- 1Biology Department and Canadian Rivers Institute, University of New Brunswick, Saint John, NB, Canada
- 2Biology, Forestry, and Environmental Management and Canadian Rivers Institute, University of New Brunswick, Fredericton, NB, Canada
- 3Department of Earth and Environmental Science, Acadia University, Wolfville, NS, Canada
Methylmercury (MeHg) bioaccumulation in lower-trophic-level organisms and its subsequent biomagnification through food webs differs in magnitude among lakes and results in intraspecific variability of MeHg in top predator fishes. Understanding these differences is critical given the reproductive and neurotoxic effects of MeHg on fishes and their predators, including humans. In this study we characterized the food webs of five lakes in New Brunswick, Canada, supporting Brook Trout (Salvelinus fontinalis) using measures of relative trophic position (δ15N) and carbon sources (δ13C), determined the concentrations of MeHg in invertebrates and total Hg (THg) in fishes, and quantified MeHg biomagnification from primary to tertiary consumers. Methyl Hg and THg concentrations were highest in biota from lakes with lower pH. The trophic magnification slopes (TMS; log Hg vs. δ15N) varied significantly among lakes (0.13–0.20; ANCOVA, p = 0.031). When combined with data from other salmonid lakes in temperate and Arctic Canada (n = 36), among-system variability in TMS was best, but weakly, positively predicted by aqueous total phosphorous (p = 0.028, 0.109). These results suggest that lake productivity directly or indirectly influences the biomagnification of MeHg through diverse food webs supporting salmonids.
Introduction
The biomagnification of methylmercury (MeHg) through aquatic food webs can result in exposures that affect the health of top predator fishes and their consumers, including humans. For example, fishes display reduced spawning success, altered reproductive behaviors, and suppressed sex hormones associated with elevated MeHg exposure (Drevnick and Sandheinrich, 2003; Sandheinrich and Miller, 2006). Similarly, productivity of fish-eating birds such as the common loon (Gavia immer) decreases as their exposure to dietary MeHg increases (Burgess and Meyer, 2008). Recent assessments suggest that the risks from MeHg exposure to wildlife have been underestimated and that concentrations in tissues believed to be protective against reproductive or other effects are exceeded in piscivorous fishes across North America (Sandheinrich et al., 2011; Depew et al., 2012).
Concentrations of total Hg (THg) in fishes can vary substantially among nearby lakes and streams, and factors underlying these differences have been the subject of a number of comprehensive reviews and/or meta-analyses to understand factors that predict concentrations of this element in different fishes (e.g., Kamman et al., 2005; Hanna et al., 2015). More specifically, concentrations of THg are generally higher in larger, older fishes and fishes higher up in the food web (Boudou and Ribeyre, 1997; Watras et al., 1998; Mason et al., 2000). In addition, the source of dietary carbon (measured with carbon isotopes, δ13C) is an important determinant of fish Hg; within systems, fishes or invertebrates relying more on autochthonous or pelagic carbon sources in lakes tend to accumulate more Hg than more littoral-feeding species or those relying on allochthonous carbon (Kainz et al., 2002; Ethier et al., 2008; Chételat et al., 2011; but see Eagles-Smith et al., 2008). Given that diet is the main source of Hg to fishes, its concentrations are largely determined by Hg source strength and inputs, availability, transformation, and uptake in the base of the food web supporting the fishes. Several lake characteristics are predictive of Hg in fishes within and among regions; more specifically, fish higher in Hg are often found in lakes with lower aqueous pH and higher density of wetlands in the catchment (Kamman et al., 2005; Chasar et al., 2009). Lakes with higher atmospheric deposition of Hg, such as those in eastern North America, also tend to have biota with elevated Hg concentrations (Evers et al., 2007; Scheuhammer et al., 2016). Dissolved organic carbon (DOC) quantity and quality influence the delivery of Hg to lakes from the catchment, the availability of Hg(II) for methylation, and the uptake of MeHg into primary producers and their consumers, though not always in a consistent manner (Greenfield et al., 2001; Haitzer et al., 2003; Weech et al., 2004; Kamman et al., 2005; Simonin et al., 2008; Gabriel et al., 2009; Lescord et al., 2015). There have been contradictory results with regard to DOC as a predictor of biotic Hg concentrations (e.g., Miskimmin et al., 1992; Driscoll et al., 1995; Ravichandran, 2004) and recent evidence of a DOC threshold for bioavailability of MeHg to lower-trophic-levels (Deison et al., 2012; French et al., 2014).
The biomagnification of MeHg through food webs determines its concentrations in top predator fishes, and this process varies among systems within a region (Lescord et al., 2015) and globally (Lavoie et al., 2013). The average biomagnification of MeHg through food webs is calculated from slopes of log total Hg (THg) or MeHg vs. stable nitrogen isotope values (δ15N; measure of relative trophic position) of biota, and these trophic magnification slopes (TMS) are related to physical-chemical characteristics of ecosystems (Lavoie et al., 2013). For example, TMS within regions are positively or negatively correlated with aqueous nutrient levels (Kidd et al., 2012; Clayden et al., 2013, respectively), and greater TMS occur at higher latitudes (Lavoie et al., 2013). The reasons for these relationships remain unclear and broader comparisons are challenged by the lack of environmental data included in published studies and their differences in experimental design (Lavoie et al., 2013). Nonetheless, these comparisons are critical for understanding and predicting the fish species and lakes at greatest risk from Hg contamination.
Brook Trout (Salvelinus fontinalis) are common across northeastern North America and a popular sports fish, and some populations contain THg concentrations that pose a risk to the fish themselves and their consumers (Kamman et al., 2005). Previous studies such as this one have focused on understanding among-system variability in Hg concentrations in this species; in contrast, the trophic transfer of this element through food webs supporting Brook Trout has not been examined. Diets for this species are highly variable and can include aquatic insects, terrestrial insects, snails, crustaceans, and fish (Scott and Scott, 1988; Browne and Rasmussen, 2009). In streams in New Brunswick (NB), Canada, Brook Trout rely heavily on allochthonous carbon sources and their THg concentrations were poorly predicted by stream pH, unlike another species of fish with strong reliance on authochthonous carbon (Jardine et al., 2012). The main objectives of the current study were to: (1) examine the biological factors that predict THg in Brook Trout in New Brunswick lakes, (2) quantify the biomagnification of Hg through lakes supporting Brook Trout, and (3) compare results from these lakes to those from diverse systems in other regions of Canada to develop a broader understanding of factors related to both the trophic transfer of Hg and its concentrations in salmonids. To achieve these objectives, we first investigated biotic Hg concentrations, food web structure using δ15N and δ13C, and Hg TMS in five lakes in New Brunswick that contain Brook Trout as a top predator. We then combined these results with comparable food web and environmental data from other temperate (Kidd et al., 2012; Clayden et al., 2013) and Arctic lakes in Canada (Lescord et al., 2015) to determine whether any physical-chemical characteristics of the lakes could explain the variability in fish THg concentrations or TMS among systems.
Methods
Study Sites
Five lakes containing Brook Trout were chosen to represent systems across the province of New Brunswick, Canada, that had little or no development in the catchment. Moose and Tahoe Lakes contained allopatric Brook Trout populations while A, Bathurst, and Nictau lakes contained Brook Trout populations living sympatrically with 8, 7, and 13 other fish species, respectively (Finley, 2013).
Biological and Chemical Sampling
Profundal, pelagic, and littoral invertebrates were collected three times during 2008 for A (May, July, and October) and Tahoe (June, July, and September) Lakes, in June and August of 2005 for Bathurst and Nictau Lakes, and in June of 2008 from Moose Lake (sampled only once due to restricted access). Profundal chironomids were collected using an Ekman dredge in the deepest part of each lake, and sweep nets were used to sample and collect the more abundant macroinvertebrate taxa from shoreline areas (three or more shoreline sites were sampled in each lake on each date). Multiple vertical tows of a Wisconsin net (153 μm) through the water column were used to collect zooplankton on each date. Profundal and littoral invertebrates were kept in lake water overnight, live-sorted to major taxa on site in lake water and frozen along with bulk pelagic zooplankton samples until used for analysis.
Brook Trout, Banded Killifish (Fundulus diaphanus), Blacknose Dace (Rhinichthys atratulus), Brown Bullhead (Ictalurus nebulosus), Common Shiner (Luxilus cornutus), Creek Chub (Semotilus atromaculatus), Fourspine Stickleback (Apeltes quadracus), Golden Shiner (Notemigonus crysoleucas), Lake Chub (Couesius plumbeus), Smelt (Osmerus mordax), White Sucker (Catostomus commersonii), and Yellow Perch (Perca flavescens) were collected using trap nets and minnow traps in the same years as the invertebrate collections for a given lake. For lakes with species other than Brook Trout, sampling was done to collect fishes that represent different habitats and a range of available sizes, and a minimum sample size of 8 was targeted. Target sample size for Brook Trout was 20, though this was not achieved in all lakes, and fish representing a range of sizes in the population were processed (Finley, 2013). Weight (± 0.1 g) and fork length (± 1 mm) were measured for all fish, and they were kept on ice until dissected and the tissues were frozen.
Subsurface (<1 m) grab samples (1/lake/date) of water were collected from a central location on each lake at the same time as the biological samples, kept on ice and then analyzed at the Analytical Services Laboratory, Fredericton, NB, for the suite of analytes listed in Table 1.
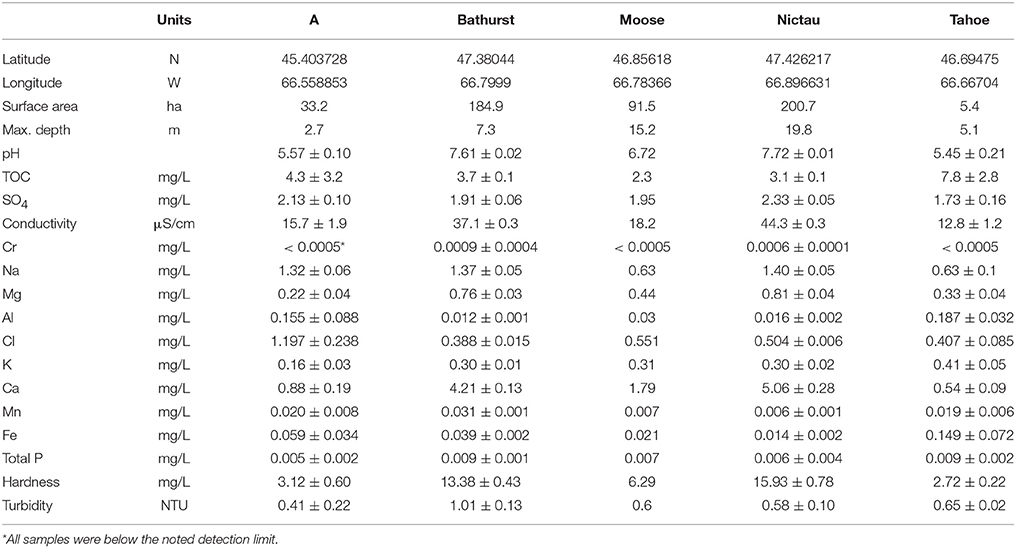
Table 1. Physical and chemical (n = 3 or 4 for all lakes but Moose) characteristics of A, Bathurst, Moose, Nictau, and Tahoe lakes in New Brunswick, Canada.
In the laboratory, macroinvertebrates were thawed and sorted in acid-washed glassware to the lowest possible taxonomic resolution [family for all but Zygoptera (suborder) and Amphipoda (order)] using Merritt and Cummins (1996) and cases (Trichoptera) were removed when present. These taxa represented a range of functional feeding groups including collectors-gatherers (Leptophlebiidae), shredders-detritivores (Limnephilidae), scrapers (Heptageniidae), and predators (Zygoptera, Aeshnidae, Chironomidae). Invertebrates were rinsed with deionized water, typically pooled within taxa to obtain adequate masses (~4–10 individuals for all taxa but chironomids; ~30–70 individuals for Chironomidae) and dates for a replicate, and freeze-dried, homogenized and subsampled for both stable isotope and MeHg analyses. Dorsal muscle from individual fish was freeze dried, homogenized and analyzed for stable isotopes and THg (a proxy for MeHg; Bloom, 1992). Otoliths from the Brook Trout were removed and used for aging as described in Finley (2013).
Stable Isotope Analysis
Individual Aeshnidae or composite (all other taxa) samples of invertebrates and muscle of individual fish were analyzed for δ13C and δ15N using either a Thermo-Finnigan Delta Plus or a Delta XP isotope-ratio mass spectrometer at the Stable Isotopes in Nature Laboratory (SINLAB) at the University of New Brunswick, Fredericton, NB. Methods are described in detail in Lescord et al. (2015). Quality assurance included three certified reference materials (sucrose (n = 3), ammonium sulfate (n = 3), nicotinamide (n = 14); International Atomic Energy Agency), and two lab working standards [bovine liver (n = 14) and smallmouth bass muscle (n = 15)], with an average deviation of 0.03‰ from the accepted values. Replicates of every 10th sample were run and precision was within 0.49 ± 0.49‰ for δ13C and 0.52 ± 0.46‰ for δ15N (n = 31). Herein we have presented only data for samples with corresponding Hg analyses. Isotope data for other littoral macroinvertebrate taxa and fishes are available in Finley (2013).
Mercury Analysis
Dried skinless dorsal muscle tissue from individual fish was analyzed for THg using atomic absorption spectrometry on a Direct Mercury Analyzer at the University of New Brunswick, Saint John, NB. Quality assurance and control for each run included analyses of standard reference materials (DORM, dogfish muscle certified reference material, run every 10 samples; TORT, lobster hepatopancreas reference material, analyzed at the beginning and the end of a run; National Research Council of Canada), one blank after every six samples, a duplicate every 10th sample, and a working laboratory standard. The mean % recovery of DORM was 88.5 ± 4.8% (range 82.0–95.6%, n = 17) and of TORT-2 was 110.0 ± 7.9% (range 98.4–129.6%, n = 24). The precision of replicate samples was 0.2 to 7.7% (n = 21). Blanks had a mean concentration of 0.002 μg/g. Sample results were not corrected for blanks or the recovery of certified reference materials. The percentage of THg as MeHg has been measured previously for these fish species in New Brunswick lakes, and ranges from 74 to 98% (Barry Hanson, 2004). Mean % moisture of Brook Trout muscle ranged from 77 to 81% across lakes.
Invertebrates were analyzed for MeHg at Acadia University, Wolfville, Nova Scotia, Canada, using basic methanol digestion, aqueous ethylation, purge and trap, desorption, and isothermal gas chromatography-atomic fluorescence spectrometry methods as described in Edmonds et al. (2012). Quality assurance for MeHg analyses included the analysis of DORM-2 (MeHg 4.47 ± 0.32 mg/kg, National Research Council of Canada), MeHg check standards (0.10%w/v, 99.999% spectral purity), triplicates analyzed on 13% of the samples, and method blanks at the start of every run and after every 10th sample. The mean recovery of DORM-2 was 93.0 ± 11.1% (range 71.8–116.0%, n = 34). Precision was measured by the relative standard deviation (%RSD) of triplicate samples and averaged 11.1 ± 6.1% (range 2.4–23.0%, n = 10). Results were corrected for method blanks.
Data Analysis
When appropriate, transformations were used to generate normally distributed data for statistical analyses and residuals were examined to ensure that transformations were valid. All statistical analysis was performed using SPSS v. 21 and Sigma Plot v. 11; α = 0.05 was used for all tests.
Comparisons of Mercury Concentrations among Brook Trout and Food Webs in New Brunswick
Condition factor of Brook Trout was calculated as follows: K = [weight (g)/length (cm)3] × 100. Lipids (indicated by C:N) tend to have more negative δ13C ratios and can confound interpretation of δ13C in whole tissues (Post et al., 2007). C:N ratios of Brook Trout muscle (3.26 to 3.44) did not warrant lipid adjustment of the δ13C data. Dietary reliance of Brook Trout on littoral vs. pelagic carbon was determined using unadjusted δ13C and a 2-source mixing model (Post, 2002). Mean δ13C for zooplankton and littoral primary consumers (pooled data for Heptageniidae, Leptohyphidae, Leptophlebiidae, Limnephilidae, where present) were the pelagic and benthic end members, respectively, and we assumed a 0.7‰ fractionation of δ13C from prey to predator based on Jardine et al. (2008). Trophic position (TP) of individual Brook Trout was calculated after adjusting their δ15N to the mean δ15N for littoral primary consumers and zooplankton from that lake based on the proportions of littoral vs. pelagic carbon in the trout's diet, and assuming a Δ15N of 3.4‰ as in Post (2002).
Among-lake differences in Brook Trout THg (log10, dw), proportion littoral carbon (calculated from δ13C), TP, condition, log10weight, and log10length were examined using ANOVA followed by Tukey's post hoc tests. Within-lake regressions were run to examine whether individual Brook Trout log THg concentrations were related to their proportion of littoral carbon or TP. Arcsine square root transformation of proportion data did not improve model residuals. A general linear mixed model was used to examine potential relationships between all individual Brook Trout log THg (dw) and their proportion of littoral carbon, TP, size (length or weight; fixed factors), and lake (random factor). Pearson correlation analyses were done to avoid including highly (>0.7) correlated variables in the models. Residuals and variance inflation factors were examined to determine the final model.
Mercury biomagnification was also quantified for each Brook Trout food web. Log10 concentrations of Hg in fish (THg - dw) and invertebrates (MeHg - dw) were regressed against their δ15N to calculate TMS (slope) values and the slopes were then compared among lakes using an ANCOVA followed by a Tukey's type test.
Comparisons of Mercury Concentrations among Salmonid Species
Data for the Brook Trout in New Brunswick were combined with data for salmonids from other regions of Canada that span a latitudinal gradient of 45 to 75°N. The lakes included 6 highly oligotrophic systems in the Canadian high Arctic (Nunavut) with only Arctic Char (Salvelinus alpinus; Lescord et al., 2015) and 14 systems from Alberta, Saskatchewan, and Ontario, Canada with Lake Trout (Salvelinus namaycush) as the top predator (Kidd et al., 2012). All lakes were sampled using similar methods and quality assurance described above and further details on analyses and their physical and chemical characteristics can be found in Kidd et al. (2012) and Lescord et al. (2015).
A principal components analysis (PCA) was run using the nine physical-chemical variables that were common across all salmonid lakes, after these variables were log10 transformed and standardized to the group mean and standard deviation (SD). Data were assessed using a varimax rotation and all components with eigen values above 0.7 were saved as variables. A rotated component matrix was used to assess the influence of each variable within a component; any loading over 0.7 was considered strong (Pallant, 2007).
Because age is a better predictor than length of Hg concentrations in slow-growing fish (Swanson and Kidd, 2010), THg levels in these fishes were standardized to a common age (5 years) using the estimated marginal means (ANCOVA model: log THg = Lake + Age + Lake*Age) within a lake. A backwards stepwise multiple regression was run using age-standardized log10 THg (logTHgage) concentrations and the components from the above-mentioned PCA, and the final regression model selection was determined using Aikake's Information Criterion (AIC). Based on Cook's Distance test for outliers, A Lake (top predator, Brook Trout) was removed from the analysis and age data were not available for Grist and Namur Lake Trout, for a total of 22 lakes within the regression.
Comparisons of Mercury Biomagnification through Diverse Food Webs
TMS values obtained for the Brook Trout lakes in New Brunswick were combined with a larger data set that included the 6 Arctic Char and 14 Lake Trout lakes above and another 11 temperate lakes in southern Nova Scotia that contain Yellow Perch (Clayden et al., 2013). A second principal component analysis (PCA) was run (as described above) using water chemistry and physical features of all 36 lakes and the resulting PCs were used in a backwards stepwise multiple regression to assess whether a combination of these physical-chemical variables predicted TMS values across these diverse freshwater systems. Because no significant models were found using this approach (see SI file), a backwards stepwise multiple regression model was run using the individual physical-chemical variables shared across all systems and the best model was determined using AIC.
Results and Discussion
Food Web Structure of the Brook Trout Lakes
Brook Trout from the New Brunswick lakes ranged in their reliance on littoral carbon from 13% in Bathurst Lake to 121% in Moose Lake (Table 2); the high value for Moose Lake is because the fish fell outside of the isotope mixing space of the end members. Brook Trout are known to shift food sources in the presence of other fish species (Magnan and Fitzgerald, 1982; Magnan, 1988; Tremblay and Magnan, 1991; Browne and Rasmussen, 2009); for example, in the presence of White Sucker, Brook Trout consumed a lower proportion of zoobenthos (Lacasse and Magnan, 1992). In the current study, Brook Trout from lakes with no other fish species (Moose and Tahoe) had a greater reliance on littoral than pelagic carbon than Bathurst and Nictau, two of the three lakes with a diverse fish community; the exception was A Lake with Brook Trout that relied mostly (86%) on littoral carbon, suggesting that interspecific competition is not the only factor responsible for between-lake differences in the energy sources supporting this species.
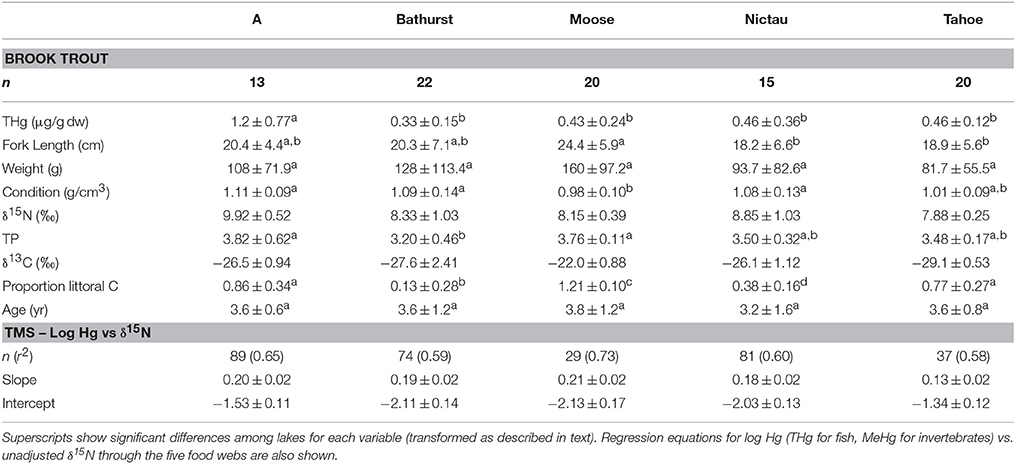
Table 2. Mean (±SD) THg, fork length, weight, condition, age, δ15N, trophic position (TP), δ13C, and proportion littoral C of Brook Trout collected from lakes in New Brunswick, Canada (see Methods for calculations of TP and proportion littoral C).
Littoral primary consumers in the New Brunswick lakes had significantly different δ15N values among lakes (ANOVA, p < 0.001), with overall within-lake means ranging from 0.82 to 3.83; as such, Brook Trout δ15N values were adjusted to baseline values as described in the methods. The TP of Brook Trout differed significantly among lakes (ANOVA, p < 0.01) but was unrelated to the composition of the fish community; mean TP for this species was 3.76 (Moose Lake) and 3.48 (Tahoe Lake) in Brook Trout only lakes, and 3.20, 3.50, and 3.82 in Bathurst, Nictau and A lakes, respectively, with diverse fish communities. This is in contrast to a study that found a relationship between the length of the food chain supporting Lake Trout and fish species richness (Vander Zanden et al., 1999). A study including more Brook Trout lakes than was examined herein is warranted to better understand how the trophic position of this species is affected by fish community composition.
Mercury Concentrations in Invertebrates
Among littoral invertebrate taxa in the New Brunswick lakes, predatory macroinvertebrates (Aeshnidae, Zygoptera) typically had the highest MeHg concentrations whereas primary consumers (Ephemeroptera, Limnephilidae) had the lowest MeHg concentrations (Table 3). In general, MeHg in these invertebrates tended to be highest in low pH and high DOC systems, as has been observed in other studies (Rennie et al., 2005; Chételat et al., 2011; Riva-Murray et al., 2011; Tsui and Finlay, 2011; Clayden et al., 2014) likely reflecting the role of these variables in promoting Hg methylation and/or bioavailability to the base of food webs. Aqueous TOC for the New Brunswick lakes were all below a DOC threshold of 8.5 mg/L observed by French et al. (2014) above which there appears to be reduced bioavailability of Hg to amphipods from Arctic lakes. In four of the lakes (A, Bathurst, Nictau, and Tahoe) from the present study, MeHg concentrations in zooplankton were higher than those of most littoral primary consumers, although sample sizes were limited. This finding supports data from mid-latitude lake of eastern North America (Chételat et al., 2011), and suggests a greater risk of MeHg accumulation for fish relying on pelagic than benthic carbon sources.
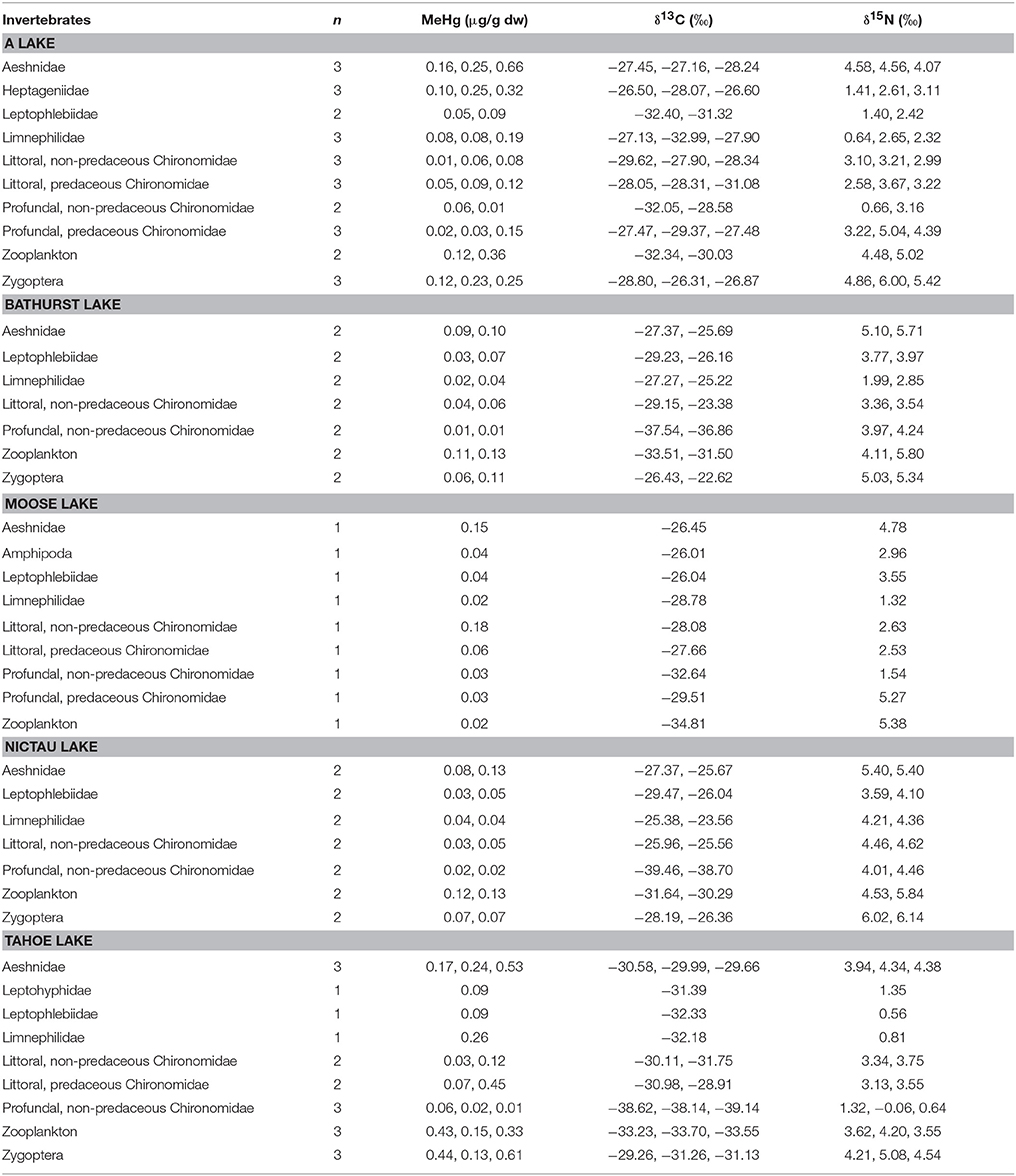
Table 3. MeHg (μg/g dw), δ13C (o), and δ15N (o) of invertebrates collected in A, Bathurst, Moose, Nictau, and Tahoe lakes, New Brunswick, Canada.
Mercury Concentrations in Brook Trout and Other Fishes
In the current study, THg concentrations in Brook Trout varied by a factor of 3–4 across lakes, despite the fish being of a similar age (Table 2). Brook Trout from A Lake had significantly higher log THg concentrations than those from other lakes (ANOVA, p = 0.001), and this trend was not explained by among-lake differences in fish size. Total Hg concentrations in Brook Trout vary across systems in northeastern North America, and tend to be higher in reservoirs than those of lakes and rivers (Kamman et al., 2005). The THg in Brook Trout filets from this large (>1100 fish) dataset averaged 0.21 μg/g ww and ranged from < 0.05 to 1.3 μg/g ww (standardized to 27.2 cm length), and their whole body concentrations of THg exceed levels of concern for fish-eating wildlife (0.16 μg/g ww) in the majority of fish collected across northeastern North America (Evers et al., 2007).
In the New Brunswick lakes, log THg of Brook Trout was positively predicted by their TP within Nictau, A and Bathurst lakes (Figure 1). Similarly, the best predictors of log THg in Brook Trout muscle across all of the New Brunswick lakes were TP and weight (general linear mixed model, log THg = –1.11 ± 0.19 + 0.26 ± 0.05 log weight + 0.16 ± 0.05 TP, 0.65; Pearson correlation of log weight vs. TP = 0.48). Many studies, including those on other trout species, report strong associations between THg concentrations and various measures of fish size (length, weight) or age, due to this element's ability to bioaccumulate (e.g., Kamman et al., 2005). Similarly, TP has long been shown to be a significant predictor of Hg in top predator fishes (e.g., Kidd et al., 1995; Vander Zanden and Rasmussen, 1996).
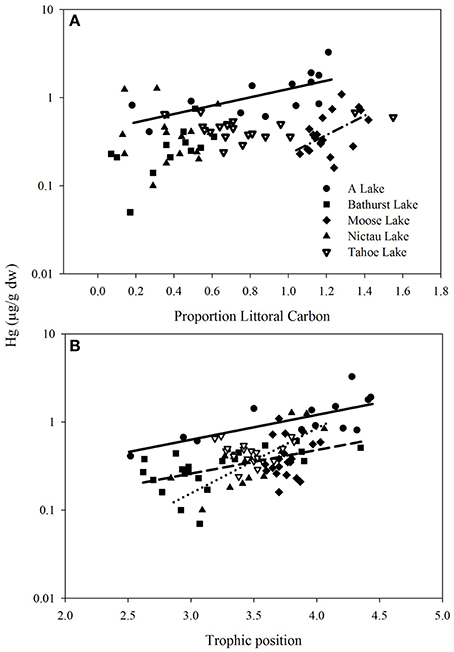
Figure 1. Total Hg (μg/g ww) vs. (A) proportion littoral carbon and (B) trophic position (see Methods for calculations) in Brook Trout from five lakes in New Brunswick, Canada. Regression lines are shown where relationships were significant. A Lake (solid line log Hg = −0.368 ± 0.147 + 0.467 ± 0.159 C, p = 0.014, r 0.38; log Hg = −1.058 ± 0.315 + 0.285 ± 0.0814 TP, p = 0.005, r 0.48), Moose Lake (dash-dot line, log Hg = −1.721 ± 0.522 + 1.082 ± 0.431 C, p = 0.04, r 0.22), Nictau Lake (dotted line, log Hg = −2.931 ± 0.604 + 0.711 ± 0.172 TP, p = 0.001, 0.53), Bathurst Lake (dashed line, log Hg = −1.393 ± 0.319 + 0.2701 ± 0.0986 TP, p = 0.013, 0.24).
Log THg of Brook Trout was predicted by their source of energy (as determined using δ13C) within two of the five lakes (A and Moose) in New Brunswick, with higher Hg concentrations in fish feeding more on littoral carbon (Figure 1). In contrast to size and trophic position effects, fewer studies have examined how Hg in fish is affected by their carbon source and results have been equivocal. For example, within a lake Eagles-Smith et al. (2008) found higher Hg in fish that relied more on benthic (littoral and profundal) than pelagic carbon, an opposite trend to that found by Chételat et al. (2013). The importance of pelagic vs. littoral carbon on Hg concentrations in fishes is likely dependent, in part, on whether these habitats support different production and uptake of MeHg to the base of the food web (as in Lindholm et al., 2014).
Among-lake differences in THg of other fish species from the New Brunswick lakes reflected the spatial patterns found for Brook Trout and the macroinvertebrates. The mean THg for other fishes ranged from a high of 2.07± 0.10 μg/g dw for Brown Bullhead from A Lake to a low of 0.23 ± 0.15 μg/g dw for White Sucker from Bathurst Lake (Table 4). Within the same species, individuals from A Lake had consistently higher THg concentrations than those from other lakes and this is likely related to the elevated MeHg concentrations in the system's invertebrates. The physical-chemical characteristics of A Lake were different from the other four lakes (Table 1), and are similar to those known to have fish with elevated Hg concentrations. More specifically, A Lake was shallower, more acidic, and smaller in surface area than the other Brook Trout lakes. Higher Hg concentrations in fishes have been found in smaller than larger lakes (Bodaly et al., 1993), and in systems with lower pH (Wiener et al., 2003).
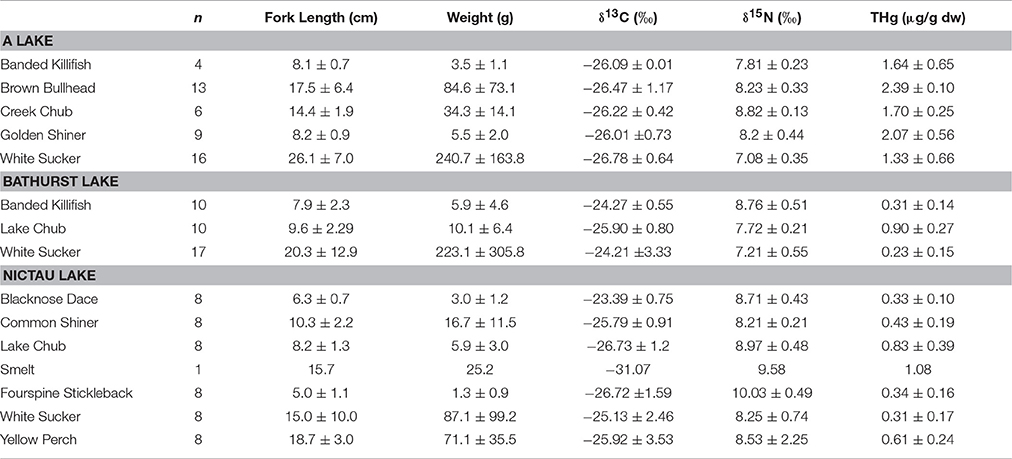
Table 4. Mean (± SD) length (cm), weight (g), δ13C (o), δ15N (o), and THg (μg/g dw) of other fishes collected from A, Bathurst, and Nictau lakes in New Brunswick, Canada.
It is interesting to note that although Tahoe Lake also had chemical characteristics that promote Hg bioavailability (e.g., lower pH) and invertebrate taxa (Aeshnidae and Zygoptera) with high MeHg concentrations, the THg of Brook Trout were similar (mean of 0.46 μg/g dw) to those for the less acidic systems (means of 0.33 to 0.46 μg/g dw) (Tables 1, 2), and the TMS was lower (see below). The reasons for this are unknown but may be related to some liming of the lake in 1998 and 2000 to increase pH (Finley, 2013). Lake liming has been suggested as a possible method to control lake pH with an overall goal of mitigating mercury bioaccumulation (Shastria and Diwekar, 2008). In other studies Hg concentrations in fish and MeHg in the water column were lower after liming, and remained lower for many years (Andersson et al., 1995; Rask and Verta, 1995; Rask et al., 2007).
THg in Salmonids across Diverse Lakes
The PCA using data for Arctic Char, Lake Trout, and Brook Trout lakes (n = 23) had components that were dominated by TP, DOC, maximum depth, and surface area (PC1), Cl−, Ca2+, and SO (PC2), and latitude and longitude (PC3). Mean THgage concentrations in the salmonid species (Table 5) were correlated with DOC (r = 0.596), latitude (r = −0.585), Cl− (r = −0.665), and Ca2+ (r = −0.543; Table S1). However, THgage in these lakes was best predicted by a combination of PC1and PC2 (multiple regression 0.45, p < 0.001; Table 6 and Table S2). PC2, dominated by ions (Ca2+, Cl−, and SO), was the strongest (r = −0.539) predictor of mean THgage concentrations across salmonid lakes (Table S3). Various ions have complex relationships with Hg bioavailability, some of which negatively impact Hg uptake by sulfate reducing bacteria and therefore methylation (Kelly et al., 2003; Daguené et al., 2012). Fish also tend to have lower Hg concentrations in lakes with high Ca2+ concentrations and pH (Lange et al., 1993). Although diet is their main pathway of MeHg exposure (Hall et al., 1997), aqueous uptake occurs (Wang et al., 2010) but can be limited by cations due to their competitive binding with Hg in gill tissues (Laporte et al., 2002; Wang and Wang, 2010).
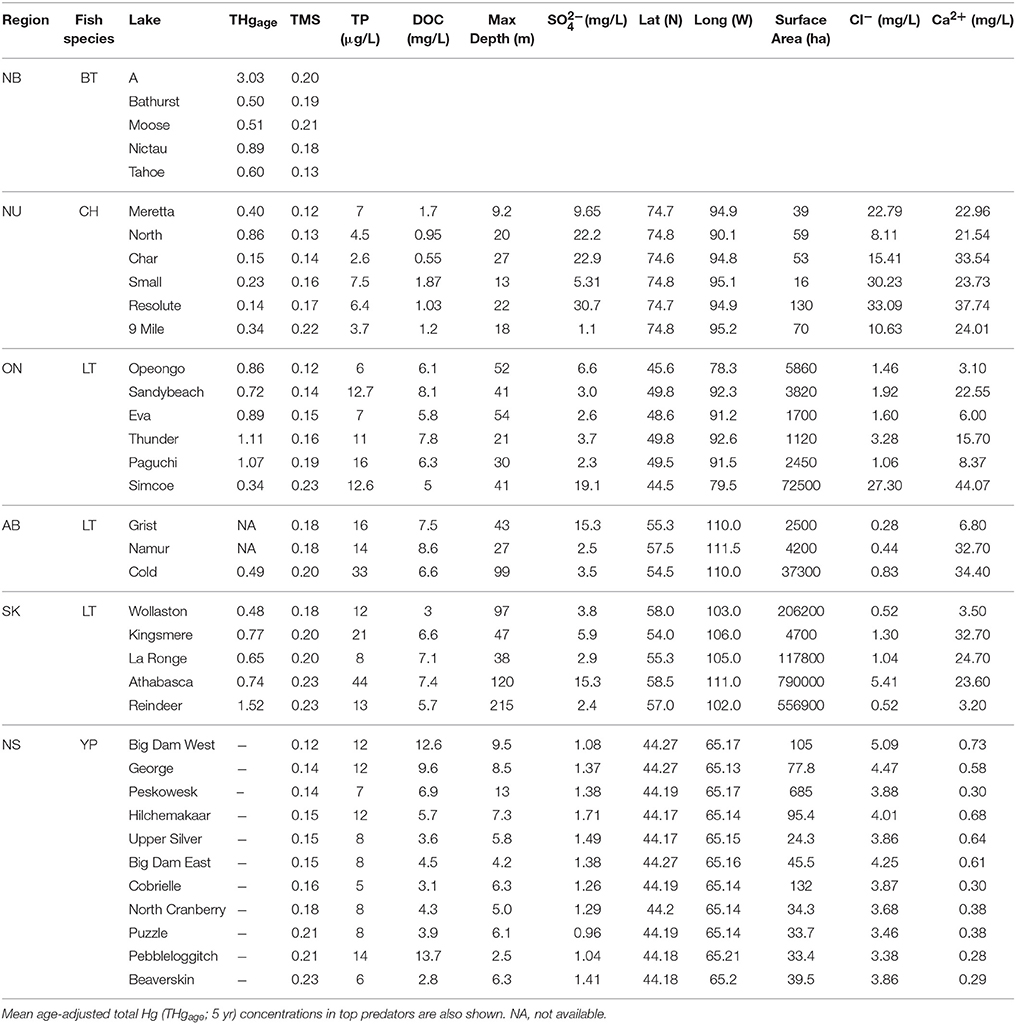
Table 5. Trophic magnification slope (TMS) and select physical and chemical characteristics of Brook Trout (BT; this study; see Table 1 for water chemistry etc. data), Arctic Char (CH), Lake Trout (LT) and Yellow Perch (YP) lakes from New Brunswick (NB), Nunavut (NU), Ontario (ON), Alberta (AB), Saskatchewan (SK), and Nova Scotia (NS) Canada (data from Kidd et al., 2012; Clayden et al., 2013; Lescord et al., 2015).
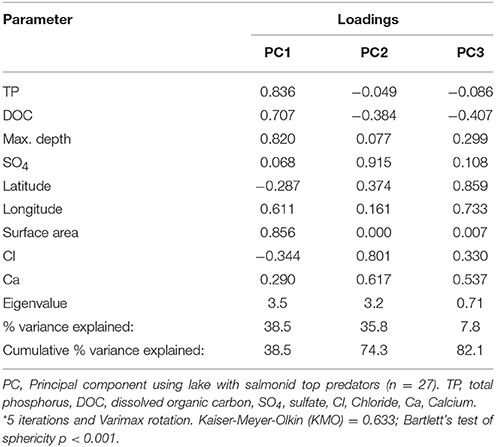
Table 6. Rotated component matrix* showing the loading strength of the physical and chemical variables common across all salmonid lakes in each Principal Component (PC) resulting from the PCA.
Mean THgage concentrations in salmonids were also positively predicted by PC1 (r = 0.478; Figure 2), which was dominated by nutrients (TP, DOC) and lake area and depth (Table 6; Table S3). Lakes with smaller surface areas tend to have warmer epilimnia and higher methylation of Hg, resulting in higher Hg concentrations in all fishes but benthivorous species (Bodaly et al., 1993). Lake depth is a determinant of thermoclines and DOC concentrations can influence oxyclines, both potentially affecting levels of anoxia and the activity of sulfate reducing bacteria that methylate Hg (Verta et al., 2010; Perron et al., 2014). Finally, more productive systems (higher TP, etc.) tend to have lower biotic Hg concentrations (Chen et al., 2005). Overall, results from the current study support earlier research demonstrating that several physical and chemical characteristics of lakes predict THg in fishes.
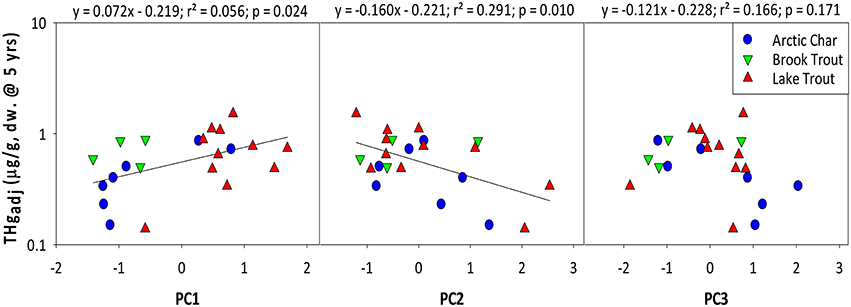
Figure 2. Relationships between age-adjusted THg concentrations (5 years) in salmonids and principal components as shown in Table 6. Brook Trout data are from the current study whereas Arctic Char and Lake Trout data are from Lescord et al. (2015) and Kidd et al. (2012), respectively.
Mercury Biomagnification in Food Webs
As observed elsewhere, δ15N was a significant predictor of Hg concentrations in each of the food webs supporting Brook Trout in New Brunswick and the TMS values ranged from 0.13 to 0.21 (Table 2). Significant differences in the slopes were found (ANCOVA, interaction term δ15N*lake p = 0.031), with Tahoe Lake having a lower slope (0.13) than the four other lakes (0.18–0.21; Figure 3).While Tahoe was one of the smallest lakes in this study, its lower slope is contrary to the results of Kidd et al. (2012) who found that smaller lakes had higher TMS. Tahoe Lake also had among the highest concentrations of MeHg in invertebrates, second only to A Lake, and was more acidic, had higher TOC, and had lower Ca2+ concentrations than all systems but A Lake. While less is known about how these factors affect TMS, they are conducive to increased MeHg production and input to the base of food webs, as discussed above and in French et al. (2014) and Rolfhus et al. (2011), and lower Hg biomagnification in fish has been observed when their prey Hg concentrations are higher (Jardine et al., 2013).
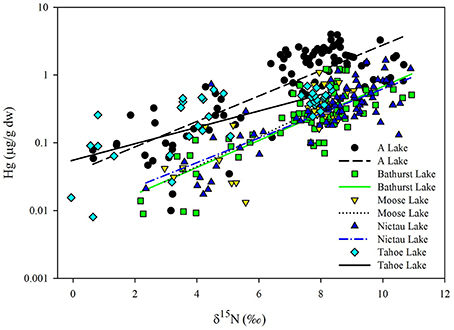
Figure 3. Regressions of log Hg (THg in fish, MeHg in invertebrates; μg/g dw) vs. unadjusted δ15N (o) for fish and invertebrates collected from A, Bathurst, Moose, Tahoe and Nictau lakes, New Brunswick. See Table 2 for regression equations.
When we combined our findings from the New Brunswick lakes supporting Brook Trout with existing data on food webs supporting Arctic Char (Lescord et al., 2015), Lake Trout (Kidd et al., 2012), and Yellow Perch (Clayden et al., 2013), there was considerable overlap in TMS values across these diverse systems (Table 5; Figure 3). Moreover, TMS values from the current study fell within the range of estimates from other freshwater, estuarine and marine food webs around the globe, with an average TMS of 0.23 across all freshwater systems (Lavoie et al., 2013).
The TMS across these Canadian food webs were not predicted by individual PCs or any combination thereof (Figures S1–S3; Tables S3, S4, S6), as was found in Lescord et al. (2015). These results are in contrast to findings of Clayden et al. (2013); TMS values across 11 Yellow Perch lakes were negatively correlated with a PC dominated by aqueous nutrients (Ca, DOC, TN, TP), MeHg and chloride, suggesting that water quality influences the food web biomagnification of Hg, albeit through yet unknown mechanisms. These Yellow Perch lakes are acidic and relatively low in nutrients (aqueous TP of 6 to 12 μg/L), representing a more limited range of systems than those presented in the current study (e.g., aqueous TP of 2.6 to 44 μg/L). It is possible that the lack of some water chemistry data (i.e., water pH, MeHg) for all of the lakes in the current study limited the ability to use PCs to predict TMS values.
When individual physical-chemical variables (TP, DOC (or TOC), SO, surface area, and Cl−; latitude, longitude, depth, and Ca2+ were excluded due to tolerance values <0.2; Tabachnick and Fidell, 2007) were entered into a backward stepwise multiple regression, the best model for TMS across all lakes included only TP (p = 0.028, 0.109; Figure 4; Table S5) a positive but weak relationship was observed. In a broader synthesis, aqueous pH, TP and DOC explained about 25% of the variability of TMS values across diverse freshwater systems (n = 44; Lavoie et al., 2013). Interestingly, TMS values are either higher (this study, Kidd et al., 2012; Verburg et al., 2014) or lower (Clayden et al., 2013; Lavoie et al., 2013) in lakes with greater TP for reasons that are unknown and warrant further investigation. While there are numerous studies in the literature that have quantified the trophic transfer of Hg using δ15N (e.g., Rolfhus et al., 2011), most have not examined whether the TMS values are related to system characteristics.
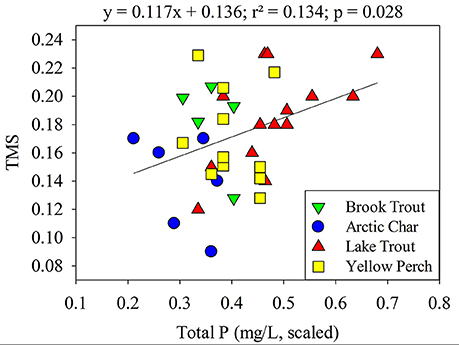
Figure 4. Relationship between TMS values and scaled total phosphorous (mg/L) of lakes. Brook Trout data are from the current study whereas Yellow Perch, Arctic Char, and Lake Trout data are from Clayden et al. (2013), Lescord et al. (2015), and Kidd et al. (2012), respectively.
In summary, results from this study on Brook Trout in New Brunswick lakes support the paradigms that trophic position, source of dietary carbon, and size affect concentrations of this toxic element in fish and that higher Hg is found in biota from acidic systems, and provide new knowledge on the magnitude of Hg biomagnification through lacustrine food webs supporting Brook Trout. In addition, there was some—albeit weak—evidence in a broader dataset that the trophic transfer (TMS) of Hg is higher in lakes with greater nutrients (i.e., TP), as has been seen previously. The mechanisms behind the influence of water chemistry variables on TMS values, however, remain speculative and may be direct or indirect. Further comparisons are warranted because of the known effects of MeHg on top predator fishes and their consumers and the lack of studies with consistent experimental designs and/or the supporting information needed to assess broader trends and to improve our understanding of systems at greatest risk (Lavoie et al., 2013).
Author Contributions
MF conducted the field work, analyzed, and interpreted the data and co-wrote the manuscript. KK co-designed the study, analyzed and interpreted the data and co-wrote the manuscript. AC and NO co-designed the study and assisted with data interpretation and writing. MC and GL conducted data analysis and co-wrote the manuscript.
Funding
This project was undertaken with the financial support of the Government of Canada through the Federal Department of Environment (#1114181), the New Brunswick Environmental Trust Fund (#090162), the New Brunswick Wildlife Trust Fund (F008-001), the Natural Sciences and Engineering Research Council Discovery Program (#312237-2012-RGPIN), and the Canada Research Chairs Program (211803). Logistical and in-kind support was received from the New Brunswick Departments of Environment and Local Government and Natural Resources, International Pulp and Paper, Mount Carleton Provincial Park, and the A Lake Gun Club. We thank Heidi Swanson, Leanne Baker, Angella Mercer, Ben Salmon, Mark Gautreau, Michelle Dobrin, Tim Barrett, and John O'Keefe for field and lab assistance, and the reviewers for constructive comments on an earlier version of this manuscript.
Conflict of Interest Statement
The authors declare that the research was conducted in the absence of any commercial or financial relationships that could be construed as a potential conflict of interest.
Supplementary Material
The Supplementary Material for this article can be found online at: http://journal.frontiersin.org/article/10.3389/fenvs.2016.00023
References
Andersson, P., Borg, H., and Karrhage, P. (1995). Mercury in fish muscle in acidified and limed lakes. Water Air Soil Pollut. 80, 889–892.
Barry Hanson, E. (2004). Mercury Concentrations and Trophic Interactions of Fish Species in Southwestern New Brunswick lakes. M.Sc. Thesis, University of New Brunswick, Canada.
Bloom, N. S. (1992). On the chemical form of mercury in edible fish and marine invertebrate tissue. Can. J. Fish. Aquat. Sci. 49, 1010–1017.
Bodaly, R. A., Rudd, J. W. M., Fudge, R. J. P., and Kelly, C. A. (1993). Mercury concentrations in fish related to size of remote Canadian Shield lakes. Can. J. Fish. Aquat. Sci. 50, 980–987.
Boudou, A., and Ribeyre, F. (1997). “Mercury in the food web: accumulation and transfer mechanisms,” in Metal Ions in Biological Systems Vol. 34: Mercury and its Effects on Environment and Biology, eds A. Sigel and H. Sigel (New York, NY: Marcel Dekker) 89–319.
Browne, D. R., and Rasmussen, J. B. (2009). Shifts in the trophic ecology of brook trout resulting from interactions with yellow perch: an intraguild predator-prey interaction. Trans. Am. Fish. Soc. 138, 1109–1122. doi: 10.1577/T08-113.1
Burgess, N. M., and Meyer, M. W. (2008). Methylmercury exposure associated with reduced productivity in common loons. Ecotoxicology 17, 83–91. doi: 10.1007/s10646-007-0167-8
Chasar, L. C., Scudder, B. C., Stewart, A. R., Bell, A. H., and Aiken, G. R. (2009). Mercury cycling in stream ecosystems 3. Trophic dynamics and methylmercury bioaccumulation. Environ. Sci. Technol. 43, 2733–2739. doi: 10.1021/es8027567
Chen, C. Y., Stemberger, R. S., Kamman, N. C., Mayes, B. M., and Folt, C. L. (2005). Patterns of Hg bioaccumulation and transfer in aquatic food webs across multi-lake studies in the northeast US. Ecotoxicology 14, 135–147. doi: 10.1007/s10646-004-6265-y
Chételat, J., Amyot, M., and Garcia, E. (2011). Habitat-specific bioaccumulation of methylmercury in invertebrates of small mid-latitude lakes in North America. Environ. Pollut. 159, 10–17 doi: 10.1016/j.envpol.2010.09.034
Chételat, J., Cloutier, L., and Amyot, M. (2013). An investigation of enhanced mercury bioaccumulation in fish from offshore feeding. Ecotoxicology 22, 1020–1032. doi: 10.1007/s10646-013-1087-4
Clayden, M. G., Kidd, K. A., Chételat, J., Hall, B. E., and Garcia, E. (2014). Environmental, geographic and trophic influences on methylmercury concentrations in macroinvertebrates from lakes and wetlands across Canada. Ecotoxicology 23, 273–284. doi: 10.1007/s10646-013-1171-9
Clayden, M. G., Kidd, K. A., Wyn, B., Kirk, J., Muir, D. C. G., and O'Driscoll, N. (2013). Mercury biomagnification through food webs is affected by physical and chemical characteristics of lakes. Environ. Sci. Technol. 21, 12047–12053. doi: 10.1021/es4022975
Daguené, V., McFall, E., Yumvihoze, E., Xian, S., Amyot, M., and Poulain, A. J. (2012). Divalent base cations hamper HgII uptake. Environ. Sci. Technol. 46, 6645–6653. doi: 10.1021/es300760e
Deison, R., Smol, J. P., Kokelj, V., Pisaric, M. F. J., Kimpe, L. E., Poulain, A. J., et al. (2012). Spatial and temporal assessment of mercury and organic matter in thermokarst affected lakes of the Mackenzie Delta Uplands, NT, Canada. Environ. Sci. Technol. 46, 8748–8755. doi: 10.1021/es300798w
Depew, D. C., Basu, N., Burgess, N. M., Campbell, L. M., Devlin, E. W., Drevnick, P. E., et al. (2012). Toxicity of dietary methylmercury to fish: Derivation of ecologically meaningful threshold concentrations. Environ. Toxicol. Chem. 31, 1536–1547. doi: 10.1002/etc.1859
Drevnick, P. E., and Sandheinrich, M. B. (2003). Effects of dietary methylmercury on reproductive endocrinology of fathead minnows. Environ. Sci. Technol. 37, 4390–4396. doi: 10.1021/es034252m
Driscoll, C. T., Blette, V., Yan, C., Schofield, C. L., Munson, R., and Holsapple, J. (1995). The role of dissolved organic-carbon in the chemistry and bioavailability of mercury in remote Adirondack lakes. Water Air Soil Pollut. 80, 499–508.
Eagles-Smith, C. A., Suchanek, T. H., Colwell, A. E., and Anderson, N. L. (2008). Mercury trophic transfer in a eutrophic lake: the importance of habitat-specific foraging. Ecol. Appl. 18 (8 Suppl.), A196–A212. doi: 10.1890/06-1476.1
Edmonds, S. T., O'Driscoll, N. J., Hillier, N. K., Atwood, J. L., and Evers, D. C. (2012). Factors regulating the bioavailability of methylmercury to breeding rusty blackbirds in northeastern wetlands. Environ. Pollut. 171, 148–154. doi: 10.1016/j.envpol.2012.07.044
Ethier, A. L. M., Scheuhammer, A. M., and Bond, D. E. (2008). Correlates of fish mercury in fish from lakes near Clyde Forks, Ontario, Canada. Environ. Pollut. 154, 89–97. doi: 10.1016/j.envpol.2008.01.009
Evers, D. C., Han, Y. J., Driscoll, C. T., Kamman, N. C., Goodale, M. W., Lambert, K. F., et al. (2007). Biological mercury hotspots in the northeastern United States and southeastern Canada. Bioscience 57, 29–43. doi: 10.1641/B570107
Finley, M. (2013). The Effects of Fish Community Complexity on Mercury Bioaccumulation on Lake Food Webs Supporting Brook Trout (Salvelinus fontinalis). M.Sc. Thesis, University of New Brunswick, Canada.
French, T. D., Houben, A. J., Desforges, J. W., Kimpe, L. E., Kokelj, S. V., Poulain, A. J., et al. (2014). Dissolved organic carbon thresholds affect mercury bioaccumulation in Arctic Lakes. Environ. Sci. Technol. 48, 3162–3168. doi: 10.1021/es403849d
Gabriel, M. C., Kolka, R., Wickman, T., Nater, E., and Woodruff, L. (2009). Evaluating the spatial variation of total mercury in young-of-year yellow perch (Perca flavescens), surface water and upland soil for watershed-lake systems within the southern Boreal Shield. Sci. Total Environ. 407, 4117–4126. doi: 10.1016/j.scitotenv.2009.03.019
Greenfield, B. K., Hrabik, T. R., Harvey, C. J., and Carpenter, S. R. (2001). Predicting mercury levels in yellow perch: use of water chemistry, trophic ecology, and spatial traits. Can. J. Fish. Aquat. Sci. 58, 1419–1429. doi: 10.1139/cjfas-58-7-1419
Haitzer, M., Aiken, G. R., and Ryan, J. N. (2003). Binding of mercury(II) to aquatic humic substances: influence of pH and source of humic substances. Environ. Sci. Technol. 37, 2436–2441. doi: 10.1021/es026291o
Hall, B. D., Bodaly, R. A., Fudge, R. J. P., Rudd, J. W. M., and Rosenberg, D. M. (1997). Food as the dominant pathway of methylmercury uptake by fish. Water Air Soil Pollut. 100, 13–24.
Hanna, D. E., Solomon, C. T., Poste, A. E., Buck, D. G., and Chapman, L. J. (2015). A review of mercury concentrations in freshwater fishes of Africa: patterns and predictors. Environ. Toxicol. Chem. 34, 215–223. doi: 10.1002/etc.2818
Jardine, T. D., Chernoff, E., and Curry, R. A. (2008)., Maternal transfer of carbon and nitrogen to progeny of sea-run and resident brook charr (Salvelinus fontinalis). Can. J. Fish Aquat. Sci. 65, 2201–2210. doi: 10.1139/F08-132
Jardine, T. D., Kidd, K. A., and O'Driscoll, N. (2013). Food web analysis reveals effects of pH on mercury bioaccumulation at multiple trophic levels in streams. Aquat. Toxicol. 132–133, 46–52. doi: 10.1016/j.aquatox.2013.01.013
Jardine, T. D., Kidd, K. A., and Rasmussen, J. B. (2012). Terrestrial organic matter in the diet of stream consumers: implications for mercury bioaccumulation. Ecol. Appl. 22, 843–855. doi: 10.1890/11-0874.1
Kainz, M., Lucotte, M., and Parrish, C. C. (2002). Methyl mercury in zooplankton - the role of size, habitat, and food quality. Can. J. Fish. Aquat. Sci. 59, 1606:1615. doi: 10.1139/F02-125
Kamman, N. C., Burgess, N. M., Driscoll, C. T., Simonin, H., Goodale, W., Linehan, J., et al. (2005). Mercury in freshwater fish of northeast North America - A geographic perspective based on fish tissue monitoring databases. Ecotoxicology. 14, 163–180. doi: 10.1007/s10646-004-6267-9
Kelly, C. A., Rudd, J. W. M., and Holoka, M. H. (2003). Effect of pH on mercury uptake by an aquatic bacterium: Implications for Hg cycling. Environ. Sci. Technol. 37, 2941–2946. doi: 10.1021/es026366o
Kidd, K. A., Hesslein, R. H., Fudge, R. J. P., and Hallard, K. A. (1995). The influence of trophic level as measured by d15N on mercury concentrations in freshwater organisms. Water Air Soil Pollut. 80, 1011–1015.
Kidd, K. A., Muir, D. C. G., Evans, M. S., Wang, X., Whittle, M., Swanson, H. K., et al. (2012). Biomagnification of mercury through lake trout (Salvelinus namaycush) food webs of lake with different physical, chemical and biological characteristics. Sci. Total Environ. 438, 135–143 doi: 10.1016/j.scitotenv.2012.08.057
Lacasse, S., and Magnan, P. (1992). Biotic and abiotic determinants of the diet of Brook Trout, Salvelinus fontinalis, in lakes of the Laurentian Shield. Can. J. Fish Aquat. Sci. 49, 1001–1009.
Lange, T. R., Royals, H. E., and Connor, L. L. (1993). Influence of water chemistry on mercury concentration in largemouth bass from Florida lakes. Trans. Am. Fish. Soc. 122, 74–84.
Laporte, J., Andres, S., and Mason, R. P. (2002). Effect of ligands and other metals on the uptake of mercury and methylmercury across the gills and the intestine of the blue crab (Callinectes sapidus). Comp. Biochem. Physiol. Part C 131, 185–196. doi: 10.1016/s1532-0456(01)00289-7
Lavoie, R. A., Jardine, T. D., Chumchal, M. M., Kidd, K. A., and Campbell, L. M. (2013). Biomagnification of mercury in aquatic food webs: a worldwide meta-analysis. Environ. Sci. Technol. 47, 13385–13394. doi: 10.1021/es403103t
Lescord, G. L., Kidd, K. A., Kirk, J. L., O'Driscoll, N. J., Wang, X., and Muir, D. C. G. (2015). Factors affecting biotic mercury concentrations and biomagnification through lake food webs in the Canadian high Arctic. Sci. Total Environ. 509, 195–205. doi: 10.1016/j.scitotenv.2014.04.133
Lindholm, M., De Wit, H. A., Eriksen, T. E., and Braaten, H. F. V. (2014). The influence of littoral on mercury bioaccumulation in a humic lake. Water Air Soil Pollut. 225, 2141. doi: 10.1007/s11270-014-2141-4
Magnan, P., and Fitzgerald, G. J. (1982). Resource partitioning between brook trout (Salvelinus-fontinalis Mitchill) and creek chub (Semotilus atromaculatus Mitchill) in selected oligotrophic lakes of southern Quebec. Can. J. Zool. 60, 1612–1617.
Magnan, P. (1988). Interactions between Brook Charr, Salvelinus fontinalis, and nonsalmonid species: ecological shift, morphological shift, and their impact on zooplankton communities. Can. J. Fish. Aquat. Sci. 45, 999–1009.
Mason, R. P., Laporte, J. M., and Andres, S. (2000). Factors controlling the bioaccumulation of mercury, methylmercury, arsenic, selenium, and cadmium by freshwater invertebrates and fish. Arch. Environ. Contam. Toxicol. 38, 283–297. doi: 10.1007/s002449910038
Merritt, R. W., and Cummins, K. W. (1996). An Introduction to the Aquatic Insects of North America 3rd Edn. Dubuque, IA: Kendall/Hunt Publishing Company.
Miskimmin, B. M., Rudd, J. W. M., and Kelly, C. A. (1992). Influence of dissolved organic carbon, pH, and microbial respiration rates on mercury methylation and demethylation in lake water. Can. J. Fish. Aquat. Sci. 49, 17–22. doi: 10.1139/f92-002
Pallant, J. (2007). SPSS Survival Manual: A Step by Step Guide to Data Analysis using SPSS for Windows, 3rd Edn. Berkshire: Open University Press.
Perron, T., Chètelat, J., Gunn, J., Beisner, B. E., and Amyot, M. (2014). Effects of experimental thermocline and oxycline deepening on methylmercury bioaccumulation in a Canadian Shield lake. Environ. Sci. Technol. 48, 2626–2634. doi: 10.1021/es404839t
Post, D. M., Layman, C. A., Arrington, D. A., Takimoto, G., Quattrochi, J., and Montana, C. G. (2007). Getting to the fat of the matter: models, methods and assumptions for dealing with lipids in stable isotope analyses. Oecologia 152, 179–189. doi: 10.1007/s00442-006-0630-x
Post, D. M. (2002). Using stable isotopes to estimate trophic position: models, methods and assumptions. Ecology 83, 703–718. doi: 10.1890/0012-9658(2002)083[0703:USITET]2.0.CO;2
Rask, M., Jones, R. I., Jarvinen, M., Paloheimo, A., Salonen, M., Syvaranta, J., et al. (2007). Changes in fish mercury concentrations over 20 years in an acidified lake subject to experimental liming. Appl. Geochem. 22, 1229–1240. doi: 10.1016/j.apgeochem.2007.03.015
Rask, M., and Verta, M. (1995). Concentrations and amounts of methylmercury in water and fish in the limed and acid basins of a small lake. Water Air Soil Pollut. 80, 577–580. doi: 10.1007/BF01189708
Ravichandran, M. (2004). Interactions between mercury and dissolved organic matter—-a review. Chemosphere 55, 319–331. doi: 10.1016/j.chemosphere.2003.11.011
Rennie, M. D., Collins, N. C., Purchase, C. F., and Tremblay, A. (2005). Predictive models of benthic invertebrate methylmercury in Ontario and Quebec lakes. Can. J. Fish. Aquat. Sci. 62, 2770–2783. doi: 10.1139/f05-181
Riva-Murray, K., Chasar, L. C., Bradley, P. M., Burns, D. A., Brigham, M. E., Smith, M. J., et al. (2011). Spatial patterns of mercury in macroinvertebrates and fishes from streams of two contrasting forested landscapes in the eastern United States. Ecotoxicology 20, 1530–1542. doi: 10.1007/s10646-011-0719-9
Rolfhus, K. R., Hall, B. D., Monson, B. A., Paterson, M. J., and Jeremiason, J. D. (2011). Assessment of mercury bioaccumulation within the pelagic food web of lakes in the western Great Lakes region. Ecotoxicology 20, 1520–1529. doi: 10.1007/s10646-011-0733-y
Sandheinrich, M. B., Bhavsar, S. P., Bodaly, R. A., Drevnick, P. E., and Paul, E. A. (2011). Ecological risk of methylmercury to piscivorous fish of the Great Lakes region. Ecotoxicology 20, 1577–1587. doi: 10.1007/s10646-011-0712-3
Sandheinrich, M. B., and Miller, K. M. (2006). Effects of dietary methylmercury on reproductive behavior of fathead minnows (Pimephales promelas). Environ. Toxicol. Chem. 25, 3053–3057. doi: 10.1897/05-641R.1
Scheuhammer, A. M., Lord, S. I., Wayland, M., Burgess, N. M., Champoux, L., and Elliot, J. E. (2016). Major correlates of mercury in small fish and common loons (Gavia immer) across four large study areas in Canada. Environ. Pollut. 210, 361–370. doi: 10.1016/j.envpol.2016.01.015
Scott, W. B., and Scott, M. G. (1988). Atlantic Fishes of Canada. Toronto, ON: University of Toronto Press in cooperation with the Minister of Fisheries and Oceans and the Canadian Govt. Pub. Centre.
Shastria, Y., and Diwekar, U. (2008). Optimal control of lake pH for mercury bioaccumulation control. Ecol. Modell. 216, 1–17. doi: 10.1016/j.ecolmodel.2008.03.019
Simonin, H. A., Loukmas, J. J., Skinner, L. C., and Roy, K. A. (2008). Lake variability: key factors controlling mercury concentrations in New York State fish. Environ. Pollut. 154, 107–115. doi: 10.1016/j.envpol.2007.12.032
Swanson, H. K., and Kidd, K. A. (2010). Mercury concentrations in Arctic food fishes reflect presence of anadromous Arctic charr (Salvelinus alpinus), species, and life history. Environ. Sci. Technol. 44, 3286–3292. doi: 10.1021/es100439t
Tabachnick, B. G., and Fidell, L. S. (2007). Using Multivariate Statistics. 5th Edn. Toronto, ON Pearson.
Tremblay, S., and Magnan, P. (1991). Interactions between 2 distantly related species, Brook Trout (Salvelinus fontinalis) and white sucker (Catostomus commersoni). Can. J. Fish. Aquat. Sci. 48, 857–867. doi: 10.1139/f91-102
Tsui, M. T. K., and Finlay, J. C. (2011). Influence of dissolved organic carbon on methylmercury bioavailability across Minnesota stream ecosystems. Environ. Sci. Technol. 45, 5981–5987. doi: 10.1021/es200332f
Vander Zanden, M. J., and Rasmussen, J. B. (1996). A trophic position model of pelagic food webs: Impact on contaminant bioaccumulation in lake trout. Ecol. Monogr. 66, 451–477. doi: 10.2307/2963490
Vander Zanden, M. J., Shuter, B. J., Lester, N., and Rasmussen, J. B. (1999). Patterns of food chain length in lakes: a stable isotope study. Am. Nat. 154, 406–416. doi: 10.1086/303250
Verburg, P., Hickey, C. W., and Phillips, N. (2014). Mercury biomagnification in three geothermally-influenced lakes differing in chemistry and algal biomass. Sci. Total Environ. 493:342–354. doi: 10.1016/j.scitotenv.2014.05.097
Verta, M., Salo, S., Korhonen, M., Porvari, P., Paloheimp, A., and Munthe, J. (2010). Climate induced thermocline change has an effect on the methyl mercury cycle in small boreal lakes. Sci. Total Environ. 408, 3639–3647. doi: 10.1016/j.scitotenv.2010.05.006
Wang, R., and Wang, W. (2010). Importance of speciation in understanding mercury bioaccumulation in tilapia controlled by salinity and dissolved organic matter. Environ. Sci. Technol. 44, 7964–7969. doi: 10.1021/es1011274
Wang, R., Wong, M., and Wang, W. (2010). Mercury exposure in the freshwater tilapia Oreochromis niloticus. Environ. Pollut. 158, 2694–2701. doi: 10.1016/j.envpol.2010.04.019
Watras, C. J., Back, R. C., Halvorsen, S., Hudson, R. J. M., Morrison, K. A., and Wente, S. P. (1998). Bioaccumulation of mercury in pelagic freshwater food webs. Sci. Total Environ. 219, 183–208. doi: 10.1016/S0048-9697(98)00228-9
Keywords: stable isotopes, food webs, mercury, trophic magnification slope, brook trout
Citation: Finley MLD, Kidd KA, Curry RA, Lescord GL, Clayden MG and O'Driscoll NJ (2016) A Comparison of Mercury Biomagnification through Lacustrine Food Webs Supporting Brook Trout (Salvelinus fontinalis) and Other Salmonid Fishes. Front. Environ. Sci. 4:23. doi: 10.3389/fenvs.2016.00023
Received: 02 December 2015; Accepted: 14 March 2016;
Published: 06 April 2016.
Edited by:
Sergi Sabater, University of Girona, SpainReviewed by:
Vera I. Slaveykova, University of Geneva, SwitzerlandKaren Riva Murray, U.S. Geological Survey, USA
Copyright © 2016 Finley, Kidd, Curry, Lescord, Clayden and O'Driscoll. This is an open-access article distributed under the terms of the Creative Commons Attribution License (CC BY). The use, distribution or reproduction in other forums is permitted, provided the original author(s) or licensor are credited and that the original publication in this journal is cited, in accordance with accepted academic practice. No use, distribution or reproduction is permitted which does not comply with these terms.
*Correspondence: Karen A. Kidd, a2lkZGtAdW5iLmNh