- 1Department of Civil and Mechanical Engineering, University of Cassino and Southern Lazio, Cassino, Italy
- 2Department of Civil, Architectural and Environmental Engineering, University of Naples Federico II, Naples, Italy
- 3Department of Mathematics and Applications Renato Caccioppoli, University of Naples Federico II, Naples, Italy
- 4UNESCO-IHE Institute for Water Education, Delft, Netherlands
HIGHLIGHTS
• Direct correlation between substrate composition and TP effect was identified.
• The new experimental TP set-up minimized organic compound loss during TP of FW.
• The solubilization of carbohydrate and protein determined the optimal temperature of FW TP.
• Low temperature (80°C) TP attained the highest carbohydrate solubilization and methane yield.
The effects of thermal pretreatment (TP) on the main characteristics of food waste (FW) and its biochemical methane potential (BMP) and distribution of volatile fatty acids (VFAs) under mesophilic condition (35°C) were investigated. The TP experiments were carried out at 80, 100, 120°C for 2 h and 140°C for 1 h. The designed TP set-up was able to minimize the organic matter loss during the course of the pretreatment. Soluble organic fractions evaluated in terms of chemical oxygen demand (COD) and soluble protein increased linearly with pretreatment temperature. In contrast, the carbohydrate solubilization was more enhanced (30% higher solubilization) by the TP at lower temperature (80°C). A slight increment of soluble phenols was found, particularly for temperatures exceeding 100°C. Thermally pretreated FW under all conditions exhibited an improved methane yield compared to the untreated FW, due to the increased organic matter solubilization. The highest cumulative methane yield of 442 (±8.6) mL/gVSadded, corresponding to a 28.1% enhancement compared to the untreated FW, was obtained with a TP at 80°C. No significant variation in the VFAs trends were observed during the BMP tests under all investigated conditions.
Introduction
With the worldwide economic development and population growth, food waste (FW) production is alarmingly increasing and imposes a great challenge in waste management for most countries (Zhang et al., 2014; Li and Jin, 2015). Today, anaerobic digestion (AD) is one of the most consolidated technologies for waste treatment and valorization, compared to other possible treatment routes (Ferreira et al., 2013; Wagner et al., 2014; Zhang et al., 2014). The process allows to achieve a two-fold advantage of obtaining continuous power generation, whilst reducing the amount of waste to be disposed and thus alleviating environmental pollution (Elbeshbishy et al., 2012). Moreover, different directives such as the Renewable Energy directive (2009/28/EC, EU, 2009) and the Landfill directive (1999/31/EC, EU, 1999) were enacted by European countries. These laws stimulated further research and encouraged the intensive practice of AD for organic waste treatment (Bougrier et al., 2007; Marin et al., 2011; Wang et al., 2014).
The AD process is an integrated biochemical conversion of organic matter into biogas, through a sequence of four basic steps: hydrolysis, acidogenesis, acetogenesis and methanogenesis (Minale and Worku, 2014; Zhang et al., 2014). More in details, during the AD of FW, the first step of hydrolysis involves solubilization of particulate matter and bioconversion of organic polymers to monomers/dimers, making it the rate-limiting step for the overall process, and thus resulting in the necessity of larger reactor volumes for this specific type of organic waste (Bougrier et al., 2008; Kondusamy and Kalamdhad, 2014; Tampio et al., 2014; Ariunbaatar et al., 2014a). This is due to the nature of the substrate, which is mainly constituted of complex organic matter in particulate form (carbohydrate, protein, lipid and fat, lignocellulosic material) and a smaller inorganic part (Marin et al., 2010; Vavouraki et al., 2013; Wang et al., 2014). Therefore, through accelerating the FW solubilization, the whole process as well as reactor efficiency can greatly improve (Jiang et al., 2014; Wang et al., 2014). In these aspects, substrate pretreatments are effective methods for enhancing the methane yield from FW AD (Ferreira et al., 2013; Ariunbaatar et al., 2014b).
Several methods for the pretreatment of organic substrates have been proposed. The most common methods include mechanical grinding (Izumi et al., 2010), ultrasound (Elbeshbishy et al., 2011; Jiang et al., 2014), microwave (Marin et al., 2010, 2011), thermal (Liu et al., 2012; Tampio et al., 2014; Ariunbaatar et al., 2014b), chemical (Elbeshbishy et al., 2011; Ma et al., 2011), biological (Vavouraki et al., 2014) pretreatments or their combination (Strong and Gapes, 2012; Vavouraki et al., 2013). Among these methods, thermal pretreatment (TP) is considered as an economically viable and environmentally friendly alternative, as reported by different authors (Strong et al., 2011; Gianico et al., 2013; Zhou et al., 2013; Ariunbaatar et al., 2014a).
TP methods have been applied to several types of organic wastes in order to modify their structure by breaking the intermolecular bonds and thus aid in the release of soluble organic monomers/dimers, that are more accessible and readily biodegradable by anaerobic bacteria (Vavouraki et al., 2013). Therefore, the kinetics of the AD process are improved, leading to a higher reactor efficiency (Bougrier et al., 2007; Wang et al., 2010; Zhou et al., 2013; Kondusamy and Kalamdhad, 2014; Li and Jin, 2015). Heating temperature and pretreatment time have been found as the key factors in determining the effectiveness of TP (Carrère et al., 2009; Rincón et al., 2013). Accordingly, wide ranges of temperature (50–250°C) and treatment time (0.5–12 h) have been adopted and tested based on the ultimate methane yield from FW (Elbeshbishy et al., 2011; Liu et al., 2012; Zhou et al., 2013; Prabhudessai et al., 2014; Tampio et al., 2014; Ariunbaatar et al., 2014a). In particular, these two parameters influence (i) the hydrolysis of organic matter in particulate form and the subsequent biodegradation enhancement, (ii) the loss of volatile organic matter, and (iii) the formation of refractory/inhibitory compounds (Carlsson et al., 2012). The latter two represent the main negative effects of TP on AD processes, and both processes usually occur at higher TP temperatures (Kondusamy and Kalamdhad, 2014). Loss of organic compounds can occur due to evaporation by the applied heat during TP, and hence induce a net decrease of available organic matter for methane production (Carlsson et al., 2012). Formation of inhibitory compounds during the course of TP can occur by two main phenomena: the release of soluble refractory compounds, e.g., phenolic compounds (Marin et al., 2010) or through chemical reactions of different soluble monomers, named as Maillard reactions (Liu et al., 2012). The reactions involve the non-enzymatic transformation of sugars and soluble protein and cause the formation of melanoidin compounds (Ariunbaatar et al., 2014a).
Despite of the large number of studies on this specific topic, no systematic research that relates the aforementioned effects of TP on the solubilization of FW organic matter and the subsequent biodegradation enhancement, and the correlation with the substrate organic composition has been conducted. In addition, conflicting results about the TP effects on FW have been reported in the literature. For instance, Liu et al. (2012) reported that TP of kitchen waste at 175°C for 60 min induced an increase in solubilization of particulate matter from 96.6 to 116.5 g/kg in terms of volatile dissolved solid concentration and a decrease (7.9%) of methane production as a result of melanoidin compound formation. In contrast, Wang et al. (2010) obtained a 13.6% higher cumulative methane yield due to the higher dissolved organic solid concentration achieved (greater than 30% hydrolysis ratio) after TP of municipal biowaste at 175°C for 60 min. In general, studies affirmed that the best temperature for TP of FW is in the range of 80–100°C (Ariunbaatar et al., 2014b). Other studies, instead, reported the proper temperature range for FW TP as 120–160°C (Ma et al., 2011; Yin et al., 2014) or even 170–175°C (Wang et al., 2010; Zhou et al., 2013).
In the present work, the effect of TP on FW characteristics at different temperatures was studied in order to better understand and fill the existing knowledge gaps on the topic. Deep investigations on solubilization of FW during TP and methane yield enhancement were performed using a newly proposed TP system. The release of soluble compounds, i.e., carbohydrate, protein, soluble chemical oxygen demand (COD) and inhibitory compounds were monitored, and their impacts on AD biodegradability were evaluated for each TP condition. In particular, the relationship between soluble carbohydrate and soluble protein with the methane production was assessed. Biochemical methane potential (BMP) tests were used for evaluating the improvement of the methane yield and individual VFAs production after each TP condition.
Materials and Methods
Substrate and Inoculum
FW synthetically prepared according to the method described by Ariunbaatar et al. (2014b) was used as the substrate. The FW composition was based on the actual characteristics of the food waste of most EU countries. The use of synthetic waste allows the repeatability of experimental results and their comparison with previous research. The following composition was used: 79% of vegetables and fruits; 5% of cooked pasta and rice; 6.0% of bread and bakery; 8.0% of meat and fish; and 2.0% dairy products (on wet basis). The synthetic FW was blended and stored in a refrigerator at −20°C until use. The anaerobic inoculum used in BMP tests was obtained from a full scale AD plant located in Capaccio-Salerno (Italy). The plant treats buffalo manure and dairy wastes at mesophilic conditions. The main physico-chemical characteristics of both the FW and inoculum are reported in Table 1.
Thermal Pretreatment (TP) Set-Up
Lab-scale TP experiments are mostly conducted in open systems using either an autoclave (Laurent et al., 2011; Gianico et al., 2013), oven or thermal baths (Rincón et al., 2013; Xue et al., 2015), which usually lead to high loss of organic compounds and odor emission during pretreatment of substrates. In this study, a new experimental TP set-up was developed (Figure 1). This set-up consisted of a thermostat oil bath equipped with a magnetic stirrer bar and temperature control unit, 500 mL pyrex glass bottle, two 50 mL trapping columns and water displacing gas measuring systems. The treatment bottle was connected with an inert plastic tube to the trapping column and gas measuring systems. The system operated in closed and airtight conditions. The trapping columns were placed in series (Figure 1) and filled with high pH sodium phosphate buffer solution (Na3PO3 and Na2HPO3 at a pH of 12), intended to trap volatile organic compounds released during the course of the TP. Phosphate buffers have been widely used in many biological and pharmaceutical applications in order to maintain organic compounds in their original forms (Soliman et al., 2015). In addition, as phosphoric acid has multiple dissociation constants, the buffer can be prepared near to three pHs, i.e., 2.15, 6.86, and 12.32. The trapping potential of the buffer solution as well as experimental replicability of the TP using the developed set-up was preliminary evaluated at each TP condition. This was performed using known concentrations of a synthetic organic acid solution containing a mixture of acetic acid, propionic acid and butyric acid (data not shown). The concentrations of the mixture before and after each TP and the amount trapped in the buffer were quantified and balanced.
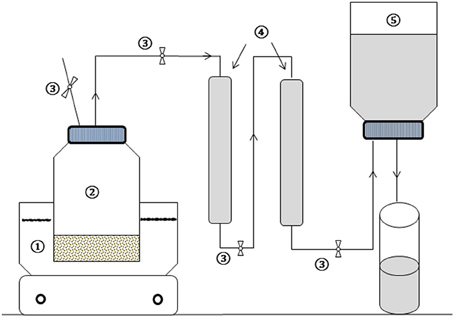
Figure 1. Schematic diagram of the TP experimental set-up: (1) Oil bath heater with stirrer, (2) treatment bottle, (3) controlling valves, (4) trapping columns, and (5) water displacement gas measuring system.
Afterwards, 100 g of raw FW was placed in the treatment bottle for each TP condition. The gaseous mixture released during TP passed through the buffer trap solution and was volumetrically quantified by means of a water displacement system. After TP, the treatment bottle was cooled to ambient temperature prior to opening to avoid evaporation/volatilization and further organic matter loss. The gases were taken from the headspace of the displacement water system and analyzed for gaseous composition. Buffer samples were taken for COD analysis.
Four different temperatures 80, 100, 120°C for 2 h and 140°C for 1 h, were tested with regard to their effectiveness in terms of organic matter solubilization and enhancing methane production. These temperature ranges and pretreatment times were chosen based on the EU regulation (EC 1774/2002), which states that catering waste should be sterilized at a temperature of ≥70°C for at least 60 min, or at a temperature >133°C for at least 30 min. Moreover, these TP temperatures were shown to improve the AD process of various organic wastes (Carrère et al., 2009; Rincón et al., 2013; Ariunbaatar et al., 2014b). Each TP condition was performed in triplicate and results are presented as average values.
The proposed system provides operational advantages such as reduction of odor during TP experiments, simplicity of construction, easy operation and, in particular, the system allows not only to reduce the loss of organic matter during TP, but to capture and quantify the loss of volatile organic compounds in the buffer solutions.
Sample Preparation and Calculations
Each sample of the raw and thermally pretreated FW was diluted with ultrapure water (1 g/50 mL) to properly measure the soluble fractions. The diluted samples were centrifuged at 4000 rpm for 15 min and filtered through a 0.45 μm microfiber filter prior to analysis. The following parameters of the solid and soluble fraction for raw and pretreated FW were analyzed: COD, protein, carbohydrate, phenols and VFAs. The effect of TP on the FW characteristics was mainly quantified by the extent of solubilization (Laurent et al., 2011). As defined in the work of Rincón et al. (2013) and Kim et al. (2003), solubilization is the transformation of the particulate fraction of FW to the soluble fraction and estimated by the following equation:
Where S refers to COD, carbohydrate or protein; Ss and St represent the soluble and total fraction of each parameter.
Biochemical Methane Potential Tests
BMP tests were carried out in 1 L glass bottles at mesophilic (35 ± 2°C) conditions, with a substrate to inoculum ratio (S/I) of 0.5 gVS/gVS, following the protocol described by Esposito et al. (2012). The temperature was maintained constant by a water bath connected with a thermostatically controlled flow heater. BMP tests of the untreated FW were carried out in order to quantify the effect of pretreatment on the substrate. BMP tests of the inoculum were also conducted in order to determine the net biogas production rate for each pretreated and untreated FW. Prior to the start of BMP experiments, the headspace of each bottle was flushed with argon gas to provide anaerobic conditions. Mixing was done manually daily. Biogas produced was measured daily by the displacement of acidified water to reduce CO2 solubility. Subsequently, gas samples were collected from the headspace with an air tight syringe for methane (CH4) and carbon dioxide (CO2) determination. The BMP tests were followed until no gas was produced anymore. All tests were carried out in duplicate and results are given as average values of the tests. The cumulative methane production was normalized to standard temperature and pressure (STP).
Analytical Methods
Total solids (TS), volatile solids (VS), total and soluble COD, total Kjeldahl Nitrogen (TKN) and phenols (total and soluble) were determined according to standard methods for examination of water and wastewater (APHA, 1998). Total main elemental content, i.e., Carbon (TEC), Hydrogen (TEH) and Sulfur (TES) of FW was measured simultaneously by catalytic oxidation using an elemental analyzer (PerkinElmer® 2400 Series II). The temperature of the combustion and reduction zones were set at 970°C and 500°C, respectively, and argon gas was used as a purging gas.
Total and soluble protein were quantified by the Lowry-Folin method using bovine serum albumin (BSA) as the standard (Lowry et al., 1951). Total and soluble carbohydrate were analyzed using the phenol-sulfuric acid method with glucose as the standard (Herbert et al., 1971). Both the protein and carbohydrate were measured through spectrophotometry (PhotoLab® 6600 UV-VIS SERIUS). Liquid-liquid extraction with chloroform and methanol as solvent was used to quantify total lipid (Phillips et al., 1997). To analyse individual VFAs, 1.5 mL samples were collected from each BMP bottle during the BMP tests. Subsequently, samples were centrifuged at a speed of 5000 rpm for 7 min and filtered through a 0.2 μm microfiber filter. The filtrate samples were analyzed with High Pressure Liquid Chromatography (HPLC) (Dionex LC 25 Chromatography Oven) equipped with Synergi 4u Hydro RP 80A (size 250 × 4.60 mm) column and UV detector (Dionex AD25 Absorbance Detector).
The biogas composition was determined using gas chromatography (GC, Varian STAR 3400), equipped with a ShinCarbon ST 80/100 column and thermal conductivity detector. The temperatures of injector port, detector and oven temperature were 50, 120, and 120°C, respectively, with argon as the carrier gas.
Statistical Analysis
Statistical analysis was carried out using anova1 MATLAB tool. Data were analyzed by one-way ANOVA. The level of significance was set at p < 0.05. The significance of differences in the average methane yields, soluble and total COD, soluble and total carbohydrates, soluble and total proteins were determined.
Results
Characteristics of FW before and after Each TP
Table 3 presents the main characteristics of FW before and after each TP. A general decreasing trend of the total COD and total elemental carbon (TEC) concentrations were observed with increasing TP temperature. The total COD was reduced by 3.6, 4.6, 5.4, and 7.4% for TP at 80, 100, 120, and 140°C, respectively, compared to the untreated FW. Similarly, the TEC concentration with respect to the untreated FW was decreased by 1.1, 3.1, 5.3, and 9.1% at 80, 100, 120, and 140°C, respectively. In contrast, the soluble COD concentration shows an increasing trend with TP temperature. Figure 2 illustrates the solubilization of COD, carbohydrate and protein of FW before and after each TP. The carbohydrate and protein solubilization have different trends, while the concentration of soluble COD increased linearly with TP temperature. The highest carbohydrate solubilization of around 30% was obtained at 80°C, followed by 28.4% at 100°C, 27.8% at 120°C, 26.5% at 140°C and 18.4% for untreated FW (Figure 2). Protein solubilization increased linearly with pretreatment temperature and reached its maximum value (around 20%) at 140°C. The protein solubilization had a similar trend as the COD solubilization (Figure 2). A one-way ANOVA analysis showed a large statistical difference between the data sets (Table 2).
The lactic acid and VFAs concentrations (i.e., acetic, propionic and butyric acid) of thermally pretreated FW were lower than the control and this reduction was more significant at higher temperature TPs (Table 3). The reason was that some of the VFAs were evaporated during the course of the TP. This was supported by the gas analysis in the headspace after each TP, in which neither CO2 nor H2 gas was detected (data not shown), which indicates that evaporation of organic compounds was the main cause for the decrease of VFAs concentration and thus TEC during the TPs of FW.
Total and soluble phenol before and after TP were determined to assess the possible release of soluble phenols due to the increased temperature exposure (Table 4). The presence of soluble phenols is an important parameter as they can inhibit the AD process (Rincón et al., 2013). A slight increase of the soluble phenol concentration was observed for pretreatment temperatures higher than 100°C.
Cumulative Biogas and Methane Yields
Figure 3 shows the cumulative biogas and methane production from the BMP tests of thermally pretreated and untreated FW. The specific cumulative methane yields of each thermally pretreated and untreated FW are illustrated in Figure 4. All the pretreated FW achieved a higher volumetric biogas and methane production than the control. The highest volumetric methane production was obtained for FW treated at 80°C (Figure 3B). The cumulative specific methane yield was 442 (± 8.6), 374 (± 10.9), 390 (± 12.4), 414 (± 8.9), and 345 (± 12.7) mL/gVSadded for 80, 100, 120, 140°C and the control, respectively (Figure 4). The one-way ANOVA showed that there was a significant difference among the methane yields of the pretreated FW (Table 2). The corresponding enhancement of the specific methane yield with respect to the control was 28.1, 8.3, 12.9, and 20% at 80, 100, 120, and 140°C, respectively. Furthermore, the specific methane yields in terms of mL/gCOD before and after TP are given in Table 5. It should be noted that the higher specific methane yield of all thermally treated FW compared to the untreated (Table 5) coupled with the minor COD loss in the new TP system (Table 3) might have a positive energy balance and thus lead to increased economic profits of the AD process.
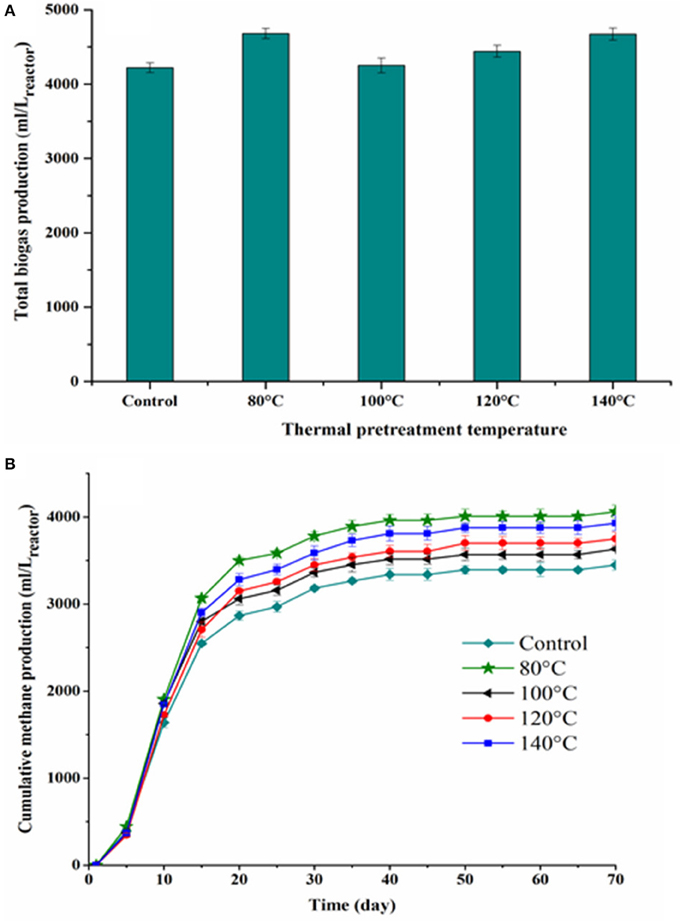
Figure 3. Total biogas production (A) and cumulative methane production as a function of digestion time (B) for each TP.
Figure 5 presents the percentage of methane gas in the produced biogas during each BMP test. The methane percentage increased from around 20 to 80% during the first 10 days of the digestion period for all tested conditions and afterwards remained around 58–69% until the end of the digestion period. A higher methane percentage in the produced biogas was observed for all thermally treated FW compared to the control, while no significant variations of the methane percentage were noticed between the TP conditions.
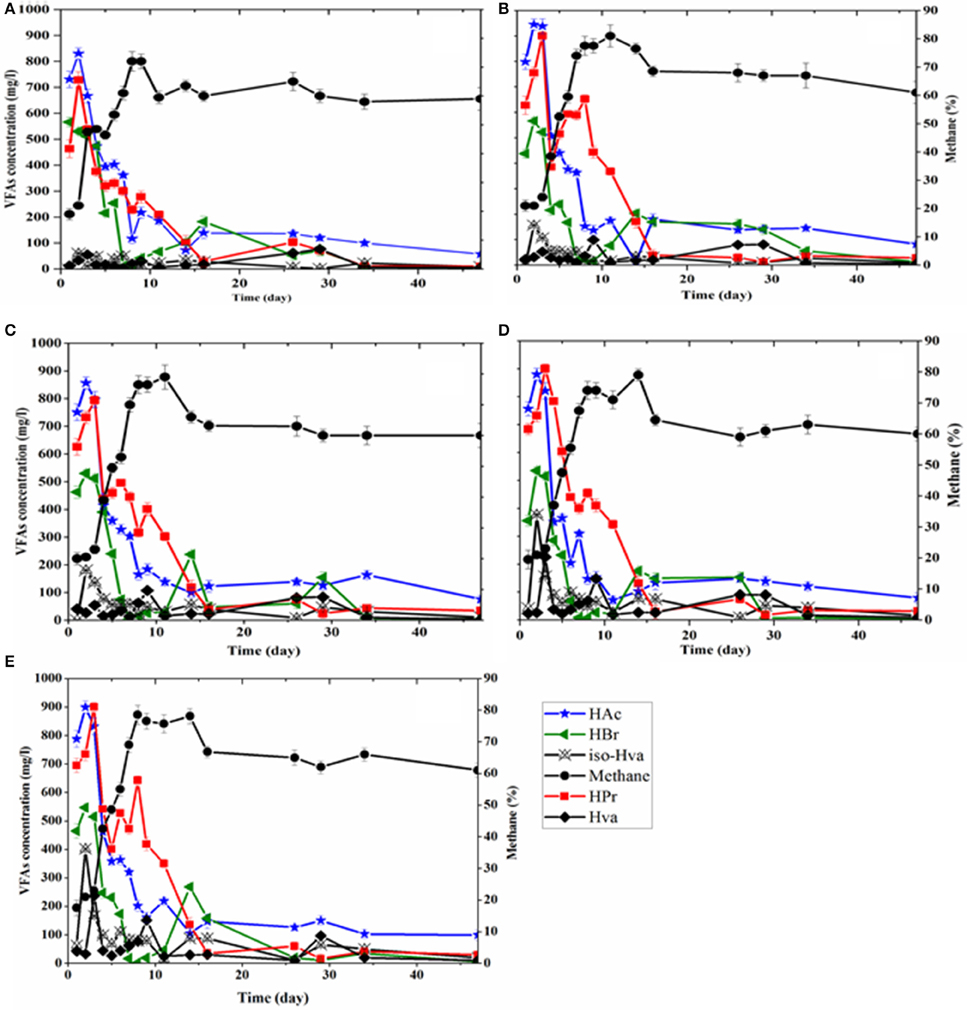
Figure 5. Methane concentration and VFAs production pattern as a function of digestion time: (A) control, and TP at (B) 80°C, (C) 100°C, (D) 120°C, and (E) 140°C.
VFAs Distribution during BMP Tests
The VFAs distribution trends were followed during the BMP tests in order to observe any possible impact of TP on their production rate in the digestion process. The concentration profiles of the VFAs with respect to the digestion time in each BMP test are shown in Figure 5. Higher values of VFAs were obtained for all pretreated FWs compared to the control, though the concentration of some of the acids, i.e., lactic acid and VFAs (acetic and propionic acids), was reduced during the course of the TP (Table 3). Acetic, propionic and butyric acids were the major VFAs produced during the early experimental period, with acetic acid being the dominant VFA under all tested conditions. After 3 day of digestion, the highest total acetate concentration achieved was 2681.5 mg/L for the 80°C TP, followed by 2520.0 mg/L in 140°C TP, 2457.5 mg/L in 120°C TP, 2403.5 mg/L in 100°C TP and 2227.5 mg/L in the control BMP test bottle. The respective concentrations of propionic and butyric acids in these experimental days were 2282.0 and 1526 mg/L for TP at 80°C, 2152.0 and 1504.5 mg/L for TP at 100°C, 2322.0 and 1406.0 mg/L for TP at 120°C, 2328.5 and 1527.0 mg/L for TP at 140°C and 1729.0 and 1607.0 mg/L for the control. Valeric and iso-valeric acid concentrations were found higher for all thermally treated FW compared to the control and were more significant at higher temperatures (Figure 5).
In contrast, the pattern of VFAs production and consumption during the BMP tests appeared similar regardless of a thermal pretreatment. The higher concentrations of VFAs in the initial days (days 1–5) were due to the faster hydrolysis and acidification of FW (Li and Jin, 2015). Nonetheless, the concentration of the VFAs did not inhibit the methanogenic activity, as evidenced by the increased methane concentration during these operational days (days 1–5). From day 6 onwards, the VFAs concentration showed a descending trend in all BMP tests (Figure 5).
Analysis of the Carbon Balance
Carbon balances for each TP condition were estimated (Figure 6) in order to better understand the phenomena occurring during TP of FW. The carbon balance estimations were determined by considering the total elemental carbon (TEC) before and after each TP (Table 3) as well as the total COD concentration of the buffer solutions after each TP, according to the following equation:
Where: TECinitial = the total elemental carbon concentration of untreated FW TECafter TP = the total elemental carbon concentration after TP Total CODbuffer = the total COD concentration trapped at each TP others = the organic compounds that were lost during the course of TP
The TEC content linearly declined with increasing pretreatment temperatures (Figure 6 and Table 3). The amount of organic compounds trapped in the buffer solution was 1.1, 2.7, 4.5, and 7.4% for TP at 80, 100, 120, and 140°C, respectively. At the TP temperature of 120 and 140°C, relatively higher amounts of organics were lost compared to the lower temperature TPs. This was due to the observed high pressure in the TP bottles at these temperatures.
Discussion
Influence of TP on FW Characteristics
This study demonstrated the influence of TP on the main characteristics of FW. The higher COD solubilization after each TP compared to the control indicates that the TP significantly improved the FW characteristics by promoting the breakdown of complex organic particulates into soluble compounds. The carbohydrate and protein solubilization were also remarkably increased for all TP conditions, achieving a maximum value of 30 and 20% solubilization of the carbohydrate and protein, respectively (Figure 2). These results are also comparable with previous studies. Marin et al. (2010) obtained an improvement of starch hydrolysis at a temperature of 60–70°C for kitchen waste. Yin et al. (2014) observed an increase of soluble COD from 73.3 to 121.3 g/kg and soluble protein from 1.33 to 21.38 g/kg after hydrothermal pretreatment of FW at 100–200°C. Thermal pretreatment temperatures around 120–121°C have also been found to increase COD solubilization of FW by 19% (Ma et al., 2011) and from 0.86 to 2.6 g/L of soluble COD of cottage cheese waste (Prabhudessai et al., 2014).
The increased solubilization of these compounds compared to the untreated FW could be due to thermal disintegration of the bonds that link the polysaccharides and amino acid strands, thus aiding the release of soluble monomers/dimers from the bulk complex structure of FW. Vavouraki et al. (2013) reported that during pretreatment of FW, cleaving of glycoside bonds of carbohydrate polymers occurred and resulted in an increase of the concentration of mono sugars (glucose and fructose).
The carbohydrate solubilization was more favored at lower temperature pretreatments, i.e., 80°C, in contrast to poor protein solubilization at this particular temperature. Indeed, at higher temperatures it could be hypothesized to have a larger amount of soluble monomers of carbohydrates. However, the decrease of the soluble carbohydrate concentration at higher temperatures might be attributed to the formation of complex melanoidin compounds. The production of these compounds was confirmed by the observed turning of color to light brown (data not shown) and scorched flavor during the TP of FW at higher temperatures. Indeed, during higher temperature TPs, soluble monomers of carbohydrates might be involved in Maillard and caramelization reactions, which result in the formation of various flavoring and coloring compounds (Bougrier et al., 2008; Liu et al., 2012). In addition, the formation of these less biodegradable compounds was confirmed by the decrease of methane production at higher TP temperatures. These compounds are heterogeneous polymers characterized by a high-molecular weight that are not only difficult to degrade, but can also inhibit the degradation of other organics by suppressing the methanogenic activity (Tampio et al., 2014).
The loss of organic carbon was smaller (Figure 6) compared to the work of Elbeshbishy et al. (2011), who obtained a decrease of around 12% of the particulate organic fraction after TP of FW at 70°C for 30 min. For all TP investigated, indeed, the proposed new TP set-up succeeded in trapping the evaporated organics in the buffer solution (Figure 6). These results confirmed the feasibility of counteracting one of the negative effects of high temperature TP, i.e., the loss of organics during TP. Considering the scale up of the TP system, the captured organics such as lactic acid and VFAs can be further extracted and valorized for other applications, e.g., in the chemical industry (Wang et al., 2014; Yin et al., 2014), for biopolymer production such as polyhydroxyalkanoates (PHAs) via bacterial strains (Kumar et al., 2016), or biohydrogen production via photofermentation (Ghimire et al., 2015).
Enhancement of Methane and VFA Yields in the BMP Tests
The production of biogas and methane gas from FW was highly facilitated by the TP under all conditions (Figure 4). The highest specific methane yield for the pretreatment temperature of 80°C highlighted the impact of low temperature TP on enhancing the biodegradability of FW. A similar higher methane yield was obtained by Ariunbaatar et al. (2014b) after thermal pretreatment of FW at 80°C for 1.5 h compared to other pretreatment temperatures and times. In another study, Elbeshbishy et al. (2011) observed an improvement of the biogas yield after TP of FW at 70°C for 30 min.
The methane yield with pretreatment temperatures of 120 and 140°C was found higher than with a TP at 100°C, though at these temperatures a decrease of soluble carbohydrate and total COD were observed (Figure 2 and Table 3). However, it should be noted that the solubilization of other organic molecules, in particular protein, was higher at these temperatures. The results indicate that despite the soluble carbohydrate concentration was lower at these temperatures, there is a positive effect due to the solubilization of protein, resulting in an increase of methane yield. It has been reported in the literature that for substrates containing a high amount of protein, a maximum solubilization was achieved at higher TP temperatures (Bougrier et al., 2007). Raju et al. (2013) compared thermal pretreatment of pig manure from 100 to 225°C and found a lower methane yield at 100°C and an increasing trend with temperature from 125 until 200°C. Prabhudessai et al. (2014) found around 15% increment in methane yield from thermally treated cottage cheese solid waste at 120°C for 20 min. Strong and Gapes (2012) reported that thermal pretreatment of chicken feathers at 140°C led to a 3.5-fold higher methane yield compared to heating at 70°C.
The formation of inhibitory compounds, i.e., the formation of melanoidin and slight increment of the soluble phenol concentration at higher temperature TP (Table 4), did not completely alter the methanogenic activity as confirmed by the higher methane yield compared to the control (Figures 3, 4). According to literature data, the inhibitory concentrations of such compounds are obtained at pretreatment temperatures higher than 150°C (Marin et al., 2010; Liu et al., 2012; Ariunbaatar et al., 2014a). A lower methane yield was achieved after autoclaving FW at 160°C, due to the occurrence of Maillard reactions as evidenced by a dark color and caramelized odor (Tampio et al., 2014).
Considering the VFAs profile during the BMP tests, the higher production of lower molecular weight VFAs (acetic, propionic and butyric acid, Figure 5), could be ascribed to the larger proportion of carbohydrate concentrations present in the FW and its enhanced solubilization after TP (Lim et al., 2008; Wang et al., 2014). The results are consistent with the reports of Li and Jin (2015), who obtained higher concentrations of acetic, propionic and n-butyric acid for thermally treated kitchen waste compared to untreated kitchen waste during the anaerobic fermentation process. The rapid decrease of acetic acid corresponded to the increased methane percentage in the produced biogas in all BMP tests (Figure 5), which reflected the dominant activities of acetoclastic methanogens during the BMP tests (Luo et al., 2011). These results in turn suggest the wellbeing and stable operation of the AD process, since 70% of the methane gas in the AD process is derived from acetate that is mainly consumed by acetoclastic methanogens (Wagner et al., 2014; Aydin et al., 2015). The increase of valeric and iso-valeric acid for TP temperatures exceeding 100°C might be due to the higher solubilization of protein after the TPs, as the formation of these VFAs has been associated with degradation of protein compounds (Parawira et al., 2004).
Correlation between Soluble Carbohydrate and Protein Concentration with Methane Yield
The correlation between the concentration of soluble carbohydrate and soluble protein with cumulative methane yield was performed for each TP (Figure 7). A direct relationship between soluble carbohydrate/soluble protein and methane yields was obtained in this study. The low temperature TP (80°C) attained the highest methane yield. This was mainly attributed to the increased soluble carbohydrate at this specific temperature coupled to the high amount of carbohydrate in the FW (Table 3). However, higher temperature TP (>100°C) had positive effects on solubilization of protein, which also improves the methane yields. Based on this relationship, it is possible to reason out the apparent conflicting results reported in previous studies (Wang et al., 2010; Liu et al., 2012). The efficiency of a TP is the combinatory effect of pretreatment temperature and substrate composition (Carrère et al., 2009; Rincón et al., 2013). The TP of substrates rich in carbohydrate, e.g., FW, was more effective at lower temperature, accelerating the breakdown of complex compounds and increasing the release of soluble monomers. In addition, the lower temperature TP maintained the bioavailability of these soluble monomers for the subsequent enzymatic and biochemical reactions by avoiding the formation of less biodegradable melanoidin compounds. Conversely, when the substrates are characterized by a high protein content, higher temperature TP favored their solubilization and thus improved the methane yields. Similarly, Kondusamy and Kalamdhad (2014) highlighted that the positive effects of TP were strongly dependent on both pretreatment temperature and substrate composition, and hence a TP should be properly designed to induce the desired positive effects on the AD of FW (Kondusamy and Kalamdhad, 2014).
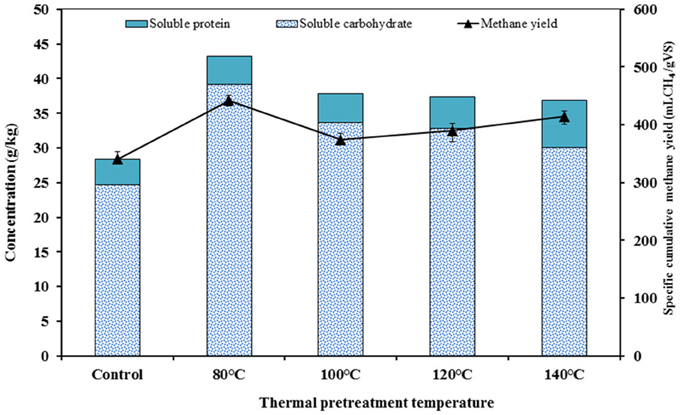
Figure 7. Correlation between the concentration of soluble carbohydrate and soluble protein with the methane yield for each TP investigated.
Conclusion
- In this study, a remarkable improvement of organic matter solubilization and methane production of FW was obtained after TP.
- A direct correlation between soluble carbohydrate, soluble protein and methane yields with pretreatment temperature was observed. Lower temperature TP (80°C) was better suited for substrates owing a high carbohydrate content, resulting in the highest soluble carbohydrate concentration and enhanced methane yield.
- This study showed that the loss of organic compounds through evaporation was significantly reduced at lower TP temperature.
- At higher TP temperatures, the solubilization of protein from FW was more favored and melanoidin compounds were formed. However, the amounts of these less biodegradable compounds did not alter the digestion process and methane yields.
- The new proposed TP set-up is promising with several advantages: minimized negative effects of TP (loss of organics and odor emission), simple to implement and able to evaluate the main effects of TP on FW.
Author Contributions
MY designed the study, performed the experiments as well as interpreted the data, wrote the manuscript and revised it until its final version. LF participated in designing the study as well as in analyzing the output data and in writing the manuscript. GE, PL, and FP participated in writing the manuscript and revising it critically.
Conflict of Interest Statement
The authors declare that the research was conducted in the absence of any commercial or financial relationships that could be construed as a potential conflict of interest.
Acknowledgments
The authors acknowledge the financial support from the European Union (EACEA) under the Erasmus Mundus program of Environmental Technologies for Contaminated Solids, Soils and Sediments (ETeCoS3, grant agreement of FPA No. 2010-0009) and the project of “Modular photo—biologic reactor for bio—hydrogen: application to dairy waste—RE—MIDA” from the Agriculture department of the Campania Region (programme of rural development 2007–2013, Measure 124).
References
APHA (1998). Standard Methods for the Examination of Water and Wastewater, 20th Edn. Washington, DC: American Public Health Association/American Water Works Association/Water Environment Federation.
Ariunbaatar, J., Panico, A., Esposito, G., Pirozzi, F., and Lens, P. N. L. (2014a). Pretreatment methods to enhance anaerobic digestion of organic solid waste. Appl. Energy 123, 143–156. doi: 10.1016/j.apenergy.2014.02.035
Ariunbaatar, J., Panico, A., Frunzo, L., Esposito, G., Lens, P. N. L., and Pirozzi, F. (2014b). Enhanced anaerobic digestion of food waste by thermal and ozonation pretreatment methods. J. Environ. Manage. 146, 142–149. doi: 10.1016/j.jenvman.2014.07.042
Aydin, S., Ince, B., and Ince, O. (2015). Application of real-time PCR to determination of combined effect of antibiotics on Bacteria, Methanogenic Archaea, Archaea in anaerobic sequencing batch reactors. Water Res. 76, 88–98. doi: 10.1016/j.watres.2015.02.043
Bougrier, C., Delgenès, J. P., and Carrère, H. (2007). Impacts of thermal pre-treatments on the semi-continuous anaerobic digestion of waste activated sludge. Biochem. Eng. J. 34, 20–27. doi: 10.1016/j.bej.2006.11.013
Bougrier, C., Delgenès, J. P., and Carrère, H. (2008). Effects of thermal treatments on five different waste activated sludge samples solubilisation, physical properties and anaerobic digestion. Chem. Eng. J. 139, 236–244. doi: 10.1016/j.cej.2007.07.099
Carlsson, M., Lagerkvist, A., and Morgan-Sagastume, F. (2012). The effects of substrate pre-treatment on anaerobic digestion systems: a review. Waste Manag. 32, 1634–1650. doi: 10.1016/j.wasman.2012.04.016
Carrère, H., Sialve, B., and Bernet, N. (2009). Improving pig manure conversion into biogas by thermal and thermo-chemical pretreatments. Bioresour. Technol. 100, 3690–3694. doi: 10.1016/j.biortech.2009.01.015
Elbeshbishy, E., Hafez, H., Dhar, B. R., and Nakhla, G. (2011). Single and combined effect of various pretreatment methods for biohydrogen production from food waste. Int. J. Hydrogen Energy 36, 11379–11387. doi: 10.1016/j.ijhydene.2011.02.067
Elbeshbishy, E., Nakhla, G., and Hafez, H. (2012). Biochemical methane potential (BMP) of food waste and primary sludge: influence of inoculum pre-incubation and inoculum source. Bioresour. Technol. 110, 18–25. doi: 10.1016/j.biortech.2012.01.025
Esposito, G., Frunzo, L., Liotta, F., Panico, A., and Pirozzi, F. (2012). Bio-methane potential tests to measure the biogas production from the digestion and co-digestion of complex organic substrates. Open Environ. Eng. J. 5, 1–8. doi: 10.2174/1874829501205010001
Ferreira, L. C., Donoso-Bravo, A., Nilsen, P. J., Fdz-Polanco, F., and Pérez-Elvira, S. I. (2013). Influence of thermal pretreatment on the biochemical methane potential of wheat straw. Bioresour. Technol. 143, 251–257. doi: 10.1016/j.biortech.2013.05.065
Ghimire, A., Frunzo, L., Pirozzi, F., Trably, E., Escudie, R., Lens, P. N. L., et al. (2015). A review on dark fermentative biohydrogen production from organic biomass: process parameters and use of by-products. Appl. Energy 144, 73–95. doi: 10.1016/j.apenergy.2015.01.045
Gianico, A., Braguglia, C. M., Mescia, D., and Mininni, G. (2013). Ultrasonic and thermal pretreatments to enhance the anaerobic bioconversion of olive husks. Bioresour. Technol. 147, 623–626. doi: 10.1016/j.biortech.2013.08.054
Herbert, D., Philipps, P. J., and Strange, R. E. (1971). Carbohydrate analysis. Meth. Enzymol. 5B, 265–277.
Izumi, K., Okishio, Y.-K., Nagao, N., Niwa, C., Yamamoto, S., and Toda, T. (2010). Effects of particle size on anaerobic digestion of food waste. Int. Biodeterior. Biodegradation 64, 601–608. doi: 10.1016/j.ibiod.2010.06.013
Jiang, J., Gong, C., Wang, J., Tian, S., and Zhang, Y. (2014). Effects of ultrasound pre-treatment on the amount of dissolved organic matter extracted from food waste. Bioresour. Technol. 155, 266–271. doi: 10.1016/j.biortech.2013.12.064
Kim, J., Park, C., Kim, T.-H., Lee, M., Kim, S., Kim, S.-W., et al. (2003). Effects of various pretreatments for enhanced anaerobic digestion with waste activated sludge. J. Biosci. Bioeng. 95, 271–275. doi: 10.1016/S1389-1723(03)80028-2
Kondusamy, D., and Kalamdhad, A. S. (2014). Pre-treatment and anaerobic digestion of food waste for high rate methane production- a review. J. Environ. Chem. Eng. 2, 1821–1830. doi: 10.1016/j.jece.2014.07.024
Kumar, P., Ray, S., and Kalia, V. C. (2016). Production of co-polymers of polyhydroxyalkanoates by regulating the hydrolysis of biowastes. Bioresour. Technol. 200, 413–419. doi: 10.1016/j.biortech.2015.10.045
Laurent, J., Casellas, M., Carrère, H., and Dagot, C. (2011). Effects of thermal hydrolysis on activated sludge solubilization, surface properties and heavy metals biosorption. Chem. Eng. J. 166, 841–849. doi: 10.1016/j.cej.2010.11.054
Li, Y., and Jin, Y. (2015). Effects of thermal pretreatment on acidification phase during two-phase batch anaerobic digestion of kitchen waste. Renewable Energy 77, 550–557. doi: 10.1016/j.renene.2014.12.056
Lim, S.-J., Kim, B. J., Jeong, C.-M., Choi, J.-D.-R., Ahn, Y. H., and Chang, H. N. (2008). Anaerobic organic acid production of food waste in once-a-day feeding and drawing-off bioreactor. Bioresour. Technol. 99, 7866–7874. doi: 10.1016/j.biortech.2007.06.028
Liu, X., Wang, W., Gao, X., Zhou, Y., and Shen, R. (2012). Effect of thermal pretreatment on the physical and chemical properties of municipal biomass waste. Waste Manag. 32, 249–255. doi: 10.1016/j.wasman.2011.09.027
Lowry, O. H., Rosebrough, N. J., Farr, A. L., and Randall, R. J. (1951). Protein measurement with the Folin phenol reagent. J. Biol. Chem. 193, 265–275.
Luo, G., Xie, L., Zhou, Q., and Angelidaki, I. (2011). Enhancement of bioenergy production from organic wastes by two-stage anaerobic hydrogen and methane production process. Bioresour. Technol. 102, 8700–8706. doi: 10.1016/j.biortech.2011.02.012
Ma, J., Duong, T. H., Smits, M., Verstraete, W., and Carballa, M. (2011). Enhanced biomethanation of kitchen waste by different pre-treatments. Bioresour. Technol. 102, 592–599. doi: 10.1016/j.biortech.2010.07.122
Marin, J., Kennedy, K. J., and Eskicioglu, C. (2010). Effect of microwave irradiation on anaerobic degradability of model kitchen waste. Waste Manage. 30, 1772–1779. doi: 10.1016/j.wasman.2010.01.033
Marin, J., Kennedy, K. J., and Eskicioglu, C. (2011). Enhanced solubilization and anaerobic biodegradability of source-separated kitchen waste by microwave pre-treatment. Waste Manag. Resour. 29, 208–218. doi: 10.1177/0734242X10362705
Minale, M., and Worku, T. (2014). Anaerobic co-digestion of sanitary wastewater and kitchen solid waste for biogas and fertilizer production under ambient temperature: waste generated from condominium house. Int. J. Environ. Sci. Technol. 11, 509–516. doi: 10.1007/s13762-013-0255-7
Parawira, W., Murto, M., Read, J. S., and Mattiasson, B. (2004). Volatile fatty acid production during anaerobic mesophilic digestion of solid potato waste. J. Chem. Technol. Biotechnol. 79, 673–677. doi: 10.1002/jctb.1012
Phillips, K. M., Tarrago-Trani, M. T., Grove, T. M., Grün, I., Lugogo, R., Harris, R. F., et al. (1997). Simplified gravimetric determination of total fat in food composites after chloroform-methanol extraction. J. Am. Oil Chemist. Soc. 74, 137–142. doi: 10.1007/s11746-997-0158-1
Prabhudessai, V., Salgaonkar, B., Braganca, J., and Mutnuri, S. (2014). Pretreatment of cottage cheese to enhance biogas production. Biomed. Res. Int. 2014:374562. doi: 10.1155/2014/374562
Raju, C. S., Sutaryo, S., Ward, A. J., and Møller, H. B. (2013). Effects of high-temperature isochoric pre-treatment on the methane yields of cattle, pig and chicken manure. Environ. Technol. 34, 239–244. doi: 10.1080/09593330.2012.689482
Rincón, B., Bujalance, L., Fermoso, F. G., Martín, A., and Borja, R. (2013). Biochemical methane potential of two-phase olive mill solid waste: influence of thermal pretreatment on the process kinetics. Bioresour. Technol. 140, 249–255. doi: 10.1016/j.biortech.2013.04.090
Soliman, M. G., Pelaz, B., Parak, W. J., and del Pino, P. (2015). Phase transfer and polymer coating methods toward improving the stability of metallic nanoparticles for biological applications. Chem. Mater. 27, 990–997. doi: 10.1021/cm5043167
Strong, P. J., and Gapes, D. J. (2012). Thermal and thermo-chemical pre-treatment of four waste residues and the effect on acetic acid production and methane synthesis. Waste Manag. 32, 1669–1677. doi: 10.1016/j.wasman.2012.04.004
Strong, P. J., McDonald, B., and Gapes, D. J. (2011). Combined thermochemical and fermentative destruction of municipal biosolids: a comparison between thermal hydrolysis and wet oxidative pre-treatment. Bioresour. Technol. 102, 5520–5527. doi: 10.1016/j.biortech.2010.12.027
Tampio, E., Ervasti, S., Paavola, T., Heaven, S., Banks, C., and Rintala, J. (2014). Anaerobic digestion of autoclaved and untreated food waste. Waste Manag. 34, 370–377. doi: 10.1016/j.wasman.2013.10.024
Vavouraki, A. I., Angelis, E. M., and Kornaros, M. (2013). Optimization of thermo-chemical hydrolysis of kitchen wastes. Waste Manag. 33, 740–745. doi: 10.1016/j.wasman.2012.07.012
Vavouraki, A. I., Volioti, V., and Kornaros, M. E. (2014). Optimization of thermo-chemical pretreatment and enzymatic hydrolysis of kitchen wastes. Waste Manag. 34, 167–173. doi: 10.1016/j.wasman.2013.09.027
Wagner, A. O., Reitschuler, C., and Illmer, P. (2014). Effect of different acetate:propionate ratios on the methanogenic community during thermophilic anaerobic digestion in batch experiments. Biochem. Eng. J. 90, 154–161. doi: 10.1016/j.bej.2014.05.014
Wang, K., Yin, J., Shen, D., and Li, N. (2014). Anaerobic digestion of food waste for volatile fatty acids (VFAs) production with different types of inoculum: effect of pH. Bioresour. Technol. 161, 395–401. doi: 10.1016/j.biortech.2014.03.088
Wang, W., Hou, H., Hu, S., and Gao, X. (2010). Performance and stability improvements in anaerobic digestion of thermally hydrolyzed municipal biowaste by a biofilm system. Bioresour. Technol. 101, 1715–1721. doi: 10.1016/j.biortech.2009.10.010
Xue, Y., Liu, H., Chen, S., Dichtl, N., Dai, X., and Li, N. (2015). Effects of thermal hydrolysis on organic matter solubilization and anaerobic digestion of high solid sludge. Chem. Eng. J. 264, 174–180. doi: 10.1016/j.cej.2014.11.005
Yin, J., Wang, K., Yang, Y., Shen, D., Wang, M., and Mo, H. (2014). Improving production of volatile fatty acids from food waste fermentation by hydrothermal pretreatment. Bioresour. Technol. 171, 323–329. doi: 10.1016/j.biortech.2014.08.062
Zhang, C., Su, H., Baeyens, J., and Tan, T. (2014). Reviewing the anaerobic digestion of food waste for biogas production. Renewable Sustainable Energy Rev. 38, 383–392. doi: 10.1016/j.rser.2014.05.038
Keywords: anaerobic digestion, thermal pretreatment, food waste, substrate composition, solubilization, methane production potential
Citation: Yeshanew MM, Frunzo L, Lens PNL, Pirozzi F and Esposito G (2016) Mass Loss Controlled Thermal Pretreatment System to Assess the Effects of Pretreatment Temperature on Organic Matter Solubilization and Methane Yield from Food Waste. Front. Environ. Sci. 4:62. doi: 10.3389/fenvs.2016.00062
Received: 15 February 2016; Accepted: 05 September 2016;
Published: 23 September 2016.
Edited by:
Kartik Chandran, Columbia University, USAReviewed by:
Hojeong Kang, Yonsei University, South KoreaSeung Gu Shin, Pohang University of Science and Technology, South Korea
Copyright © 2016 Yeshanew, Frunzo, Lens, Pirozzi and Esposito. This is an open-access article distributed under the terms of the Creative Commons Attribution License (CC BY). The use, distribution or reproduction in other forums is permitted, provided the original author(s) or licensor are credited and that the original publication in this journal is cited, in accordance with accepted academic practice. No use, distribution or reproduction is permitted which does not comply with these terms.
*Correspondence: Martha M. Yeshanew, bWFydGFtaW5hbGVAZ21haWwuY29t