- Department of Chemistry, Université de Montréal, Montreal, QC, Canada
Applying sewage sludge and biosolids to agricultural lands has become an increasingly essential aspect of sustainable waste management and circular economy as it contributes positively to nutrient recycling, soil fertility and environmental health. Due to the widespread presence of per and poly-fluoroalkyl substances (PFAS) globally, wastewater treatment plants have become a sink for PFAS. PFAS resist degradation by conventional wastewater treatment processes and are usually adsorbed to sewage sludge and biosolids. However, there have been significant concerns that land application of sewage sludge and biosolids could become a probable pathway for PFAS to enter the food chain. This article assessed the global sewage sludge/biosolids generation and country-to-country management methods through a systematic review. The global occurrence, distribution and prevalence of different classes of PFAS were assessed. We also evaluate the factors influencing PFAS contamination in sewage sludge/biosolids and the existing regulations on the upper limit of PFAS in biosolids before their disposal or application to farmland (or other usages). Additionally, most reports revealed high PFAS concentrations in influent, effluent, sewage sludge and biosolids generated worldwide. Overall, recorded PFAS concentration on a global scale varied from 2.2 to 2,156 ng/L (influents), 1.9–4,800 ng/L (effluents) and 2.1–500,000 ng/g (biosolids). While most studies focused on legacy PFAS detection, recent studies have revealed the prevalence of diPAPs in high concentrations in sewage sludge and biosolids, contributing from 40% to 95% of the total PFAS concentration. Across all PFAS classes, PFAAs and diPAPs were the dominant groups exhibiting elevated detection rates (35%–95%). Due to documented PFAS contamination in agricultural lands, rigorous regulations need to be instituted to govern the application of these biowastes on agricultural lands. However, several countries lack data on the level of PFAS in the sewage sludges they generate, and there are currently few or no regulations guiding their application to farmlands. Notably, the diPAPs class of PFAS was shown to be present in biosolids and sewage sludge; their inclusion in the list of PFAS required in standardized analytical methods and risk assessment becomes imperative.
1 Introduction
Sewage sludge is a slurry residue produced from wastewater treatment operations. Typically, sewage sludge comprises approximately 3% solids and 97% liquids (Kumar et al., 2017). On the other hand, biosolids are treated sewage sludge that adheres to specific standards for its utilization or disposal, with an approximate solid content of nearly 90% (USEPA, 2021). Different methods, from composting, acidic oxidation/disinfection, thermal drying, alkaline stabilization, and aerobic and anaerobic digestion, are used for biosolids treatment. These treatments distinguish the physicochemical properties of biosolids from those of sewage sludge (Mohajerani et al., 2017).
The concept of resource recovery from wastes has provided an alternative use for biosolids, sewage sludge and composts because these wastes are rich in organic matter and essential plant nutrients, such as phosphorus, nitrogen, potassium, magnesium, calcium, and sulphur (Dad et al., 2019). The total nitrogen, phosphorus, and potassium concentrations in biosolids can be as high as 3%, 1.5%, and 0.7%, respectively, while the organic content can be from 30% to 40% of organic matter (Kumar et al., 2017). However, the methods used to stabilize sewage sludge greatly influence the contents of organic matter and nutrients and the fertilizer value of the resulting biosolids (Dad et al., 2019). Owing to the chemical composition inherent in these biowaste materials, they find application as fertilizers in agricultural practices, land reclamation and reforestation efforts. This utilization is a viable alternative source of essential macronutrients for soil enrichment (Rigby et al., 2016; Dad et al., 2019). Studies have also shown that biosolids-based soil amendments enhance plant accessibility of nitrates, ammonium ions, and nitrites in the soil as a consequence of the mineralization of organic nitrogen (Sharma et al., 2017; Wang et al., 2017).
Applying sewage sludge/biosolids and composts to agricultural lands has become an increasingly essential aspect of sustainable nutrient management as it contributes positively to nutrient recycling, soil fertility and properties. There are clear circular economy arguments for closing the nutrient and carbon loops so that nutrients extracted from the soils to produce our food are returned to the soils to maintain their fertility and quality. The use of nutrients from biosolids also replaces chemical fertilizers that would otherwise need to be produced, mined and transported with all of the associated environmental impacts.
Although the land application of biosolids is advancing in popularity and is beneficial in terms of improving soil health and resource recovery, there are recent apprehensions concerning environmental and human health hazards associated with their application to agricultural land. Several organic and inorganic pollutants have been found in biosolids that can potentially be transferred from the applied biosolids to crops (Clarke and Cummins, 2015; Gworek et al., 2021; Hušek et al., 2022; Kumar et al., 2022; Thompson et al., 2022; Adu et al., 2023; Marchuk et al., 2023). Recent studies have called into question the agricultural use of biosolids due to the documented reciprocal interaction between pollutants such as trace metals, per- and polyfluoroalkyl substances (PFAS), pharmaceuticals, hormones, polychlorinated biphenyls (PCBs), polybrominated diphenyl ethers (PBDEs), pesticides and plasticizers, polycyclic aromatic hydrocarbons (PAHs), flame suppressants, alkyl phenols, as well as other contaminants that could be potentially released in environment (Rathankumar et al., 2020). Many of those contaminants pose a significant risk because they can produce direct biological effects such as genotoxicity, endocrine disruption, neurotoxicity, immunotoxicity or reproductive defects in living organisms (Mohajerani et al., 2017; Rathankumar et al., 2020). Irrespective of how sewage sludge/biosolids are treated, organic and inorganic contaminants are found in biosolids; however, substantial treatment processes will usually significantly abate the volatile components and pathogens (Mohajerani et al., 2017).
PFAS are a class of synthetic aliphatic compounds characterized by a completely fluorinated carbon chain (per-) or partially fluorinated carbon chain (poly-) making up the hydrophobic tail of the compound. This hydrophobic tail is usually connected to at least one hydrophilic head that constitutes the functional group of the compound. PFAS have unique chemical features due to their extremely polar and strong carbon-fluorine linkages, such as excellent thermal and chemical stability and exceptional surface-tension-lowering capabilities (Xiao, 2017). Because of their hydrophobic and hydrophilic characteristics, PFAS have been extensively utilized globally in a variety of applications like firefighting foams, surfactants, industrial emulsifiers, non-stick cooking utensils, paints, stain and water-resistant textiles and carpets, oil-repelling containers, etc. The C-F bond found in PFAS compounds is known as the strongest bond in organic chemistry. This bond confers strong resistance to usual ecological transformation processes such as microbial, heat or chemical degradation (Meegoda et al., 2020; Nguyen et al., 2020; Wackett, 2021; Berhanu et al., 2023).
According to studies dating back nearly 2 decades, the probable route through which PFAS enter sewage sludge is mainly from industrial or residential wastewaters that flow into the wastewater treatment plants (Yu et al., 2009; Ma and Shih, 2010; Coggan et al., 2019; Semerád et al., 2020). PFAS in residential wastewaters results from the use of PFAS-containing products, such as personal care products, food packaging, carpets, and clothes (Schultes et al., 2018; Seltenrich, 2020; Zhu et al., 2021; Kumar et al., 2022; Rodgers et al., 2022). Additionally, extensive discharge of landfill leachate into municipal sewage systems has also been observed to increase PFAS concentrations in WWTPs (Masoner et al., 2020).
In a study conducted in the United States, PFAS concentrations in landfill leachate, influent and effluent samples collected from three landfill WWTPs were contrasted with similar samples from two WWTPs that received no landfill leachate (Thompson et al., 2022). The study concluded that the detection rate for all 73 PFAS analyzed in leachate (92%) was higher than in influent (55%). The total PFAS content in leachate (93,100 ng L) was ten-fold higher than that of influent (6,950 ng L) and effluent (3,730 ng L). The leachate load contributions to PFAS in the WWTPs were found to vary from 0.78 to 31 g d−1 (Thompson et al., 2022).
Investigations reveal that PFAS resists degradation in conventional WWTPs and are retained in both treated wastewater and biosolids; hence, the prevalence at different concentrations was observed (Table 1) from location to location (Meegoda et al., 2020; Kumar et al., 2022; Leung et al., 2022; Starnes et al., 2022; Brunn et al., 2023).
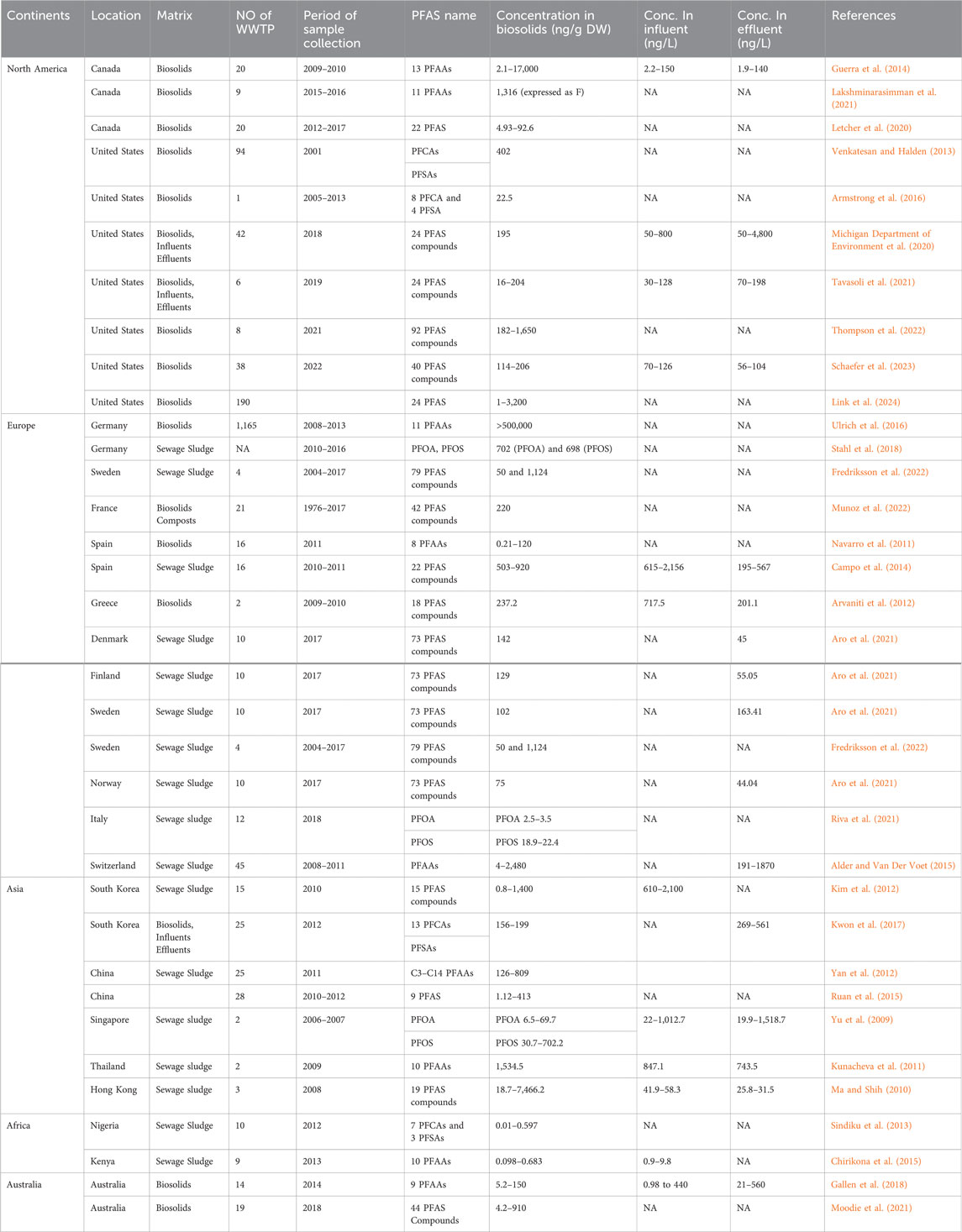
Table 1. Survey of PFAS concentrations in biosolids, influents and effluents from selected countries.
Exposure to PFAS has been associated with several health hazards, including decreased vaccination response in infants (Grandjean et al., 2017), heightened risk of type 2 diabetes in both men and women (Roth and Petriello, 2022), gestational diabetes in women (Birru et al., 2021), disrupted metabolic rate (Schillemans et al., 2021), reduced fertility (Rickard et al., 2022), reduced fetal development and obesity (Sevelsted et al., 2022; Liu et al., 2023), elevated risk of some cancers (Fenton et al., 2021; Ojo et al., 2021) and decreased immune system (Rappazzo et al., 2017).
This article systematically assessed the global generation of sewage sludge/biosolids, the diverse treatment methodologies employed, and the prevalence of contamination of per- and polyfluoroalkyl substances (PFAS) within these waste products globally. We also explored the factors influencing PFAS contamination in sewage sludge/biosolids and compiled the existing PFAS regulations or recommendations for land application.
2 Review methodology
To better understand the trends of global PFAS release into the environment through biosolid/sewage sludge applications, we reviewed multiple studies that addressed biosolid generation and management worldwide and the PFAS concentrations in biosolids from these regions.
A database search approach was implemented by independently selecting relevant keywords and terms and, in sequence, acquiring the appropriate publications from Web of Science, PubMed, ScienceDirect, Google Scholar, Scopus database, and federal and state records. The keywords were connected by the Boolean operators “AND” and “OR,” for instance, “PFAS in sewage sludge” OR “PFAS in biosolid AND “Countries” “Sewage Sludge AND Agriculture.” Other keywords include: “Biosolid generation in countries,” “PFAS variation in sewage sludge,” etc. Article exploration for this appraisal focused on articles published between 2003 and 2023. This extensive search was vital because it encompasses a substantial number of studies that will assist in validating the likely trends and movement of PFAS in the environment over time regarding PFAS use regulations. From the vast search results, the articles used in this review were selected based on their pertinence, thoroughness and focus on this review. Supplementary Table S1, presents the list of abbreviations used in the review.
3 Survey of global biosolids generation and management—case studies
The annual global production of biosolids is experiencing a remarkable upswing, primarily due to the continuous expansion and technological progress of existing WWTPs. This upsurge can be directly linked to the escalating demand for water resources (Mohajerani et al., 2017). The global generation of biosolids is estimated at 100 to 125 million tons dry weight and is predicted to increase to 150–200 million tons dry weight by 2025 (Kumar et al., 2022). Countries are constantly looking for eco-friendly and sustainable ways to manage the increasing amounts of biosolids generated.
The global evaluation of biosolids generation and management is presented in Figures 1, 2. The figures revealed that a significant percentage of biosolids end up in agricultural land applications or as landfills. Consequently, PFAS and other pollutants in these products are accessible for plant uptake and may enter our food chain.
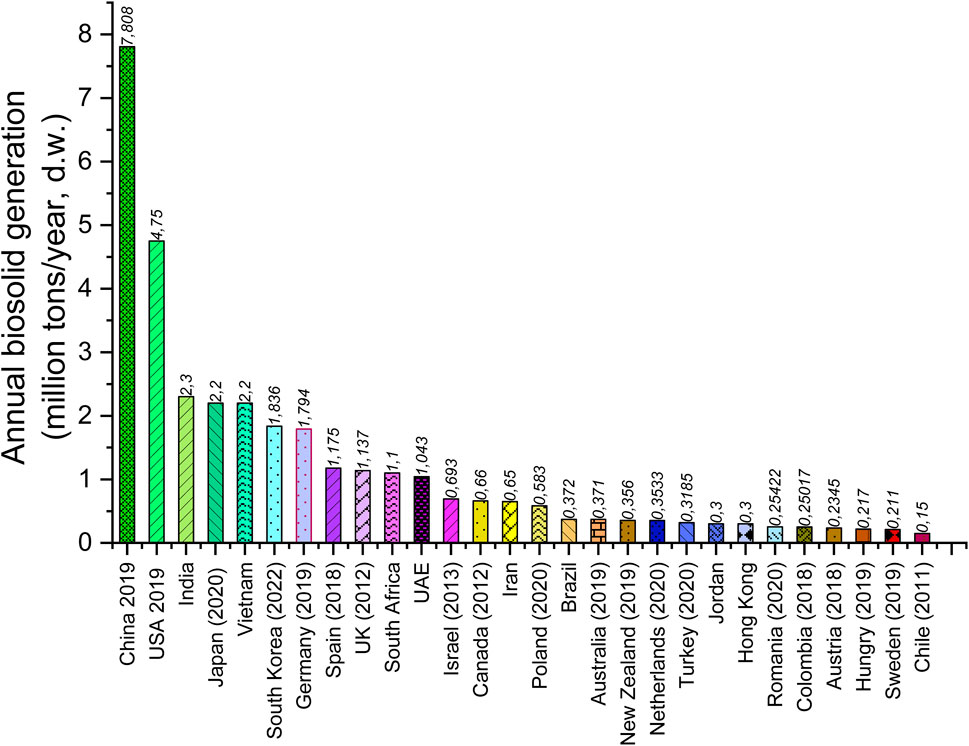
Figure 1. Annual generation of biosolids (BS) by selected countries. Source [China (Wei et al., 2020), United States (USEPA, 2021), Japan (Yosuke Matsumiya, 2020), Spain, Germany, United Kingdom, Poland, Netherlands, Türkiye, Romania, Austria, Sweden, (Eurostat, 2022), Canada (Canadian Council of Ministers of the Environment, 2012), Iran, South Korea, Jordan, Brazil (UN-Habitat, 2008; The Australian and New Zealand Biosolids Partnership ANZBP, 2019) New Zealand and Australia, Colombia (Venegas et al., 2021), Chile and Israel (Organisation for Economic Co-Operation and Development OECD, 2017), India, South Africa, Hong Kong (Sharma et al., 2017)].
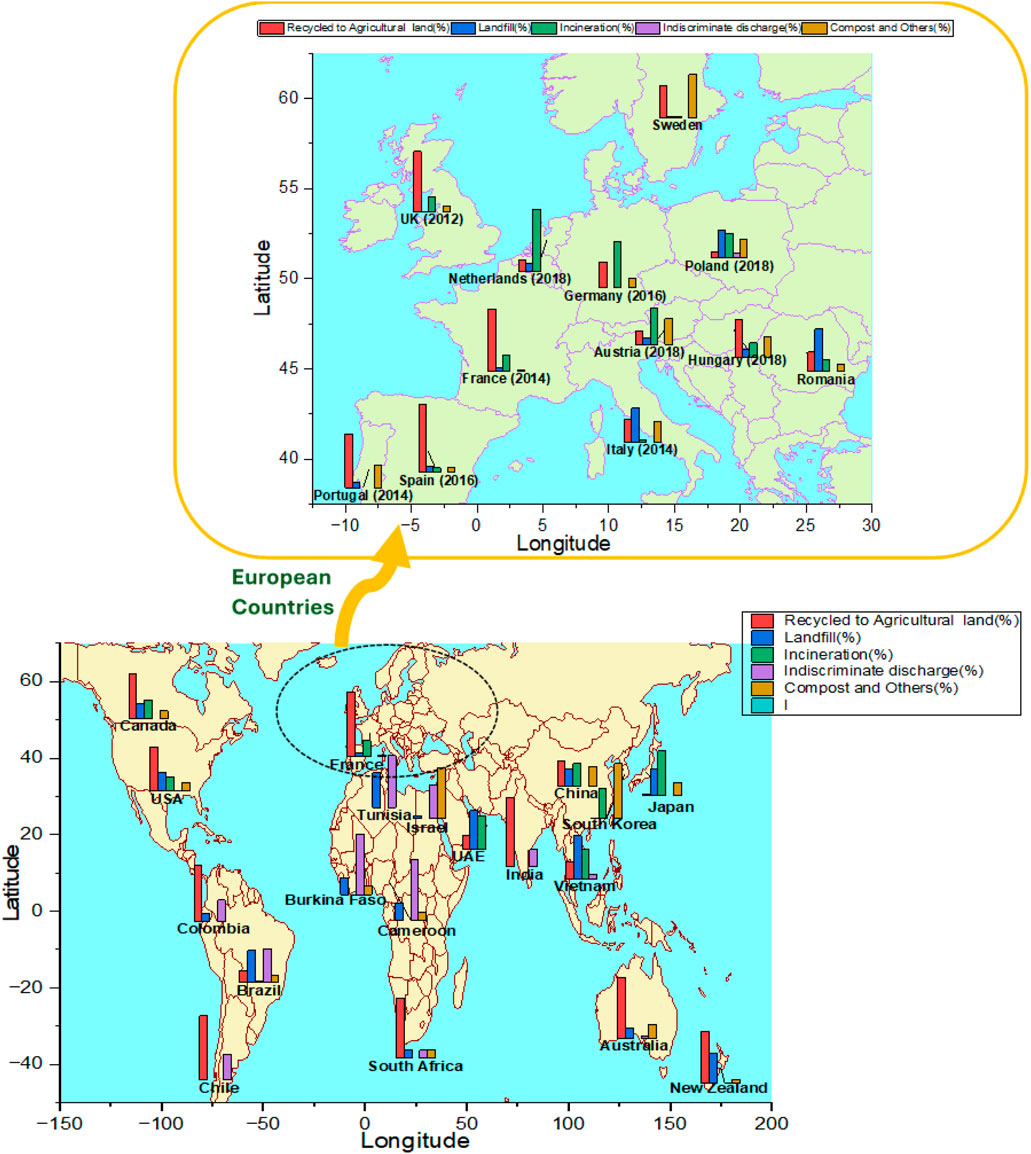
Figure 2. Main options for the management of biosolids in selected countries. Source [China (2019) (Wei et al., 2020), United States (2019) (USEPA, 2021), Japan (2020) (Yosuke Matsumiya, 2020), Spain, Germany, United Kingdom, Poland, Netherlands, Türkiye, Romania, Austria and Sweden, (Eurostat, 2022), Canada (2012) (Canadian Council of Ministers of the Environment, 2012), South Korea (2022) (South Korea National Sewer Information System, 2022), Jordan (2011) (Batarseh et al., 2011), Iran, Brazil, Burkina Faso, Cameroon (2008) (UN-Habitat, 2008; The Australian and New Zealand Biosolids Partnership ANZBP, 2019) New Zealand, Australia and Colombia (2021) (Venegas et al., 2021), Chile and Israel (2017) (Organisation for Economic Co-Operation and Development OECD, 2017), India, South Africa, Hong Kong (2017) (Sharma et al., 2017) Vietnam (2018) (ARCOWA, 2018) and UAE (2012) (Alshankiti et al., 2024)].
Based on the United States Environmental Protection Agency reports in 2021, the United States generated 4.75 million dry metric tons of biosolids in 2019 (USEPA, 2021). Currently, the total biosolids generated in the USA are being managed in a variety of ways: land application (51%), landfill (22%), incineration (16%), additional management techniques (10%) and surface discharge (1%) (USEPA, 2021). Biosolids have been widely utilized in US agriculture since the early 1970s, supported by various regulations to promote their safe and beneficial application on land. Under stringent assessments, biosolids were no longer identified as wastes but as potential resources for nutrients, resulting in greater flexibility for biosolids management through agricultural application.
To evaluate the environmental risks linked to biosolids, the United States Environmental Protection Agency (USEPA), through a literature survey in 2019, assessed different contaminants present in biosolids generated across the United States (Zhen et al., 2017). The study found more than 500 potential pollutants in at least one instance, and variations of contaminants found in biosolids were observed to depend upon inputs to individual wastewater treatment facilities over time (USEPA, 2019).
A survey conducted between 2009 and 2019 on biosolid production from different provinces in China estimated that China generates 7.8 million tons of dry biosolids annually with a population of more than one billion (USEPA, 2019). Anaerobic digestion, thermal hydrolysis, composting and incineration are China’s predominant sludge treatment technologies, with an estimated output of 6,944, 8,342, 11,250, and 27,122 t/dw in 2019, respectively. Zhen et al. (2017) affirmed that the status of sewage sludge management in China is significantly far from satisfactory, as more than 80% of sewage sludge generated is being discarded inappropriately. Under safety regulations for sanitary landfills in China, the threshold of sludge water content is 60% (Liu et al., 2021). Zhen et al. (2017) noted that even though landfill management is the most prevalent outcome for biosolids in China, most sewage sludge is disposed of directly after mechanical dewatering, with the sewage sludge still containing more than 80% moisture content and minimal compressive strength. Generally, 29.3% of the total biosolids generated in China are disposed of through land application, subsequently followed by incineration (26.7%) and sanitary landfills (20.1%), with much smaller quantities reused through their incorporation in building materials (15.9%) and various other methods (8.0%) (Wei et al., 2020; Chen et al., 2022).
Japan generates up to 2.0 million tons of biosolids annually, with reclaim and disposal rates climbing up to approximately 97%. Over 50 percent (52%) of Japan’s biosolids are recycled into construction materials, while 31% is used in landfills, and 12% is digested anaerobically for bioenergy generation (Yosuke Matsumiya, 2020). Biosolid applications for agricultural purposes in Japan are relatively low, as farmers are concerned about the environmental risks of applying biosolids to agricultural land.
Based on a study by Collivignarelli et al. (2019), Europe generates more than 10 million tons of dry sewage sludge annually, with Germany, the United Kingdom, France, Spain, and Poland being the major contributors. Similar to biosolid management in China, land utilization, either through direct application or after composting, as well as incineration, are the primary sludge management process in European countries. About 50% of the total biosolids generated in Europe are spread on agricultural land; 28% are incinerated, while 18% are disposed of via landfills (Eurostat, 2022). For instance, in Ireland, 99% of sewage sludge generated in 2018 from urban areas was reused in agriculture (Eurostat, 2022).
The residual part is disposed of through landfill cover (e.g., Sweden), reuse in forestry (e.g., Slovakia, Latvia, and Ireland), pyrolysis, and storage (e.g., Greece, Italy, and Poland) (Eurostat, 2022). The compost approach is largely utilized in some European agricultural countries such as Luxembourg, Slovakia, Estonia, Hungary, Lithuania, etc. Between 40% and 60% of sewage sludge produced in these countries is managed through composting (Eurostat, 2022).
In Türkiye, Croatia, and Malta, about 42%–100% of the total sewage generated is disposed of in landfills. In contrast, incineration has been the predominant biosolid management practice in countries such as Belgium, Netherlands, and Germany due to the strict regulation on the agricultural utilization of biosolids as a result of possible contamination of the biosolids (Gianico et al., 2021).
Based on a survey conducted by the Canadian Council of Ministers of Environment (CCME) in 2012, Canada generates 660,000 tons of dry biosolids yearly with no data on the management of the biosolids. To encourage the advantageous use of biosolids, the CCME proposed dewatering, drying, and nutrient reclaiming from wastewater as acceptable management methods to enhance the quality of the biosolids generated (Canadian Council of Ministers of the Environment, 2012). In 2015, about 28.8% of biosolids generated were incinerated, while 15% were used in landfill (Tessier, 2017). According to Tessier, disposal of biosolids via incineration is declining, while land application is rising in Canada (Venegas et al., 2021). This trend is related to regulatory influences and voluntary biosolids quality enhancements but has been drastically slowed down by recent media attention on the presence of PFAS in biosolids.
Due to the paucity of information from these regions and the lack of appropriate and sufficient wastewater treatment facilities in India, South America, and Africa, estimating the annual biosolid production and management is challenging. Biosolids legislation, generation, stabilization, and application are underdeveloped in these continents and countries.
In Latin America, the primary strategy for managing biosolids/sewage sludge predominantly involves recycling them on agricultural land. This trend is exemplified by Colombia, where, in 2018, seven cities and four districts collectively generated 250,172 dry metric tons of biosolids (Venegas et al., 2021). Notably, 65% of this quantity was employed in agricultural areas. However, several WWTPs in this country dispose of sewage sludge into the environment without proper stabilization (Venegas et al., 2021).
In some areas of Chile, the percentage of biosolids recycled to land increased to 75% between 2009 and 2017. Between 2007 and 2017, over 285,836 tons of biosolids were dumped on 15,423 ha of farmland in the Brazilian state of Paraná (Bittencourt et al., 2014; Venegas et al., 2021).
Data from the India Central Pollution Control Board sets sewage generated in India at 62,000 million litres per day (MLD); however, only 23,277 MLD are being treated, which represents 37% of total sludge generated (PFAS, 2019). In 2021, the percentage of treated sewage increased to 50%, with more treatment plants being constructed. However, the remaining 50% of untreated sewage sludge is indiscriminately discharged into the environment (CPCB, 2021).
For Africa, it is difficult to estimate the amount of sewage sludge generated because individual households are often for the most part responsible for managing their own sewage (especially in rural and sub-urban settlements). While individual households rely on on-site septic tank systems for safe treatment of their wastewater, industrial wastewater is primarily treated through advanced water treatment methods (Onu et al., 2023).
Based on the Australian and New Zealand Biosolids Partnership Report 2019, Australia generates approximately 371,000 dry tonnes of biosolids yearly. Application to agricultural land is the main disposal route (70%), while 19% is used for landscaping or land restoration, and the residual is used as landfill or discharged to the ocean (The Australian and New Zealand Biosolids Partnership ANZBP, 2019).
3.1 Variations in PFAS concentration across wastewater influent, effluent and sewage sludge/biosolids
3.1.1 PFAS variation in wastewater influent and effluent
Generally, high PFAS concentrations have been reported in wastewater influents globally, especially influent from industrial source (Lenka et al., 2021). Studies have revealed the possibility of observing higher PFAS concentrations in effluents compared to the corresponding influent in WWTPs, which could suggest the potential transformation of initially undetected precursor ions (Gallen et al., 2018). While some studies reported increased PFAS concentrations in effluents compared to influents (Gallen et al., 2018; Tavasoli et al., 2021), other studies reported divergent results with higher PFAS in influents compared to effluents (Arvaniti et al., 2012; Campo et al., 2014; Schaefer et al., 2023).
In a study conducted by Tavasoli et al. (2021), the concentration of 24 PFAS compounds in the influents and effluents from 6 WWTPs in the United States was monitored. The study recorded a higher PFAS concentration in effluents (70 and 198 ng L−1) compared to influents (30–128 ng L−1). The study also recorded a higher PFAS concentration in effluents compared to influents during warmer seasons. The seasonal difference (in terms of temperature) of the treatment basins was between 4°C and 11°C in March and 20°C–23°C in July, and the PFAS concentration in influents within these seasons was unchanged. The increased PFAS in effluent in warmer seasons was therefore ascribed to further PFAS biochemical transformations with increased basin temperature, which is in accordance with prior studies. The study suggested the presence of higher concentrations of fluorotelomers and other PFAS precursors in the effluents that could be converted during biological treatment (Tavasoli et al., 2021).
Studies show that degradation of precursor PFAS in influents results in higher PFAA concentration in effluents. Houtz et al. (2018) reported 200 and 350 ng L−1 for PFPeA and PFHxA, respectively, in wastewater influent from the US. Within 3 days of wastewater treatment, the concentration of these two PFAS in the effluent increased to 1,690 and 1,370 ng L−1, respectively. Sun et al. (2012) also reported an increase from 26,000 ng L−1 (influent) to 28,000 ng L−1 (effluent) for PFOA in wastewaters from China.
In a study conducted by Gallen et al. (2018), a targeted analysis of 9 PFAS was carried out on influents, effluents and biosolids samples collected in 2014 from 14 WWTPs in Australia. The 9 PFAS concentrations detected ranged from 0.98 to 440 ng/L, 21–560 ng/L and 5.2–150 ng/g in influents, effluents and biosolids, respectively (Gallen et al., 2018). The estimated annual levels of PFOA and PFOS in wastewaters are 65 kg and 26 kg, respectively, while the yearly loads for biosolids were 2 kg and 8 kg, respectively. The study reported about a 9.8-59-fold increase in the total PFAS concentrations in effluents (Gallen et al., 2018). The observed rise in PFAS concentrations in effluents supports the production of PFAS from larger precursor chemicals within WWTPs, consistent with conclusion from several research studies (Dinglasan et al., 2004; Xiao, 2017).
In a nationwide review of PFAS concentrations in United States wastewaters, PFOA upsurged by an average of 6.0 ± 1.6 ng/L from the influents to the effluents of WWTPs. At the same time, PFOS showed no appreciable change in concentration. This indicates that PFAS sorption to sludge is counterbalanced by the biotransformation of precursor chemicals (Thompson et al., 2022).
3.1.2 PFAS occurrence and concentrations in biosolids from different locations
The evaluation of PFAS concentrations in biosolids can be used as model variables to estimate human susceptibility to PFAS through agricultural products from lands receiving biosolids. Table 1 summarizes the detection and concentrations of PFAS in biosolids from different countries. Most of the articles reviewed in this study gave a historical trajectory of changes in PFAS concentrations over specific periods to determine the trends of PFAS in the environment.
While some studies affirmed no decrease in PFAS concentrations in sewage sludge over the study period despite regulations (Armstrong et al., 2016), others reported both a decline and an increase in concentrations (Ulrich et al., 2016; Fredriksson et al., 2022; Thompson et al., 2022). Alder and van der Voet reported that an average reduction of 77% for perfluorooctanesulfonic acid (PFOS) and 62% for perfluorooctanoic acid (PFOA) within 2008 and 2011 in sludge generated from Switzerland are possibly due to the implementation of restrictions on perfluoroalkyl acids PFAAs (Alder and Van Der Voet, 2015).
The levels of PFAS pollution in biosolids collected between 2004–2017 were investigated in Sweden (Fredriksson et al., 2022). The samples were collected from four WWTPs servicing between 2,500 and 656,000 population units in Sweden. The treatment facilities were linked to residents, industries, and hospitals. The study reported the detection of 79 PFAS with a total concentration of 50 and 1,124 ng/g dry weight. All biosolid samples in the study showed detectable concentrations of C4- and C8-FASA-based copolymers ranging from 1.4 to 22 ng/g d.w. The study also confirmed that across all tested biosolid samples, the precursors of PFCAs, such as PAPs, FTCAs, and FTSAs, were the predominant groups, accounting for 56% of the 79 anionic and neutral PFAS (Fredriksson et al., 2022).
Longer chain PFAS have been detected in biosolids with increased frequency and concentrations because of their enhanced sorption capacity compared to their detection in effluent waters (Gallen et al., 2018). Ulrich et al. (2016) investigated the trend of 11 PFAS concentrations in biosolids generated from 1,165 different WWTPs in Germany between 2008 and 2013. The study affirmed the prevalence of PFOS in 41% of all sludge samples with a concentration of up to 7,600 μg/kg dm followed by PFOA (1,043 μg/kg dm). Within 15 WWTPs, total PFAS concentration was estimated to be > 500 μg kg−1 dm with biosolids from 71 treatment plants exceeding the permissible threshold of 125 μg kg−1 dm set for ∑11 PFAAs by the Bavarian State Ministry of the Environment and Consumer Protection (Ulrich et al., 2016).
Kwon et al. (2017) investigated the release of PFAS from industrial wastewaters in South Korea. Influent, effluent, and sewage sludge samples were collected from 25 industrial WWTPs connected to paper, textile, metal, electronics, and chemical industries. The paper sector had the greatest median concentration of PFAS in influent samples (411 ng/L), followed by the chemical industry (228 ng/L), the electronics industry (91.4 ng/L), the metals industry (32.6 ng/L), and the textiles industry (15.9 ng/L). PFOA and PFOS were the dominant PFAS (49%–66%). Reduced PFAS concentrations were observed in the effluent and biosolids compared to the influent. However, PFOA persisted in both effluent and sewage sludge.
The flow of PFAS through Canadian sludges collected between 2015–2016 was estimated through a mass balance approach at an average ΣPFAS-F mass flow of 1,316 ng/g (expressed as fluorine atom) (Venkatesan and Halden, 2013). Letcher et al. (2020) studied the influence of side-chain perfluoroalkyl polymer degradation in Canadian sludges and its effect on the concentration of PFAAs in sewage sludge. The concentration of side-chain perfluorooctane sulfonamide-urethane polymer and side-chain perfluorobutane sulfonamide-urethane polymer were evaluated. The study revealed that the concentration of polymers with side chain C8F17 varied from 1.1 to 105 ng/g d.w. while those with C4F9 side-chains varied from 37.5 to 2051 ng/g d.w. However, the total concentrations of 22 other PFAS analyzed in the study ranged from 4.9 to 93 ng/g d.w., which is thirty times lower than the side chain perfluoroalkyl polymers (Letcher et al., 2020).
Data emanating from Hong Kong revealed PFOS concentrations of 7,305 ng/g d.w. in sewage sludge (Ma and Shih, 2010), while PFOA concentration was 190 ng/g in Korean sewage sludge (Kim et al., 2012).
Campo et al. (2014) evaluated the prevalence and distribution of 21 PFAS compounds in biosolid samples collected between 2010–2011 from 16 different Spanish WWTPs. The study reported concentrations of PFAS ranging from 503 to 920 ng/g d.w.
Over the past 2 decades, PFAS levels in biosolids generated from WWTPs across the United States have been assessed for PFAS (Arvaniti et al., 2014). A countrywide study was conducted in the United States to assess 13 per- and polyfluoroalkyl substances (PFAS) in representative biosolids samples collected from 94 WWTPs out of the more than 16,000 WWTPs in the U.S (Venkatesan and Halden, 2013). The samples were stored by the US EPA since the 2001 National Sewage Sludge Survey. The study discovered that the most prevalent PFAS found in biosolids mixtures from 32 US states and the District of Columbia was perfluorooctane sulfonate (PFOS) followed by perfluorooctanoate (PFOA) and perfluorooctanoate (PFDA) with concentrations of 403 ± 127 ng PFOS/g dry wt., 34 ± 22 ng PFOA/g d.w. and 26 ± 20 ng PFDA/g d.w. The study assessed the amount of PFAS in US biosolids to be between 2,749 and 3,450 kg per year, of which 1,375 to 2,070 kg are applied to farmland, and 467–587 kg are disposed of in landfills. Since 2001, however, the types of PFAS detected in biosolids have evolved due to manufacturing changes that favour short-chain PFAS. Work by Armstrong et al. (2016) evaluated the trends of 12 PFAS in biosolid samples collected from WWTPs serving more than 2 million population in the Mid-Atlantic region of the US from 2005 to 2013. The maximum mean concentrations detected over the study 8 years period for perfluorononanoic acid (PFNA), perfluorooctanoic acid (PFOA), and perfluorooctanesulfonic acid (PFOS) were 25.1 ng PFNA/g dw, 23.5 ng PFOA/g dw, and 22.5 ng PFOS/g dw, respectively, with 2.5–5 times higher detection frequency compared to others. The study affirmed that the concentrations of PFAS remain stable in WWTPs despite the regulation on the use of these compounds.
In a new study, Schaefer et al. (2023) monitored both quantifiable and semi-quantifiable PFAS in influents, effluents and sewage sludge from 38 WWTPs across 23 states within the US. The study confirmed the detection of PFAS in all samples analyzed. PFAS were thus found in every stream at every facility. In the influent, effluent, and biosolids, the aggregate concentration of detectable PFAS concentrations quantified were 98 ± 28 ng/L, 80 ± 24 ng/L, and 160,000 ± 46,000 ng/kg dw, which mainly consisted of PFAAs. The study also detected perfluorophosphonic acids (PFPAs) in 100% of the biosolid samples (Schaefer et al., 2023).
Arvaniti and Stasinakis conducted a thorough survey to review 36 PFAS monitoring studies conducted from 2005 to 2015 on wastewater and biosolids generated from the United States, North Europe, Asia, the Mediterranean area, Canada and Australia. The review concluded that PFOS appears to be the most prevalent analyte from all the studies reviewed, with a concentration of up to 7,300 ng/g dw. In contrast, the maximum PFOA concentration recorded was 240 ng/g dw, whereas the concentrations of longer PFCAs (such as C9) varied up to 3,200 ng/g dw (Arvaniti and Stasinakis, 2015).
Targeted and non-targeted screening of PFAS in biosolids and composts collected between 1976 and 2017 from France revealed the dominance of PFOS, EtFOSAA, and cationic and zwitterionic electrochemical fluorination precursors to PFOS in biosolids from municipal biosolids and composts while zwitterionic fluorotelomers dominated the urban organic waste products (Munoz et al., 2022).
Through fluorine mass balance analysis, Aro et al. (2021) evaluated the PFAS concentrations in Nordic Countries, including WWTP effluents and sewage sludge from Denmark, Finland, Faroe Islands, Iceland, Norway, Sweden, and Greenland. The study recorded the concentrations of ∑73 PFAS as follows: Greenland (effluent–116 ng/L, biosolid 11 ng/g), Iceland (effluent–59, biosolids 4.6 ng/g) Faroe Islands (effluent–40 ng/L, biosolids—NA), Norway (effluent–44 ng/L, biosolids–75 ng/g), Denmark (effluent–45 ng/L, biosolids–142 ng/g) Sweden (effluent–163 ng/L, biosolids −102 ng/g) and Finland (effluent–55 ng/L, biosolids–129 ng/g). This study confirmed the dominance of PFCA precursors, while diPAP accounted for 62% of ∑73 PFAS. The study further evaluated the concentration of extractable organofluorine (EOF) by combustion ion chromatography analysis in influent, effluent and sewage sludge. The ∑EOF recorded was relatively higher (324–1,460 ng F/L in effluent and 39–210 ng F/g dry weight in sewage sludge) than those recorded for the sum of individual PFAS. The study affirmed that 90% of the ∑EOF recorded could not be accounted for by the ∑73 PFAS concentration recorded in the study, indicating the presence of some oxidizable precursor PFAS in the wastewater stream (Aro et al., 2021).
In the United Kingdom, Rigby et al. (2021) evaluated the concentration of PFAS in biosolids recycled to agricultural soils in the UK. The analyzed biosolids were generated from both industrial and municipal WWTPs. The study reported concentrations ranging from 99 to 231 μg kg−1 for ∑9 PFAS, with PFOS, PFDA and PFBS being the dominant species.
Despite the detection of PFAS, especially PFOA and PFOS across aquatic systems (Ololade et al., 2018; Ssebugere et al., 2020) and human samples (Hanssen et al., 2010) in Africa, the sources of these contaminants have not been precisely identified. Until now, little data on PFAS emanate from Africa, especially for PFAS concentrations in sewage sludge. Sindiku et al. (2013) have evaluated PFAS concentrations in sludge generated from 10 WWTP across the western part of Nigeria and affirmed that PFAS were detected in all industrial, domestic, and hospital sewage sludge analyzed. PFOS was confirmed to be the prevalent PFAS in all sludge samples, with concentrations varying from 101 to 540 μg kg−1.
The India PFAS Situation Report in 2019 stated that PFAS use in India is unregulated (PFAS, 2019) consequently causing a significant level of PFAS in rivers (Sharma et al., 2016), groundwater (Lapworth et al., 2018) and aquatic biota (Murakami et al., 2011). Indiscriminate disposal of sewage sludge can be linked to this PFAS trend in India, as less importance has been placed on PFAS detection in sewage sludge (Kumari et al., 2023).
Bangladesh, Lebanon, Egypt, Indonesia, Jordan, Malaysia, Nepal, Vietnam, Sri Lanka, and Thailand joined the Stockholm Convention regulations on PFOS in 2009. However, these countries only regulated PFOS, while other PFAS chemical species remain unregulated. Furthermore, the regulations on PFOS and PFOA have not been implemented in these nations. Consequently, reports from these countries revealed the prevalence of PFAS in human samples, air, land, water, and aquatic life (IPEN, 2019). To our knowledge, no data is available for PFAS concentration in biosolids generated from these countries.
Reports from South America revealed PFAS pollution of both drinking water, surface, and groundwater (Kaboré et al., 2018; Souza et al., 2022). In Brazil, the concentration of ∑23 PFAS in an urban area surface and groundwater was found to range from 11 to 17 ng L−1 and 22–718 ng L−1, respectively (Stefano et al., 2023). However, no data was found for assessing PFAS in the biosolids from these nations.
3.2 The prevalence of PFAAs and diPAPs in sewage sludge and biosolids
A number of studies reviewed have consistently reported increased levels of perfluoroalkyl acids (PFAAs) during the wastewater treatment plant (WWTP) processes. This phenomenon has been attributed to either the biotransformation or oxidation of polyfluoroalkyl precursor compounds within WWTPs (Eriksson et al., 2017; Coggan et al., 2019; Zhang et al., 2021; Thompson et al., 2022). As terminal degradation products of precursor PFAS compounds, PFAAs resist degradation in WWTPs owing to their stability to both chemical and biological degradation processes (Starnes et al., 2022). The bulk of these contaminants, particularly the short-chain PFAA, infiltrate the receiving waters through the treated wastewater (Ulrich et al., 2016). Hence, WWTPs are an important contributor of PFAAs into aquatic environments (Ulrich et al., 2016; Thompson et al., 2022).
The high concentrations of PFAS observed in biosolids have been ascribed to the partial adsorption of long-chain PFAAs to sewage sludge/biosolids, attributed to their elevated affinity for the proteins and organic components of the biowastes. This makes biosolids and sewage sludge a significant source of PFAAs and potential transfer to the terrestrial environment if applied to soils (Ulrich et al., 2016).
PFOS has been widely reported as the dominant PFAS in sewage sludge (Ulrich et al., 2016; Fredriksson et al., 2022; Thompson et al., 2022). Alder and van der Voet studied the predominance of PFOS in sewage sludge generated from 45 wastewater treatment facilities in Switzerland collected between 2008 and 2011. The study reported concentrations ranging from 4–2,480 μg kg−1 for ∑PFAAs (C4–C8), while PFOS was always the predominant PFAS detected with a concentration varying between 4 and 2,440 μg kg−1 (Alder and Van Der Voet, 2015).
Semerád et al. (2020) evaluated 32 PFAS in sewage sludge sourced from the WWTPs of 43 communities in the Czech Republic from 2018 to 2019. Their analysis revealed overall PFAS concentrations ranging from 5.6 to 963 ng/g. Notably, PFOS emerged as the predominant PFAS with a concentration of 933 ng/g (Semerád et al., 2020). The study also detected GenX in 9 samples, validating the use pattern and prevalence of new PFAS in the environment.
In activated sewage sludge reactors, the high PFOS adsorption to sewage sludge has been explained by comparing the partition coefficients (log Kd) of PFOS and PFOA between sludge and wastewater. A slightly higher partition coefficient obtained for PFOS (3.4 L kg−1) compared to PFOA (2.6 L kg−1) validates higher PFOS in sewage sludge compared to PFOA (Sun et al., 2012). However, Arvaniti et al. (2012) obtained somewhat divergent log Kd values (PFOS–2.8, PFOA–3.1), indicating that sludge properties are distinct and can affect the sorption of PFAS. To support this divergent report, an assessment of PFAS pollution in sewage sludge collected in 2011 from 25 WWTPs in Shanghai, China, revealed the presence of 14 perfluoroalkyl acids (PFAAs) with varying carbon chain length (C3–C14) at concentrations ranging from 126 to 809 ng/g. Perfluorooctanoic acid (PFOA) was also affirmed to be the dominant PFAS in this study, with concentrations ranging from 23 to 298 ng/g (Yan et al., 2012).
Guo et al., 2010, recorded a PFAS concentration of 278–5,383 ng/g in sewage sludge collected from 16 WWTPs across China in 2004 and affirmed that the data represents a good distribution of PFAS in China (Guo et al., 2010). The study found varying concentrations of PFOS and PFOA across different locations, with some areas having higher levels of PFOA and others having higher levels of PFOS (Guo et al., 2010).
While older studies mainly focused on PFAAs with few exceptions, recent reports have pointed to the frequent detection and prevalence of an unexpected PFAS class called disubstituted polyfluoroalkyl phosphates esters (diPAPs). This group of PFAS is commonly used in personal care products, surface protection applications and as coating agents in food packaging. As far back as 2009, D’eon et al. (2009) detected diPAPs in paper fibers from paper mills collected from Ontario in 2002 and 2003. The study recorded diPAP concentrations ranging from 34 ± 30 to 2,200 ± 400 ng/g.
In a recent study, Thompson et al. (2023a) detected diPAPs in toilet papers collected from North America, South and Central America, Africa, and Western Europe. Out of the 34 PFAS analyzed by the study, only 3 PFAAs and 3 diPAPs were detected in the toilet paper samples, with diPAPs accounting for 91% ± 8% of the total mass of PFAS in the toilet paper samples. The detection of diPAPs in various products implies their potential occurrence in sewage sludge. Reports from Sweden (Eriksson et al., 2017), Hong Kong (Loi et al., 2013), Canada (D’eon et al., 2009), US (Thompson et al., 2023a), and Australia (Moodie et al., 2021) confirmed the occurrence and the prevalence of diPAPs in sewage sludge.
While D’eon et al. (2009) recorded a concentration ranging from 47 ± 22 to 200 ± 130 ng/g for diPAP in sewage sludge from Ontario, Canada. Thompson et al. (2023a) recorded concentrations ranging from 73 to 1,400 ng g–1 for Σ3diPAP making up 54% ± 15% of the ∑92PFA on average in sewage sludge sourced from Florida (US) in 2021.
Schaefer et al. (2022) investigated the occurrence of 54 PFAS compounds sourced from 5 WWTPs in the US. The study recorded a total PFAS concentration varying from 323 ± 14 to 1,100 ± 44 ng/g, with diPAPs being the predominant PFAS in the biosolids. Moodie et al. (2021) also confirmed the dominance of diPAPs in Australian sewage sludge with a mean concentration of 140 ng/g dw. Loi et al. (2013) reported a relatively high concentration of diPAPS in sewage sludge and influent samples from Hong Kong in 2012, contributing between 40% and 95% to the total PFAS concentration in these matrices. The prevalence of diPAPs at high concentrations in sewage sludge suggests their widespread utilization and consumption in daily life.
3.3 Drivers of PFAS variations in sewage sludge/biosolids
Globally, the manufacture and use of PFAS are governed differently from nation to nation. Variations in the utilization of products containing PFAS and their precursors among nations ultimately produce dissimilarities in the occurrence of PFAS in their biosolids. Additionally, the configurations of the proximal wastewater treatment facilities and the sludge treatment methods can be a significant source of disparity. For instance, Guerra et al. (2014) confirmed that forming PFAAs in WWTP depends on both system temperature and treatment methods, with higher formation rates in biological systems operating with extended hydraulic retention times and increased temperature.
Kim Lazcano et al. (2019) studied the effect of different sewage sludge treatment processes. They affirmed that there was an increased PFAA concentration using heat treatment and composting sewage treatment methods, while the thermal hydrolysis process had no noticeable influence on PFAA concentrations (Kim Lazcano et al., 2019). The study concluded that sources of PFAS contribute more to PFAS concentrations in biosolids than treatment processes. Thompson et al. (2023b), in a recent study, confirmed the prevalence of PFAAs in biosolids after anaerobic digestion, heat treatment, composting and lime treatment of the sewage sludge. The study recorded a decrease from Σ92PFAS = 1,650 ng/g in the raw biosolids to 584 ng/g in biosolids treated with anaerobic treatment and belt press dewatering (65% decrease). Anaerobic treatment with heat drying used in another WWTP increased the concentration of Σ92PFAS in the treated biosolids by 102%. The study explained that the belt-press dewatering process mechanically eliminates (extracts) mobile PFAS alongside the water content, whereas heat drying eliminates water through evaporation. The heat treatment results in the absorption of dissolved PFAS in the solid fraction, increasing the concentration of PFAS due to PFAA precursor transformation (Thompson et al., 2023b). To support this claim, Zhang et al. (2021) reported an increased concentration of extractable PFAAs in hydrothermally treated sludge at 165°C for 0.5/2 h and 250°C for 0.5 h. A complete degradation of PFCAs and an increase in PFSAs were observed at 300°C. Adding Ca(OH)2 to hydrothermal treatment eliminated PFAA precursors and all PFSAs at 300°C, but substantially increased extractable PFAAs, except PFHpA and PFHxS (Zhang et al., 2021).
In a more elaborate study, Ebrahimi et al. (2021) investigated the influence of several solution-specific characteristics (pH, ionic strength), sludge characteristics (organic matter, lipids and protein content), PFAS characteristics (chain length, functional group), treatment and sludge stabilization methods on PFAS partitioning to sewage sludge. The study recorded an elevated PFAS concentration in sewage sludge at three distinct pH values–6, 7, and 8. This trend of increased PFAS sorption in more acidic conditions was also reported in another study (Zhang et al., 2013). The high sorption of PFAS to biosolids at elevated pH has been attributed to the reduced negative surface charge of the sludge at more acidic pH levels (Ebrahimi et al., 2021).
At elevated cation concentrations, the study also showed an increased PFAS sorption to biosolids, with results supported by other studies (Chen et al., 2012; Zhang et al., 2013). This was ascribed to bridging mechanisms in which a divalent cation uses electrostatic attraction to bind with the negatively charged surfaces of the biosolids and the functional group of an anionic PFAS (Ebrahimi et al., 2021). High PFAS sorption to biosolids has also been linked to increased fluoroalkyl chain length, with PFSA compounds having greater sorption capacities compared to PFCA compounds of similar chain length (Coggan et al., 2019; Ebrahimi et al., 2021).
3.4 Regulations and advisory levels of PFAS in sewage sludge/biosolids
The Stockholm Convention was adopted by the international community in 2004 as an environmental protection strategy against persistent organic pollutants (POPs), and PFOA was added to the list in 2009. The convention has been ratified by 152 countries on all inhabited continents.
Between 2016 and 2022, the EPA placed the upper limits for PFOA and PFOS in drinking water at 70 ng/L. Recently, new limits have been adopted at 4 ng/L each for both PFOA and PFOS, 10 ng/L for PFNA, PFHxS, and GenX and a summation risk index for PFNA, PFHxS, GenX, and PFBS, following their initial advisory levels showing that the scientific evidence that practically no PFAS exposure level is safe (Sauvé et al., 2023; USEPA, 2024).
The upper limit of total PFAS in drinking water in Canada has been proposed to be 30 ng/L for ∑30 PFAS (Sauvé et al., 2023), while the European Union placed the upper limit at 100 ng/L for ∑20 PFAS and 500 ng/L for ∑total PFAS in drinking water (EU, 2022), China established limits only for PFOS and PFOA at 40 and 80 ng/L, respectively, in drinking water (Wang et al., 2023). However, the upper limit for PFAS in biosolids suitable for application on agricultural land has hardly been addressed.
A literature survey revealed that many countries, including China, India, Singapore, Central and South America, Japan, South Korea, and Africa, have no existing regulations on the upper limit of PFAS in biosolids before disposal or land application. In the United States, the EPA has established Regional Screening Levels (RSLs) for six PFAS compounds in soils (Table 2). However, these RSLs serve as guidance levels and do not carry legal obligations. Aside from the federal regulations on PFAS in soil, several states in the US have issued state RSLs for residential and industrial soils (Table 3). As of the time of this report, only Maine, New York and Michigan State have an existing rule on a few PFAS compounds in biosolids to be applied on agricultural land and biosolid-amended soil (Table 2).
As an interim strategy in Michigan, The Environment, Great Lakes, and Energy (EGLE) has developed a protocol for WWTPs whose biosolids may be contaminated by PFAS through industrial discharges. Michigan State has stipulated that biosolids with ≥50 ng/g PFOS require remedial actions before reuse, while biosolids with ≥125 ng/g PFOS are prohibited from reuse on agricultural lands. A similar strategy is currently in place in New York State with a limit of 20 ng/g for PFOA and 20 ng/g for PFOS in biosolids requiring remediation before land application, while biosolids with a concentration of ≥50 ng/g for PFOA and ≥50 ng/g for PFOS should not be land applied (Maine Department of Environmental Protection, 2024).
In 2009, the upper limit for PFAS in biosolids and soil was set at 100 ng/g in Germany due to recorded PFAS pollution that was explicitly linked to the land application of biosolids.
In 2019, the US’s Maine Department of Environmental Protection established a PFAS Task Force to evaluate PFAS contamination throughout the state and make recommendations. The task force, in their final report, placed the upper limit of perfluorobutane sulfonic acid (PFBS), perfluorooctane sulfonate (PFOS) and perfluorooctanoic acid (PFOA) in biosolids at 1,900, 5.2, and 2.5 μg/kg dry weight respectively (Maine PFAS Report, 2020). However, the agricultural land application of biosolids was later totally forbidden in Maine (Maine Bill, 1911).
Aside from the three regulations mentioned from Germany, Maine State, New York and Michigan State, all other global regulations emanating from different locations provide upper limits for a few PFAS in soils only, especially after biosolid application (Table 2). For instance, in Denmark (NICOLE, 2016), a limit of 400 μg/kg has been established for PFAS in soils, while in Sweden, the limit is set at 3 μg/kg for gardens and residential lands and 20 μg/kg for commercial and industrial land use (Swedishepa, 2017). Netherlands chose a different strategy by setting a stricter limit on PFOS and PFOA in soils than other countries [0.9 and 0.8 μg/kg, respectively (The Dutch National Institute for Public Health and the Environment RIVM, 2019)].
In Canada, the Environment and Climate Change released the Federal Environmental Quality Recommendations that proposed a 10 μg/kg limit for PFOS in agricultural soils (CCME, 2021).
In Australia, the limits for C9-C14 PFCAs, perfluoroalkyl sulfonamides, fluorotelomer sulfonic acids, PFOA and PFOS in soil after biosolid application were set at 10, 1, 4, 4, and 1 μg/kg by the Queensland End of Waste Code Biosolids in 2020 (End of Waste Code Biosolids, 2020).
4 Conclusion
There is a substantial correlation between a country’s level of development, industrialization and the prevalence of PFAS pollution in the environment. Basically, the development level in each country and the eventual disposal of more than 50% of biosolids generated globally to land, either through landfill or application to agricultural land, appear to be the primary local contribution to PFAS in soils globally. Potential atmospheric inputs distributed over large areas still need to be better assessed as rain and dry deposition are certainly contributing to low level large scale distribution of PFAS over soils. Albeit it is difficult to determine the actual levels of PFAS in developing countries, given the paucity of relevant data in these areas. This review article revealed a global, ubiquitous presence of PFAS in biosolids and little to no limits on the presence of PFAS in biosolids aimed for land application and introduction into our agri-food production. This does present a potential threat from the release of PFAS into the environment through agricultural land application, landfill or indiscriminate disposal of biosolids that are not usually systematically monitored for their levels of PFAS. The scant data on the occurrence of PFAS in biosolids worldwide reflects a lack of interest and recognition of the potential risks caused by PFAS in biosolids.
This review observed that only Germany, Maine, Michigan State, and the USA have established some form of limits for a few PFAS in biosolids intended for land application. Moreover, in countries with regulatory standards, there appears to be little uniformity in the approach taken to determine the limits for PFAS in biosolids or soils. For instance, while some countries adopt lower limits of ≤10 μg/kg for PFOS or PFOA for soils or biosolid-amended soils (Canada, Netherlands, and Australia), others adopted a rather higher concentration ≥100 μg/kg for the sum of FAS (Germany and the US).
A national database that records PFAS usage and its presence in consumer products, including foods, biosolids, and manufacturing and processes using PFAS can help track and prevent the release of PFAS into the environment. We must also better regulate the use of PFAS and prevent their release into wastewater and biosolids and the potential transfer to our food chain and underground water.
We must also implement better monitoring programs to document the presence and concentration of PFAS in biosolids and their production, management, and end-of-life, considering PFAS and the other emerging contaminants they contain.
Author contributions
TS: Conceptualization, Data curation, Formal Analysis, Investigation, Methodology, Validation, Visualization, Writing–original draft, Writing–review and editing. SS: Conceptualization, Project administration, Supervision, Validation, Visualization, Writing–review and editing.
Funding
The author(s) declare that financial support was received for the research, authorship, and/or publication of this article. This study was supported by the Natural Sciences and Engineering Research Council of Canada and by the Ministère de l’environnement, de la lutte aux changements climatiques, de la faune et des parcs du Québec.
Conflict of interest
The authors declare that the research was conducted in the absence of any commercial or financial relationships that could be construed as a potential conflict of interest.
Publisher’s note
All claims expressed in this article are solely those of the authors and do not necessarily represent those of their affiliated organizations, or those of the publisher, the editors and the reviewers. Any product that may be evaluated in this article, or claim that may be made by its manufacturer, is not guaranteed or endorsed by the publisher.
Supplementary material
The Supplementary Material for this article can be found online at: https://www.frontiersin.org/articles/10.3389/fenvc.2024.1383185/full#supplementary-material
References
Adu, O., Ma, X., and Sharma, V. K. (2023). Bioavailability, phytotoxicity and plant uptake of per-and polyfluoroalkyl substances (PFAS): a review. J. Hazard. Mater. 447, 130805. doi:10.1016/j.jhazmat.2023.130805
Alder, A. C., and Van Der Voet, J. (2015). Occurrence and point source characterization of perfluoroalkyl acids in sewage sludge. Chemosphere 129, 62–73. doi:10.1016/j.chemosphere.2014.07.045
Alshankiti, A., Adenew Degefa, Dr. B., Shagufta Gill, Dr., and Nurul Akhand, Dr. (2024). Sludge valorization feasibility study in United Arab Emirates. Available at: http://www.biosaline.org/.
ARCOWA (2018). Wastewater management and resource recovery in Vietnam: current status and opportunities. Available at: https://seaknowledgebank.net/sites/default/files/wastewater-management-and-resource-recovery-in-VietNam.pdf.
Armstrong, D. L., Lozano, N., Rice, C. P., Ramirez, M., and Torrents, A. (2016). Temporal trends of perfluoroalkyl substances in limed biosolids from a large municipal water resource recovery facility. J. Environ. Manag. 165, 88–95. doi:10.1016/j.jenvman.2015.09.023
Aro, R., Eriksson, U., Kärrman, A., Chen, F., Wang, T., and Yeung, L. W. Y. (2021). Fluorine mass balance analysis of effluent and sludge from nordic countries. ACS Est. Water 1 (9), 2087–2096. doi:10.1021/acsestwater.1c00168
Arvaniti, O. S., Andersen, H. R., Thomaidis, N. S., and Stasinakis, A. S. (2014). Sorption of perfluorinated compounds onto different types of sewage sludge and assessment of its importance during wastewater treatment. Chemosphere 111, 405–411. doi:10.1016/j.chemosphere.2014.03.087
Arvaniti, O. S., and Stasinakis, A. S. (2015). Review on the occurrence, fate and removal of perfluorinated compounds during wastewater treatment. Sci. Total Environ. 524–525, 81–92. doi:10.1016/j.scitotenv.2015.04.023
Arvaniti, O. S., Ventouri, E. I., Stasinakis, A. S., and Thomaidis, N. S. (2012). Occurrence of different classes of perfluorinated compounds in Greek wastewater treatment plants and determination of their solid–water distribution coefficients. J. Hazard. Mater. 239–240, 24–31. doi:10.1016/j.jhazmat.2012.02.015
Batarseh, M. (2011). “Sustainable use of wastewater and sludge in Jordan; residues of persistent organic pollutants, a review,” in Advanced water supply and wastewater treatment: a road to safer society and environment nato science for peace and security series C: environmental security Editors P. Hlavinek, I. Winkler, J. Marsalek, and I. Mahrikova (Dordrecht: Springer Netherlands), 219–226. doi:10.1007/978-94-007-0280-6_20
BCLP Client Intelligent (2023). PFAS update: state soil concentration regulations. Available at: https://www.bclplaw.com/en-US/events-insights-news/pfas-update-state-soil-concentration-regulations-july-2023.html.
Berhanu, A., Mutanda, I., Taolin, J., Qaria, M. A., Yang, B., and Zhu, D. (2023). A review of microbial degradation of per- and polyfluoroalkyl substances (PFAS): biotransformation routes and enzymes. Sci. Total Environ. 859, 160010. doi:10.1016/j.scitotenv.2022.160010
Birru, R. L., Liang, H.-W., Farooq, F., Bedi, M., Feghali, M., Haggerty, C. L., et al. (2021). A pathway level analysis of PFAS exposure and risk of gestational diabetes mellitus. Environ. Health 20 (1), 63. doi:10.1186/s12940-021-00740-z
Bittencourt, S., Serrat, B. M., Aisse, M. M., and Gomes, D. (2014). Sewage sludge usage in agriculture: a case study of its destination in the curitiba metropolitan region, Paraná, Brazil. Water Air Soil Pollut. 225 (9), 2074. doi:10.1007/s11270-014-2074-y
Brunn, H., Arnold, G., Körner, W., Rippen, G., Steinhäuser, K. G., and Valentin, I. (2023). PFAS: forever chemicals—persistent, bioaccumulative and mobile. Reviewing the status and the need for their phase out and remediation of contaminated sites. Environ. Sci. Eur. 35 (1), 20. doi:10.1186/s12302-023-00721-8
Campo, J., Masiá, A., Picó, Y., Farré, M., and Barceló, D. (2014). Distribution and fate of perfluoroalkyl substances in mediterranean Spanish sewage treatment plants. Sci. Total Environ. 472, 912–922. doi:10.1016/j.scitotenv.2013.11.056
Canadian Council of Ministers of the Environment (2012). Canada-wide approach for the management of wastewater biosolids. Available at: https://ccme.ca/en/res/biosolids_cw_approach_e.pdf.
CCME (2021). Canadian soil and groundwater quality guidelines for the protection of environmental and human health. Available at: https://ccme.ca/en/res/pfosfactsheeten.pdf.
Chen, H., Zhang, C., Yu, Y., and Han, J. (2012). Sorption of perfluorooctane sulfonate (PFOS) on marine sediments. Mar. Pollut. Bull. 64 (5), 902–906. doi:10.1016/j.marpolbul.2012.03.012
Chen, W., Liu, J., Zhu, B.-H., Shi, M.-Y., Zhao, S.-Q., He, M.-Z., et al. (2022). The GHG mitigation opportunity of sludge management in China. Environ. Res. 212, 113284. doi:10.1016/j.envres.2022.113284
Chirikona, F., Filipovic, M., Ooko, S., and Orata, F. (2015). Perfluoroalkyl acids in selected wastewater treatment plants and their discharge load within the lake victoria basin in Kenya. Environ. Monit. Assess. 187 (5), 238. doi:10.1007/s10661-015-4425-6
Clarke, R. M., and Cummins, E. (2015). Evaluation of “classic” and emerging contaminants resulting from the application of biosolids to agricultural lands: a review. Hum. Ecol. Risk Assess. Int. J. 21 (2), 492–513. doi:10.1080/10807039.2014.930295
Coggan, T. L., Moodie, D., Kolobaric, A., Szabo, D., Shimeta, J., Crosbie, N. D., et al. (2019). An investigation into per- and polyfluoroalkyl substances (PFAS) in nineteen Australian wastewater treatment plants (WWTPs). Heliyon 5 (8), e02316. doi:10.1016/j.heliyon.2019.e02316
Collivignarelli, M. C., Canato, M., Abbà, A., Miino, C., and Biosolids, M. (2019). What are the different types of reuse? J. Clean. Prod. 238, 117844. doi:10.1016/j.jclepro.2019.117844
CPCB (2021). India central pollution Control board. Available at: https://cpcb.nic.in/(Accessed June 29, 2023).
Dad, K., Wahid, A., Khan, A. A., Anwar, A., Ali, M., Sarwar, N., et al. (2019). Nutritional status of different biosolids and their impact on various growth parameters of wheat (Triticum aestivum L). Saudi J. Biol. Sci. 26 (7), 1423–1428. doi:10.1016/j.sjbs.2018.09.001
D’eon, J. C., Crozier, P. W., Furdui, V. I., Reiner, E. J., Libelo, E. L., and Mabury, S. A. (2009). Observation of a commercial fluorinated material, the polyfluoroalkyl phosphoric acid diesters, in human sera, wastewater treatment plant sludge, and paper fibers. Environ. Sci. Technol. 43 (12), 4589–4594. doi:10.1021/es900100d
Dinglasan, M. J. A., Ye, Y., Edwards, E. A., and Mabury, S. A. (2004). Fluorotelomer alcohol biodegradation yields poly- and perfluorinated acids. Environ. Sci. Technol. 38 (10), 2857–2864. doi:10.1021/es0350177
Ebrahimi, F., Lewis, A. J., Sales, C. M., Suri, R., and McKenzie, E. R. (2021). Linking PFAS partitioning behavior in sewage solids to the solid characteristics, solution Chemistry, and treatment processes. Chemosphere 271, 129530. doi:10.1016/j.chemosphere.2020.129530
End of Waste Code Biosolids. (2020). The Queensland end of waste Code biosolids in 2020. Available at: https://info.awa.asn.au/.
Eriksson, U., Haglund, P., and Kärrman, A. (2017). Contribution of precursor compounds to the release of per- and polyfluoroalkyl substances (PFASs) from waste water treatment plants (WWTPs). J. Environ. Sci. 61, 80–90. doi:10.1016/j.jes.2017.05.004
EU (2022). Amending regulation (EC) No 1881/2006 as regards maximum levels of perfluoroalkyl substances in certain foodstuffs. Available at: https://eur-lex.europa.eu/legal-content/EN/TXT/PDF/?uri=CELEX:32022R2388.
Eurostat (2022). Sewage sludge production and disposal from urban wastewater in European countries. Available at: https://ec.europa.eu/eurostat/databrowser/view/TEN00030/default/table?lang=en&category=env.env_wat.env_nwat.
Fenton, S. E., Ducatman, A., Boobis, A., DeWitt, J. C., Lau, C., Ng, C., et al. (2021). Per- and polyfluoroalkyl substance toxicity and human health review: current state of knowledge and strategies for informing future research. Environ. Toxicol. Chem. 40 (3), 606–630. doi:10.1002/etc.4890
Fredriksson, F., Eriksson, U., Kärrman, A., and Yeung, L. W. Y. (2022). Per- and polyfluoroalkyl substances (PFAS) in sludge from wastewater treatment plants in Sweden — first findings of novel fluorinated copolymers in Europe including temporal analysis. Sci. Total Environ. 846, 157406. doi:10.1016/j.scitotenv.2022.157406
Gallen, C., Eaglesham, G., Drage, D., Nguyen, T. H., and Mueller, J. F. (2018). A mass estimate of perfluoroalkyl substance (PFAS) release from Australian wastewater treatment plants. Chemosphere 208, 975–983. doi:10.1016/j.chemosphere.2018.06.024
Gianico, A., Braguglia, C., Gallipoli, A., Montecchio, D., and Mininni, G. (2021). Land application of biosolids in Europe: possibilities, con-straints and future perspectives. Water 13 (1), 103. doi:10.3390/w13010103
Grandjean, P., Heilmann, C., Weihe, P., Nielsen, F., Mogensen, U. B., Timmermann, A., et al. (2017). Estimated exposures to perfluorinated compounds in infancy predict attenuated vaccine antibody concentrations at age 5-years. J. Immunotoxicol. 14 (1), 188–195. doi:10.1080/1547691X.2017.1360968
Guerra, P., Kim, M., Kinsman, L., Ng, T., Alaee, M., and Smyth, S. A. (2014). Parameters affecting the formation of perfluoroalkyl acids during wastewater treatment. J. Hazard. Mater. 272, 148–154. doi:10.1016/j.jhazmat.2014.03.016
Guo, R., Sim, W.-J., Lee, E.-S., Lee, J.-H., and Oh, J.-E. (2010). Evaluation of the fate of perfluoroalkyl compounds in wastewater treatment plants. Water Res. 44 (11), 3476–3486. doi:10.1016/j.watres.2010.03.028
Gworek, B., Kijeńska, M., Wrzosek, J., and Graniewska, M. (2021). Pharmaceuticals in the soil and plant environment: a review. Water Air Soil Pollut. 232 (4), 145. doi:10.1007/s11270-020-04954-8
Hanssen, L., Röllin, H., Odland, J. Ø., Moe, M. K., and Sandanger, T. M. (2010). Perfluorinated compounds in maternal serum and cord blood from selected areas of South Africa: results of a pilot study. J. Environ. Monit. 12 (6), 1355. doi:10.1039/b924420d
Houtz, E. F., Wang, M., and Park, J.-S. (2018). Identification and fate of aqueous film forming foam derived per- and polyfluoroalkyl substances in a wastewater treatment plant. Environ. Sci. Technol. 52 (22), 13212–13221. doi:10.1021/acs.est.8b04028
Hušek, M., Moško, J., and Pohořelý, M. (2022). Sewage sludge treatment methods and P-recovery possibilities: current state-of-the-art. J. Environ. Manag. 315, 115090. doi:10.1016/j.jenvman.2022.115090
IPEN (2019). PFAS pollution across the Middle East and Asia. Available at: https://ipen.org/documents/pfas-pollution-across-middle-east-and-asia.
Kaboré, H. A., Vo Duy, S., Munoz, G., Méité, L., Desrosiers, M., Liu, J., et al. (2018). Worldwide drinking water occurrence and levels of newly-identified perfluoroalkyl and polyfluoroalkyl substances. Sci. Total Environ. 616–617, 1089–1100. doi:10.1016/j.scitotenv.2017.10.210
Kim, S.-K., Im, J.-K., Kang, Y.-M., Jung, S.-Y., Kho, Y. L., and Zoh, K.-D. (2012). Wastewater treatment plants (WWTPs)-Derived national discharge loads of perfluorinated compounds (PFCs). J. Hazard. Mater. 201–202, 82–91. doi:10.1016/j.jhazmat.2011.11.036
Kim Lazcano, R., Perre, C., Mashtare, M. L., and Lee, L. S. (2019). Per- and polyfluoroalkyl substances in commercially available biosolid-based products: the effect of treatment processes. Water Environ. Res. 91 (12), 1669–1677. doi:10.1002/wer.1174
Kumar, R., Vuppaladadiyam, A. K., Antunes, E., Whelan, A., Fearon, R., Sheehan, M., et al. (2022). Emerging contaminants in biosolids: presence, fate and analytical techniques. Emerg. Contam. 8, 162–194. doi:10.1016/j.emcon.2022.03.004
Kumar, V., Chopra, A. K., and Kumar, A. (2017). A review on sewage sludge (biosolids) a resource for sustainable agriculture. Arch. Agri. Environ. Sci. 2 (4), 340–347. doi:10.26832/24566632.2017.020417
Kumari, A., Singh Maurya, N., Kumar, A., Kant Yadav, R., and Kumar, A. (2023). “Options for the disposal and reuse of wastewater sludge, associated benefit, and environmental risk,” in Sustainable development vol. 5 Editor B. Kılıç Taşeli (United Kingdom: IntechOpen). doi:10.5772/intechopen.109410
Kunacheva, C., Tanaka, S., Fujii, S., Boontanon, S. K., Musirat, C., Wongwattana, T., et al. (2011). Mass flows of perfluorinated compounds (PFCs) in central wastewater treatment plants of industrial zones in Thailand. Chemosphere 83 (6), 737–744. doi:10.1016/j.chemosphere.2011.02.059
Kwon, H.-O., Kim, H.-Y., Park, Y.-M., Seok, K.-S., Oh, J.-E., and Choi, S.-D. (2017). Updated national emission of perfluoroalkyl substances (PFASs) from wastewater treatment plants in South Korea. Environ. Pollut. 220, 298–306. doi:10.1016/j.envpol.2016.09.063
Lakshminarasimman, N., Gewurtz, S. B., Parker, W. J., and Smyth, S. A. (2021). Removal and formation of perfluoroalkyl substances in Canadian sludge treatment systems – a mass balance approach. Sci. Total Environ. 754, 142431. doi:10.1016/j.scitotenv.2020.142431
Lapworth, D. J., Das, P., Shaw, A., Mukherjee, A., Civil, W., Petersen, J. O., et al. (2018). Deep urban groundwater vulnerability in India revealed through the use of emerging organic contaminants and residence time tracers. Environ. Pollut. 240, 938–949. doi:10.1016/j.envpol.2018.04.053
Lenka, S. P., Kah, M., and Padhye, L. P. (2021). A review of the occurrence, transformation, and removal of poly- and perfluoroalkyl substances (PFAS) in wastewater treatment plants. Water Res. 199, 117187. doi:10.1016/j.watres.2021.117187
Letcher, R. J., Chu, S., and Smyth, S.-A. (2020). Side-chain fluorinated polymer surfactants in biosolids from wastewater treatment plants. J. Hazard. Mater. 388, 122044. doi:10.1016/j.jhazmat.2020.122044
Leung, S. C. E., Shukla, P., Chen, D., Eftekhari, E., An, H., Zare, F., et al. (2022). Emerging technologies for PFOS/PFOA degradation and removal: a review. Sci. Total Environ. 827, 153669. doi:10.1016/j.scitotenv.2022.153669
Link, G. W., Reeves, D. M., Cassidy, D. P., and Coffin, E. S. (2024). Per- and polyfluoroalkyl substances (PFAS) in final treated solids (biosolids) from 190 Michigan wastewater treatment plants. J. Hazard. Mater. 463, 132734. doi:10.1016/j.jhazmat.2023.132734
Liu, W., Iordan, C. M., Cherubini, F., Hu, X., and Fu, D. (2021). Environmental impacts assessment of wastewater treatment and sludge disposal systems under two sewage discharge standards: a case study in kunshan, China. J. Clean. Prod. 287, 125046. doi:10.1016/j.jclepro.2020.125046
Liu, Y., Wosu, A. C., Fleisch, A. F., Dunlop, A. L., Starling, A. P., Ferrara, A., et al. (2023). Associations of gestational perfluoroalkyl substances exposure with early childhood BMI z-scores and risk of overweight/obesity: results from the ECHO cohorts. Environ. Health Perspect. 131 (6), 067001. doi:10.1289/EHP11545
Loi, E. I. H., Yeung, L. W. Y., Mabury, S. A., and Lam, P. K. S. (2013). Detections of commercial fluorosurfactants in Hong Kong marine environment and human blood: a pilot study. Environ. Sci. Technol. 47 (9), 4677–4685. doi:10.1021/es303805k
Ma, R., and Shih, K. (2010). Perfluorochemicals in wastewater treatment plants and sediments in Hong Kong. Environ. Pollut. 158 (5), 1354–1362. doi:10.1016/j.envpol.2010.01.013
Maine Bill, L. D. (1911). State of Maine state of Maine 130th legislature second regular session. Available at: https://legislature.maine.gov/legis/bills/getPDF.asp?paper=HP1417&item=7&snum=130.
Maine Department of Environmental Protection (2024). An evaluation of biosolids management in Maine and recommendations for the future. United States: Final Report. Available at: https://www.maine.gov/tools/whatsnew/attach.php?id=12198306&an=1 (Accessed January 21, 2024).
Maine PFAS Report (2020). Managing PFAS in Maine. Available at: https://www1.maine.gov/pfastaskforce/materials/report/PFAS-Task-Force-Report-FINAL-Jan2020.pdf.
Marchuk, S., Tait, S., Sinha, P., Harris, P., Antille, D. L., and McCabe, B. K. (2023). Biosolids-derived fertilisers: a review of challenges and opportunities. Sci. Total Environ. 875, 162555. doi:10.1016/j.scitotenv.2023.162555
Masoner, J. R., Kolpin, D. W., Cozzarelli, I. M., Smalling, K. L., Bolyard, S. C., Field, J. A., et al. (2020). Landfill leachate contributes per-/poly-fluoroalkyl substances (PFAS) and pharmaceuticals to municipal wastewater. Environ. Sci. Water Res. Technol. 6 (5), 1300–1311. doi:10.1039/D0EW00045K
Meegoda, J. N., Kewalramani, J. A., Li, B., and Marsh, R. W. (2020). A review of the applications, environmental release, and remediation technologies of per- and polyfluoroalkyl substances. IJERPH 17 (21), 8117. doi:10.3390/ijerph17218117
Michigan Department of Environment, Great Lakes, and Energy (EGLE) (2020). Initiatives to evaluate the presence of PFAS in municipal wastewater and associated residuals (Sludge/Biosolids) in Michigan. Available at: https://www.michigan.gov/documents/egle/wrd-pfas-initiatives_691391_7.pdf.
Michigan Department of Environment, Great Lakes, and Energy (EGLE) (2022). Biosolids and PFAS: quick facts for landowners/farmers. Available at: https://www.michigan.gov/-/media/Project/Websites/egle/Documents/Programs/WRD/Biosolids/biosolids-pfas-facts-landowners-farmers.pdf?rev=641c693bd1c24188a5a14a83704302cf.
Miller, K. (2022). ‘Complete crisis’ as PFAS discovery upends life and livelihood of young Maine farming family, Maine public (feb. 7, 2022). Available at: https://www.mainepublic.org/environment-and-outdoors/2022-02-07/complete-crisisas-pfas-discovery-upends-life-and-livelihood-of-young-maine-farming-family.
Mohajerani, A., Lound, S., Liassos, G., Kurmus, H., Ukwatta, A., and Nazari, M. (2017). Physical, mechanical and chemical properties of biosolids and raw Brown coal fly ash, and their combination for road structural fill applications. J. Clean. Prod. 166, 1–11. doi:10.1016/j.jclepro.2017.07.250
Moodie, D., Coggan, T., Berry, K., Kolobaric, A., Fernandes, M., Lee, E., et al. (2021). Legacy and emerging per- and polyfluoroalkyl substances (PFASs) in Australian biosolids. Chemosphere 270, 129143. doi:10.1016/j.chemosphere.2020.129143
Munoz, G., Michaud, A. M., Liu, M., Vo Duy, S., Montenach, D., Resseguier, C., et al. (2022). Target and nontarget screening of PFAS in biosolids, composts, and other organic waste products for land application in France. Environ. Sci. Technol. 56 (10), 6056–6068. doi:10.1021/acs.est.1c03697
Murakami, M., Adachi, N., Saha, M., Morita, C., and Takada, H. (2011). Levels, temporal trends, and tissue distribution of perfluorinated surfactants in freshwater fish from asian countries. Arch. Environ. Contam. Toxicol. 61 (4), 631–641. doi:10.1007/s00244-011-9660-4
Navarro, I., Sanz, P., and Martínez, M. Á. (2011). Analysis of perfluorinated alkyl substances in Spanish sewage sludge by liquid chromatography–tandem mass spectrometry. Anal. Bioanal. Chem. 400 (5), 1277–1286. doi:10.1007/s00216-011-4655-6
NEMP (2020). PFAS national environmental management plan, Australia. Available at: https://www.dcceew.gov.au/environment/protection/publications/pfas-nemp-2.
Nguyen, T. M. H., Bräunig, J., Thompson, K., Thompson, J., Kabiri, S., Navarro, D. A., et al. (2020). Influences of chemical properties, soil properties, and solution pH on soil–water partitioning coefficients of per- and polyfluoroalkyl substances (PFASs). Environ. Sci. Technol. 54 (24), 15883–15892. doi:10.1021/acs.est.0c05705
NICOLE (2016). Network for industrially contaminated land in Europe, (NICOLE 2016), environmental fate and effects of poly and perfluoroalkyl substances (PFAS). Available at: https://www.concawe.eu/wp-content/uploads/2016/06/Rpt_16-8.pdf.
Ojo, A. F., Peng, C., and Ng, J. C. (2021). Assessing the human health risks of per- and polyfluoroalkyl substances: a need for greater focus on their interactions as mixtures. J. Hazard. Mater. 407, 124863. doi:10.1016/j.jhazmat.2020.124863
Ololade, I. A., Oladoja, N. A., Ololade, O. O., Oloye, F. F., Adeola, A. O., Alabi, A. B., et al. (2018). Geographical distribution of perfluorooctanesulfonate and perfluorooctanoate in selected rivers from Nigeria. J. Environ. Chem. Eng. 6 (4), 4061–4069. doi:10.1016/j.jece.2018.06.020
Onu, M. A., Ayeleru, O. O., Oboirien, B., and Olubambi, P. A. (2023). Challenges of wastewater generation and management in sub-saharan Africa: a review. Environ. Challenges 11, 100686. doi:10.1016/j.envc.2023.100686
Organisation for Economic Co-Operation and Development (OECD) (2017). Perfluoroalkylated substances: PFOA, PFOS and PFOSA - evaluation of health hazards and proposal of a health based quality criterion for drinking water, soil and ground water report in Denmark. Available at: https://www.oecd.org/chemicalsafety/portal-perfluorinated-chemicals/countryinformation/denmark.htm.
PFAS (2019). The India PFAS situation report- 2019. Available at: https://ipen.org/sites/default/files/documents/india_pfas_country_situation_report_mar_2019.pdf.
Rappazzo, K., Coffman, E., and Hines, E. (2017). Exposure to perfluorinated alkyl substances and health outcomes in children: a systematic review of the epidemiologic literature. IJERPH 14 (7), 691. doi:10.3390/ijerph14070691
Rathankumar, A. K., Saikia, K., Nagarajan, K. T., Vaithyanathan, V. K., Vaidyanathan, V. K., and Cabana, H. (2020). Development of efficient and sustainable added-value products from municipal biosolids through an industrially feasible process. J. Clean. Prod. 266, 121749. doi:10.1016/j.jclepro.2020.121749
Rickard, B. P., Rizvi, I., and Fenton, S. E. (2022). Per- and poly-fluoroalkyl substances (PFAS) and female reproductive outcomes: PFAS elimination, endocrine-mediated effects, and disease. Toxicology 465, 153031. doi:10.1016/j.tox.2021.153031
Rigby, H., Clarke, B. O., Pritchard, D. L., Meehan, B., Beshah, F., Smith, S. R., et al. (2016). A critical review of nitrogen mineralization in biosolids-amended soil, the associated fertilizer value for crop production and potential for emissions to the environment. Sci. Total Environ. 541, 1310–1338. doi:10.1016/j.scitotenv.2015.08.089
Rigby, H., Dowding, A., Fernandes, A., Humphries, D., Jones, N. R., Lake, I., et al. (2021). Concentrations of organic contaminants in industrial and municipal bioresources recycled in agriculture in the UK. Sci. Total Environ. 765, 142787. doi:10.1016/j.scitotenv.2020.142787
Riva, F., Zuccato, E., Pacciani, C., Colombo, A., and Castiglioni, S. (2021). A multi-residue analytical method for extraction and analysis of pharmaceuticals and other selected emerging contaminants in sewage sludge. Anal. Methods 13 (4), 526–535. doi:10.1039/D0AY02027C
Rodgers, K. M., Swartz, C. H., Occhialini, J., Bassignani, P., McCurdy, M., and Schaider, L. A. (2022). How well do product labels indicate the presence of PFAS in consumer items used by children and adolescents? Environ. Sci. Technol. 56 (10), 6294–6304. doi:10.1021/acs.est.1c05175
Roth, K., and Petriello, M. C. (2022). Exposure to per- and polyfluoroalkyl substances (PFAS) and type 2 diabetes risk. Front. Endocrinol. 13, 965384. doi:10.3389/fendo.2022.965384
Ruan, T., Lin, Y., Wang, T., Liu, R., and Jiang, G. (2015). Identification of novel polyfluorinated ether sulfonates as PFOS alternatives in municipal sewage sludge in China. Environ. Sci. Technol. 49 (11), 6519–6527. doi:10.1021/acs.est.5b01010
Sauvé, S., Barbeau, B., Bouchard, M. F., Verner, M.-A., and Liu, J. (2023). How should we interpret the new water quality regulations for per- and polyfluoroalkyl substances? ACS Est. Water 3 (9), 2810–2815. doi:10.1021/acsestwater.3c00217
Schaefer, C. E., Hooper, J., Modiri-Gharehveran, M., Drennan, D. M., Beecher, N., and Lee, L. (2022). Release of poly- and perfluoroalkyl substances from finished biosolids in soil mesocosms. Water Res. 217, 118405. doi:10.1016/j.watres.2022.118405
Schaefer, C. E., Hooper, J. L., Strom, L. E., Abusallout, I., Dickenson, E. R. V., Thompson, K. A., et al. (2023). Occurrence of quantifiable and semi-quantifiable poly- and perfluoroalkyl substances in United States wastewater treatment plants. Water Res. 233, 119724. doi:10.1016/j.watres.2023.119724
Schillemans, T., Shi, L., Donat-Vargas, C., Hanhineva, K., Tornevi, A., Johansson, I., et al. (2021). Plasma metabolites associated with exposure to perfluoroalkyl substances and risk of type 2 diabetes – a nested case-control study. Environ. Int. 146, 106180. doi:10.1016/j.envint.2020.106180
Schultes, L., Vestergren, R., Volkova, K., Westberg, E., Jacobson, T., and Benskin, J. P. (2018). Per- and polyfluoroalkyl substances and fluorine mass balance in cosmetic products from the Swedish market: implications for environmental emissions and human exposure. Environ. Sci. Process. Impacts 20 (12), 1680–1690. doi:10.1039/C8EM00368H
Seltenrich, N. (2020). PFAS in food packaging: a hot, greasy exposure. Environ. Health Perspect. 128 (5), 054002. doi:10.1289/EHP6335
Semerád, J., Hatasová, N., Grasserová, A., Černá, T., Filipová, A., Hanč, A., et al. (2020). Screening for 32 per- and polyfluoroalkyl substances (PFAS) including GenX in sludges from 43 WWTPs located in the Czech republic - evaluation of potential accumulation in vegetables after application of biosolids. Chemosphere 261, 128018. doi:10.1016/j.chemosphere.2020.128018
Sevelsted, A., Gürdeniz, G., Rago, D., Pedersen, C.-E. T., Lasky-Su, J. A., Checa, A., et al. (2022). Effect of perfluoroalkyl exposure in pregnancy and infancy on intrauterine and childhood growth and anthropometry. Sub study from COPSAC2010 birth cohort. eBioMedicine 83, 104236. doi:10.1016/j.ebiom.2022.104236
Sharma, B., Sarkar, A., Singh, P., and Singh, R. P. (2017). Agricultural utilization of biosolids: a review on potential effects on soil and plant grown. Waste Manag. 64, 117–132. doi:10.1016/j.wasman.2017.03.002
Sharma, B. M., Bharat, G. K., Tayal, S., Larssen, T., Bečanová, J., Karásková, P., et al. (2016). Perfluoroalkyl substances (PFAS) in river and ground/drinking water of the ganges river basin: emissions and implications for human exposure. Environ. Pollut. 208, 704–713. doi:10.1016/j.envpol.2015.10.050
Sindiku, O., Orata, F., Weber, R., and Osibanjo, O. (2013). Per- and polyfluoroalkyl substances in selected sewage sludge in Nigeria. Chemosphere 92 (3), 329–335. doi:10.1016/j.chemosphere.2013.04.010
South Korea National Sewer Information System (2022). Sewerage information system. Available at: http://www.hasudoinfo.or.kr/.
Souza, M. C. O., Rocha, B. A., Adeyemi, J. A., Nadal, M., Domingo, J. L., and Barbosa, F. (2022). Legacy and emerging pollutants in Latin America: a critical review of occurrence and levels in environmental and food samples. Sci. Total Environ. 848, 157774. doi:10.1016/j.scitotenv.2022.157774
Ssebugere, P., Sillanpää, M., Matovu, H., Wang, Z., Schramm, K.-W., Omwoma, S., et al. (2020). Environmental levels and human body burdens of per- and poly-fluoroalkyl substances in Africa: a critical review. Sci. Total Environ. 739, 139913. doi:10.1016/j.scitotenv.2020.139913
Stahl, T., Gassmann, M., Falk, S., and Brunn, H. (2018). Concentrations and distribution patterns of perfluoroalkyl acids in sewage sludge and in biowaste in hesse, Germany. J. Agric. Food Chem. 66 (39), 10147–10153. doi:10.1021/acs.jafc.8b03063
Starnes, H. M., Rock, K. D., Jackson, T. W., and Belcher, S. M. (2022). A critical review and meta-analysis of impacts of per- and polyfluorinated substances on the brain and behavior. Front. Toxicol. 4, 881584. doi:10.3389/ftox.2022.881584
Stefano, P. H. P., Roisenberg, A., D’Anna Acayaba, R., Roque, A. P., Bandoria, D. R., Soares, A., et al. (2023). Occurrence and distribution of per-and polyfluoroalkyl substances (PFAS) in surface and groundwaters in an urbanized and agricultural area, southern Brazil. Environ. Sci. Pollut. Res. 30 (3), 6159–6169. doi:10.1007/s11356-022-22603-x
Sun, H., Zhang, X., Wang, L., Zhang, T., Li, F., He, N., et al. (2012). Perfluoroalkyl compounds in municipal WWTPs in tianjin, China—concentrations, distribution and mass flow. Environ. Sci. Pollut. Res. 19 (5), 1405–1415. doi:10.1007/s11356-011-0727-6
Swedishepa (2017). Sahlin. Available at: http://www.swedishepa.se/hazards (Accessed July 04, 2023).
Tavasoli, E., Luek, J. L., Malley, J. P., and Mouser, P. J. (2021). Distribution and fate of per- and polyfluoroalkyl substances (PFAS) in wastewater treatment facilities. Environ. Sci. Process. Impacts 23 (6), 903–913. doi:10.1039/D1EM00032B
Tessier (2017) Tessier. Available at: http://cwwa.ca/pdf_files/2017WOO/Tessier.pdf (Accessed June 29, 2023).
The Dutch National Institute for Public Health and the Environment (RIVM) (2019). Nitrogen and PFAS suddenly big societal issues in The Netherlands. Available at: https://www.rivm.nl/en/newsletter/content/2020/issue1/nitrogen-pfas-in-NL (Accessed July 04, 2023).
The Australian and New Zealand Biosolids Partnership (ANZBP) (2019). Fact sheet on biosolids and PFAS. Available at: https://www.biosolids.com.au/.
Thompson, J. T., Chen, B., Bowden, J. A., and Townsend, T. G. (2023a). Per- and polyfluoroalkyl substances in toilet paper and the impact on wastewater systems. Environ. Sci. Technol. Lett. 10 (3), 234–239. doi:10.1021/acs.estlett.3c00094
Thompson, J. T., Robey, N. M., Tolaymat, T. M., Bowden, J. A., Solo-Gabriele, H. M., and Townsend, T. G. (2023b). Underestimation of per- and polyfluoroalkyl substances in biosolids: precursor transformation during conventional treatment. Environ. Sci. Technol. 57 (9), 3825–3832. doi:10.1021/acs.est.2c06189
Thompson, K. A., Mortazavian, S., Gonzalez, D. J., Bott, C., Hooper, J., Schaefer, C. E., et al. (2022). Poly- and perfluoroalkyl substances in municipal wastewater treatment plants in the United States: seasonal patterns and meta-analysis of long-term trends and average concentrations. ACS Est. Water 2 (5), 690–700. doi:10.1021/acsestwater.1c00377
Ulrich, H., Freier, K. P., and Gierig, M. (2016). Getting on with persistent pollutants: decreasing trends of perfluoroalkyl acids (PFAAs) in sewage sludge. Chemosphere 161, 527–535. doi:10.1016/j.chemosphere.2016.07.048
UN-Habitat (2008). Global atlas of excreta, wastewater sludge, and biosolids management: moving forward the sustainable and welcome uses of a global resource. Available at: https://unhabitat.org/sites/default/files/download-manager-files/Global%20Atlas%20of%20Excreta%2C%20Wastewater%20Sludge%2C%20and%20Biosolids%20Management.pdf.
USEPA (2019). Biennial report No. 8 (reporting period 2018-2019). Available at: https://www.epa.gov/biosolids/biennial-report-no-8-reporting-period-2018-2019.
USEPA (2021). Standards for the use or disposal of sewage sludge (40 CFR Part 503). Available at: https://www.epa.gov/biosolids/biosolids-laws-and-regulations#standards.
USEPA (2024). Biden-harris administration finalizes first-ever national drinking water standard to protect 100M people from PFAS pollution. Available at: https://www.epa.gov/newsreleases/biden-harris-administration-finalizes-first-ever-national-drinking-water-standard#:∼:text=EPA%20is%20setting%20enforceable%20Maximum,are%20feasible%20for%20effective%20implementation.
Venegas, C., Sánchez-Alfonso, A. C., Celis, C., Vesga, F.-J., and Mendez, M. G. (2021). Management strategies and stakeholders analysis to strengthen the management and use of biosolids in a Colombian municipality. Sustainability 13 (21), 12180. doi:10.3390/su132112180
Venkatesan, A. K., and Halden, R. U. (2013). National inventory of perfluoroalkyl substances in archived U.S. Biosolids from the 2001 EPA national sewage sludge survey. J. Hazard. Mater. 252–253, 413–418. doi:10.1016/j.jhazmat.2013.03.016
Wackett, L. P. (2021). Why is the biodegradation of polyfluorinated compounds so rare? mSphere 6 (5), e0072121–21. doi:10.1128/mSphere.00721-21
Wang, M., Xue, J., Horswell, J., Kimberley, M. O., and Huang, Z. (2017). Long-term biosolids application alters the composition of soil microbial groups and nutrient status in a pine plantation. Biol. Fertil. Soils 53 (7), 799–809. doi:10.1007/s00374-017-1219-8
Wang, X., Zhang, H., He, X., Liu, J., Yao, Z., Zhao, H., et al. (2023). Contamination of per- and polyfluoroalkyl substances in the water source from a typical agricultural area in North China. Front. Environ. Sci. 10, 1071134. doi:10.3389/fenvs.2022.1071134
Wei, L., Zhu, F., Li, Q., Xue, C., Xia, X., Yu, H., et al. (2020). Development, current state and future trends of sludge management in China: based on exploratory data and CO2-equivaient emissions analysis. Environ. Int. 144, 106093. doi:10.1016/j.envint.2020.106093
Xiao, F. (2017). Emerging poly- and perfluoroalkyl substances in the aquatic environment: a review of current literature. Water Res. 124, 482–495. doi:10.1016/j.watres.2017.07.024
Yan, H., Zhang, C.-J., Zhou, Q., Chen, L., and Meng, X.-Z. (2012). Short- and long-chain perfluorinated acids in sewage sludge from Shanghai, China. Chemosphere 88 (11), 1300–1305. doi:10.1016/j.chemosphere.2012.03.105
Yosuke Matsumiya (2020). Management of sewage in Japan. Available at: http://sawea.org/pdf/2020/11-February-2020/Japan-Sewage-Works-Association.pdf (Accessed June 29, 2023).
Yu, J., Hu, J., Tanaka, S., and Fujii, S. (2009). Perfluorooctane sulfonate (PFOS) and perfluorooctanoic acid (PFOA) in sewage treatment plants. Water Res. 43 (9), 2399–2408. doi:10.1016/j.watres.2009.03.009
Zhang, C., Yan, H., Li, F., Hu, X., and Zhou, Q. (2013). Sorption of short- and long-chain perfluoroalkyl surfactants on sewage sludges. J. Hazard. Mater. 260, 689–699. doi:10.1016/j.jhazmat.2013.06.022
Zhang, W., Pang, S., Lin, Z., Mishra, S., Bhatt, P., and Chen, S. (2021). Biotransformation of perfluoroalkyl acid precursors from various environmental systems: advances and perspectives. Environ. Pollut. 272, 115908. doi:10.1016/j.envpol.2020.115908
Zhen, G., Lu, X., Kato, H., Zhao, Y., and Li, Y.-Y. (2017). Overview of pretreatment strategies for enhancing sewage sludge disintegration and subsequent anaerobic digestion: current advances, full-scale application and future perspectives. Renew. Sustain. Energy Rev. 69, 559–577. doi:10.1016/j.rser.2016.11.187
Keywords: sewage sludge, biosolids, nutrient recycling, PFAS, agricultural land, wastewater
Citation: Saliu TD and Sauvé S (2024) A review of per- and polyfluoroalkyl substances in biosolids: geographical distribution and regulations. Front. Environ. Chem. 5:1383185. doi: 10.3389/fenvc.2024.1383185
Received: 07 February 2024; Accepted: 28 May 2024;
Published: 13 June 2024.
Edited by:
Liting Hao, Beijing University of Civil Engineering and Architecture, ChinaReviewed by:
Jianzhou He, Auburn University, United StatesYongjie Liu, China University of Labor Relations, China
Copyright © 2024 Saliu and Sauvé. This is an open-access article distributed under the terms of the Creative Commons Attribution License (CC BY). The use, distribution or reproduction in other forums is permitted, provided the original author(s) and the copyright owner(s) are credited and that the original publication in this journal is cited, in accordance with accepted academic practice. No use, distribution or reproduction is permitted which does not comply with these terms.
*Correspondence: Sébastien Sauvé, c2ViYXN0aWVuLnNhdXZlQHVtb250cmVhbC5jYQ==