsson and Eklov, 2005). Nevertheless, such “positive effects” on an individual level can still be of ecological importance as their cascading effects on other organisms can be considerable (Klaminder et al., 2014).
One intriguing finding is that behavioral responses measured in the lab that would not have been statistically significant according to a typical two-tailed test were significant when measured in the field over a period of weeks. The study approach where behaviors of a control group are compared with that of a group of exposed individuals, using a two-tailed test, is currently common practice for protocols assessing effects of contaminants on behavior of aquatic organisms (Cachat et al., 2010; Maximino et al., 2010) and standard for general ecotoxicological protocols (OECD, 2015). In other words, if effects of oxazepam were not previously known, the laboratory results on how boldness and activity of fish is affected by the oxazepam treatment would have been considered non-significant, although these effects were later expressed in a natural ecosystem. This implies that behavior tests when used within an ecotoxicological context risk committing type II errors, i.e., consider effects as insignificant even though they are causing significant behavioral modifications in the wild. The most intuitive explanation for this is that laboratory assays are conducted over short periods (minutes to hours) where unpredictable intra-individual variability may lower the statistical power (Stamps et al., 2012). Measurements over longer periods, as in our field experiment, reduce the importance of short-term intra-individual variability and thus reduce the overall variation, increasing the possibility to detect treatment effects.
Because of apparent difficulties in detecting effects in short-term behavioral assays, it seems likely that treatments generating significant effects on activity or boldness of fish in laboratory settings will have at least as strong effects on fish behavior in the wild. Clearly, this is a concern given that many common contaminants have been found to generate responses in activity and boldness of fish in simple laboratory environments. Here, the GBM and subsequent increase in activity and boldness generated in oceans due to the ongoing rise of CO2 levels in the atmosphere seem alarming given the spatial scale of this process (Ou et al., 2015). At a more local scale, laboratory-based assays indicate that perch living in environments heavily affected by effluent water can experience concentrations of oxazepam sufficient for generating a GBM in the wild (Brodin et al., 2013). Such effects can become amplified as urbanization and more efficient water use strategies can increase concentrations in urban waters (Klaminder et al., 2015) or as natural bioaccumulation processes increase oxazepam concentrations in organisms over time (Lagesson et al., 2016). Indeed, behavioral assays measuring boldness and activity seem to have the capacity to detect such effects of oxazepam and other aquatic contaminants in the wild.
Author Contributions
JK designed the study and wrote the manuscript with scientific inputs from all co-authors. Laboratory work and field work were conducted mainly by JFa and GH with assistance from JK. GH made the statistical analyses with assistance of EB.
Funding
The study was financed through a fellowship to JK given by Knut and Alice Wallenberg foundation.
Conflict of Interest Statement
The authors declare that the research was conducted in the absence of any commercial or financial relationships that could be construed as a potential conflict of interest.
Acknowledgments
We thank the two reviewers for valuable comments on an early version of this manuscript, Johan Leander for field assistance, and Knut and Alice Wallenberg Stiftelse for financial support via a Wallenberg Academy Fellowship to JK.
Supplementary Material
The Supplementary Material for this article can be found online at: http://journal.frontiersin.org/article/10.3389/fenvs.2016.00081/full#supplementary-material
References
Adriaenssens, B., and Johnsson, J. I. (2011). Shy trout grow faster: exploring links between personality and fitness-related traits in the wild. Behave. Ecol. 22, 135–143. doi: 10.1093/beheco/arq185
Atchison, G. J., Henry, M. G., and Sandheinrich, M. B. (1987). Effects of metals on fish behavior: a review. Environ. Biol. Fishes 18, 11–25. doi: 10.1007/BF00002324
Beckmann, C., and Biro, P. A. (2013). On the validity of a single (boldness) assay in personality research. Ethology 119, 937–947. doi: 10.1111/eth.12137
Bertrand, M. R., DeNicola, A. J., Beissinger, S. R., and Swihart, R. K. (1996). Effects of parturition on home ranges and social affiliations of female white-tailed deer. J. Wildl. Manag. 60, 899–909. doi: 10.2307/3802391
Biro, P. A., Abrahams, M. V., Post, J. R., and Parkinson, E. A. (2004). Predators select against high growth rates and risk-taking behaviour in domestic trout populations. Proc. R. Soc. B Biol. Sci. 271, 2233–2237. doi: 10.1098/rspb.2004.2861
Biro, P. A., Abrahams, M. V., Post, J. R., and Parkinson, E. A. (2006). Behavioural trade-offs between growth and mortality explain evolution of submaximal growth rates. J. Anim. Ecol. 75, 1165–1171. doi: 10.1111/j.1365-2656.2006.01137.x
Blanchfield, P. J., Tate, L. S., Plumb, J. M., Acolas, M. L., and Beaty, K. G. (2009). Seasonal habitat selection by lake trout (Salvelinus namaycush) in a small Canadian shield lake: constraints imposed by winter conditions. Aquatic Ecol. 43, 777–787. doi: 10.1007/s10452-009-9266-3
Brodin, T., Fick, J., Johnson, M., and Klaminder, J. (2013). Dilute concentrations of a psychiatric drug alter behavior of fish from natural populations. Science 15, 814–815. doi: 10.1126/science.1226850
Brodin, T., Piovano, S., Fick, J., Klaminder, J., Heynen, M., and Jonsson, M. (2014). Ecological effects of pharmaceuticals in aquatic systems—impacts through behavioural alterations. Philos. Trans. R. Soc. Lond. B Biol. Sci. 369:20130580. doi: 10.1098/rstb.2013.0580
Cachat, J., Stewart, A., Grossman, L., Gaikwad, S., Kadri, F., Chung, K. M., et al. (2010). Measuring behavioral and endocrine responses to novelty stress in adult zebrafish. Nat. Protoc. 5, 1786–1799. doi: 10.1038/nprot.2010.140
Conrad, J. L., Weinersmith, K. L., Brodin, T., Saltz, J. B., and Sih, A. (2011). Behavioural syndromes in fishes: a review with implications for ecology and fisheries management. J. Fish Biol. 78, 395–435. doi: 10.1111/j.1095-8649.2010.02874.x
Dzieweczynski, T. L., Kane, J. L., Campbell, B. A., and Lavin, L. E. (2016). Fluoxetine exposure impacts boldness in female Siamese fighting fish, Betta splendens. Ecotoxicology 25, 69–79. doi: 10.1007/s10646-015-1568-8
Hara, T. (1976). Effects of pH on the olfactory responses to amino acids in rainbow trout Salmo gairdneri. Comp. Biochem. Physiol A 54, 37–39. doi: 10.1016/S0300-9629(76)80068-0
Havas, M., and Rosseland, B. O. (1995). Response of zooplankton, benthos, and fish to acidification: an overview. Water Air Soil Pollut. 85, 51–62. doi: 10.1007/BF00483688
Hellström, G., Klaminder, J., Finn, F., Persson, L., Alanärä, A., Jonsson, M., et al. (2016). GABAergic anxiolytic drug in water increases migration behaviour in salmon. Nat. Commun. 7:13460. doi: 10.1038/ncomms13460
Heynen, M., Fick, J., Jonsson, M., Klaminder, J., and Brodin, T. (2016). Effect of bioconcentration and trophic transfer on realized exposure to oxazepam in 2 predators, the dragonfly larvae (aeshna grandis) and the eurasian perch (perca fluviatilis). Environ. Toxicol. Chem. 35, 930–937. doi: 10.1002/etc.3368
Horne, J. S., Garton, E. O., Krone, S. M., and Lewis, J. S. (2007). Analyzing animal movements using Brownian bridges. Ecology 88, 2354–2363. doi: 10.1890/06-0957.1
Jones, J. C., and Reynolds, J. D. (1997). Effects of pollution on reproductive behaviour of fishes. Rev. Fish Biol. Fish 7, 463–491. doi: 10.1023/A:1018456315671
Klaminder, J., Brodin, T., Sundelin, A., Anderson, N. J., Fahlman, J., Jonsson, M., et al. (2015). Long-term persistence of an anxiolytic drug (Oxazepam) in a large freshwater lake. Environ. Sci. Technol. 49, 10406–10412. doi: 10.1021/acs.est.5b01968
Klaminder, J., Jonsson, M., Fick, J., Sundelin, A., and Brodin, T. (2014). The conceptual imperfection of aquatic risk assessment tests: highlighting the need for tests designed to detect therapeutic effects of pharmaceutical contaminants. Environ. Res. Lett. 9:084003. doi: 10.1088/1748-9326/9/8/084003
Lagesson, A., Fahlman, J., Brodin, T., Fick, J., Jonsson, M., Bystrom, P., et al. (2016). Bioaccumulation of five pharmaceuticals at multiple trophic levels in an aquatic food web - Insights from a field experiment. Sci. Total Environ. 568, 208–215. doi: 10.1016/j.scitotenv.2016.05.206
Lanctôt, C., Melvin, S. D., Leusch, F. D. L., Wilson, S., and Fabbro, L. (2016). Locomotor and behavioural responses of empire gudgeons (Hypseleotris compressa) exposed to coal mine wastewater. Chemosphere 144, 1560–1566. doi: 10.1016/j.chemosphere.2015.10.026
Laundre, J. W., Hernandez, L., and Ripple, W. J. (2010). The landscape of fear: ecological implications of being afraid. Open Ecol. J. 3, 1–7. doi: 10.2174/1874213001003030001
Liedtke, T., and Rub, M. (2012). “Techniques for telemetry transmitter attachment and evaluation of transmitter effects on fish performance: Chapter 4,” in Telemetry Techniques: A User Guide for Fisheries Research, eds N. S. Adams, J. W. Beeman, and J. H. Eiler (Bethesda, MD: American Fisheries Society).
Maximino, C., Marques de Brito, T., Dias, C., Gouveia, A. Jr., and Morato, S. (2010). Scototaxis as anxiety-like behavior in fish. Nat. Protoc. 5, 209–216. doi: 10.1038/nprot.2009.225
Melvin, S. D., and Wilson, S. P. (2013). The utility of behavioral studies for aquatic toxicology testing: a meta-analysis. Chemosphere 93, 2217–2223. doi: 10.1016/j.chemosphere.2013.07.036
Munday, P. L., Dixson, D. L., Donelson, J. M., Jones, G. P., Pratchett, M. S., Devitsina, G. V., et al. (2009). Ocean acidification impairs olfactory discrimination and homing ability of a marine fish. Proc. Natl. Acad. Sci. U.S.A. 106, 1848–1852. doi: 10.1073/pnas.0809996106
Munday, P. L., Dixson, D. L., McCormick, M. I., Meekan, M., Ferrari, M. C. O., and Chivers, D. P. (2010). Replenishment of fish populations is threatened by ocean acidification. Proc. Natl. Acad. Sci. U.S.A. 107, 12930–12934. doi: 10.1073/pnas.1004519107
Nilsson, G. E., Dixson, D. L., Domenici, P., McCormick, M. I., Sorensen, C., Watson, S. A., et al. (2012). Near-future carbon dioxide levels alter fish behaviour by interfering with neurotransmitter function. Nat. Clim. Chang. 2, 201–204. doi: 10.1038/nclimate1352
OECD (2015).OECD Guidelines for the Testing of Chemicals, Section 2 Effects on Biotic Systems. Paris: OECD publishing.
Olsson, J., and Eklov, P. (2005). Habitat structure, feeding mode and morphological reversibility: factors influencing phenotypic plasticity in perch. Evol. Ecol. Res. 7, 1109–1123. Available online at: http://www.diva-portal.org.proxy.ub.umu.se/smash/get/diva2:158847/FULLTEXT01.pdf
Ou, M., Hamilton, T. J., Eom, J., Lyall, E. M., Gallup, J., Jiang, A., et al. (2015). Responses of pink salmon to CO2-induced aquatic acidification. Nat. Clim. Chang. 5, 950�955. doi: 10.1038/nclimate2694
Pereira, P., Puga, S., Cardoso, V., Pinto-Ribeiro, F., Raimundo, J., Barata, M., et al. (2016). Inorganic mercury accumulation in brain following waterborne exposure elicits a deficit on the number of brain cells and impairs swimming behavior in fish (white seabream—Diplodus sargus). Aquat. Toxicol. 170, 400–412. doi: 10.1016/j.aquatox.2015.11.031
Persson, L., Andersson, J., Wahlstrom, E., and Eklov, P. (1996). Size-specific interactions in lake systems: predator gape limitation and prey growth rate and mortality. Ecology 77, 900–911. doi: 10.2307/2265510
Réale, D., Reader, S. M., Sol, D., McDougall, P. T., and Dingemanse, N. J. (2007). Integrating animal temperament within ecology and evolution. Biol. Rev. 82, 291–318. doi: 10.1111/j.1469-185X.2007.00010.x
Regan, M. D., Turko, A. J., Heras, J., Andersen, M. K., Lefevre, S., Wang, T., et al. (2016). Ambient CO2, fish behaviour and altered GABAergic neurotransmission: exploring the mechanism of CO2-altered behaviour by taking a hypercapnia dweller down to low CO2 levels. J. Exp. Biol. 219, 109–118. doi: 10.1242/jeb.131375
Reyhanian, N., Volkova, K., Hallgren, S., Bollner, T., Olsson, P. E., Olsen, H., et al. (2011). 17 alpha-Ethinyl estradiol affects anxiety and shoaling behavior in adult male zebra fish (Danio rerio). Aquat. Toxicol. 105, 41–48. doi: 10.1016/j.aquatox.2011.05.009
Scott, G. R., and Sloman, K. A. (2004). The effects of environmental pollutants on complex fish behaviour: integrating behavioural and physiological indicators of toxicity. Aquat. Toxicol. 68, 369–392. doi: 10.1016/j.aquatox.2004.03.016
Sprague, J. B. (1971). Measurement of pollutant toxicity to fish—III: Sublethal effects and “safe” concentrations. Water Res. 5, 245–266. doi: 10.1016/0043-1354(71)90171-0
Stamps, J. A., Briffa, M., and Biro, P. A. (2012). Unpredictable animals: individual differences in intraindividual variability (IIV). Anim. Behav. 83, 1325–1334. doi: 10.1016/j.anbehav.2012.02.017
Svanback, R., and Eklov, P. (2003). Morphology dependent foraging efficiency in perch: a trade-off for ecological specialization? Oikos 102, 273–284. doi: 10.1034/j.1600-0706.2003.12657.x
Team, R. C. (2015).R: A Language and Environment for Statistical Computing. Vienna: R Foundation for Statistical Computing. Available online at: http://www.R-project.org
Vignet, C., Joassard, L., Lyphout, L., Guionnet, T., Goubeau, M., Le Menach, K., et al. (2015). Exposures of zebrafish through diet to three environmentally relevant mixtures of PAHs produce behavioral disruptions in unexposed F1 and F2 descendant. Environ. Sci. Pollut. Res. 22, 16371–16383. doi: 10.1007/s11356-015-4157-8
Ward, A. J. W., Thomas, P., Hart, P. J. B., and Krause, J. (2004). Correlates of boldness in three-spined sticklebacks (Gasterosteus aculeatus). Behav. Ecol. Sociobiol. 55, 561–568. doi: 10.1007/s00265-003-0751-8
Welch, M. J., Watson, S. A., Welsh, J. Q., McCormick, M. I., and Munday, P. L. (2014). Effects of elevated CO2 on fish behaviour undiminished by transgenerational acclimation. Nat. Clim. Chang. 4, 1086–1089. doi: 10.1038/nclimate2400
Keywords: anxiety, field manipulation, behavior, GABAergic, landscape of fear
Citation: Klaminder J, Hellström G, Fahlman J, Jonsson M, Fick J, Lagesson A, Bergman E and Brodin T (2016) Drug-Induced Behavioral Changes: Using Laboratory Observations to Predict Field Observations. Front. Environ. Sci. 4:81. doi: 10.3389/fenvs.2016.00081
Received: 31 July 2016; Accepted: 09 December 2016;
Published: 26 December 2016.
Edited by:
Karen A. Kidd, University of New Brunswick, CanadaReviewed by:
Rebecca Klaper, University of Wisconsin–Milwaukee, USAPaul Blanchfield, Fisheries and Oceans Canada, Canada
Copyright © 2016 Klaminder, Hellström, Fahlman, Jonsson, Fick, Lagesson, Bergman and Brodin. This is an open-access article distributed under the terms of the Creative Commons Attribution License (CC BY). The use, distribution or reproduction in other forums is permitted, provided the original author(s) or licensor are credited and that the original publication in this journal is cited, in accordance with accepted academic practice. No use, distribution or reproduction is permitted which does not comply with these terms.
*Correspondence: Jonatan Klaminder, am9uYXRhbi5rbGFtaW5kZXJAdW11LnNl