- 1School of Human Settlements and Environmental Engineering, Xi’an Jiaotong University, Xi’an, China
- 2Department of Civil and Environmental Engineering, Faculty of Engineering, National University of Singapore, Singapore, Singapore
1 Introduction
Wastewater is now considered a resource where energy and valuable products can be generated (Hao et al., 2022a). Many presently widely used wastewater treatment technologies, such as activated sludge processes, advanced oxidation processes (AOPs) and membrane filtration, have the problems of large sludge output, low energy efficiency and considerable greenhouse gas (GHG) emission. Resource recovery is rarely considered in the design and construction of wastewater treatment projects, which is out of the standard of sustainable development (Smol et al., 2020). In the circular economy, resource recovery and recycling are becoming more critical perspectives in wastewater treatment beyond reducing the contaminants (Hao et al., 2022b). Carbon neutrality is presently a much-debated topic for wastewater treatment plants, involving the reduction of energy consumption, energy resources recovery, and GHG emissions (Bae and Kim, 2021).
Under the global circumstance of circular economy and carbon neutrality, technological innovations for reducing consumption and improving resource recovery are essential in wastewater treatment. Consumption reduction involves refining the treatment strategy with more effective use of energy and chemicals (Smol et al., 2020). Resource recovery involves the recovery of energy, nutrients, minerals and salts: 1) energy recovery includes heat utilization and generation of electricity, hydrogen (H2), and methane (CH4) from organics; 2) nutrient recovery refers to the utilization of nitrogen (N) and phosphorus (p) for fertilizer and biosolid production (Hao et al., 2022b); 3) recovery of valuable minerals includes the recycle of potassium (K), magnesium (Mg), copper (Cu) and silver (Ag), particularly that in industrial wastewater; and 4) salt recovery refers to the recovery of valuable salts from saline wastewater, which could even be potential energy source, e.g., osmotic power and salinity gradient power (Panagopoulos and Giannika, 2022a). The recovery of organics could significantly further contribute to reducing carbon dioxide (CO2) emission from wastewater treatment (Deng et al., 2022) and CH4 and N2O emissions from sludge disposal (Nagarajan et al., 2020). As reported, nearly 2.2 and 0.8 MMT CO2-equivalent CH4 and N2O would be released when producing 1 MMT through the municipal sludge digestion process, respectively (Nagarajan et al., 2020).
Instead of releasing resource-rich waste streams into the water bodies increasing the environmental risk, resource recovery could lead to multiple benefits, such as generating valuable products, greatly improving wastewater treatment processes, reclaiming wastewater, maintaining the ecological balance of aquatic environments, and reducing carbon footprint in wastewater disposal. Over the above, a perspective frame of resource recovery under the circumstance of circular economy and carbon neutrality could be established in Figure 1.
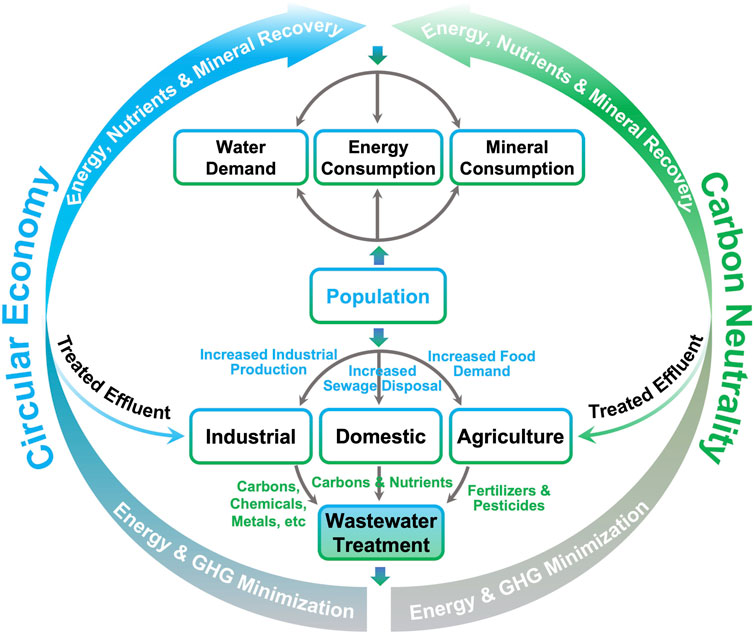
FIGURE 1. The perspective of resource recovery, energy consumption reduction and greenhouse gas emission minimization under the circumstance of circular economy and carbon neutrality.
Most reviews and suggestions in this field have focused on only one specific type of wastewater and technologies and lack overall opinions on the current situation of wastewater treatment from the perspective of resource recovery and GHG emission reduction. This paper aims to highlight the advanced wastewater treatment technologies and strategies status for treating various types of waste streams from the perspectives of circular economy and carbon neutrality, including developing, optimizing, and applying advanced technologies for consumption reduction and resource recovery. It could provide an overview as well as a primary reference of wastewater technologies for circular economy and carbon neutrality.
2 Technological opinions on energy consumption reduction and GHG emission minimization
A considerable amount of energy is required by the major wastewater processes accompanying GHG emissions. Treatment technology selection, operation condition optimization and technological advancement could contribute to energy consumption and GHG emission minimization.
2.1 Technology selection and optimization
Energy consumption in wastewater treatment plants (WWTPs) largely depends on the treatment processes. Traditional technologies, for example, their energy consumption in domestic wastewater treatment (DWW) could be sorted: constructed wetlands (0.253 kWh/m3) < anaerobic/anoxic/oxic process (0.267 kWh/m3) < conventional activated sludge process (CAS, 0.269 kWh/m3) < anoxic/oxic process (0.283 kWh/m3), oxidation ditch (0.302 kWh/m3) < sequencing batch reactor (0.336 kWh/m3) < membrane bioreactor (MBR, over 0.370 kWh/m3) (Yang et al., 2010; Krzeminski et al., 2012). Additionally, shortcut nitrification-denitrification could theoretically reduce 25% oxygen (O2) consumption and 40% organic carbon (OC) consumption, and anammox could theoretically respectively decrease 62.5% O2 consumption and 100% OC consumption (Adams et al., 2022), possessing a high potential of energy minimization and GHG emission reduction. Autotrophic denitrification (ADN) utilizes inorganic reductants, e.g., hydrogen (H2) (Deng et al., 2020b), iron (Fe0/Fe2+) (Peng et al., 2019) and sulfur compounds (S0, S2- and S2O32-) (Di Capua et al., 2019), as electron donors for denitrification, reducing 100% of OC consumption and corresponding CO2 emission. Moreover, ADN utilizes CO2 as a carbon source (Di Capua et al., 2019), obtaining the advantage of CO2 caption in denitrification.
Process optimization could further minimize energy consumption and GHG emission. In traditional WWTPs, aeration consumes almost 60% of the total energy (Lozano Avilés et al., 2019). Thus, many studies have focused on reducing energy consumption in aeration through aeration optimization, e.g., the propose of ammonia-N-based feedback aeration control strategy (Sun et al., 2016) and the air supply process optimization through modeling (Jiang et al., 2023). For instance, Jiang et al. (2023) applied a comprehensive model composed of a mass flow analysis module, an oxygen transfer rate module and an aeration optimization module to develop an aeration optimization strategy to reduce the energy consumption of a full-scale anaerobic/anoxic/oxic system. It reduced the energy-saving potential of blowers by 14.1% with an improved air supply prediction accuracy (Jiang et al., 2023). In terms of GHG emission, proper optimization of denitrification processes, e.g., the Fe0-/H2-supported ADN, could also reduce over 90% of the N2O emission (Li et al., 2017; Deng et al., 2020a). In addition to N2O, process optimization could also reduce CH4 production during H2 generation from wastewater, e.g., that in microbial electrolysis cells (MECs) (He et al., 2022).
2.2 Technology advancement
Technology advancement mainly involves the innovation, strengthening and combination of the existing technologies, which also showed potential for reducing energy consumption and GHG emission in wastewater treatment.
In carbon (i.e., COD, mg/L) to nitrogen (i.e., total nitrogen, mg/L) (C/N) ratio wastewater treatment, traditional biological treatment processes could be innovated by coupling ADN, reducing CO2 emission compared to dosing external OC (e.g., CH3OH and CH3COOH) (Deng et al., 2019). For instance, Fe0-ADN has been successfully introduced into the anaerobic/anoxic/oxic process (Peng et al., 2020) and constructed wetland (Deng et al., 2020b), and achieved efficient N removal. In ADN, OC addition could be an innovative strategy for GHG emission reduction. Deng et al. (2022) reported that OC dose at 0.25 mg-C/mg-N in Fe0-ADN could reduce N2O emission by over 80%.
In AOPs, innovations could be focused on developing and applying catalysts to reduce chemical and energy consumption (Wu et al., 2021). Ozonation as an example, Lakshmi et al. (2018) and Deng et al. (2021) respectively developed FeOx-doped granular activated carbon (Deng et al., 2021a) and TiO2/Fe3O4-composited graphene oxide (Jothinathan et al., 2021) to build catalytic ozonation processes, and both obtained enhanced performance and reduced ozone (O3) consumption, reducing the energy consumption.
In membrane processes, innovations should be focused on membrane fouling mitigation, e.g., membrane material and operational mode development, to improve energy efficiency (Deng et al., 2022). Deng et al. (2022) introduced iron-carbon galvanic cells (Fe0||C) into MBR, confirming a 22% improvement in phenolic-compounds removal and a 71% membrane fouling extension. Wang et al. (2021) developed a novel vibrating MBR for DWW treatment. It showed superior energy efficiency for fouling control and saved 51.7%–78.5% energy compared to the conventional air-sparging MBR (Wang et al., 2021).
Beyond innovation and strengthening, process combination is also an alternative. Deng et al. (2021b) combined ozonation with MBR for phenolic wastewater treatment. The pretreatment by ozonation reduced the acute biotoxicity and increased the 5-day biochemical oxygen demand (BOD5) to chemical oxygen demand (COD) ratio (BOD5/COD), contributing to performance enhancement and membrane fouling mitigation. This combination decreased the membrane fouling rate by over 88% and reduced the O3 consumption of ozonation by over 50% (Deng et al., 2021b).
3 Technological opinions on resource recovery
Wastewater is an abundant source of energy, nutrients and minerals. Energy recovery comprises heat extraction and energy generation from organics. As estimated, the COD-related energy in DWW is about 23 W per capita (Wang et al., 2017). For inorganic nutrients, Robles et al. (2020) estimated that the global resource recovery from DWW could retrieve p consumed by humans and could constitute around 50% of the present N market (Robles et al., 2020). Minerals and salts are also rich in waste streams, e.g., K and Mg in dairy and manure wastewater (Goglio et al., 2019a), Ag and Cu in deplating wastewater (Gu et al., 2020), Cd, Cu, Pb and Zn in mining processing wastewater (Meng et al., 2022) and Cr in tannery effluents (Li et al., 2020).
3.1 Energy recovery
3.1.1 Energy extraction from heat
DWW is a promising source of heat energy with the advantages of constant supply, abundant quantity and small temperature variation. A coefficient of performance of 2.2–5.5 for cooling and 1.8–10.6 for heating could be achieved by a wastewater-sourced heat pump (Hepbasli et al., 2014; Chae and Ren, 2016). Fouling of heat pump systems is the critical issue of wastewater-sourced heat recovery (Culha et al., 2015). Studies have been focused on developing anti-fouling technologies/designs and efficient cleaning devices (Qi et al., 2014). A WWTP has been utilized as a local thermal power station by connecting it to the district heating network, which covers the regional external heat demand (Kretschmer et al., 2021).
3.1.2 Energy production from organics
As containing a wealth of organics, wastewater possesses great energy production potential. Silvestre et al. (2015) indicated that 52% of the energy transferred to sludge could be recovered (Silvestre et al., 2015). Heidrich et al. precisely determined the energy content of domestic wastewater (DWW) through freeze-drying samples to minimize the loss of volatiles and organic matter. Their results indicated that DWW contains an energy generation potential of 7.6 kJ/L (Heidrich et al., 2011). Anaerobic technologies, including the up-flow anaerobic sludge blanket (UASB) and anaerobic MBR (AnMBR) based on the anaerobic digestion (AD) (Smith et al., 2014; Wang et al., 2020), and microbial electrochemical technologies (METs) including microbial fuel cells (MFCs), MECs and microbial recycling cells (MRCs) (Vadillo et al., 2013), are the main advancement in energy production from wastewater.
Among the anaerobic technologies, AnMBR is more efficient than conventional AD systems in biogas production and is currently in potential for practical application (Wang et al., 2020). The combined process of AnMBR and anammox has shown high effectiveness in energy production, where anammox could preserve OC from the denitrification (Dai et al., 2015). The process achieved 70% of energy self-sufficiency with a low energy consumption of 0.09 kWh/m3 (Dai et al., 2015). Whereas membrane fouling is the key issue of AnMBR which increases energy consumption and operation fee. The evaluation of the feasibility of AnMBR to the targeted wastewater and further studies on membrane fouling reduction are required.
METs enabled the generation of electricity or H2 from organic in wastewater (Vadillo et al., 2013). Their main advantages include either positive energy gain or low energy consumption due to biological intervention and relatively inexpensive operation costs (Kim et al., 2020). These advantages signified their potential to contribute to the circular economy and carbon neutrality. Nonetheless, up-scaled research and life cycle assessment (LCA) are still lacking in promoting application.
3.2 Recovery of nutrients
Various technologies have been devised for nutrient recovery from wastewater (Wu and Vaneeckhaute, 2021), including physicochemical technologies (e.g., crystallization and stripping), anaerobic technologies, photo-based biotechnologies (PBTs, e.g., microalgae-based technologies and photobacteria-based technologies) and METs.
Anaerobic technologies, e.g., AnMBR, also enables nutrients together with energy generation (Wang et al., 2022). However, coupled techniques, e.g., MgNH4PO4•6H2O (MAP) formation, NH3 stripping-absorption, membrane distillation or PBTs, are required for nutrient recovery from AnMBR-treated effluent. PBTs are promising alternatives for nutrient recovery, capturing CO2 from the air and producing a small carbon footprint (You et al., 2023). The combined AnMBR-PBTs process enabled the efficient simultaneous recovery of energy and nutrients and addressed the problem of microalgae and photobacteria harvest in traditional PBTs (Li et al., 2022). However, PBTs are sensitive to toxicity from heavy metals or organics (Tiang et al., 2020). Hence, they are mainly considered for DWW treatment, whereas the development of toxicity resistance photobacteria and corresponding treatment systems are in need to widen the application of PBTs (Li et al., 2022; You et al., 2023).
METs, especially MRCs, also could recover nutrients during energy generation and exhibited a much higher toxicity tolerance than PBTs. Huggins et al. (2016) developed an MRC based on a tubular overflow style MFC for resource recovery from actual industrial wastewater. The system achieved a COD removal rate of 0.55 kg/(m3·d) and a maximum power density of 6 W/m3, and recovered 2 g P/kg and cathode 1 g K/kg on the cathode in 20 days’ continuous operation (Huggins et al., 2016). METs are constructed from low-cost, biogenic and biocompatible materials that recover organic carbon and nutrients (Goglio et al., 2019b). With the development of MRCs, the electrodes saturated by micro- and macro-nutrients could be reused as organic-mineral fertilizers (Goglio et al., 2019a). The concept of MRCs also greatly increased the possibility of scaling up of METs (Deng et al., 2023). Thus, MRCs are potential biotechnology for energy and nutrient recovery from wastewater, and efficiency improvement and up-scaling are the future perspectives.
3.3 Recovery of minerals and salts
MRCs also enable the recovery of minerals, especially those that can be utilized as nutrients (e.g., K and Mg), and the products could be applied as organic-mineral conditioners or fertilizers for agricultural soil improvement (Goglio et al., 2019a). Nevertheless, many of the other minerals, e.g., the toxic heavy metals, are not suitable to be recovered by MRCs due to their side effects in agricultural application (Goglio et al., 2019b). Otherwise, they can be effectively recovered by traditional adsorption, sedimentation, electro-deposition and electrodialysis processes (Taghvaie Nakhjiri et al., 2022). As MRCs have the risk enriching toxic minerals, the risk of the products to agricultural soil should be evaluated prior to application.
Several technologies have been developed and applied for the valuable salts recovery from industrial and domestic wastewater, such as the thermal process (e.g., multi-effect distillation and multi-stage flash distillation), electrochemical process, and membrane process (e.g., high-pressure reverse osmosis and forward osmosis) (Panagopoulos and Giannika, 2022b). Panagopoulos et al., 2022b treated brine with high-pressure reverse osmosis (HPRO) and obtained a salt recovery of up to 0.435 under the optimum conditions. In addition, the use of energy recovery systems has reduced energy consumption by about 26% (Panagopoulos, 2022). Although HPRO is a promising technology for saline wastewater and salt recovery, its performance is also limited by problems such as scaling, membrane fouling and membrane compaction (Panagopoulos, 2022). Future research should focus on the development of new membrane materials and the stability of the membrane treatment process.
4 Discussion and conclusion
A considerable amount of energy is consumed and GHGs are released during wastewater treatment. Treatment technology selection and optimization, and technological advancement could markedly minimize energy consumption and GHG emission. Moreover, wastewater is an abundant energy source, nutrients, and minerals. Instead of releasing resource-rich waste streams into the water bodies increasing the environmental risk, resource recovery could lead to multiple benefits, such as generating valuable products and reducing carbon footprint, contributing to the circular economy and carbon neutrality.
To achieve the goal of circular economy and carbon neutralization, the development, optimization and application of advanced technologies have been proceeded for low consumption and resource recovery. Figure 2 summarizes the technological suggestions on energy and GHG minimization and resource recovery in wastewater treatment. Treatment technology selection, operation condition optimization and technological advancement are critical for energy consumption reduction and GHG emission minimization. In terms of resource recovery, the proper application and optimization of technologies corresponding to the targeted resource are essential. While in many cases, a technology or a technology combination should be considered aiming for the simultaneous recovery of several valuables.
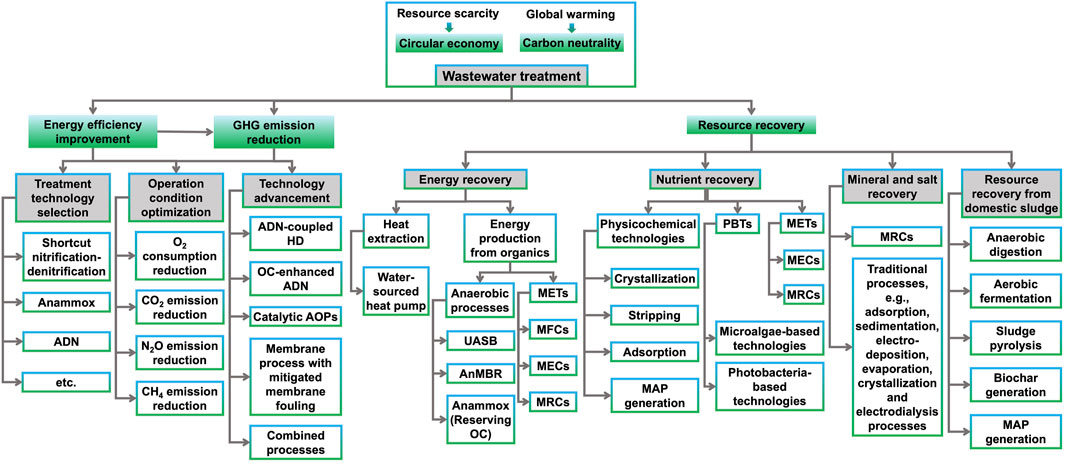
FIGURE 2. Potential technologies for the minimization of energy consumption and GHG emission and resource recovery in wastewater treatment. GHG, greenhouse gas; ADN, autotrophic denitrification; HD, heterotrophic denitrification; OC, organic carbon; AOPs, advanced oxidation processes; UASB, up-flow anaerobic sludge blanket; AnMBR, anaerobic membrane bioreactor; METs, microbial electrochemical processes; MFCs, microbial fuel cells; MECs, microbial electrolysis cells; MRCs, microbial recycling cells; MAP, magnesium ammonium phosphate; PBTs, photo-based biotechnologies.
This paper first overviewed the development, optimization and application of the advanced technologies for low consumption and resource recovery. Technological opinions on energy consumption reduction, GHG emission minimization and resource recovery are also proposed. It could direct the follow-up research, development and successful implementation of technologies toward the circular economy and carbon neutrality in wastewater treatment.
Although technology are being advanced, benchmark projects and standard protocols are rarely found in the literature, and comparison among the appropriate benchmarks can hardly be conducted. Challenges are still existing in practical implementation. Therefore, further efforts are essential in up-scaled research for benchmarking, LCA, risk assessment and standard protocols are required for promoting and guiding successful implementation.
Author contributions
CW: Conceptualization, Data curation, Investigation, Writing–original draft. S-HD: Conceptualization, Funding acquisition, Supervision, Writing–original draft. NY: Data curation, Investigation, Writing–review and editing. YB: Data curation, Investigation, Writing–review and editing. PJ: Conceptualization, Supervision, Writing–review and editing. JH: Supervision, Writing–review and editing.
Funding
This work is funded by the Qinchuangyuan High-level Talents Recruiting Program of Shaanxi Province, China (No. QCYRCXM-2022-227) and the Natural Science Foundation of Shaanxi Province for Youth, China (No. 2023-JC-QN-0477).
Conflict of interest
The authors declare that the research was conducted in the absence of any commercial or financial relationships that could be construed as a potential conflict of interest.
Publisher’s note
All claims expressed in this article are solely those of the authors and do not necessarily represent those of their affiliated organizations, or those of the publisher, the editors and the reviewers. Any product that may be evaluated in this article, or claim that may be made by its manufacturer, is not guaranteed or endorsed by the publisher.
References
Adams, M., Xie, J., Kabore, A. W. J., Chang, Y., Xie, J., Guo, M., et al. (2022). Research advances in anammox granular sludge: A review. Crit. Rev. Environ. Sci. Technol. 52, 631–674. doi:10.1080/10643389.2020.1831358
Bae, S., and Kim, Y. M. (2021). Carbon-neutrality in wastewater treatment plants: advanced technologies for efficient operation and energy/resource recovery. Energies 14, 8514. doi:10.3390/en14248514
Chae, K. J., and Ren, X. (2016). Flexible and stable heat energy recovery from municipal wastewater treatment plants using a fixed-inverter hybrid heat pump system. Appl. energy 179, 565–574. doi:10.1016/j.apenergy.2016.07.021
Culha, O., Gunerhan, H., Biyik, E., Ekren, O., and Hepbasli, A. (2015). Heat exchanger applications in wastewater source heat pumps for buildings: A key review. Energy Build. 104, 215–232. doi:10.1016/j.enbuild.2015.07.013
Dai, W., Xu, X., Liu, B., and Yang, F. (2015). Toward energy-neutral wastewater treatment: A membrane combined process of anaerobic digestion and nitritation–anammox for biogas recovery and nitrogen removal. Chem. Eng. J. 279, 725–734. doi:10.1016/j.cej.2015.05.036
Deng, S., Jothinathan, L., Cai, Q., Li, R., Wu, M., Ong, S. L., et al. (2021a). FeOx@GAC catalyzed microbubble ozonation coupled with biological process for industrial phenolic wastewater treatment: catalytic performance, biological process screening and microbial characteristics. Water Res. 190, 116687. doi:10.1016/j.watres.2020.116687
Deng, S., Li, D., Yang, X., Cai, Q., Peng, S., Peng, X., et al. (2019). Novel characteristics on micro-electrolysis mediated Fe(0)-oxidizing autotrophic denitrification with aeration: efficiency, iron-compounds transformation, N2O and NO2− accumulation, and microbial characteristics. Chem. Eng. J. 387, 123409. doi:10.1016/j.cej.2019.123409
Deng, S., Peng, S., Ngo, H. H., Oh, S. J. A., Hu, Z., Yao, H., et al. (2022). Characterization of nitrous oxide and nitrite accumulation during iron (Fe(0))- and ferrous iron (Fe(II))-driven autotrophic denitrification: mechanisms, environmental impact factors and molecular microbial characterization. Chem. Eng. J. 438, 135627. doi:10.1016/j.cej.2022.135627
Deng, S., Peng, S., Xie, B., Yang, X., Sun, S., Yao, H., et al. (2020a). Influence characteristics and mechanism of organic carbon on denitrification, N2O emission and NO2− accumulation in the iron [Fe(0)]-oxidizing supported autotrophic denitrification process. Chem. Eng. J. 393, 124736. doi:10.1016/j.cej.2020.124736
Deng, S., Wang, C., Ngo, H. H., Guo, W., You, N., Tang, H., et al. (2023). Comparative review on microbial electrochemical technologies for resource recovery from wastewater towards circular economy and carbon neutrality. Bioresour. Technol. 376, 128906. doi:10.1016/j.biortech.2023.128906
Deng, S., Wang, Q., Cai, Q., Ong, S. L., and Hu, J. (2021b). Efficient bio-refractory industrial wastewater treatment with mitigated membrane fouling in a membrane bioreactor strengthened by the micro-scale ZVI@GAC galvanic-cells-initiated radical generation and coagulation processes. Water Res. 209, 117943. doi:10.1016/j.watres.2021.117943
Deng, S., Xie, B., Kong, Q., Peng, S., Wang, H., Hu, Z., et al. (2020b). An oxic/anoxic-integrated and Fe/C micro-electrolysis-mediated vertical constructed wetland for decentralized low-carbon greywater treatment. Bioresour. Technol. 315, 123802. doi:10.1016/j.biortech.2020.123802
Di Capua, F., Pirozzi, F., Lens, P. N. L., and Esposito, G. (2019). Electron donors for autotrophic denitrification. Chem. Eng. J. 362, 922–937. doi:10.1016/j.cej.2019.01.069
Goglio, A., Marzorati, S., Rago, L., Pant, D., Cristiani, P., and Schievano, A. (2019a). Microbial recycling cells: first steps into a new type of microbial electrochemical technologies, aimed at recovering nutrients from wastewater. Bioresour. Technol. 277, 117–127. doi:10.1016/j.biortech.2019.01.039
Goglio, A., Tucci, M., Rizzi, B., Colombo, A., Cristiani, P., and Schievano, A. (2019b). Microbial recycling cells (MRCs): A new platform of microbial electrochemical technologies based on biocompatible materials, aimed at cycling carbon and nutrients in agro-food systems. Sci. Total Environ. 649, 1349–1361. doi:10.1016/j.scitotenv.2018.08.324
Gu, J.-N., Liang, J., Chen, C., Li, K., Zhou, W., Jia, J., et al. (2020). Treatment of real deplating wastewater through an environmental friendly precipitation-electrodeposition-oxidation process: recovery of silver and copper and reuse of wastewater. Sep. Purif. Technol. 248, 117082. doi:10.1016/j.seppur.2020.117082
Hao, X., Wang, X., Shi, C., Van Loosdrecht, M. C. M., and Wu, Y. (2022a). Creating coagulants through the combined use of ash and brine. Sci. Total Environ. 845, 157344. doi:10.1016/j.scitotenv.2022.157344
Hao, X., Wu, D., Li, J., Liu, R., and Van Loosdrecht, M. (2022b). Making waves: A sea change in treating wastewater – why thermodynamics supports resource recovery and recycling. Water Res. 218, 118516. doi:10.1016/j.watres.2022.118516
He, K., Li, W., Tang, L., Li, W., Lv, S., and Xing, D. (2022). Suppressing methane production to boost high-purity hydrogen production in microbial electrolysis cells. Environ. Sci. Technol. 56, 11931–11951. doi:10.1021/acs.est.2c02371
Hepbasli, A., Biyik, E., Ekren, O., Gunerhan, H., and Araz, M. (2014). A key review of wastewater source heat pump (WWSHP) systems. Energy Convers. Manag. 88, 700–722. doi:10.1016/j.enconman.2014.08.065
Huggins, T. M., Latorre, A., Biffinger, J. C., and Ren, Z. J. (2016). Biochar based microbial fuel cell for enhanced wastewater treatment and nutrient recovery. Sustainability 8, 169. doi:10.3390/su8020169
Jiang, L. M., Zhang, W., Li, Y., Shao, Y., Zhang, Z., Zhang, M., et al. (2023). Applying mass flow analysis and aeration optimization strategy to reduce energy consumption of a full-scale anaerobic/anoxic/oxic system. J. Water Process Eng. 54, 104037. doi:10.1016/j.jwpe.2023.104037
Jothinathan, L., Cai, Q. Q., Ong, S. L., and Hu, J. Y. (2021). Organics removal in high strength petrochemical wastewater with combined microbubble-catalytic ozonation process. Chemosphere 263, 127980. doi:10.1016/j.chemosphere.2020.127980
Kim, T., An, J., Jang, J. K., and Chang, I. S. (2020). Determination of optimum electrical connection mode for multi-electrode-embedded microbial fuel cells coupled with anaerobic digester for enhancement of swine wastewater treatment efficiency and energy recovery. Bioresour. Technol. 297, 122464. doi:10.1016/j.biortech.2019.122464
Kretschmer, F., Hrdy, B., Neugebauer, G., and Stoeglehner, G. (2021). Wastewater treatment plants as local thermal power stations—modifying internal heat supply for covering external heat demand. Processes 9, 1981. doi:10.3390/pr9111981
Krzeminski, P., Van Der Graaf, J. H. J. M., and Van Lier, J. B. (2012). Specific energy consumption of membrane bioreactor (MBR) for sewage treatment. Water Sci. Technol. 65, 380–392. doi:10.2166/wst.2012.861
Li, H., Watson, J., Zhang, Y., Lu, H., and Liu, Z. (2020). Environment-enhancing process for algal wastewater treatment, heavy metal control and hydrothermal biofuel production: A critical review. Bioresour. Technol. 298, 122421. doi:10.1016/j.biortech.2019.122421
Li, P., Wang, Y., Zuo, J., Wang, R., Zhao, J., and Du, Y. (2017). Nitrogen removal and N2O accumulation during hydrogenotrophic denitrification: influence of environmental factors and microbial community characteristics. Environ. Sci. Technol. 51, 870–879. doi:10.1021/acs.est.6b00071
Li, S.-N., Zhang, C., Li, F., Ren, N. Q., and Ho, S.-H. (2022). Recent advances of algae-bacteria consortia in aquatic remediation. Crit. Rev. Environ. Sci. Technol. 53, 315–339. ahead-of-print. doi:10.1080/10643389.2022.2052704
Lozano Avilés, A. B., Del Cerro Velázquez, F., and Llorens Pascual Del Riquelme, M. (2019). Methodology for energy optimization in wastewater treatment plants. Phase I: control of the best operating conditions. Sustainability 11, 3919. doi:10.3390/su11143919
Meng, S., Wen, S., Han, G., Wang, X., and Feng, Q. (2022). Wastewater treatment in mineral processing of non-ferrous metal resources: A review. Water 14, 726. doi:10.3390/w14050726
Nagarajan, D., Lee, D. J., Chen, C. Y., and Chang, J. S. (2020). Resource recovery from wastewaters using microalgae-based approaches: A circular bioeconomy perspective. Bioresour. Technol. 302, 122817. doi:10.1016/j.biortech.2020.122817
Panagopoulos, A., and Giannika, V. (2022a). Comparative techno-economic and environmental analysis of minimal liquid discharge (MLD) and zero liquid discharge (ZLD) desalination systems for seawater brine treatment and valorization. Sustain. Energy Technol. Assessments 53, 102477. doi:10.1016/j.seta.2022.102477
Panagopoulos, A., and Giannika, V. (2022b). Decarbonized and circular brine management/valorization for water & valuable resource recovery via minimal/zero liquid discharge (MLD/ZLD) strategies. J. Environ. Manag. 324, 116239. doi:10.1016/j.jenvman.2022.116239
Peng, S., Deng, S., Li, D., Xie, B., Yang, X., Lai, C., et al. (2020). Iron-carbon galvanic cells strengthened anaerobic/anoxic/oxic process (Fe/C-A2O) for high-nitrogen/phosphorus and low-carbon sewage treatment. Sci. Total Environ. 722, 137657. doi:10.1016/j.scitotenv.2020.137657
Peng, S., Kong, Q., Deng, S., Xie, B., Yang, X., Li, D., et al. (2019). Application potential of simultaneous nitrification/Fe0-supported autotrophic denitrification (SNAD) based on iron-scraps and micro-electrolysis. Sci. Total Environ. 711, 135087. doi:10.1016/j.scitotenv.2019.135087
Qi, Z., Gao, Q., Liu, Y., Yan, Y. Y., and Spitler, J. D. (2014). Status and development of hybrid energy systems from hybrid ground source heat pump in China and other countries. Renew. Sustain. Energy Rev. 29, 37–51. doi:10.1016/j.rser.2013.08.059
Robles, A., Aguado, D., Barat, R., Borras, L., Bouzas, A., Gimenez, J. B., et al. (2020). New frontiers from removal to recycling of nitrogen and phosphorus from wastewater in the Circular Economy. Bioresour. Technol. 300, 122673. doi:10.1016/j.biortech.2019.122673
Silvestre, G., Fernández, B., and Bonmatí, A. (2015). Significance of anaerobic digestion as a source of clean energy in wastewater treatment plants. Energy Convers. Manag. 101, 255–262. doi:10.1016/j.enconman.2015.05.033
Smith, A. L., Stadler, L. B., Cao, L., Love, N. G., Raskin, L., and Skerlos, S. J. (2014). Navigating wastewater energy recovery strategies: A life cycle comparison of anaerobic membrane bioreactor and conventional treatment systems with anaerobic digestion. Environ. Sci. Technol. 48, 5972–5981. doi:10.1021/es5006169
Sun, J., Liang, P., Yan, X., Zuo, K., Xiao, K., Xia, J., et al. (2016). Reducing aeration energy consumption in a large-scale membrane bioreactor: process simulation and engineering application. Water Res. 93, 205–213. doi:10.1016/j.watres.2016.02.026
Taghvaie Nakhjiri, A., Sanaeepur, H., Ebadi Amooghin, A., and Shirazi, M. M. A. (2022). Recovery of precious metals from industrial wastewater towards resource recovery and environmental sustainability: A critical review. Desalination 527, 115510. doi:10.1016/j.desal.2021.115510
Tiang, M. F., Hanipa, M. A. F., Abdul, P. M., Jahim, J. M., Mahmod, S. S., Takriff, M. S., et al. (2020). Recent advanced biotechnological strategies to enhance photo-fermentative biohydrogen production by purple non-sulphur bacteria: an overview. Int. J. Hydrogen Energy 45, 13211–13230. doi:10.1016/j.ijhydene.2020.03.033
Vadillo, V., Sánchez-Oneto, J., Portela, J. R., and Martínez De La Ossa, E. J. (2013). Problems in supercritical water oxidation process and proposed solutions. Industrial Eng. Chem. Res. 52, 7617–7629. doi:10.1021/ie400156c
Wang, C., Ng, T. C. A., and Ng, H. Y. (2021). Comparison between novel vibrating ceramic MBR and conventional air-sparging MBR for domestic wastewater treatment: performance, fouling control and energy consumption. Water Res. 203, 117521. doi:10.1016/j.watres.2021.117521
Wang, K., Sheng, Y., Cao, H., Yan, K., and Zhang, Y. (2017). Impact of applied current on sulfate-rich wastewater treatment and microbial biodiversity in the cathode chamber of microbial electrolysis cell (MEC) reactor. Chem. Eng. J. 307, 150–158. doi:10.1016/j.cej.2016.07.106
Wang, K., Soares, A., Jefferson, B., Wang, H., Zhang, L., Jiang, S., et al. (2020). Establishing the mechanisms underpinning solids breakthrough in UASB configured anaerobic membrane bioreactors to mitigate fouling. Water Res. 176, 115754. doi:10.1016/j.watres.2020.115754
Wang, S., Liu, H., Gu, J., Zhang, M., and Liu, Y. (2022). Towards carbon neutrality and water sustainability: an integrated anaerobic fixed-film MBR-reverse osmosis-chlorination process for municipal wastewater reclamation. Chemosphere 287, 132060. doi:10.1016/j.chemosphere.2021.132060
Wu, H., and Vaneeckhaute, C. (2021). Nutrient recovery from wastewater: A review on the integrated physicochemical technologies of ammonia stripping, adsorption and struvite precipitation. Chem. Eng. J. 433, 133664. doi:10.1016/j.cej.2021.133664
Wu, M. Y., Cai, Q. Q., Xu, H. P., Ong, S. L., and Hu, J. Y. (2021). Simulation of FBR-Fenton/GAC process for recalcitrant industrial wastewater treatment with a computational fluid dynamics-kinetic model framework. Water Res. 203, 117504. doi:10.1016/j.watres.2021.117504
Yang, L., Zeng, S., Chen, J., He, M., and Yang, W. (2010). Operational energy performance assessment system of municipal wastewater treatment plants. Water Sci. Technol. 62, 1361–1370. doi:10.2166/wst.2010.394
Keywords: energy minimization, greenhouse gas emission, wastewater treatment technologies, circular economy, carbon neutrality
Citation: Wang C, Deng S-H, You N, Bai Y, Jin P and Han J (2023) Pathways of wastewater treatment for resource recovery and energy minimization towards carbon neutrality and circular economy: technological opinions. Front. Environ. Chem. 4:1255092. doi: 10.3389/fenvc.2023.1255092
Received: 08 July 2023; Accepted: 21 September 2023;
Published: 17 October 2023.
Edited by:
Nadeem A. Khan, Assistant Professor Mewat Engineering College, IndiaReviewed by:
Zhongsen Yan, Fuzhou University, ChinaCopyright © 2023 Wang, Deng, You, Bai, Jin and Han. This is an open-access article distributed under the terms of the Creative Commons Attribution License (CC BY). The use, distribution or reproduction in other forums is permitted, provided the original author(s) and the copyright owner(s) are credited and that the original publication in this journal is cited, in accordance with accepted academic practice. No use, distribution or reproduction is permitted which does not comply with these terms.
*Correspondence: Shi-Hai Deng, c2hpaGFpLmRlbmdAeGp0dS5lZHUuY24=