- 1Accelerator Mass Spectrometry and Isotope Research, Helmholtz-Zentrum Dresden-Rossendorf, Dresden, Germany
- 2Department of Nuclear Physics and Accelerator Applications, The Australian National University, Canberra, ACT, Australia
- 3Institute of Nuclear and Particle Physics, Technical University Dresden, Dresden, Germany
- 4Department of Physics and Astronomy, University of Bonn, Bonn, Germany
Long-lived radionuclides in our environment provide important information on natural and anthropogenic processes. Their presence and concentration reflect the balance of production and decay. Geological archives store such information and the nuclides can be chemically extracted from the bulk sample. Accelerator mass spectrometry (AMS) represents a sensitive method to quantify those nuclides at natural levels. Three different terrestrial archives are discussed here as examples for radionuclide extraction using various chemical separation methods for subsequent AMS measurements. We focus on sample preparation for the cosmogenic radionuclides 10Be and 26Al, various anthropogenic actinide isotopes such as U, Pu, and Am as well as the astrophysically interesting nuclides 41Ca, 53Mn, and 60Fe. The processed materials cover samples with masses between a few mg and up to a few hundred kg and protocols are presented for the quantitative extraction of some 10,000 atoms of cosmogenic or interstellar origin per sample and even as low as a few hundred actinide atoms.
1 Radionuclides in the environment analysed using accelerator mass spectrometry
The concentration of rare radionuclides in our environment is an imprint of natural processes or anthropogenic activities. The detection of longer-lived radionuclides, thus, provides valuable information on our environment at large as radionuclides decay over time and their abundance is the result of the interplay of build-up and decay. The half-life of a nuclide defines the relevant time-scale of those processes that can be studied via a specific radionuclide. Longer-lived radionuclides with half-lives of 103 yr
Today, anthropogenic radionuclides are found as a global signal through the intense nuclear weapons testing period between 1952 and 1963. Also, the nuclear activities associated with energy production (such as reactor accidents and release of radioactivity into the environment from reprocessing plants) or in medical applications imprinted an artificial isotopic signature on our environment that can be used for various environmental studies. In fact, the distinctive imprint may even define a new geological epoch, the Anthropocene. The measurement of such radionuclides with the ultrasensitive method of Accelerator Mass Spectrometry (AMS) not only allows for the study of these environmental processes and monitoring anthropogenic activities, but also paves the way for research into fundamental scientific questions.
AMS offers the potential to quantify these radionuclides as isotopic abundances (radionuclides or rare isotopes versus stable isotopes) at their natural levels, with isotopic ratios typically ranging between 10–10 and 10–16. It represents a method to directly count and identify individual rare atoms. As such, it allows the measurement of minute amounts of radionuclides such as 14C, 10Be or 239Pu, via their respective isotopic ratios, e.g., 14C/12C, 10Be/9Be or 239Pu/242Pu (Finkel and Suter, 1993; Synal, 2013; 2022; Kutschera et al., 2023). 12C or 9Be represent the natural elemental content via a stable isotope. In some cases, the content of the reference isotope may be too small (e.g., for 9Be in seawater, see below) or, as in the case of Pu, no stable isotope exists at all. In these cases, the sample may be spiked with a quantitative amount of the reference isotope (e.g., 9Be); or for Pu-AMS e.g., 242Pu is an artificial tracer added to allow for isotope ratio measurements. The respective concentrations of the radionuclides (i.e., the absolute number of nuclides in a sample) can be determined from an independent measurement of the stable element concentrations (e.g., via inductively-coupled plasma mass spectrometry, ICP-MS); or in case of 239Pu simply by using the well-known amount of 242Pu added to the sample.
The key difference of accelerator mass spectrometry compared to conventional mass spectrometry techniques is the addition of a particle accelerator. The use of an accelerator reduces measurement background by orders of magnitude compared to techniques such as ICP-MS or secondary-ion mass spectrometry (SIMS) by providing complete background suppression of molecular isobars. In AMS, an ion beam is produced, and subsequently, the ions are separated and analysed according to their mass, particle energy, and charge state by electric and magnetic components. Rare isotopes are counted with particle detectors one by one, while the beam intensity of stable ions is measured as an electric current with Faraday cups. During the measurement the sample material is consumed slowly at consumption rates of less than or on the order of mg per measurement hour. The abundance sensitivity in AMS is limited by the overall efficiency of the rare isotope detection (this is the fraction of radionuclides in the sample that is eventually counted by the detector) but also by any residual isobaric and isotopic interferences in the detector mimicking a rare radionuclide detector signal.
AMS represents the most sensitive technique for quantifying radionuclides with half-lives 103 yr
2 Geological samples
2.1 Case 1: deep-ocean ferromanganese crusts (10Be, 26Al)
Deep-ocean ferromanganese (Fe/Mn) crusts are slow-growing metallic underwater rocks found in all major oceans on Earth (Hein and Koschinsky, 2014). The growth of the here considered class of Fe/Mn crusts is induced by hydrogenetic precipitation of vernadite and feroxyhyte out of seawater onto a sediment-free rock substrate (Koschinsky and Halbach, 1995; Halbach et al., 2017). Typical growth-rates of Fe/Mn crusts are on the order of mm/Myr. This allows to investigate Earth’s history for interstellar radionuclide influxes (Knie et al., 1999; 2004; Wallner et al., 2015; 2016; 2021; Koll, 2023) on a Myr timescale in addition to deep-ocean sediments (Fitoussi et al., 2008; Ludwig et al., 2016; Wallner et al., 2016; 2020) and Antarctic ice and snow (Koll et al., 2019). Fe/Mn crusts are rich in rare-earth elements as well as in Ni and in particular Co (0.2–2%), leading to their similarly common name Co-rich Fe/Mn crusts and making them an important resource for future industrial mining (Halbach et al., 2017).
The radioactive decay of incorporated 10Be (t1/2 = 1.39 Myr, Korschinek et al., 2010; Chmeleff et al., 2010) is most commonly used to date Fe/Mn crusts and to determine their growth-rate. Cosmogenic 10Be is produced in the upper atmosphere by galactic cosmic rays striking nitrogen and oxygen molecules, breaking the nuclides up and forming the spallation product 10Be (Masarik and Beer, 1999). A steady cosmic ray flux and composition of the atmosphere lead to a relatively constant production of 10Be over geological timescales (Ku et al., 1982; Smith et al., 2019). The freshly produced 10Be is continuously incorporated into Fe/Mn crusts. The decay of 10Be can then be used as a radioactive clock to date the Fe/Mn crust, if the incorporation of Be into the crust stays constant over time. Several studies indicate a good constancy of Be incorporation in several different Fe/Mn crusts over the last 10 Myr (Bourles et al., 1989; Segl et al., 1984; Koll and Lachner, 2023; von Blanckenburg et al., 1996b). Differences in Be uptake in deep-ocean sediments were assigned to varying Ca concentrations in them (Feige, 2014). The Ca content of Fe/Mn crusts is low and constant and does, therefore, not influence the Be uptake (Koll, 2023).
The production rate of 10Be in the upper atmosphere of ≈ 6 × 105 at/cm2/yr (Masarik and Beer, 1999; Nagai et al., 2000; Webber and Higbie, 2003) and the 9Be concentration in sea water of ≈ 6 × 10−6 mg/L (Haynes et al., 2014) lead to isotopic ratios of 10Be/9Be ≈ 10–7 in sea water and, hence, in Fe/Mn crusts. This intrinsic ratio is several orders of magnitude higher than the sensitivity of AMS of 10–16. However, the 9Be concentration in Fe/Mn crusts is only a few ppm (von Blanckenburg et al., 1996a), leading to less than micrograms of 9Be for the final AMS sample. Due to the high intrinsic ratio, either a carrier-free approach can be chosen, where
The chemical extraction of Be and several other elements from Fe/Mn crusts was described in detail by Koll et al. (2022b) and Koll (2023). Here, the chemistry protocol is described with a focus on Be and more recent findings. A schematic summary of the procedure can be found in Figure 1.
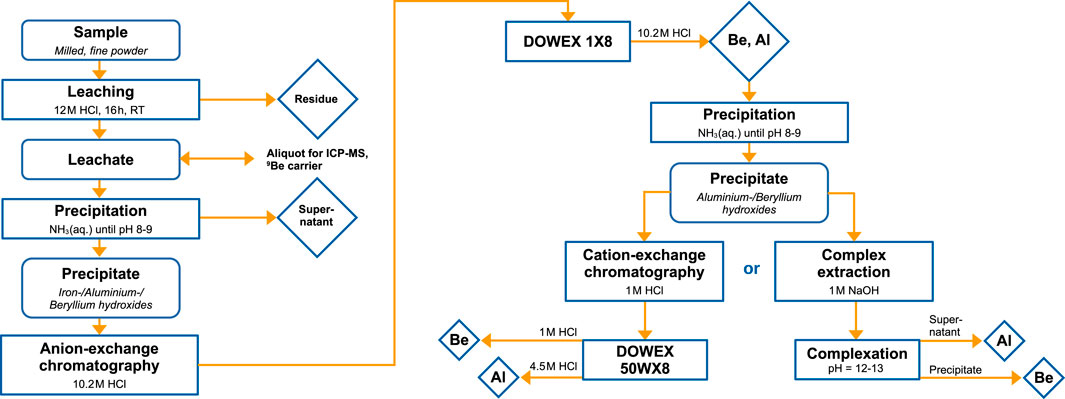
FIGURE 1. Flow chart of chemical work-up procedure for 10Be and 26Al extraction from 10–100 mg Fe/Mn crust. RT: room temperature.
Beryllium is extracted from 10–100 mg of milled Fe/Mn crust powder by first dissolving the powder in 15 mL of 10.2 M HCl and 0.25 mL of 30% H2O2 at room temperature. Seawater beryllium, and therefore, atmospheric 10Be that were incorporated in the leachable part of the Fe/Mn matrix are dissolved. Any unwanted in-situ produced 10Be as well as terrestrial Be in the insoluble mineral fraction of silicates and zircons are discarded. The dissolved Be is precipitated together with Fe, Al, and Ti hydroxides by the addition of NH3(aq.) until pH = 8–9. Both, Mn and Co, can either partially or not precipitate due to its oxidation state ambivalence or the formation of an ammine-complex, respectively. Abundant elements such as Na, Mg or Ca do not form insoluble hydroxides at this pH and stay in the supernatant. The most important isobaric element for 10Be AMS, B, is present as anionic borate in solution and similarly separated. The pre-concentrated and purified precipitate is redissolved in 10.2 M HCl and charged to a 12 mL anion-exchange column (DOWEX 1X8). Iron is strongly retained on the column in contrast to Be, Al and Ti. After the column chromatography, the solution containing Be, Al and Ti is precipitated again by the addition of NH3(aq.). The precipitate can directly be transformed into an AMS target by igniting it at 900°C since the Al and Ti contents are low and do not interfere with the 10Be AMS measurement. In case an isolated Al fraction is required, a cation-exchange column or a complexation at pH = 12–13 (Ochs and Ivy-Ochs, 1997) can be added to separate Al from Be (see Figure 1 and Subsection 2.3.2).
It is advantageous that the final AMS target contains both fractions, Be and Al, because 10Be and 26Al are highly desired AMS radionuclides (see e.g., Nishiizumi et al., 1986; Granger, 2006). The measurement of both radionuclides from the same target would improve the sample throughput significantly. Typically, a good performance of Al targets with respect to ion beam current output is more critical than for Be due to the lower 26Al concentration and worse negative ion formation efficiency in the sputter ion source. Therefore, a combined sample should be optimised for Al AMS. First tests, where the combined sample of BeO and Al2O3 was split into two and prepared as an individual Be-optimised target (mixed 1:4 wt/wt with Nb) and as an Al-optimised target (mixed 1:1 wt/wt with Ag) showed that both targets performed well in their respective radionuclide measurement. The next step is to optimise the sample preparation such that both measurements for 10Be and 26Al perform well from the same AMS target.
The 10Be AMS technique to determine a Fe/Mn crust’s growth rate is well-established and the methodology at the DREsden Accelerator Mass Spectrometry (DREAMS) facility (Akhmadaliev et al., 2013; Rugel et al., 2016; Lachner et al., 2023) will be briefly explained. The prepared BeO target (mixed with Nb, 1:4 wt/wt) is inserted into the negative ion source. After sputtering, 9,10BeO− is selected and injected into the accelerator where electrons are stripped and molecules dissociate. The positively charged 9,10Be ions are then selected after the accelerator and guided to the detector. Interfering 10B is separated from 10Be by differential energy loss in a SiN foil and the subsequent passage of an electrostatic analyser. Macroscopic currents of 9Be are measured in offset Faraday cups. Isotopic ratios of processed crust surface samples with previously added Be carrier are in the range of 10Be/9Be = 10–10–10–11. At the DREAMS facility, dating of ferromanganese crusts beyond 15 Myr is possible with high precision. Recently, a piece of the Fe/Mn crust VA13/2-237KD was 10Be-dated at the DREAMS facility till 18 Myr for the search of supernova-produced 60Fe and r-process 244Pu (Koll et al., 2022b; Koll, 2023).
2.2 Case 2: urban soil (U, Pu, Am)
Atmospheric nuclear weapons test during the 1950s and 60s released huge amounts of actinides (e.g., U, Np, Pu, Am) and fission products (e.g., 90Sr, 99Tc, 129I, 137Cs) into the environment (UNSCEAR, 2010). These nuclides have spread globally and can be found on Earth’s surface in oceans, soils, sediments and plants. Furthermore, major nuclear accidents as happened in Chernobyl (1986) or Fukushima-Daichi (2011) contributed to the anthropogenic radionuclide signal in the environment (NEA/OECD, 2002; Onda et al., 2020). Additionally, local sources from nuclear facilities such as nuclear power plants, reprocessing or fuel production sites could be present. The isotopic signature of actinides in environmental samples gives the unique opportunity to distinguish different sources and is essential for nuclear safeguards and non-proliferation purposes. Thus, several actinide isotopes are of interest due to their different production pathways and hence their ability to account for various sources.
Many studies focused on the isotopic ratio of the plutonium isotopes 240Pu/239Pu, which is only accessible with mass spectrometric techniques due to the similar α energies of both nuclides (Kelley et al., 1999). Although the Pu isotopes with higher mass numbers (i.e., 241Pu, 242Pu and 244Pu) are produced in much lower quantities, their assessment holds potential for a deeper understanding of the source of Pu in the environment (Steier et al., 2013). Especially the shorter-lived 241Pu (t1/2 = 14.3 years) and its daughter 241Am may be used for dating the last chemical separation of both elements or the date of irradiation (Steier et al., 2013; Corcho Alvarado et al., 2022. In recent years, the rare uranium isotopes 233U and 236U and their atomic ratio have gained much attention, especially looking in ocean water and sediments worldwide (Hain et al., 2020; Lin et al., 2021; Qiao et al., 2022).
A compilation of expected isotope ratios for global nuclear fallout in comparison to measurements from the Chernobyl debris can be found in Table 1. Within this work we present our chemical work-up protocol for the determination of actinides (i.e., U, Pu, Am) from different environmental samples like soil, sediments or plant material. The required sample size strongly depends on the isotopes to be investigated. Several studies reported the concentration of 239Pu of anthropogenic origin for top soil layers in the order of 50–500 fg/g (Harley, 1980; Kelley et al., 1999; Muramatsu et al., 2000; Tighe et al., 2021). This corresponds to 108–109 atoms 239Pu/g soil (1 fg Pu
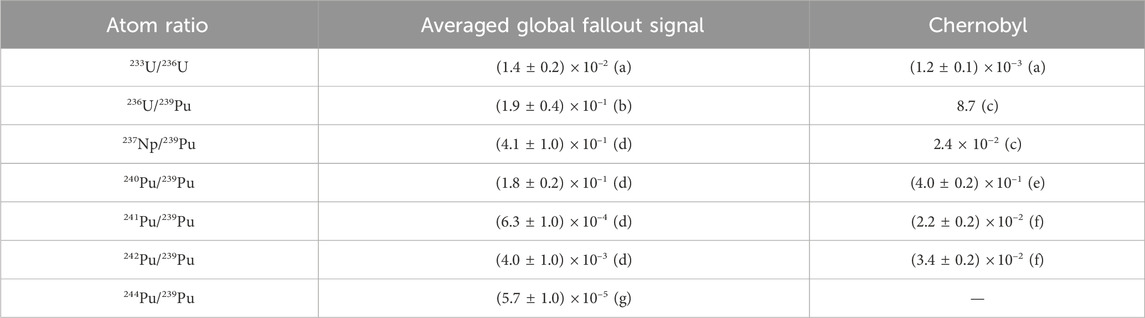
TABLE 1. Expected atomic ratios of actinide isotopes relative to 239Pu for the global averaged nuclear fallout signal and Chernobyl debris. 241Pu/239Pu isotopic ratio decay corrected to 01.01.2024. Data taken from (a) Hain et al. (2020), (b) Ketterer et al. (2013), (c) UNSCEAR (2000), (d) Kelley et al. (1999), (e) Muramatsu et al. (2000), (f) Ketterer et al. (2004) and (g) Steier et al. (2013).
As already introduced in Section 1, AMS is an ultra-trace analytical method measuring isotopic ratios between rare and abundant isotopes with high sensitivity. In the case of actinides there are no abundant isotopes present in nature despite the long-lived 232Th and 235,238U and their daughter nuclides. Thus, for determining the content of the rare uranium isotopes 233U and 236U, the concentration of the primordial uranium can be analysed with less sensitive analytical methods like ICP-MS. However, the measurement of transuranium elements requires the addition of a (preferably mono-isotopic) spike of known quantity as a reference. For plutonium determination, different isotopes can be added as tracers (i.e., 236Pu, 242Pu or 244Pu). Isotopic purity, availability and interference with other nuclides are the major factors for selecting an appropriate isotopic spike. For many actinides the limited number of long-lived and readily accessible isotopes prevents the use of an isotopic spike. Instead, neighbouring elements may be used as non-isotopic spikes (e.g., 243Am as spike for 247Cm or 242Pu for 237Np). The prerequisite for using non-isotopic spikes is a similar chemical behavior during sample preparation and a well-characterised standard material to correct the AMS measurement for differences in their ionisation efficiency and transmission through the accelerator.
The general work-up scheme for different environmental samples is shown in Figure 2. Commonly, the samples are first dried in a drying cabinet at 95°C to constant mass content. Subsequently, larger grains are removed by sieving (2 mm). Afterwards, the samples are weighed and ashed in a muffle furnace at 550°C for 5 h in order to destroy all organic materials present and determine the loss-on-ignition. After ashing the residues are leached in 10 mL 8 M HNO3 per gram sample for 2 h under reflux conditions. Although this acid leaching process does not dissolve the whole sample (silicates, zircons and refractory oxides remain) it has been shown that the majority of the targeted anthropogenic actinides are accessible (Krey and Bogen, 1987).
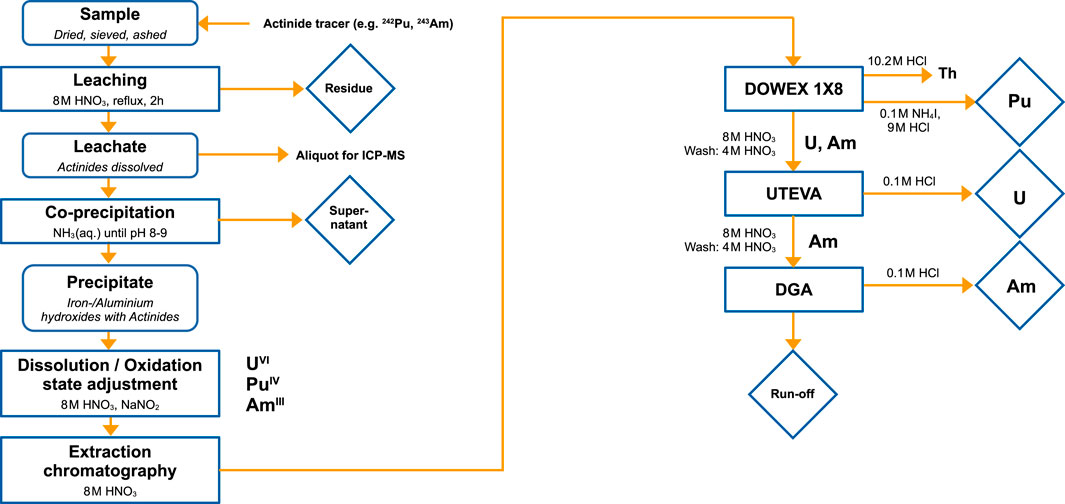
FIGURE 2. Flow chart of chemical work-up procedure for U, Pu and Am extraction from 1–5 g urban soil.
The separation of the actinides from the bulk sample is done by means of chemical purification steps like co-precipitation and column chromatography. For environmental samples we perform a co-precipitation of the actinides with Fe and Al hydroxides by raising the pH of the acidified solutions from leaching to pH = 8–9 with diluted NH3(aq.). The alkali and earth-alkali metals, with the valuable exception of Be, do not form hydroxide precipitates at these pH-values and stay in the supernatant. High concentrations of iron(III) can subsequently be removed by dissolving the precipitate in 7 M hydrochloric acid and liquid-liquid extraction with diisopropyl ether. Another major constituent of soil, Al, can be decreased by making use of it’s amphoteric character, forming soluble hydroxide complexes [Al(OH)4]− at pH > 12. Generally, less matrix material facilitates a smooth column chromatography setup and avoids column blockage.
Prior to separation of the actinides from the sample using column chromatography, their oxidation state has to be adjusted. Under the given oxidising conditions in diluted nitric acid medium, uranium exclusively exists as U(VI) and americium as Am(III). Hence, only the oxidation state of Pu needs to be adjusted to Pu(IV) in order to separate the most relevant actinides according to their oxidation state and their connected chemical behavior. Addition of solid NaNO2 and boiling for 30 min is sufficient for this purpose (Koll et al., 2022a).
In general, Pu is extracted from the remaining matrix as [PuIV(NO3)6]2− complex on anion exchange resin DOWEX 1X8, U as phosphonate complex on UTEVA resin and finally Am as TODGA complex on DGA resin. Hence, a stacked three column approach is used to sequentially extract the actinides of interest. After sample loading, the columns are washed with 8 M and 4 M HNO3 to remove matrix elements (e.g., Al, Fe, Mn). Thereafter the columns are separated and treated differently to finally obtain pure fractions of the actinides. The anion exchange column is subsequently washed with 10.2 M HCl to remove the coextracted Th. Finally, Pu is eluted by reducing it to its trivalent oxidation state with 0.1 M NH4I - 9 M HCl. Evaporation of the solution and fuming with HNO3 and HCl destroys the remaining ammonium iodide. U and Am can be eluted from UTEVA resp. DGA columns by 0.1 M HCl.
The final AMS target preparation is similar for all separated actinides which are subsequently either co-precipitated with iron as iron(III)-hydroxides or dissolved in HNO3 acidic iron solution and dried. The dried residues are transferred to quartz crucibles which are calcined in a muffle furnace at 600°C for 2 h to form Fe2O3 with embedded actinides. The resulting iron oxide powder is mixed with metal powders (e.g., Ag, Nb) and pressed into cathodes for sputtering in negative ion sources. Nowadays, actinides are more efficiently measured at compact AMS systems Vockenhuber et al., 2013; Hotchkis et al., 2019; Steier et al., 2019. Here, we exemplify the AMS setup for the two AMS facilities Vega (ANSTO) and VERA (Univ. of Vienna). For plutonium measurements at ANSTO (Wilcken et al., 2015; Hotchkis et al., 2019), mixtures of Fe2O3:Nb (1:1 wt/wt) are pressed into aluminium target holders. Negatively charged PuO− ions are extracted and accelerated by setting the terminal voltage to 0.86 MV. On the high-energy side Pu3+ ions are counted in a gas-ionization chamber. Fast isotope cycling is applied to the low- and high-energy magnets to inject up to 8 masses of different isotopes quasi-simultaneously (Hotchkis et al., 2019). For actinide (An) measurements at the VERA facility (Steier et al., 2019; Hain et al., 2020) the iron oxide material is mixed with silver in 1:1 weight ratio. Again, AnO− ions are extracted and stripped to An3+ ions in the accelerator using 1.65 MV terminal voltage.
2.3 Case 3: Antarctic ice (10Be, 26Al, 41Ca, 53Mn and 60Fe)
Stars freshly synthesise chemical elements in their interior by nuclear reactions. The life cycle of massive stars concludes with a stellar explosion, a supernova, that is ejecting most of the stars material into space, including freshly produced radionuclides. While the solar system traverses the interstellar medium, it captures interstellar dust containing live radionuclides from former supernovae (Ellis et al., 1996; Athanassiadou and Fields, 2011). These dust particles can accumulate on Earth over millions of years, leaving their isotopic fingerprints in terrestrial archives to be uncovered.
Latest endeavours focus on establishing a new geological archive for nuclear astrophysics—the ice of Antarctica. Antarctic ice serves as a unique geological reservoir for the study of extraterrestrial material due to its isolated location and the climatic conditions that preserve materials chronologically over millennia. In this project, we analyse radionuclide concentrations in continuous-flow analysis (CFA) water, extracted from an Antarctic ice core. The ice core is sourced from a drilling site near the Kohnen Station in Dronning Maud Land, Antarctica.
Our objective is to investigate an ongoing interstellar 60Fe (t1/2 = 2.6 Myr, Rugel et al., 2009; Wallner et al., 2015) influx by covering a time period between 50,000 and 80,000 years ago. Earlier studies showed 60Fe activity about 2–3 Myr ago (Knie et al., 2004; Ludwig et al., 2016; Wallner et al., 2016; 2021; Koll, 2023), as well as an older influx 7–8 Myr ago (Wallner et al., 2016; 2021; Koll, 2023), both of which are attributed to interstellar dust containing traces of supernova-produced 60Fe. Furthermore, a recent interstellar 60Fe influx was discovered in Antarctic surface snow (Koll et al., 2019) and independently in deep-sea sediments covering the past 35 kyr (Wallner et al., 2020). A study of Antarctic ice for 60Fe will, therefore, yield time-resolved data between the previously investigated timescales (Koll et al., 2020). In addition to 60Fe, the atmospheric radionuclides 10Be and 26Al are used to characterise the Antarctic ice sample further, whereas the interplanetary isotopes 41Ca and 53Mn are utilised to distinctively differentiate interstellar 60Fe from cosmic-ray produced 60Fe (Koll et al., 2022a).
The rarity of the influx and deposition of interstellar radionuclides onto Earth constrains the usability of potential environmental archives. In order to clearly detect 60Fe, a minimum of 25,000 atoms of 60Fe must be present in a sample in order to obtain 10 detector events at the Heavy-Ion Accelerator Facility (HIAF) at the Australian National University (Wallner et al., 2023). Furthermore, the atomic ratio 60Fe/Fe must exceed 2 × 10−16, which is 10 times the lowest blank level. Addressing the first condition, we consider the known recent 60Fe deposition rate in Antarctica of 1 at/cm2/yr (Koll et al., 2019) and the pre-industrial accumulation rate of 66 mm w.e. yr−1 (Oerter et al., 2000; Birnbaum et al., 2006; Medley et al., 2018). A minimum of 165 kg of Antarctic ice is necessary to contain the required 25,000 60Fe atoms. A typical terrestrial Fe flux of 1 mg/m2/yr (Conway et al., 2015), yields 2.5 mg of Fe in 165 kg of Antarctic ice. This results in a ratio of 60Fe/Fe = 9 × 10−16, thereby fulfilling the second condition. A higher accumulation rate of 110–250 mm w.e. yr−1 (Karlsson et al., 2016) and an up to 20 times higher iron content (Xiao et al., 2020) in Greenland ice would lead to a reduction of the 60Fe/Fe ratio beyond detectability, primarily driven by the higher stable iron content.
The relatively low accumulation rates in Dronning Maud Land, however, still require high sample volumes to reach the sensitivity required to detect a few single atoms from an interstellar dust influx. Additionally, a chemically pure sample is essential to prevent a dilution in the Fe concentration. The chemical procedure to extract the aforementioned radionuclides from such large ice samples is based on the established procedure for a deep-ocean ferromanganese crust (Koll et al., 2022b) and meteorites (Merchel and Herpers, 1999). It is an update to the previously employed procedure for Antarctic snow (Koll, 2018; Koll et al., 2019). The chemical protocol is depicted in the flowchart in Figure 3.
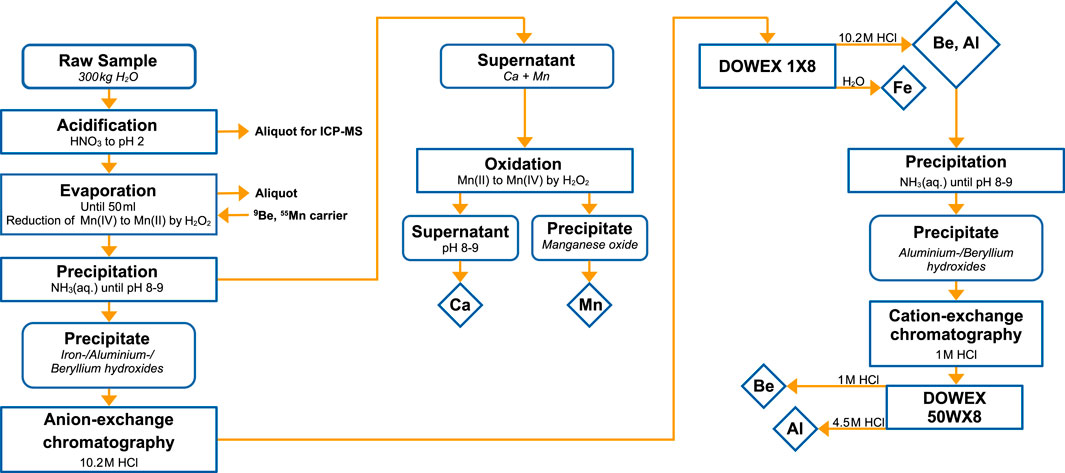
FIGURE 3. Flow chart of chemical work-up procedure for 10Be, 26Al, 41Ca, 53Mn and 60Fe extraction from 300 kg Antarctic ice.
A total of 300 kg of CFA water is acidified to pH = 2 by adding HNO3 to prevent microbial contamination and ensure sample stability. An aliquot of the total volume is analysed by ICP-MS for already dissolved elements. The volume is then significantly reduced through evaporation over three to 4 weeks in two insulated and covered 10 L glass beakers under temperature-controlled conditions at 80–90 °C to prevent boiling. A 10 L blank sample from purified (18 MΩ) water is placed next to the beakers to monitor the introduction of contaminants. The progressing evaporation gradually increases the acid concentration of the sample, prompting repeated checks of the element concentrations by ICP-MS until the sample reaches 50 mL. The increasing acid concentration continuously leaches terrestrial elements from solid particles in the sample. If necessary, a filtration step could be included to restrict further leaching at the expense of potential radionuclide losses. Here, the low stable element concentrations did not require filtration even in concentrated HNO3. The addition of H2O2 reduces any Mn(IV) to soluble Mn(II) in HNO3. After evaporation, a final aliquot from the remaining 50 mL sample is taken for stable element determination before carrier solutions are added to the sample and the blank. The low natural concentrations of Be and Mn in Antarctic ice (Koll, 2018) require the addition of 2 mg Be and 3 mg Mn, whereas natural Al, Ca and Fe concentrations are high enough for carrier-free chemistry of the Antarctic ice sample. The typically added Be carrier mass in AMS is in the range of 200–500 μg, but here, this large natural sample with a considerable atmospheric 10Be concentration requires further dilution to reduce the expected 10Be/9Be ratio.
2.3.1 Be, Al, Fe
The elements Be, Al and Fe are separated from Ca and Mn by hydroxide precipitation at pH = 8 – 9 through the addition of NH3(aq.). This also separates the isobaric interferences 10B and 60Ni from the respective radionuclide of interest. The precipitate containing Be, Al and Fe is redissolved in 10.2 M HCl and loaded onto a 12 mL anion-exchange column (DOWEX 1X8), where Be and Al are passed, but Fe is retained on the column. Iron is eluted by the addition of 27.5 mL H2O. The Be/Al fraction and the Fe fraction are precipitated again by the addition of NH3(aq.) at pH = 8–9. The iron hydroxide is then dried and ignited at 600°C to form Fe2O3.
2.3.2 Be, Al
The Be/Al hydroxide is redissolved in 1 M HCl and charged to a 12 mL cation-exchange column (DOWEX 50WX8) to separate Be from retained Al. Residual B is separated from Be by addition of 35 mL 1 M HCl, followed by 110 mL 1 M HCl for Be elution. The addition of 47.5 mL 4.5 M HCl finally elutes Al. This column separation is repeated due to the high concentration of Al in this sample to obtain pure Be and Al fractions. A complexation approach at pH = 12–13 (Ochs and Ivy-Ochs, 1997) is less preferable due to the large quantities of natural Al in this particular sample. The separated Be and Al fraction are precipitated by the addition of NH3(aq.) until pH = 8–9. Both, Be and Al hydroxides, are dried and then ignited at 900°C for 2 h to form Al2O3 and BeO, respectively.
2.3.3 Mn
To isolate Mn from the supernatant that contains the dissolved Ca and Mn, H2O2 is added to oxidise Mn(II) to MnO2, which precipitates out of solution. The AMS of 53Mn requires a further purification from its isobar 53Cr by a KClO3 precipitation in small volumes. The redissolved Mn fraction is placed on a hot plate in 10 mL concentrated HNO3, to which potassium chlorate is added sequentially until the precipitation of MnO2 as brown precipitate is observed. This purification step is repeated twice to ensure the lowest Cr levels necessary for 53Mn AMS measurements. The purified MnO2 is dried at 90°C in a drying cabinet for 72 h.
2.3.4 Ca
The Ca fraction is further processed in three consecutive precipitation steps. First, Ca is separated from Mg by precipitation as hydroxide with 1 M NaOH at pH = 10–11. (NH4)2C2O4 selectively precipitates Ca as CaC2O4. Taking advantage of the fact that ammonium oxalate is more soluble at higher temperatures, the Ca sample is precipitated in warm conditions. This is followed by a CaF2 precipitation in 3 mL 4 M HNO3 and addition of 1 mL 48% HF. Lastly, CaF2 is dried at 70°C overnight.
2.3.5 AMS targets
The final samples are prepared as AMS targets by mixing them with metal powders: BeO (1:4 wt/wt with Nb), Al2O3 (1:1 wt/wt with Ag), CaF2 (1:4 wt/wt with Ag), MnO2 (1:1 wt/wt with Cr-reduced Ag powder) and Fe2O3 (1:1 wt/wt with Ag) and then pressed into Cu cathodes. The radionuclides 10Be, 26Al and 41Ca are measured at the DREAMS facility (Akhmadaliev et al., 2013; Lachner et al., 2023; Vivo-Vilches et al., 2023), while 53Mn and 60Fe are measured at HIAF (Wallner et al., 2023).
3 Summary
Accelerator mass spectrometry has proven to be a versatile tool for the detection of rare radionuclides. It offers a dynamical range in abundance ratios over several orders of magnitude and detection sensitivities are approaching a few hundred atoms per sample for some nuclides. However, even at these ultra-low detection limits, the measurement of certain radionuclides, especially of extraterrestrial origin, still require large sample sizes. Using production rates, deposition and incorporation efficiencies of some radionuclides, we calculated above the relevant sample sizes for three different cases to enable their detection with AMS. Proper chemical treatment strategies are indispensable to extract and separate radionuclides out of a large sample matrix. The case studies presented show the chemical work-up protocol for determining cosmogenic 10Be and 26Al from 10–100 mg Fe/Mn crust, anthropogenic U, Pu and Am isotopes from 1–5 g of urban soil and extraterrestrial 41Ca, 53Mn and 60Fe from 300 kg of Antarctic ice. In all chemical treatment strategies hydroxide precipitation out of acidified solutions is the first step to separate high-valent cations which are forming sparingly soluble hydroxide precipitates (i.e., Al, Fe, Ti, Be and actinides) from mono- and divalent cations (i.e., Na, K, Mg, Ca, Mn, Ni) which stay in the supernatant at pH = 8–9. Subsequently, different complex formation tendencies of metal cations are used to separate them using anion- and cation-exchange columns (DOWEX 1X8 and DOWEX 50WX8) and extraction chromatography with organic ligands impregnated onto an inert support (UTEVA and DGA resin from Triskem for actinide separation). Finally, AMS targets are prepared by embedding the radionuclides into a stable inorganic oxide or fluoride matrix consisting of the stable reference nuclide or, in the case of actinides, into iron(III) oxide. These chemical treatment strategies are not limited to the presented sample types and can be easily adapted and scaled to new projects. In addition to such optimised chemical separation strategies, also the recent technological progress made in rare isotope detection, as, e.g., implemented at the new AMS facility HAMSTER (Helmholtz Accelerator Mass Spectrometer Tracing Environmental Radionuclides) at HZDR, will further improve measurement sensitivities. Consequently, AMS represents an important tool to address open questions in climate research, Earth science or in astrophysics, but also for studying the anthropogenic environmental impact.
Data availability statement
The original contributions presented in the study are included in the article/Supplementary material, further inquiries can be directed to the corresponding authors.
Author contributions
SF: Conceptualization, Methodology, Writing–original draft, Writing–review and editing, Funding acquisition. DK: Conceptualization, Methodology, Writing–original draft, Writing–review and editing, Funding acquisition. AR: Writing–original draft, Writing–review and editing, Methodology. AW: Funding acquisition, Methodology, Project administration, Writing–original draft, Writing–review and editing.
Funding
The author(s) declare that financial support was received for the research, authorship, and/or publication of this article. DK was supported by an AINSE Ltd. Postgraduate Research Award (PGRA). The AMS measurements were funded through the RADIATE project from the EU Research and Innovation program HORIZON 2020, project numbers 21002421-ST, 20002142-ST, 22003021-ST and 22003093-ST, the Ion Beam Center of HZDR, project numbers 22002913-EF, 22003072-EF and 23003438-ST and the National Collaborative Research Infrastructure Strategy (NCRIS) of the Australian Government.
Acknowledgments
The authors want to express their gratitude to Florian Adolphi and Maria Hoerhold for providing the Antarctic ice sample and valuable input. Karin Hain and Peter Steier from VERA are gratefully acknowledged for performing actinide AMS measurements.
Conflict of interest
The authors declare that the research was conducted in the absence of any commercial or financial relationships that could be construed as a potential conflict of interest.
Publisher’s note
All claims expressed in this article are solely those of the authors and do not necessarily represent those of their affiliated organizations, or those of the publisher, the editors and the reviewers. Any product that may be evaluated in this article, or claim that may be made by its manufacturer, is not guaranteed or endorsed by the publisher.
References
Akhmadaliev, S., Heller, R., Hanf, D., Rugel, G., and Merchel, S. (2013). The new 6MV AMS-facility DREAMS at Dresden. Nucl. Instrum. Methods Phys. Res. Sect. B Beam Interact. Mater. Atoms 294, 5–10. doi:10.1016/j.nimb.2012.01.053
Athanassiadou, T., and Fields, B. (2011). Penetration of nearby supernova dust in the inner solar system. New Astron. 16, 229–241. doi:10.1016/j.newast.2010.09.007
Birnbaum, G., Brauner, R., and Ries, H. (2006). Synoptic situations causing high precipitation rates on the Antarctic plateau: observations from Kohnen Station, Dronning Maud Land. Antarct. Sci. 18, 279–288. doi:10.1017/S0954102006000320
Bourles, D., Raisbeck, G., and Yiou, F. (1989). 10Be and 9Be in marine sediments and their potential for dating. Geochimica Cosmochimica Acta 53, 443–452. doi:10.1016/0016-7037(89)90395-5
Bouvier, A., and Wadhwa, M. (2010). The age of the solar system redefined by the oldest Pb–Pb age of a meteoritic inclusion. Nat. Geosci. 3, 637–641. doi:10.1038/ngeo941
Chmeleff, J., von Blanckenburg, F., Kossert, K., and Jakob, D. (2010). Determination of the 10Be half-life by multicollector ICP-MS and liquid scintillation counting. Nucl. Instrum. Methods Phys. Res. Sect. B Beam Interact. Mater. Atoms 268, 192–199. doi:10.1016/j.nimb.2009.09.012
Conway, T. M., Wolff, E. W., Röthlisberger, R., Mulvaney, R., and Elderfield, H. (2015). Constraints on soluble aerosol iron flux to the southern ocean at the last glacial maximum. Nat. Commun. 6, 7850. doi:10.1038/ncomms8850
Corcho Alvarado, J. A., Röllin, S., Sahli, H., and McGinnity, P. (2022). Isotopic signatures of plutonium and uranium at Bikar Atoll, northern Marshall Islands. J. Environ. Radioact. 242, 106795. doi:10.1016/j.jenvrad.2021.106795
Ellis, J., Fields, B. D., and Schramm, D. N. (1996). Geological isotope anomalies as signatures of nearby supernovae. Astrophysical J. 470, 1227. doi:10.1086/177945
Feige, J. (2014). Supernova-produced radionuclides in deep-sea sediments measured with AMS. Universitaet Wien: Ph.D. thesis.
Fifield, L. K., Froehlich, M., Koll, D., Pavetich, S., Slavkovská, Z., Tims, S., et al. (2022). Backgrounds and blanks in iodine-129 measurements at the Australian National University. Nucl. Instrum. Methods Phys. Res. Sect. B Beam Interact. Mater. Atoms 530, 8–12. doi:10.1016/j.nimb.2022.08.014
Finkel, R., and Suter, M. (1993). “AMS in the earth sciences: technique and applications,” in Advances in analytical geochemistry (Stamford, CT: Jai Press).
Fitoussi, C., Raisbeck, G. M., Knie, K., Korschinek, G., Faestermann, T., Goriely, S., et al. (2008). Search for supernova-produced 60Fe in a marine sediment. Phys. Rev. Lett. 101, 121101. doi:10.1103/physrevlett.101.121101
Granger, D. E. (2006). “A review of burial dating methods using 26Al and 10Be,” in In situ-produced cosmogenic nuclides and quantification of geological processes (Boulder, CO: Geological Society of America). doi:10.1130/2006.2415(01)
Hain, K., Steier, P., Froehlich, M. B., Golser, R., Hou, X., Lachner, J., et al. (2020). 233U/236U signature allows to distinguish environmental emissions of civil nuclear industry from weapons fallout. Nat. Commun. 11, 1275. doi:10.1038/s41467-020-15008-2
Halbach, P. E., Jahn, A., and Cherkashov, G. (2017). Marine Co-rich ferromanganese crust deposits: description and formation, occurrences and distribution, estimated world-wide resources. Springer International Publishing, 65–141. doi:10.1007/978-3-319-52557-0_3
Harley, J. H. (1980). Plutonium in the environment—a review. J. Radiat. Res. 21, 83–104. doi:10.1269/jrr.21.83
Haynes, W. M., Lide, D. R., and Bruno, T. J. (2014). CRC handbook of chemistry and Physics (Boca Raton, FL: CRC Press), 95. doi:10.1201/b17118
Hein, J. R., and Koschinsky, A. (2014). “Deep-ocean ferromanganese crusts and nodules,” in Treatise on geochemistry (Elsevier), 273–291. doi:10.1016/B978-0-08-095975-7.01111-6
Horiuchi, K., and Matsuzaki, H. (2015). Exploration of 10Be analysis using 10 μg of Be carrier. Nucl. Instrum. Methods Phys. Res. Sect. B Beam Interact. Mater. Atoms 361, 423–430. doi:10.1016/j.nimb.2015.07.123
Hotchkis, M. A. C., Child, D., Froehlich, M., Wallner, A., Wilcken, K., and Williams, M. (2019). Actinides AMS on the VEGA accelerator. Nucl. Instrum. Methods Phys. Res. Sect. B Beam Interact. Mater. Atoms 438, 70–76. doi:10.1016/j.nimb.2018.07.029
Karlsson, N. B., Eisen, O., Dahl-Jensen, D., Freitag, J., Kipfstuhl, S., Lewis, C., et al. (2016). Accumulation rates during 1311–2011 CE in North-Central Greenland derived from air-borne radar data. Front. Earth Sci. 4. doi:10.3389/feart.2016.00097
Kelley, J. M., Bond, L. A., and Beasley, T. M. (1999). Global distribution of Pu isotopes and 237Np. Sci. Total Environ. 237-238, 483–500. doi:10.1016/S0048-9697(99)00160-6
Ketterer, M. E., Groves, A. D., Strick, B. J., Asplund, C. S., and Jones, V. J. (2013). Deposition of 236U from atmospheric nuclear testing in Washington state (USA) and the Pechora region (Russian Arctic). J. Environ. Radioact. 118, 143–149. doi:10.1016/j.jenvrad.2012.10.007
Ketterer, M. E., Hafer, K. M., and Mietelski, J. W. (2004). Resolving Chernobyl vs. global fallout contributions in soils from Poland using plutonium atom ratios measured by inductively coupled plasma mass spectrometry J. Environ. Radioact. 73, 183–201. doi:10.1016/j.jenvrad.2003.09.001
Knie, K., Korschinek, G., Faestermann, T., Dorfi, E. A., Rugel, G., and Wallner, A. (2004). 60Fe anomaly in a deep-sea manganese crust and implications for a nearby supernova source. Phys. Rev. Lett. 93, 171103. doi:10.1103/PhysRevLett.93.171103
Knie, K., Korschinek, G., Faestermann, T., Wallner, C., Scholten, J., and Hillebrandt, W. (1999). Indication for supernova produced 60Fe activity on Earth. Phys. Rev. Lett. 83, 18–21. doi:10.1103/physrevlett.83.18
Koll, D. (2018). Search for recent 60Fe deposition in Antarctica with AMS. Technical University of Munich.
Koll, D. (2023). A 10-million year time profile of interstellar influx to Earth mapped through supernova60Fe and r-process244Pu. The Australian National University and Technical University Dresden. doi:10.25911/5NJB-YC98
Koll, D., Faestermann, T., Korschinek, G., Leya, I., Merchel, S., and Wallner, A. (2022a). The dyadic radionuclide system 60Fe/53Mn to distinguish interstellar from interplanetary 60Fe. EPJ Web Conf. 260, 11022. doi:10.1051/epjconf/202226011022
Koll, D., Faestermann, T., Korschinek, G., and Wallner, A. (2020). Origin of recent interstellar 60Fe on Earth. EPJ Web Conf. 232, 02001. doi:10.1051/epjconf/202023202001
Koll, D., Korschinek, G., Faestermann, T., Gómez-Guzmán, J. M., Kipfstuhl, S., Merchel, S., et al. (2019). Interstellar 60Fe in Antarctica. Phys. Rev. Lett. 123, 072701. doi:10.1103/PhysRevLett.123.072701
Koll, D., and Lachner, J. (2023). Re-evaluation of cosmogenic 10Be dating of the ferromanganese crust VA13/2 archiving interstellar radionuclides on Earth. Res. Notes AAS 7, 34. doi:10.3847/2515-5172/acbb75
Koll, D., Wallner, A., Battisson, S., Fichter, S., Fifield, K., Froehlich, M., et al. (2022b). Element separation chemistry and cosmogenic 10Be-dating of a ferromanganese crust. Nucl. Instrum. Methods Phys. Res. Sect. B Beam Interact. Mater. Atoms 530, 53–58. doi:10.1016/j.nimb.2022.08.017
Korschinek, G., Bergmaier, A., Faestermann, T., Gerstmann, U., Knie, K., Rugel, G., et al. (2010). A new value for the half-life of 10Be by heavy-ion elastic recoil detection and liquid scintillation counting. Nucl. Instrum. Methods Phys. Res. Sect. B Beam Interact. Mater. Atoms 268, 187–191. doi:10.1016/j.nimb.2009.09.020
Koschinsky, A., and Halbach, P. E. (1995). Sequential leaching of marine ferromanganese precipitates: genetic implications. Geochimica Cosmochimica Acta 59, 5113–5132. doi:10.1016/0016-7037(95)00358-4
Krey, P. W., and Bogen, D. C. (1987). Determination of acid leachable and total plutonium in large soil samples. J. Radioanalytical Nucl. Chem. 115, 335–355. doi:10.1007/BF02037448
Ku, T., Kusakabe, M., Nelson, D., Southern, J., Korteling, R., Vogel, J., et al. (1982). Constancy of oceanic deposition of 10Be as recorded in manganese crusts. Nature 299, 240–242. doi:10.1038/299240a0
Kutschera, W., Jull, A., Paul, M., and Wallner, A. (2023). Atom counting with accelerator mass spectrometry. Rev. Mod. Phys. 95, 035006. doi:10.1103/revmodphys.95.035006
Lachner, J., Ploner, M., Steier, P., Sakaguchi, A., and Usui, A. (2020). Accumulation of ferromanganese crusts derived from carrier-free 10Be/9Be. Nucl. Instrum. Methods Phys. Res. Sect. B Beam Interact. Mater. Atoms 467, 146–151. doi:10.1016/j.nimb.2019.11.047
Lachner, J., Rugel, G., Vivo Vilches, C., Koll, D., Stübner, K., Winkler, S., et al. (2023). Optimization of 10Be measurements at the 6MV AMS facility DREAMS. Nucl. Instrum. Methods Phys. Res. Sect. B Beam Interact. Mater. Atoms 535, 29–33. doi:10.1016/j.nimb.2022.11.008
Lin, M., Qiao, J., Hou, X., Dellwig, O., Steier, P., Hain, K., et al. (2021). 70-Year anthropogenic uranium imprints of nuclear activities in Baltic Sea sediments. Environ. Sci. Technol. 55, 8918–8927. doi:10.1021/acs.est.1c02136
Ludwig, P., Bishop, S., Egli, R., Chernenko, V., Deneva, B., Faestermann, T., et al. (2016). Time-resolved 2-million-year-old supernova activity discovered in Earth’s microfossil record. Proc. Natl. Acad. Sci. 113, 9232–9237. doi:10.1073/pnas.1601040113
Maden, C., Döbeli, M., Kubik, P., Frank, M., and Suter, M. (2004). Measurement of carrier-free 10Be samples with AMS: the method and its potential. Nucl. Instrum. Methods Phys. Res. Sect. B Beam Interact. Mater. Atoms 223, 247–252. doi:10.1016/j.nimb.2004.04.050
Masarik, J., and Beer, J. (1999). Simulation of particle fluxes and cosmogenic nuclide production in the Earth’s atmosphere. J. Geophys. Res. Atmos. 104, 12099–12111. doi:10.1029/1998JD200091
Medley, B., McConnell, J. R., Neumann, T. A., Reijmer, C. H., Chellman, N., Sigl, M., et al. (2018). Temperature and snowfall in western Queen Maud Land increasing faster than climate model projections. Geophys. Res. Lett. 45, 1472–1480. doi:10.1002/2017GL075992
Merchel, S., Braucher, R., Lachner, J., and Rugel, G. (2021). Which is the best 9Be carrier for 10Be/9Be accelerator mass spectrometry? MethodsX 8, 101486. doi:10.1016/j.mex.2021.101486
Merchel, S., and Herpers, U. (1999). An update on radiochemical separation techniques for the determination of long-lived radionuclides via accelerator mass spectrometry. Radiochim. Acta 84, 215–220. doi:10.1524/ract.1999.84.4.215
Muramatsu, Y., Rühm, W., Yoshida, S., Tagami, K., Uchida, S., and Wirth, E. (2000). Concentrations of 239Pu and 240Pu and their isotopic ratios determined by ICP-MS in soils collected from the Chernobyl 30-km zone. Environ. Sci. Technol. 34, 2913–2917. doi:10.1021/es0008968
Nagai, H., Tada, W., and Kobayashi, T. (2000). Production rates of 7Be and 10Be in the atmosphere. Nucl. Instrum. Methods Phys. Res. Sect. B Beam Interact. Mater. Atoms 172, 796–801. doi:10.1016/S0168-583X(00)00124-5
Nishiizumi, K., Lal, D., Klein, J., Middleton, R., and Arnold, J. (1986). Production of 10Be and 26Al by cosmic rays in terrestrial quartz in situ and implications for erosion rates. Nature 319, 134–136. doi:10.1038/319134a0
Ochs, M., and Ivy-Ochs, S. (1997). The chemical behavior of Be, Al, Fe, Ca and Mg during AMS target preparation from terrestrial silicates modeled with chemical speciation calculations. Nucl. Instrum. Methods Phys. Res. Sect. B Beam Interact. Mater. Atoms 123, 235–240. doi:10.1016/s0168-583x(96)00680-5
Oerter, H., Wilhelms, F., Jung-Rothenhäusler, F., Göktas, F., Miller, H., Graf, W., et al. (2000). Accumulation rates in Dronning Maud Land, Antarctica, as revealed by dielectric-profiling measurements of shallow firn cores. Ann. Glaciol. 30, 27–34. doi:10.3189/172756400781820705
Onda, Y., Taniguchi, K., Yoshimura, K., Kato, H., Takahashi, J., Wakiyama, Y., et al. (2020). Radionuclides from the Fukushima Daiichi nuclear power plant in terrestrial systems. Nat. Rev. Earth Environ. 1, 644–660. doi:10.1038/s43017-020-0099-x
Qiao, J., Ransby, D., and Steier, P. (2022). Deciphering anthropogenic uranium sources in the equatorial northwest Pacific margin. Sci. Total Environ. 806, 150482. doi:10.1016/j.scitotenv.2021.150482
Rugel, G., Faestermann, T., Knie, K., Korschinek, G., Poutivtsev, M., Schumann, D., et al. (2009). New measurement of the 60Fe half-life. Phys. Rev. Lett. 103, 072502. doi:10.1103/PhysRevLett.103.072502
Rugel, G., Pavetich, S., Akhmadaliev, S., Enamorado Baez, S. M., Scharf, A., Ziegenrücker, R., et al. (2016). The first four years of the AMS-facility DREAMS: status and developments for more accurate radionuclide data. Nucl. Instrum. Methods Phys. Res. Sect. B Beam Interact. Mater. Atoms 370, 94–100. doi:10.1016/j.nimb.2016.01.012
Segl, M., Mangini, A., Bonani, G., Hofmann, H., Nessi, M., Suter, M., et al. (1984). 10Be-dating of a manganese crust from central North Pacific and implications for ocean palaeocirculation. Nature 309, 540–543. doi:10.1038/309540a0
Smith, T., Cook, D. L., Merchel, S., Pavetich, S., Rugel, G., Scharf, A., et al. (2019). The constancy of galactic cosmic rays as recorded by cosmogenic nuclides in iron meteorites. Meteorit. Planet. Sci. 54, 2951–2976. doi:10.1111/maps.13417
Steier, P., Hain, K., Klötzli, U., Lachner, J., Priller, A., Winkler, S., et al. (2019). The actinide beamline at VERA. Nucl. Instrum. Methods Phys. Res. Sect. B Beam Interact. Mater. Atoms 458, 82–89. doi:10.1016/j.nimb.2019.07.031
Steier, P., Hrnecek, E., Priller, A., Quinto, F., Srncik, M., Wallner, A., et al. (2013). AMS of the minor plutonium isotopes. Nucl. Instrum. Methods Phys. Res. Sect. B Beam Interact. Mater. Atoms 294, 160–164. doi:10.1016/j.nimb.2012.06.017
Synal, H.-A. (2013). Developments in accelerator mass spectrometry. Int. J. Mass Spectrom. 349-350, 192–202. doi:10.1016/j.ijms.2013.05.008
Synal, H.-A. (2022). Accelerator mass spectrometry: ultra-sensitive detection technique of long-lived radionuclides. Chimia 76, 45–51. doi:10.2533/chimia.2022.45
Tighe, C., Castrillejo, M., Christl, M., Degueldre, C., Andrew, J., Semple, K. T., et al. (2021). Local and global trace plutonium contributions in fast breeder legacy soils. Nat. Commun. 12, 1381. doi:10.1038/s41467-021-21575-9
UNSCEAR (2000). “Sources and effects of ionizing radiation,” in United nations scientific committee on the effects of atomic radiation (UNSCEAR) 2000 report. doi:10.18356/49c437f9-en
UNSCEAR (2010). UNSCEAR 2008 - sources and effects of ionizing radiation. Report. doi:10.18356/cb7b6e26-en
Vivo-Vilches, C., Rugel, G., Lachner, J., Koll, D., Stübner, K., Fichter, S., et al. (2023). Pushing the limits of 41Ca AMS with CaF2 targets at DREAMS. Nucl. Instrum. Methods Phys. Res. Sect. B Beam Interact. Mater. Atoms 540, 188–193. doi:10.1016/j.nimb.2023.04.050
Vockenhuber, C., Alfimov, V., Christl, M., Lachner, J., Schulze-König, T., Suter, M., et al. (2013). The potential of He stripping in heavy ion AMS. Nucl. Instrum. Methods Phys. Res. Sect. B 294, 382–386. doi:10.1016/j.nimb.2012.01.014
von Blanckenburg, F., Belshaw, N., and O’Nions, R. (1996a). Separation of 9Be and cosmogenic 10Be from environmental materials and SIMS isotope dilution analysis. Chem. Geol. 129, 93–99. doi:10.1016/0009-2541(95)00157-3
von Blanckenburg, F., O’Nions, R., Belshaw, N., Gibb, A., and Hein, J. (1996b). Global distribution of beryllium isotopes in deep ocean water as derived from Fe-Mn crusts. Earth Planet. Sci. Lett. 141, 213–226. doi:10.1016/0012-821x(96)00059-3
Wallner, A., Bichler, M., Buczak, K., Dressler, R., Fifield, L. K., Schumann, D., et al. (2015). Settling the half-life of 60Fe: fundamental for a versatile astrophysical chronometer. Phys. Rev. Lett. 114, 041101. doi:10.1103/PhysRevLett.114.041101
Wallner, A., Feige, J., Fifield, L. K., Froehlich, M. B., Golser, R., Hotchkis, M. A. C., et al. (2020). 60Fe deposition during the late Pleistocene and the Holocene echoes past supernova activity. Proc. Natl. Acad. Sci. 117, 21873–21879. doi:10.1073/pnas.1916769117
Wallner, A., Feige, J., Kinoshita, N., Paul, M., Fifield, L., Golser, R., et al. (2016). Recent near-Earth supernovae probed by global deposition of interstellar radioactive 60Fe. Nature 532, 69–72. doi:10.1038/nature17196
Wallner, A., Fifield, L., Froehlich, M., Koll, D., Leckenby, G., Martschini, M., et al. (2023). Accelerator mass spectrometry with ANU’s 14 million volt accelerator. Nucl. Instrum. Methods Phys. Res. Sect. B Beam Interact. Mater. Atoms 534, 48–53. doi:10.1016/j.nimb.2022.10.021
Wallner, A., Froehlich, M. B., Hotchkis, M. A. C., Kinoshita, N., Paul, M., Martschini, M., et al. (2021). 60Fe and 244Pu deposited on Earth constrain the r-process yields of recent nearby supernovae. Science 372, 742–745. doi:10.1126/science.aax3972
Webber, W. R., and Higbie, P. R. (2003). Production of cosmogenic Be nuclei in the Earth’s atmosphere by cosmic rays: its dependence on solar modulation and the interstellar cosmic ray spectrum. J. Geophys. Res. Space Phys. 108. doi:10.1029/2003JA009863
Wilcken, K., Hotchkis, M., Levchenko, V., Fink, D., Hauser, T., and Kitchen, R. (2015). From carbon to actinides: a new universal 1MV accelerator mass spectrometer at ANSTO. Nucl. Instrum. Methods Phys. Res. Sect. B Beam Interact. Mater. Atoms 361, 133–138. doi:10.1016/j.nimb.2015.04.054
Keywords: accelerator mass spectrometry, radionuclides, environment, chemical purification, astrophysics
Citation: Fichter S, Koll D, Rolofs A and Wallner A (2024) Case studies of three geological archives for rare radionuclide measurements using accelerator mass spectrometry. Front. Environ. Chem. 5:1379862. doi: 10.3389/fenvc.2024.1379862
Received: 31 January 2024; Accepted: 16 February 2024;
Published: 01 March 2024.
Edited by:
Susanta Lahiri, Saha Institute of Nuclear Physics (SINP), IndiaReviewed by:
Gunther Korschinek, Technical University of Munich, GermanyTimothy Jull, University of Arizona, United States
Copyright © 2024 Fichter, Koll, Rolofs and Wallner. This is an open-access article distributed under the terms of the Creative Commons Attribution License (CC BY). The use, distribution or reproduction in other forums is permitted, provided the original author(s) and the copyright owner(s) are credited and that the original publication in this journal is cited, in accordance with accepted academic practice. No use, distribution or reproduction is permitted which does not comply with these terms.
*Correspondence: Sebastian Fichter, cy5maWNodGVyQGh6ZHIuZGU=; Dominik Koll, ZC5rb2xsQGh6ZHIuZGU=