- 1Department of Chemistry, Université de Montréal, Montréal, QC, Canada
- 2Centre de Recherche Du CHU de Québec–Université Laval, Institut de Biologie Intégrative et des Systèmes (IBIS), Département de Médecine Sociale et Préventive, Université Laval, Québec City, QC, Canada
- 3Centre Québécois sur La Santé des Animaux Sauvages, Canadian Wildlife Health Cooperative, Faculté de Médecine Vétérinaire, Université de Montréal, Montréal, QC, Canada
Limited data are available for the occurrence of more recent per and polyfluorinated alkyl substances (PFAS) in marine mammals, especially from the St. Lawrence Estuary and Gulf. This study investigates the occurrence of PFAS in liver and muscle tissues of various marine mammals, including the harbor seal, gray seal, harp seal, hooded seal, harbor porpoise, white-sided dolphin, white-beaked dolphin, and True’s beaked whale. Among the 80 target PFAS (including PFCAs, PFSAs, Cyclic PFSA, FASAs/FASAAs, FTCAs/FTUCAs, FTSAs, Ether-PFAS, diPAPs, and ESI + ECF precursors) perfluorooctanoic sulfonate (PFOS) dominates in all the marine mammal species and several other long-chain PFCAs, such as PFNA, PFDA, PFUnA, PFTrDA, and PFHxDA, were detected at 100% frequency in both muscle and liver samples. PFDoA and 7:3 fluorotelomer carboxylic acid (7:3 acid) also showed a 100% detection frequency for liver samples. Harp seal tissues displayed notably low PFAS concentrations, with average total PFAS concentrations of 7 ng/g (ww: wet weight) in muscle and 44 ng/g (ww) in the liver. In contrast, the white-sided dolphin exhibited the highest average concentrations, reaching 39 ng/g (ww) in muscle and 334 ng/g (ww) in liver samples. The Pearson correlation analysis reveals a strong correlation between the concentration of PFOS, perfluoroalkyl carboxylic acids (PFCAs), and electrochemical fluorination (ECF) precursors. Species at the top of the marine food chain (harbor porpoise, white sided dolphin, and white beaked dolphin) presented the highest concentrations of PFAS, particularly PFOS and long-chain PFCAs, highlighting the need for an increased regulation of these persistent molecules in order to protect marine mammal’s health.
1 Introduction
Per and polyfluorinated alkyl substances (PFAS) encompass a wide range of synthetic chemicals, now recognized as potent environmental contaminants, colloquially known as “forever chemicals” (Sciancalepore et al., 2021; Helmer et al., 2022; Bilela et al., 2023). They have gained widespread use owing to their unique properties, including amphiphilic characteristics and robust covalent bonds. These chemicals find extensive applications in various industries, including food packaging, cosmetics, pharmaceuticals, firefighting foams, electronics, and paints (Sciancalepore et al., 2021; Sadia et al., 2023). Understanding the fate and environmental distribution of PFAS is vital for revealing the various processes by which PFAS discharge and migration occur, including surface water runoff, atmospheric and vapour transport, infiltration into the subsurface, etc. By comprehensively studying these pathways, environmental experts can better grasp how PFAS contaminants move through different environmental compartments, aiding in the development of effective mitigation and remediation strategies to tackle PFAS pollution (Bilela et al., 2023; Macorps et al., 2023; Manojkumar et al., 2023; Munoz et al., 2023; Sadia et al., 2023).
The presence of several PFAS has been reported in various environmental matrices such as groundwater, surface water, sediments, and marine biota (Bilela et al., 2023; Macorps et al., 2023; Manojkumar et al., 2023; Munoz et al., 2023; Sadia et al., 2023). The primary sources of PFAS in marine aquatic environments are typically associated with PFAS production sites, such as chemical industries, as well as improper disposal practices, often originating from nonpoint sources and runoff from landfills containing PFAS (Bilela et al., 2023). Inland activities are another significant contributor to PFAS pollution in marine ecosystem as they can introduce PFAS into the marine environment through riverine discharges. Additionally, volatile PFAS can be transported through the atmosphere, serving as another major source of PFAS contamination in marine and terrestrial environments (Bilela et al., 2023; Haque et al., 2023).
Among the categories of PFAS, perfluorooctanesulfonic acid (PFOS) and perfluorooctanoic acid (PFOA) are generally referred to as “legacy PFAS” (Manojkumar et al., 2023). Various countries and regions have taken action to ban or limit the usage of PFAS (Sosnowska et al., 2023; Klingelhöfer et al., 2024). However, due to the scarcity of viable alternatives for essential applications, exceptions have been granted for the utilization of PFOS in specific instances, notably in AFFFs (aqueous film-foaming foams) utilized for firefighting purposes. PFOS came under control in 2007, followed by PFOA and PFHxS in 2019 and 2022, respectively (Stockholm Convention, 2007; Stockholm Convention, 2023). The long chain PFCA regulation process is presently underway on a global scale. In an effort to phase out nonessential usage of PFAS, the EU has imposed limitations on some long chain PFCAs (European Chemical Agency, 2023) and also in Canada (2016) Minister of environment published in the Canada Gazette). The purpose of this restriction is to reduce or eliminate PFCA exposure to humans and the environment. In 2021, long chain PFCAs and their precursors have also been proposed by the Canadian government for inclusion in the Stockholm Convention (Stockholm Convention, 2022). The Stockholm convention has allowed exemptions for planned or ongoing regulatory actions related to long chain PFCAs in response to economic challenges. This includes potential financial strains associated with transitioning away from the use of specific substances and the impact on industries and economies dependent on these chemicals (Stockholm Convention, 2023). It is worth emphasizing that a number of PFAS compounds, including the GenX chemicals (a group used as substitutes for PFOA and PFOS), FTOH (fluorotelomer alcohol) and PFBS (perfluorobutane sulfonate), a shorter-chain PFAS used in various applications, remain for the most part unregulated (Stockholm Convention, 2007; Stockholm Convention, 2022; Stockholm Convention, 2023, Stockholm Convention, 2023).
The presence of PFAS in aquatic ecosystems can lead to their bioaccumulation in freshwater and marine food chains, including invertebrates, fish, and marine mammals (Bilela et al., 2023). Several studies have already unveiled the detrimental effects of PFAS on the reproductive, endocrine, and immune systems of aquatic organisms (Hamid et al., 2023).
Because of their environmental persistence and bioaccumulative properties, PFAS are transferred through various trophic levels (Cara et al., 2022; Bilela et al., 2023; Navarathna et al., 2023) such as primary consumers (herbivores), secondary consumers (carnivores), and tertiary consumers (top predators), leading to markedly higher concentrations in marine animals at the top of the food chains (Cara et al., 2022; Bilela et al., 2023; Navarathna et al., 2023). Despite being largely regulated, PFOS levels measured in the tissues of marine mammals worldwide remain elevated, including in Arctic and Antarctic wildlife (Casas et al., 2023; Xie and Kallenborn, 2023). Moreover, an increasing trend for long chain PFCAs has been observed in marine mammals from many regions including finless porpoises from South China sea (Guo et al., 2023) and ringed seals, polar bears and killer whales from East Greenland (Gebbink et al., 2016).
According to numerous investigations, the highest concentrations of PFAS are found in the livers followed by kidneys of fish and marine mammals including Indo-Pacific humpback dolphins and finless porpoises (Cara et al., 2022; Kah and Kookana, 2023; Liu et al., 2023). The interactions between proteins and PFAS are intimately linked to the underlying molecular mechanism of PFAS bioaccumulation over lifetime and liver and renal tissues are significant accumulation sites for PFAS due to their high binding affinity with liver type fatty acid-binding proteins (LFABP) and serum albumin (Han et al., 2003; Woodcroft et al., 2010; Sheng et al., 2016; Cheng et al., 2021).
Although many studies have documented PFAS concentrations in marine mammals in the Arctic and the Antarctic (Casas et al., 2023; Xie and Kallenborn, 2023), there is a limited information in marine mammals from more southern latitudes, including those living in the St-Lawrence Estuary and Gulf, Quebec, Canada. The St. Lawrence River in Quebec, Canada, has been grappling with contamination issues since 2012 (Giroux et al., 2016), particularly from certain PFAS, notably perfluoroalkyl sulfonates (PFSA) (Munoz et al., 2022). A comprehensive study delved into the presence of 60 PFAS in the St. Lawrence river’s freshwater species near a major urban area, revealing significantly higher PFAS in smallmouth bass compared to species lower in the food chain (Kaboré et al., 2022; Munoz et al., 2022). Moreover, research highlighted distinctive fluorochemical markers in fish from the St. Lawrence River, indicating the higher concentrations of PFAS such as PFBA, PFOS, PFOA, FASA and long chain PFCAs (Kaboré et al., 2022; Munoz et al., 2022). Because of its geographic significance in marine ecosystems, research on PFAS contamination in marine mammals in the St. Lawrence River is essential because it provides insights into the spread and fate of PFAS. Evaluating PFAS levels in a variety of species, such as dolphin and harbor seals, can help assess potential effects and health of the ecosystem. To manage health hazards, it is imperative for humans to understand the fate and resulting tissue concentrations of PFAS, especially for individuals who consume hunted species like harp and gray seal. This information may influence environmental regulations aimed at mitigating PFAS pollution, thereby promoting preservation and long-term administration of the marine ecosystem in the St. Lawrence River.
Employing an analytical approach targeting 80 distinct PFAS, the objective of the present study is to investigate the exposure levels to PFAS in various marine mammals living in the St. Lawrence River (Estuary and Gulf), such as harbor seal, gray seal, harp seal, hooded seal, harbor porpoise, white-sided dolphin, white-beaked dolphin, and True’s beaked whale, to provide insights into the potential impact of these substances on the health of the marine mammal’s population of this area. On top of the effects on the health of the sea mammals, we are also considering the potential risks associated with the consumption of marine mammal muscle and liver by human populations when the species are allowed for commercial, subsistence or recreative hunting (i.e., gray and harp seal), PFAS concentrations in both liver and muscle were documented.
2 Materials and methods
2.1 Chemical and standards
The certified standards of PFAS were purchased from Wellington Laboratories (Guelph, ON, Canada), Synquest Laboratories (Alachua, FL, United States), and DuPont (Wilmington, DE, United States). Details on PFAS (shown in Table 1), internal standards, and details on other chemicals used in this study are provided in the supplementary information.
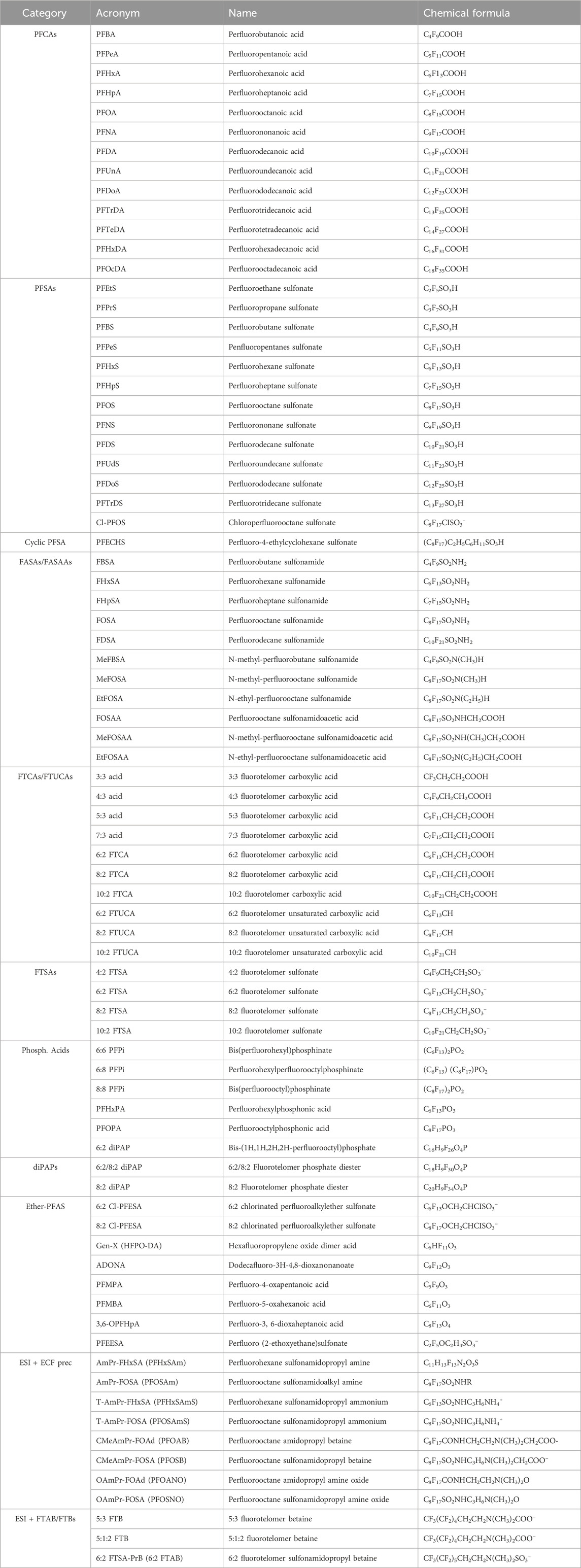
Table 1. List of 80 PFAS quantitatively targeted in the present study, including acronyms, and name.
2.2 Sample collections
Samples were collected from marine mammals [harbor seal (Phoca vitulina, n = 5), gray seal (Halichoerus grypus, n = 2), harp seal (Pagophilus groenlandicus, n = 1), hooded seal (Cystophora cristata, n = 1), harbor porpoise (Phocoena Phocoena, n = 22), white-sided dolphin (Lagenorhynchus acutus, n = 6), white-beaked dolphin (Lagenorhynchus albirostris, n = 1), and True’s beaked whale (Mesoplodon mirus, n = 1)] found dead ashore along the St. Lawrence Estuary and Gulf, Canada. The details of each species body weight, sex, sampling location site and total PFAS concentrations are shown in Supplementary Tables S4, S5 in the Supplementary Materials. The sampling locations are shown in Figure 1. These carcasses were transported, usually frozen, by the Réseau québécois d’urgences pour les mammifères marins to the Canadian Wildlife Health Cooperative Quebec Regional Center where sampling and a full necropsy were performed.
The St. Lawrence River has a mean annual flow of 12,600 cubic meters per second and an expansive watershed exceeding one million square kilometres, ranks as the second most voluminous river in North America. Spanning a length of 400 km, the St. Lawrence River and Estuary display a dynamic environment, ranging in width from a few kilometers near Quebec City to over 25 km downstream. While the maximum seafloor depth reaches 60 m, numerous shallower areas with depths less than 10 m also exist. The St. Lawrence River/Estuary and Gulf is marked by intricate seafloor topography. St. Lawrence River/Gulf is a important estuary located at the mouth of St. Lawrence River, serving as a meeting for freshwater and saltwater. Multiple physical forces, such as tides, waves, wind, ship waves, and ice, contribute to the notable dynamisms observed in this region (Vis et al., 1998; Hudon and Carignan, 2008).
2.3 Sample preparation
Sample preparation was performed as follows: prior to analysis, a 50 mg of freeze-dried aliquot of the marine specimen’s sample was weighed in a 15-mL polypropylene (PP) tube. The samples were spiked with surrogate internal standards, and a 1-h waiting time was applied. Subsequently, 5 mL of MeOH was added as the extraction solvent, followed by high-speed vertexing (3,200 rpm, 0.5 min), ultrasonication in ice-cold water (10 min), centrifugation (6,000 rpm, 5 min), and transfer of the supernatant to a fresh 15-mL PP tube (triplicate samples). The combined supernatants were concentrated to 1.8 mL and subjected to cleanup with Supelclean ENVI-Carb cartridges (250 mg). Finally, the prepared samples were aliquoted for analysis in LCMS vials (200 µL).
QA/QC was conducted for each LC/MS batch sequence using in house protocol. Matrix matched calibration curves all had determination coefficient (R2 > 0.99). Method blanks are injected in the sequence and low level of specific PFAS. Recoveries results are included in the supplementary information (Supplementary Table S5).
2.4 Instrumental analysis
Analysis was carried out using ultra high-performance liquid chromatography high resolution mass spectrometry (UHPLC-HRMA Q-Exactive Orbitrap, Thermo Scientific, Waltham, MA, United States). The scan range was m/z 150–1,000, with a resolution setting of 700 00 FWHM at m/z 200. Details on the acquisition method of UHPLC-HRMS are mostly similar to Carrizo et al. (2023) and are provided in the supporting information. In both ionization techniques, we used the same mobile phase. The method limit of detection (mLOD) and corresponding Instrumental limits of detection (iLOD) are shown in Supplementary Table S8. For the diPAPs analysis, samples were analyzed by ultra-high-performance liquid chromatography coupled to tandem mass spectrometry (UP-HPLC-MS/MS) and analytical details are shown in the supplementary information.
2.5 Statistical analysis
The statistical software IBM SPSS software (version 27; Armonk, Ny, United States) was employed to analyze concentrations (geometric mean, standard deviation, minimum, maximum) of PFAS in muscle and liver samples. If every assumption of the model was satisfied, including the linearity of residual, homogeneity of variances, and normal distribution, the Pearson correlation coefficient (r) was utilized. Parametric students T tests for independent samples were utilized to assess whether the mean concentration of PFAS exhibited differences based on body weight and sex. As for the comparative statistical analysis two factors were chosen body weight and sex.
To examine the relationships between data, Principal Component analysis (PCA) and cluster analysis were used. The objectives of PCA, a technique for reducing dimensionality, is to identify the most important basis for re-expressing data, thereby reducing noise and revealing underlying patterns.
3 Results and discussion
3.1 PFAS concentration and distribution in muscle and liver samples of marine mammals
The sum of the 80 PFAS measured in the 38 specimens included in our study varied from 0.03 to 64 ng/g ww for muscle and 0.03–535 ng/g ww for liver samples. The highest average total PFAS concentration was observed in the white sided dolphin (secondary consumers) for muscle and liver samples (39 ng/g ww for muscle and 333 ng/g ww for liver samples), whereas very low concentration was found in the harp seal (high trophic level, 7 ng/g ww for muscle and 44 ng/g ww for liver samples), as shown in Figure 2. Total average PFAS concentrations in muscle and liver tissues across all marine mammal specimens analysed were significantly correlated (Spearman’s r = 0.39, p > 0.05, n = 38). The substantial correlation found in the PFAS concentrations between the liver and muscle tissues of marine mammals suggest that the distribution and accumulation of PFAS within these organs are closely related. This link implies that PFAS are carried and dispersed systematically throughout the body after being consumed, resulting in detectable amounts in the liver and muscle tissues. This illustrates that PFAS exposure and accumulation are not confined to a single tissue type and has significant bioaccumulation in marine mammals.
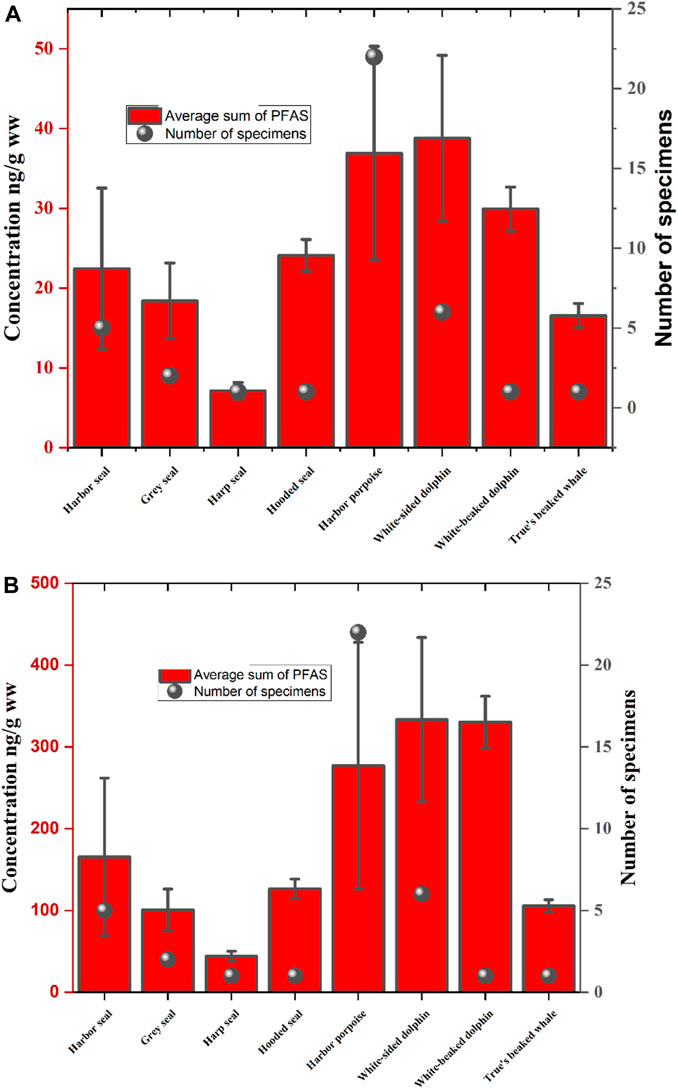
Figure 2. (A) Average sum of 80 PFAS in muscle samples with number of analyzed specimen; (B) Average sum of 80 PFAS in liver samples with number of specimens analyzed.
From the results we observed that marine mammals at elevated trophic levels accumulate PFAS through distinct mechanisms. This means that PFAS accumulation varies among these mammals due to factors such as varying concentrations of PFAS in preys, which affects dietary intake; different metabolic rates, which influence elimination speed; biomagnification, which results in higher concentration in apex predators. PFAS preferentially accumulate in specific tissues, like the liver and physiological differences in liver function and binding proteins can affect bioaccumulation and genetic variations can also have an impact on PFAS transport, distribution, and metabolism (Spaan et al., 2020; Haque et al., 2023). Harbor porpoise, white sided dolphin, and white beaked dolphin consistently showed higher accumulation of PFAS. The average ratio of the liver and muscle samples are also shown in the supplementary information (Supplementary Table S7), and as expected, the greatest bioaccumulation was systematically found in the liver when compared to the muscle tissues (all ratios >1). However, a substantial disparity in the liver to muscle ratios was found between species, with an average ratio of 76, and the highest mean ratio for 7:3 Acid in white beaked dolphin (ratio of 22.1) followed by PFOS in harp seal (ratio of 20.1).
PFAS compounds with the detection frequency (D.F) above 10 are detailed in Tables 2 and 3 for muscle and liver samples by species respectively. Out of 80 target PFAS, we detected 33 PFAS in muscle and 41 PFAS in liver tissues. The detected groups of PFAS include PFCAs (including PFOA branch isomers, Long chain PFCAs include eight carbon atoms or more, whereas short chain PFCAs normally contain less than eight), perfluoroalkyl sulfonic acids (PFOS, PFHxS, and PFHps), emerging PFAS (it refers to recently found PFAS and short-chain PFAAs that are used to replace older PFAS) and PFAS intermediates or precursors (includes fluorotelomer sulfonates, fluorotelomer alcohols and side chain fluorinated polymers). The PFAS compounds chemical stability and lipophilic characteristics help explain the reason they persist in the environment and biomagnify within the food chain (Spaan et al., 2020). Due to their carbon-fluorine linkages, long chain PFCAs exhibit excellent chemical and thermal stability, allowing them to remain in the environment for extended periods (Spaan et al., 2020). This is clear in the increased concentrations observed in the liver samples compared to muscle samples, indicating that their lipophilic tails facilitate bioaccumulation in the lipid rich tissue organism (Spaan et al., 2020).
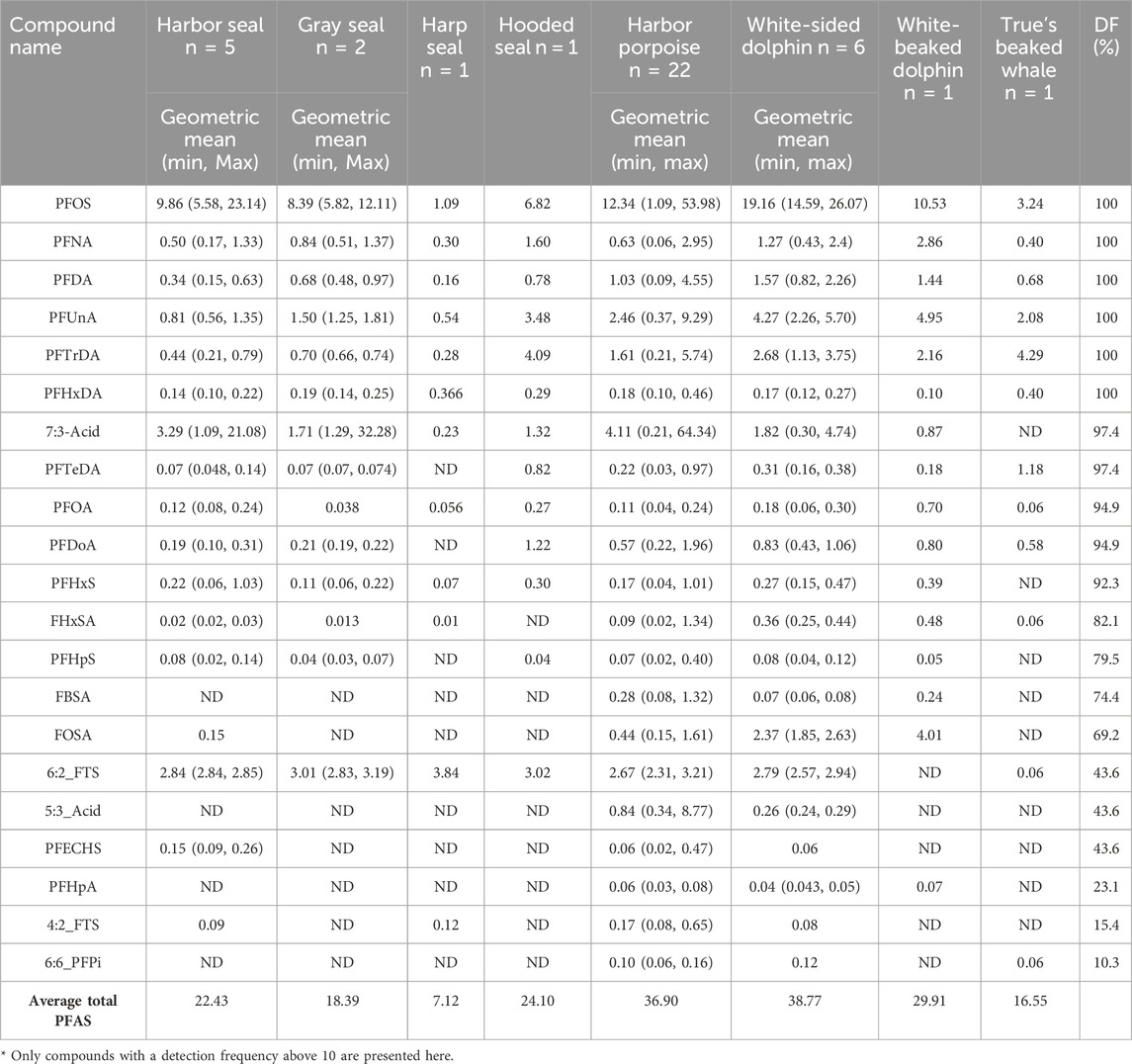
Table 2. PFAS concentration ranges of muscle samples (min, max, Geometric mean, ng/g wet weight) in harbor seal, gray seal, harp seal, hooded seal, harbor porpoise, white-sided dolphin, white-beaked dolphin, and True’s beaked whale.
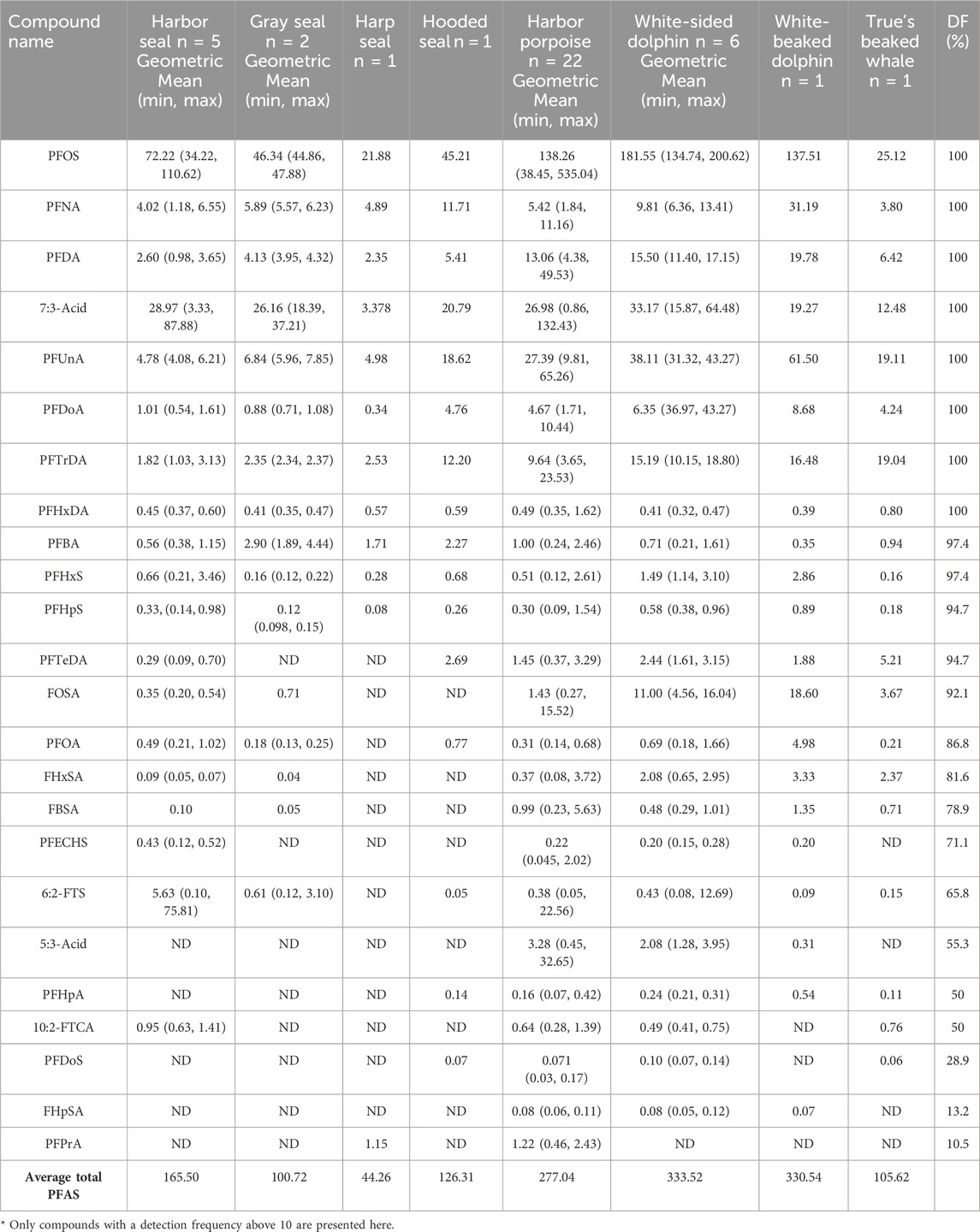
Table 3. PFAS concentration ranges of liver samples (min, max, Geometric mean, ng/g wet weight) in Harbor seal, Gray seal, Harp seal, Hooded seal, Harbor porpoise, White-sided dolphin, White-beaked dolphin, and True’s beaked whale.
PFOS presented the highest average concentrations in both muscle and liver samples across all species, as previously observed in most wildlife and human exposure studies (Arctic monitoring and assessment programme, 2016). This is probably caused by the sulfonate functional group and long carbon chain, which increases the molecular binding affinity to serum proteins and causes bioaccumulation. The observation that PFAS accumulate more consistently in the liver than in the muscle tissue is consistent with PFAS chemical tendency to attach to fatty acid binding proteins and albumin (Spaan et al., 2020). A 100% detection frequency was also observed among all species for other long chain PFCAs such as PFNA, PFDA, PFUnA, PFTrDA, and PFHxDA in both muscle and liver samples. In liver samples, 7:3-Acid and PFDoA also exhibited a 100% detection frequency. Positive correlations were found between muscle and liver tissues across all species for PFNA (p = 0.03, rSpearman = 0.46), PFOS (p = 0.006, rSpearman = 0.43), and PFUnA (p < 0.001, rSpearman = 0.63).
As shown in Table 2, the maximum concentration of PFAS in muscle tissue was detected for 7:3 acid in a harbor porpoise sample (64 ng/g ww). In other species, the maximum concentrations were reported for PFOS and in the following order: white-sided dolphin (26 ng/g ww), harbor seal (23 ng/g ww), gray seal (12 ng/g ww), white-beaked dolphin (11 ng/g ww, n = 1), hooded seal (6.8 ng/g ww, n = 1), True’s beaked whale (3.2 ng/g ww, n = 1), and harp seal (1.1 ng/g ww, n = 1).
As detailed in Table 3, PFOS was the congener with the highest maximum values in liver samples across all species and in the following order: harbor porpoise (535 ng/g ww), white-sided dolphin (201 ng/g ww), white-beaked dolphin (138 ng/g ww), harbor seal (110 ng/g ww), gray seal (48 ng/g ww), hooded seal (45 ng/g ww), True’s beaked whale (25 ng/g ww), and harp seal (22 ng/g ww).
The geometric mean concentration of PFOS in liver samples of the white sided dolphin, as observed in this study, is 182 ng/g ww. Similar concentrations were reported in livers of bottlenose dolphin specimens in recent studies conducted in the North Adriatic sea in Italy (194 ng/g ww) (Sciancalepore et al., 2021), and in the Western Mediterranean Sea (211 ng/g ww). Positive correlations were found between muscle and liver tissues across all species for PFNA (p = 0.03, rSpearman = 0.46), PFOS (p = 0.006, rSpearman = 0.43), PFUnA (p < 0.001, rSpearman = 0.63), and PFTrDA (p < 0.001 rSpearman = 0.383).
The physicochemical properties of certain PFAS, such as chain length and functional groups, may have an impact on their distribution, as suggested by variations in liver to muscle ratios and varying bioaccumulation of PFAS among species. These characteristics may have an impact on the mobility and solubility of PFAS in aquatic habitats, which may result in exposure pathways that are unique to certain sea mammal species. Further investigation into the mechanism of PFAS bioaccumulation in these species is needed, as the positive correlation between muscle and liver tissues that have been discovered indicate a systematic uptake and distribution pattern in these marine mammals.
3.2 Relative PFAS composition and concentration
The distribution of average PFAS concentrations (%) in the muscle and liver samples of each species are shown in Figures 3, 4. For muscle tissues, the largest total average concentration of PFAS was observed in harbor porpoise, white sided dolphin, and white beaked dolphin. The results indicated that PFOS is the predominant PFAS in all the species, with the highest average percentage found in the gray seal (49%), white sided dolphin (48%), and harbor seal (48%). In harp seal, 6:2 FTS was 89% higher than PFOS, while in True’s beaked whale, PFTrDA was approximately 28% higher than PFOS. Conversely, in the liver samples, the highest percentage of PFOS concentration was found in white sided dolphin (55%), and harbour porpoise (56%), followed by harp seal (50%), followed by gray seal (49%). Apart from PFOS, long chain PFCAs (PFUnA, PFTrDA, and PFNA) are quite dominant in the liver samples, except for 6:2 FTS in harbor seal. Both PFOS and long-chain exhibit significant bioaccumulative and biomagnification tendencies in the food chain, as observed in other marine mammal studies (Martin et al., 2004; Gebbink et al., 2016; Cara et al., 2022). Notably, 6:2 FTS is widely used for the replacement in the AFFF (Langberg et al., 2019). The liver serves as the primary organ for the buildup of PFAS in marine animals because it is a highly perfused organ that detoxifies harmful chemicals. Long chain PFAS can partition into the liver tissue more preferentially than other organ due to their lipophilic nature and low water solubility. In comparison to short chain PFAS, long chain PFCAs and PFSAs have a larger potential for bioaccumulation (Savoca and Pace, 2021; Khan et al., 2023; Wang et al., 2023). Their larger octanol-water partition coefficient (Kow) cause more bioconcentration and biomagnification higher up the food chain, because they are apex predators and so at the highest trophic levels, marine mammals also accumulate the highest levels of PFAS through food web biomagnification and nutritional intake (Savoca and Pace, 2021; Khan et al., 2023; Wang et al., 2023).
Besides PFAS, 7:3 Acid (7:3 fluorotelomer carboxylic acid) was frequently detected in harbor seal, hooded seal, harbor porpoise, and white sided dolphins. This molecule serves as the primary stable byproducts of the aerobic and anaerobic biodegradation of fluorotelomer alcohols. Previous reports indicated the presence of this compound in harbor seals (64%) and harbor porpoise (71%) from the US Atlantic coast. In the current analysis, a maximum concentration of 132 ng/g ww was observed. The third highest occurrence of PFAS was observed for 6:2 FTS for harbor seal in liver samples. This compound is widely used as an alternate PFAS for various applications, mainly for electroplating industry (Liu et al., 2022). Recent studies show that this compound is highly toxic and bioaccumulative in aquatic system (Lu et al., 2017). The PFAS 6:2 FTS is primarily used as an alternative to PFOS and has been identified in polar regions as well (Xie and Kallenborn, 2023).
In addition to PFAS, significant percentages of 7:3 acid, PFUnA, and PFTrDA were found in muscle samples. The harbor seal and harbor porpoise species were found to have high levels of 7: 3 acid. PFTrDA percentages were higher in beaked whales and hooded seals. A high relative percentage of the long chain PFUnA was observed in the following species: white beaked dolphin (17%, mean concentration, 5.0 ng/g ww), white sided dolphin (11%, mean concentration, 4.5 ng/g ww), hooded seal (14%, mean concentration, 3.5 ng/g ww), and True’s beaked whale (16%, mean concentration, 2.1 ng/g ww). One recent study has shown that PFUnA occurrence was frequently found in the fish samples from European subalpine lakes in an urbanized region (Rüdel et al., 2022). The concentration ranges and patterns are comparable to those found in the present investigation. Studies shown that PFUnA concentration was dominant in marine mammals from East Greenland. For example, PFUnA concentration was found to be dominant in Killer whales at 168 ng/g and in polar bears at 252 ng/g. In white beaked and white sided dolphins, the major contribution of PFAS were linearly structured, such as PFOS, PFUnA, PFTrDA, and FOSA. Again, similar patterns were previously reported in stranded common dolphins from New Zealand (Stockin et al., 2021). The seals from Greenland have highest fraction of these compounds (Spaan et al., 2020).
Previous studies have indicated a high distribution of PFOA and PFBA in the St. Lawrence River. In our study, the detection frequency of PFOA was 94.9% in muscle samples and 86.8% in liver samples. High concentrations were observed in white beaked dolphins, with 0.7 ng/g ww in muscle and 4.98 ng/g ww in liver samples. For PFBA in liver samples, the detection frequency was 97.4%, with the maximum concentration observed in gray seal, reaching 4.44 ng/g ww. PFAS compounds, including the class of PFSA and FASA, are prevalent in the St. Lawrence River in Quebec, Canada. However, our research revealed a lower presence of these compounds in marine mammals. This can be attributed to various factors. It has been observed that PFAS compounds tend to accumulate and magnify in food webs. Nevertheless, the specific distribution and accumulation of PFAS compounds can vary based on factors like the unique traits of the organisms and the structure of the food chain in particular environment (Munoz et al., 2022; Brunn et al., 2023).
The accumulation of PFAS is influenced by a number of factors, including geographic location, local pollution, and industrial discharge (Zhang et al., 2019; Melake et al., 2022; Guo et al., 2023). They are not equally distributed among the different marine species. When compared to muscle tissue, the liver has a higher concentration of PFAS, the majority of which are PFOS and long chain PFCAs. Numerous investigations have demonstrated that marine mammals are increasingly exposed to newer PFAS. For example, a study on marine mammals from South China Sea revealed significantly rising trends in several specific PFAS, indicating an increase in their concentration over time (Lam et al., 2016). This pattern could be explained using some PFAS rising after regulatory limits were put in place on others. Furthermore, a study conducted on bottlenose dolphin in the northern Adriatic Sea revealed the durability and harmful effects of certain PFAS (Sciancalepore et al., 2021), raising questions about their impact on marine mammals. In terms of their relative toxicity and contribution, emerging PFAS are increasingly causing concern, despite potentially minimal contributions to the overall levels of major PFOS and long chain PFAS. The evidence suggests that due to their potential toxicity (including adverse effects on immune system, liver function, reproductive health, hormone level), emerging PFAS are indeed becoming more prevalent in marine mammals (Lam et al., 2016; Sciancalepore et al., 2021; Ma et al., 2022)
The concentration of perfluoroalkyl carboxylic acids (PFCA), such as PFTeDA, PFTrDA, PFNA, and PFDA, as well as PFOS, and electrochemical fluorination (ECF) precursors, such as FBSA, FHxSA, and FOSA were significantly correlated in liver samples (Figure 5). These results point to a high correlation between marine mammal’s exposure sources to certain PFAS chemicals.
3.3 Clustering of PFAS contamination in marine mammals
In our research, we conducted a PCA cluster analysis focusing only on harbor porpoise, harbor seal, and white sided dolphin because there sample size of the other mammals was too small for PCA (see Figure 6). Through this analytical approach, we gained valuable insights into the distribution and grouping patterns of these three marine mammal’s species based on the presence of PFAS. The results of our study clearly indicate that their significant difference in the occurrence of PFAS among the harbor porpoise, harbor seal, and white sided dolphin. The PCA analysis indicates that harbor porpoises and white sided dolphin exhibit intriguing characteristics (Law et al., 2008; Stockin et al., 2021). One potential explanation for the elevated levels observed could be the distinct feeding behaviours observed in these species. Harbor porpoise, which typically inhabit nearshore areas, may be more exposed to PFAS contaminated preys found in coastal regions (Law et al., 2008). Conversely, white sided dolphins are known for their high mobility and may cover larger areas, potentially encountering PFAS sources in offshore settings (Stockin et al., 2021).
Understanding the varying contamination levels among different species in marine ecosystem involves investigating their habitats and tracking PFAS sources, such as industrial discharge or air deposition (Law et al., 2008; Lynch et al., 2019; Stockin et al., 2021). Elevated PFAS concentration in white sided dolphin and harbor porpoise populations may suggest broader ecological implications, emphasizing the importance of comprehensive monitoring and conservation efforts to address this concern.
3.4 Occurrence of perfluoroalkyl carboxylic acid (PFCA) in marine mammals
The concentrations of PFCAs in liver samples from marine mammals are shown in Figure 7. We investigated PFCAs, which are carboxylic acid analogues with organofluorine counterparts with chain lengths ranging from 8 to 12 carbons. Significantly, every sample that was analyzed showed a regular pattern in which the chain lengths alternated between odd and even values. A noteworthy discovery in that dataset showed that the amounts of PFCAs with odd and even chain lengths was found to be lower than that of PFCAs with odd chain lengths when looking at the liver samples of harbor seal, harp seal, white sided dolphin, and white beaked dolphin.
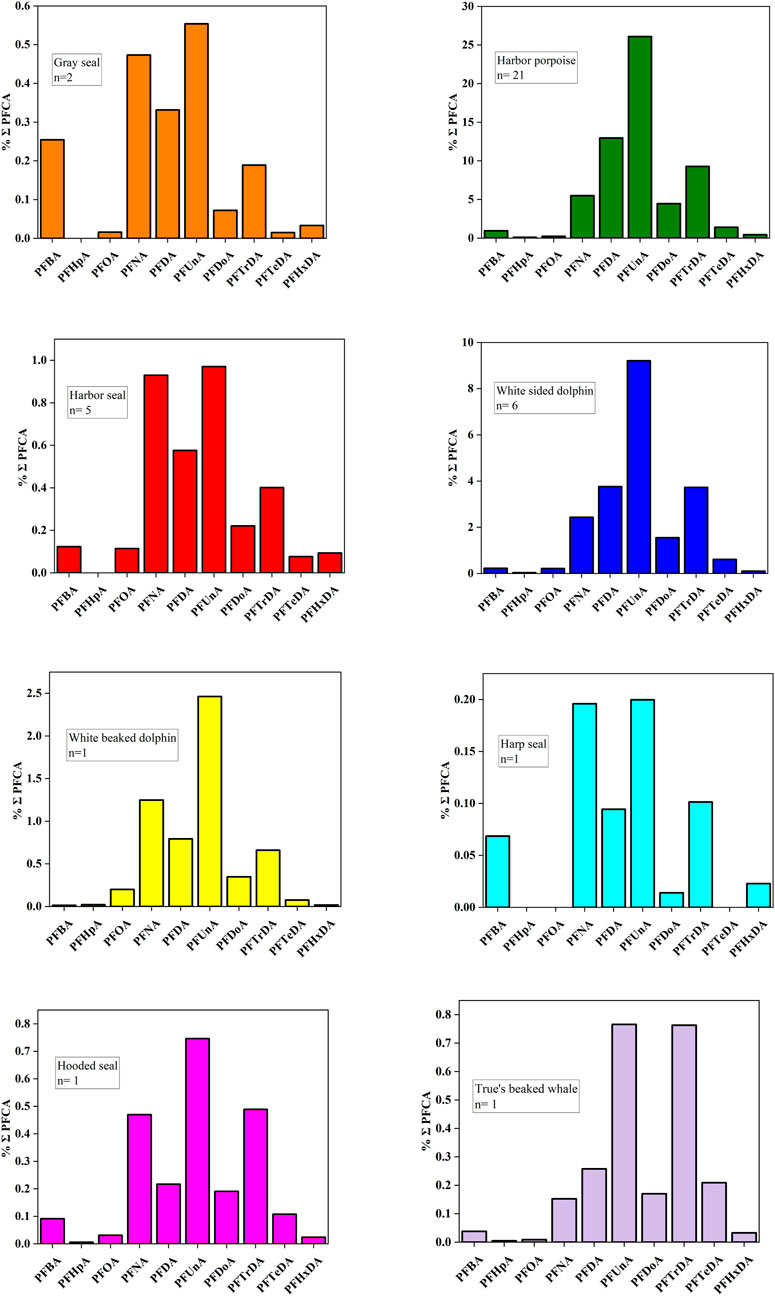
Figure 7. Average percentage contribution of liver samples of PFCAs to ΣPFCA concentrations in gray seal, harbor porpoise, harbor seal, white-sided dolphin, white baked dolphin, harp seal, hooded seal, and True’s beaked whale.
For instance, within each studied species, PFUnA is higher in all the species and PFNA was consistently detected at higher levels than PFOA. Examining the particular species in further detail, like hooded seals and True’s beaked whales, our data showed an interesting pattern. In these species, the concentrations of PFTrDA were consistently higher than those of PFDA and PFTeDA. Considering all the species, PFHxDA concentrations are comparatively lower compared to other PFCAs.
It is interesting to consider the mechanisms and processes determining the bioaccumulation and distribution of these compounds in various organisms in light of the reported disparity in the amounts of PFCAs with odd and even chain lengths in PFCAs across various species. The specific variations in concentrations provide crucial new insights for future studies on the dynamics of PFCA in diverse ecological settings.
Similar types of patterns have already been reported in marine mammals in earlier studies (Spaan et al., 2020). The peculiar chemical reactions leading to the formation of PFCAs are responsible for the phenomenon wherein odd chains end up having higher concentrations during the atmospheric oxidation of fluorotelomer alcohols (Spaan et al., 2020). Using this technique, PFCAs with both even and odd chain lengths can be produced. Specifically, PFCAs with odd numbered carbon chains are generated through fluorotelomer olefin based (C9-) yields (Spaan et al., 2020). This outcome results from the processes and chemical structures involved in the PFCAs, encompassing both even and odd numbers of carbon chains during the atmospheric oxidation of fluorotelomer alcohols (Spaan et al., 2020).
In our study, we observed a very diverse distribution of PFCA homologues among different species, even though the consistent odd or even chain pattern was observed. These variations could potentially be clarified by considering the unique metabolism of each species. For example, other than PFUnA, PFNA (C9) was the dominant PFCA in gray seal, harp seal, harbor seal, and white beaked dolphin, while PFDA (C10) in harbor porpoise. Second dominant PFCAs in hooded seal, True’s beaked whale and white sided dolphin was PFTrDA (C13). Rather than being heavily reliant on the species, the distribution of PFCAs in marine mammals is impacted by geographical differences in exposure sources and sample locations (Spaan et al., 2020). Studies have indicated that the length of the PFAS, and the amount of PFCAs in marine mammals differ depending on the region (Spaan et al., 2020). For instance, there may be regional differences in the dominant PFCA among marine mammals, reflecting differences in the distribution of PFCA. Additionally, species-specific variations in metabolism, diet, and habitat have an impact on the levels of PFAS in marine mammals (Spaan et al., 2020).
3.5 Association between PFAS concentration, sex and body weight
There were no significant differences in PFAS levels between male and female white-sided dolphins in both muscle and liver tissues for the concentration of the six predominant PFAS using t-tests (p > 0.05) (Figure 8). The same was found for all other species (Supplementary Figure S1). The absence of difference by sex were likewise observed in previous studies, such as those involving bottlenose dolphins from the northern Adriatic Sea and striped dolphins from the west Mediterranean Sea (López-Berenguer et al., 2020; Sciancalepore et al., 2021).
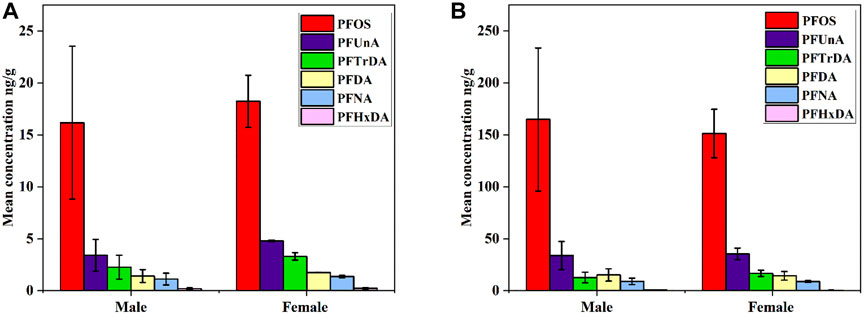
Figure 8. Average concentrations of prominent PFAS among male and female species of white sided dolphin: (A) Muscle tissue; (B) liver tissue.
We compared the correlation between body weight (kg) and quantified predominant PFAS (PFOA, PFNA, PFDA, PFOS, PFUnA, PFTrDA, and PFHxDA) in liver samples for harbor porpoise (n = 21) (Table 4) and white sided dolphins (n = 6) (Table 4). While no significant correlations were found between body weight and the predominant PFAS in the liver of white sided dolphin, a significant negative correlation was found for all predominant PFAS excepted for PFHxDA, which increased with body weight, in the liver of harbor porpoise.
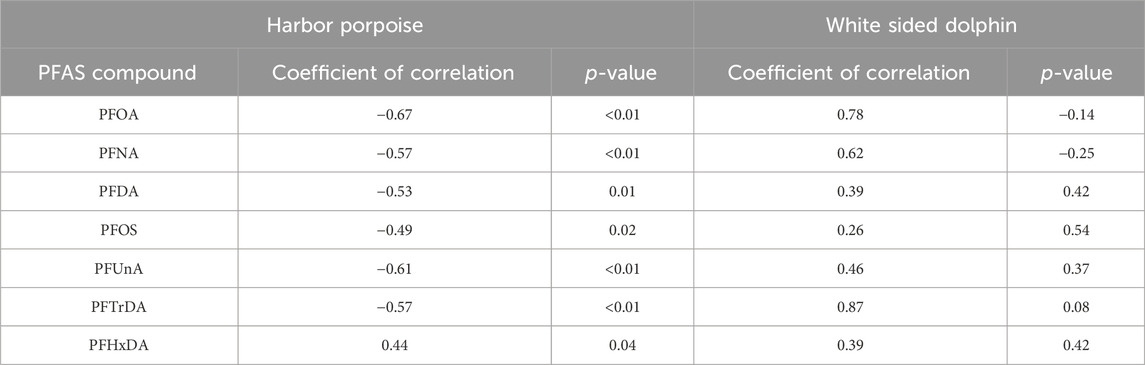
Table 4. Illustrates the correlations between the predominant PFAS in the liver of harbor porpoise and white sided dolphin with their respective body weight (kg).
The accumulation of PFAS in marine organisms can have long term effects on genetic biodiversity, species biodiversity, and ecosystem health (Bilela et al., 2023). The primary constrains affecting the robustness of the study lie in the limited sample size and the ambiguity surrounding the ages of the studied species. One noteworthy limitation pertains to the relatively small number of subjects include in our research, which may hinder the generalizability of our findings. Additionally, the study faced challenges associated with the unavailability of age information for the selected species. During our investigations, we relied on decreased sea mammals’ specimens obtained from both the Saint-Lawrence River and Gulf. This method of sample collection, while practical for certain aspects of our study, introduced a significant drawback in the inability to ascertain the age of each individual mammals. Consequently, this lack of age data poses a potential limitation to the comprehensive understanding of the life cycle and developmental patterns within the studied populations. It must nevertheless be emphasized that it is not easy to come by tissues samples from sea mammals and the collected data are quite precious and useful.
4 Conclusions
In summary, a thorough analysis of 80 PFAS found in liver and muscle samples from 39 marine mammals’ specimens has shed light on the distribution and accumulation patterns of these contaminants. The results highlight the liver as the principal location where PFAS builds up, with a significant 76% greater concentration there than in the muscle. This emphasized role was more prominent for at least forty distinct PFAS chemicals.
Among the noteworthy findings is the discovery that the liver of white sided dolphin had an average PFOS content of 183 ng/g ww. The study carefully detected 41 PFAS in liver sample, whereas 31 PFAS were found in muscle samples. White sided dolphins, white beaked dolphin, and harbor porpoise species that occupy higher trophic levels in the marine food web, consistently displayed greater accumulation. Interestingly, True’s beaked whales did not exhibit higher PFAS buildup despite their placement at a higher trophic level.
The growing knowledge of PFAS negative effects on the environment is reflected in the increasing prohibitions and bans on the chemical by numerous governmental bodies and organizations. The study implies, ironically, that prohibiting one class of PFAS could cause an increase in the accumulation of another. This emphasizes how difficult it is to manage PFAS contamination and how important it is to have a broader, all-encompassing regulatory strategy.
Data availability statement
The original contributions presented in the study are included in the article/Supplementary Material, further inquiries can be directed to the corresponding author.
Ethics statement
Ethical approval was not required for the study involving animals in accordance with the local legislation and institutional requirements because this study was made using carcasses of deceased animals that float ashore and included in a surveillance program. No permits are required for the collection of such samples.
Author contributions
NK: Project administration, Validation, Investigation, Data curation, Visualization, Writing–original draft. ML: Formal Analysis, Investigation, Methodology, Validation, Writing–original draft. ML: Writing–review and editing. QD: Formal Analysis, Investigation, Methodology, Writing–review and editing, Supervision. JF: Methodology, Supervision, Writing–review and editing, Investigation, Data curation. SL: Investigation, Methodology, Supervision, Writing–review and editing, Conceptualization, Validation. SS: Conceptualization, Funding acquisition, Methodology, Project administration, Supervision, Validation, Writing–review and editing.
Funding
The author(s) declare that financial support was received for the research, authorship, and/or publication of this article. NSERC and New Frontiers Exploration provided the financial support for this work. The transport and sampling of the carcasses were partially supported by the Department of Fisheries and Oceans, Canada. Mélanie Lemire is the titular of the Littoral Research Chair (mainly funded by Sentinel North and Northern Contaminant Programme of the Crown-Indigenous Relations and Northern Affairs Canada), is a member of Quebec Océan and also receives a salary grant from the Fonds de recherche du Québec–Santé (FRQS).
Acknowledgments
We would like to thank the staff of the Réseau québécois d’urgences pour les mammifères marins and the Canadian Wildlife Health Cooperative Quebec regional for their assistance with this project.
Conflict of interest
The authors declare that the research was conducted in the absence of any commercial or financial relationships that could be construed as a potential conflict of interest.
Publisher’s note
All claims expressed in this article are solely those of the authors and do not necessarily represent those of their affiliated organizations, or those of the publisher, the editors and the reviewers. Any product that may be evaluated in this article, or claim that may be made by its manufacturer, is not guaranteed or endorsed by the publisher.
Supplementary material
The Supplementary Material for this article can be found online at: https://www.frontiersin.org/articles/10.3389/fenvc.2024.1403728/full#supplementary-material
References
Bilela, L. L., Matijošytė, I., Krutkevičius, J., Alexandrino, D. A., Safarik, I., Burlakovs, J., et al. (2023). Impact of per-and polyfluorinated alkyl substances (PFAS) on the marine environment: raising awareness, challenges, legislation, and mitigation approaches under the One Health concept. Mar. Pollut. Bull. 194, 115309. doi:10.1016/j.marpolbul.2023.115309
Brunn, H., Arnold, G., Körner, W., Rippen, G., Steinhäuser, K. G., and Valentin, I. (2023). PFAS: forever chemicals—persistent, bioaccumulative and mobile. Reviewing the status and the need for their phase out and remediation of contaminated sites. Environ. Sci. Eur. 35, 20. doi:10.1186/s12302-023-00721-8
Cara, B., Lies, T., Thimo, G., Robin, L., and Lieven, B. (2022). Bioaccumulation and trophic transfer of perfluorinated alkyl substances (PFAS) in marine biota from the Belgian North Sea: distribution and human health risk implications. Environ. Pollut. 311, 119907. doi:10.1016/j.envpol.2022.119907
Carrizo, J. C., Munoz, G., Duy, S. V., Liu, M., Houde, M., Amé, M. V., et al. (2023). PFAS in fish from AFFF-impacted environments: analytical method development and field application at a Canadian international civilian airport. Sci. Total Environ. 879, 163103. doi:10.1016/j.scitotenv.2023.163103
Casas, G., Iriarte, J., D'Agostino, L. A., Roscales, J. L., Martinez-Varela, A., Vila-Costa, M., et al. (2023). Inputs, amplification and sinks of perfluoroalkyl substances at coastal Antarctica. Environ. Pollut. 338, 122608. doi:10.1016/j.envpol.2023.122608
Cheng, W., Doering, J. A., Lalone, C., and Ng, C. (2021). Integrative computational approaches to inform relative bioaccumulation potential of per-and polyfluoroalkyl substances across species. Toxicol. Sci. 180, 212–223. doi:10.1093/toxsci/kfab004
European Chemical Agency (2023). European Chemical Agency. Available at: https://echa.europa.eu/es/view-article/-/journal_content/title/9109026-275.
Gebbink, W. A., Bossi, R., Rigét, F. F., Rosing-Asvid, A., Sonne, C., and Dietz, R. (2016). Observation of emerging per-and polyfluoroalkyl substances (PFASs) in Greenland marine mammals. Chemosphere 144, 2384–2391. doi:10.1016/j.chemosphere.2015.10.116
Giroux, I., Hébert, S., and Berryman, D. (2016). Qualité de l’eau du Saint-Laurent de 2000 à 2014: paramètres classiques, pesticides et contaminants émergents. Le. Nat. Can. 140, 26–34. doi:10.7202/1036500ar
Guo, Y., Shi, W., Liang, Y., Liu, Z., Xie, Q., Wu, J., et al. (2023). Spatiotemporal and life history related trends of per-and polyfluoroalkyl substances in Indo-Pacific finless porpoises from south China sea (2007–2020). Chemosphere 310, 136780. doi:10.1016/j.chemosphere.2022.136780
Hamid, N., Junaid, M., Manzoor, R., Sultan, M., Chuan, O. M., Wang, J., et al. (2023). An integrated assessment of ecological and human health risks of per-and polyfluoroalkyl substances through toxicity prediction approaches. Sci. Total Environ. 905, 167213. doi:10.1016/j.scitotenv.2023.167213
Han, X., Snow, T. A., Kemper, R. A., and Jepson, G. W. (2003). Binding of perfluorooctanoic acid to rat and human plasma proteins. Chem. Res. Toxicol. 16, 775–781. doi:10.1021/tx034005w
Haque, F., Soerensen, A. L., Sköld, M., Awad, R., Spaan, K. M., Lauria, M. Z., et al. (2023). Per-and polyfluoroalkyl substances (PFAS) in white-tailed sea eagle eggs from Sweden: temporal trends (1969–2021), spatial variations, fluorine mass balance, and suspect screening. Environ. Sci. Process. Impacts 25, 1549–1563. doi:10.1039/d3em00141e
Helmer, R. W., Reeves, D. M., and Cassidy, D. P. (2022). Per-and Polyfluorinated Alkyl Substances (PFAS) cycling within Michigan: contaminated sites, landfills and wastewater treatment plants. Water Res. 210, 117983. doi:10.1016/j.watres.2021.117983
Hudon, C., and Carignan, R. (2008). Cumulative impacts of hydrology and human activities on water quality in the St. Lawrence River (Lake Saint-Pierre, Quebec, Canada). Can. J. Fish. Aquatic Sci. 65, 1165–1180. doi:10.1139/f08-069
Kaboré, H. A., Goeury, K., Desrosiers, M., Vo Duy, S., Liu, J., Cabana, G., et al. (2022). Novel and legacy per- and polyfluoroalkyl substances (PFAS) in freshwater sporting fish from background and firefighting foam impacted ecosystems in Eastern Canada. Sci. Total Environ. 816, 151563. doi:10.1016/j.scitotenv.2021.151563
Kah, M., and Kookana, R. (2023). Poly-and perfluroalkyl substances (PFAS) in the environment. Chem. Int. 45, 40. doi:10.1515/ci-2023-0220
Khan, B., Burgess, R. M., and Cantwell, M. G. (2023). Occurrence and bioaccumulation patterns of per-and polyfluoroalkyl substances (PFAS) in the marine environment. ACS ES&T Water 3, 1243–1259. doi:10.1021/acsestwater.2c00296
Klingelhöfer, D., Braun, M., Groneberg, D. A., and Brüggmann, D. (2024). The “forever” per-and polyfluoroalkyl substances (PFAS): a critical accounting of global research on a major threat under changing regulations. Chemosphere 354, 141694. doi:10.1016/j.chemosphere.2024.141694
Lam, J. C. W., Lyu, J., Kwok, K. Y., and Lam, P. K. S. (2016). Perfluoroalkyl substances (PFASs) in marine mammals from the south China Sea and their temporal changes 2002–2014: concern for alternatives of PFOS? Environ. Sci. Technol. 50, 6728–6736. doi:10.1021/acs.est.5b06076
Langberg, H. A., Breedveld, G. D., Grønning, H. M., Kvennås, M., Jenssen, B. M., and Hale, S. E. (2019). Bioaccumulation of fluorotelomer sulfonates and perfluoroalkyl acids in marine organisms living in aqueous film-forming foam impacted waters. Environ. Sci. Technol. 53, 10951–10960. doi:10.1021/acs.est.9b00927
Law, R. J., Bersuder, P., Mead, L. K., and Jepson, P. D. (2008). PFOS and PFOA in the livers of harbour porpoises (Phocoena phocoena) stranded or bycaught around the UK. Mar. Pollut. Bull. 56, 792–797. doi:10.1016/j.marpolbul.2008.01.001
Liu, S., Jin, B., Arp, H. P. H., Chen, W., Liu, Y., and Zhang, G. (2022). The fate and transport of chlorinated polyfluorinated ether sulfonates and other PFAS through industrial wastewater treatment facilities in China. Environ. Sci. Technol. 56, 3002–3010. doi:10.1021/acs.est.1c04276
Liu, Y., Wang, Q., Ma, L., Jin, L., Zhang, K., Tao, D., et al. (2023). Identification of key features relating to the coexistence mechanisms of trace elements and per-and polyfluoroalkyl substances (PFASs) in marine mammals. Environ. Int. 178, 108099. doi:10.1016/j.envint.2023.108099
López-Berenguer, G., Bossi, R., Eulaers, I., Dietz, R., Peñalver, J., Schulz, R., et al. (2020). Stranded cetaceans warn of high perfluoroalkyl substance pollution in the western Mediterranean Sea. Environ. Pollut. 267, 115367. doi:10.1016/j.envpol.2020.115367
Lu, M., Cagnetta, G., Zhang, K., Huang, J., and Yu, G. (2017). Mechanochemical mineralization of “very persistent” fluorocarbon surfactants‒6: 2 fluorotelomer sulfonate (6: 2FTS) as an example. Sci. Rep. 7, 17180. doi:10.1038/s41598-017-17515-7
Lynch, K. M., Fair, P. A., Houde, M., Muir, D. C. G., Kannan, K., Bossart, G. D., et al. (2019). Temporal trends in per- and polyfluoroalkyl substances in bottlenose dolphins (Tursiops truncatus) of Indian river lagoon, Florida and charleston, South Carolina. Environ. Sci. Technol. 53, 14194–14203. doi:10.1021/acs.est.9b04585
Macorps, N., Labadie, P., Lestremau, F., Assoumani, A., and Budzinski, H. (2023). Per-and polyfluoroalkyl substances (PFAS) in surface sediments: occurrence, patterns, spatial distribution and contribution of unattributed precursors in French aquatic environments. Sci. Total Environ. 874, 162493. doi:10.1016/j.scitotenv.2023.162493
Mahmoudnia, A. (2023). The role of PFAS in unsettling ocean carbon sequestration. Environ. Monit. Assess. 195, 310. doi:10.1007/s10661-023-10912-8
Manojkumar, Y., Pilli, S., Rao, P. V., and Tyagi, R. D. (2023). Sources, occurrence and toxic effects of emerging per-and polyfluoroalkyl substances (PFAS). Neurotoxicology Teratol. 97, 107174. doi:10.1016/j.ntt.2023.107174
Martin, J. W., Smithwick, M. M., Braune, B. M., Hoekstra, P. F., Muir, D. C., and Mabury, S. A. (2004). Identification of long-chain perfluorinated acids in biota from the Canadian Arctic. Environ. Sci. Technol. 38, 373–380. doi:10.1021/es034727+
Ma, T., Ye, C., Wang, T., Li, X., and Luo, Y. (2022). Toxicity of per- and polyfluoroalkyl substances to aquatic invertebrates, planktons, and microorganisms. Int. J. Environ. Res. Public Health 19, 16729. doi:10.3390/ijerph192416729
Melake, B. A., Bervoets, L., Nkuba, B., and Groffen, T. (2022). Distribution of perfluoroalkyl substances (PFASs) in water, sediment, and fish tissue, and the potential human health risks due to fish consumption in Lake Hawassa, Ethiopia. Environ. Res. 204, 112033. doi:10.1016/j.envres.2021.112033
Munoz, G., Liu, M., Duy, S. V., Liu, J., and Sauvé, S. (2023). Target and nontarget screening of PFAS in drinking water for a large-scale survey of urban and rural communities in Québec, Canada. Water Res. 233, 119750. doi:10.1016/j.watres.2023.119750
Munoz, G., Mercier, L., Duy, S. V., Liu, J., Sauvé, S., and Houde, M. (2022). Bioaccumulation and trophic magnification of emerging and legacy per-and polyfluoroalkyl substances (PFAS) in a St. Lawrence River food web. Environ. Pollut. 309, 119739. doi:10.1016/j.envpol.2022.119739
Navarathna, C. M., Pray, H., Rodrigo, P. M., Arwenyo, B., Mcneely, C., Reynolds, H., et al. (2023). Microplastics and per-and polyfluoroalkyl substances (PFAS) analysis in sea turtles and bottlenose dolphins along Mississippi’s coast. Analytica 4, 12–26. doi:10.3390/analytica4010003
Rüdel, H., Radermacher, G., Fliedner, A., Lohmann, N., Koschorreck, J., and Duffek, A. (2022). Tissue concentrations of per-and polyfluoroalkyl substances (PFAS) in German freshwater fish: derivation of fillet-to-whole fish conversion factors and assessment of potential risks. Chemosphere 292, 133483. doi:10.1016/j.chemosphere.2021.133483
Sadia, M., Nollen, I., Helmus, R., Ter Laak, T. L., Béen, F., Praetorius, A., et al. (2023). Occurrence, fate, and related health risks of PFAS in raw and produced drinking water. Environ. Sci. Technol. 57, 3062–3074. doi:10.1021/acs.est.2c06015
Savoca, D., and Pace, A. (2021). Bioaccumulation, biodistribution, toxicology and biomonitoring of organofluorine compounds in aquatic organisms. Int. J. Mol. Sci. 22, 6276. doi:10.3390/ijms22126276
Sciancalepore, G., Pietroluongo, G., Centelleghe, C., Milan, M., Bonato, M., Corazzola, G., et al. (2021). Evaluation of per-and poly-fluorinated alkyl substances (PFAS) in livers of bottlenose dolphins (Tursiops truncatus) found stranded along the northern Adriatic Sea. Environ. Pollut. 291, 118186. doi:10.1016/j.envpol.2021.118186
Sheng, N., Li, J., Liu, H., Zhang, A., and Dai, J. (2016). Interaction of perfluoroalkyl acids with human liver fatty acid-binding protein. Archives Toxicol. 90, 217–227. doi:10.1007/s00204-014-1391-7
Sosnowska, A., Bulawska, N., Kowalska, D., and Puzyn, T. (2023). Towards higher scientific validity and regulatory acceptance of predictive models for PFAS. Green Chem. 25, 1261–1275. doi:10.1039/d2gc04341f
Spaan, K. M., van Noordenburg, C., Plassmann, M. M., Schultes, L., Shaw, S., Berger, M., et al. (2020). Fluorine mass balance and suspect screening in marine mammals from the Northern Hemisphere. Environ. Sci. Technol. 54, 4046–4058. doi:10.1021/acs.est.9b06773
Stockin, K., Yi, S., Northcott, G., Betty, E., Machovsky-Capuska, G., Jones, B., et al. (2021). Per-and polyfluoroalkyl substances (PFAS), trace elements and life history parameters of mass-stranded common dolphins (Delphinus delphis) in New Zealand. Mar. Pollut. Bull. 173, 112896. doi:10.1016/j.marpolbul.2021.112896
Stockholm Convention (2007). The POPs [WWW document]. Stockholm convention on persistent organic pollutants. https://chm.pops.int/TheConvention/ThePOPs/Listi ngofPOPs/tabid/2509/Default.aspx.
Stockholm Convention (2022). The POPs [WWW document]. Stockholm convention on persistent organic pollutants. https://chm.pops.int/TheConvention/ThePOPs/Listi ngofPOPs/tabid/2509/Default.aspx.
Stockholm Convention (2023). The POPs [WWW document]. Stockholm convention on persistent organic pollutants. https://chm.pops.int/TheConvention/ThePOPs/Listi ngofPOPs/tabid/2509/Default.aspx.
Vis, C., Hudon, C., Cattaneo, A., and Pinel-Alloul, B. (1998). Periphyton as an indicator of water quality in the St Lawrence River (Québec, Canada). Environ. Pollut. 101, 13–24. doi:10.1016/s0269-7491(98)00042-6
Wang, Q., Ruan, Y., Jin, L., Tao, L. S., Lai, H., Li, G., et al. (2023). Legacy and emerging per-and polyfluoroalkyl substances in a subtropical marine food web: suspect screening, isomer profile, and identification of analytical interference. Environ. Sci. Technol. 57, 8355–8364. doi:10.1021/acs.est.3c00374
Woodcroft, M. W., Ellis, D. A., Rafferty, S. P., Burns, D. C., March, R. E., Stock, N. L., et al. (2010). Experimental characterization of the mechanism of perfluorocarboxylic acids' liver protein bioaccumulation: the key role of the neutral species. Environ. Toxicol. Chem. 29, 1669–1677. doi:10.1002/etc.199
Xie, Z., and Kallenborn, R. (2023). Legacy and emerging per-and polyfluoroalkyl substances in polar regions. Curr. Opin. Green Sustain. Chem. 42, 100840. doi:10.1016/j.cogsc.2023.100840
Zhang, X., Lohmann, R., and Sunderland, E. M. (2019). Poly-and perfluoroalkyl substances in seawater and plankton from the northwestern Atlantic margin. Environ. Sci. Technol. 53, 12348–12356. doi:10.1021/acs.est.9b03230
Keywords: marine mammals, PFAS, liver, muscle, white-sided dolphin, St. Lawrence Estuary and Gulf
Citation: Khalid NK, Le Calvez M, Lemire M, Dinh QT, Fontaine J, Lair S and Sauvé S (2024) Occurrence of 80 per and polyfluorinated alkyl substances (PFAS) in muscle and liver tissues of marine mammals of the St. Lawrence Estuary and Gulf, Quebec, Canada. Front. Environ. Chem. 5:1403728. doi: 10.3389/fenvc.2024.1403728
Received: 19 March 2024; Accepted: 06 June 2024;
Published: 04 July 2024.
Edited by:
Zhijiang Lu, Wayne State University, United StatesReviewed by:
Yunqiao Zhou, Institute of Tibetan Plateau Research (CAS), ChinaShizhen Zhao, Guangzhou Institute of Geochemistry (CAS), China
Copyright © 2024 Khalid, Le Calvez, Lemire, Dinh, Fontaine, Lair and Sauvé. This is an open-access article distributed under the terms of the Creative Commons Attribution License (CC BY). The use, distribution or reproduction in other forums is permitted, provided the original author(s) and the copyright owner(s) are credited and that the original publication in this journal is cited, in accordance with accepted academic practice. No use, distribution or reproduction is permitted which does not comply with these terms.
*Correspondence: Sébastien Sauvé, c2ViYXN0aWVuLnNhdXZlQHVtb250cmVhbC5jYQ==