- 1Department of Civil and Environmental Engineering and Architecture (DICAAR), University of Cagliari, Cagliari, Italy
- 2Department of Applied Chemistry, National School of Agro-Industrial Sciences (ENSAI), University of Ngaoundéré, Ngaoundéré, Cameroon
- 3Environmental Geology and Geoengineering Institute of the National Research Council (IGAG-CNR), Cagliari, Italy
- 4Fabbrica Italiana Leghe Metalliche Sinterizzate (F.I.L.M.S.) SpA, Anzola d’Ossola, Italy
- 5Department of Chemistry, Green Chemistry Centre of Excellence, University of York, York, United Kingdom
- 6Department of Chemistry, Life Sciences and Environmental Sustainability, University of Parma, Parma, Italy
- 7Unit of National Interuniversity Consortium for Materials Science and Technology (INSTM), Florence, Italy
Peculiar chemical, mechanical, and magnetic properties make cobalt a key metal for a variety of “hot” applications like the cathode production of Li-ion batteries. Cobalt is also the preferred metallic binder for tungsten carbide tool manufacturing. The recent increasing criticality of cobalt and tungsten is driving the interest of manufacturers and researchers toward high-rate recycling of hard-metal (HM) waste for limiting the demand for raw materials. A simple and environmentally friendly hydrometallurgical route for Co-selective dissolution from HM wastes was developed by using weak, bio-derived, and biodegradable organic acids (OAs). In this study, OAs, namely, acetic (HAc), citric (H3Cit), maleic (H2Mal), lactic (HLac), succinic (H2Suc), lactobionic (HLB), and itaconic (H2It) acids, were selected for their pKa1 values spanning from 1.8 to 4.7 and systematically tested as selective cobalt leaching agents from WC-Co-based wastes in water, isolating the formed complexes in the solid state. Thereby, all of them seemed to be efficient in selective Co leaching, achieving almost quantitative Co dissolution from HM by-products still at low concentration levels and room conditions in a short time, leaving the residual WC unreacted and ready to be re-employed for industrial purposes. Nevertheless, two main categories of organic acids were distinguished depending on their oxidizing/complexing behavior: class 1 OAs, where the metal oxidation is carried out by H+, and class 2 OAs, where oxidation is carried out by an external oxidant like O2. A combined experimental/theoretical investigation is described here to show the reasons behind this peculiar behavior and lay the foundation for a wider discussion on the leaching capabilities of OAs toward elemental metals. Due to the demonstrated effectiveness, low cost, eco-friendliness, and large availability through biotechnological fermentative processes, particular attention is devoted here to the use of HLac in hydrometallurgy as an example of class 2 OA. WC-Co materials recovered by HLac mild hydrometallurgy demonstrated a metallurgical quality suitable for re-employment in the HM manufacturing process.
1 Introduction
Cobalt is a metal with peculiar chemical, mechanical, and magnetic properties that allow its use in numerous applications such as magnetic alloys (Barbosa et al., 2004), catalysts (Ackerman et al., 2015), batteries (especially Li-ion) (Chen et al., 2018; Golmohammadzadeh et al., 2018), and other high-performance material production (Ortner et al., 2015; Tang et al., 2016). Among the latter, cemented carbides, also referred to as hard metals (HMs), are liquid-phase sintered composite materials consisting of at least one hard and wear-resistant phase (WC in the majority of cases) embedded in a soft and ductile metallic one based on one or more elements from the iron group, i.e., Co, Ni, and Fe, with Co and its alloys being the most widely used, which act as a matrix binder (Shemi et al., 2018; Srivastava et al., 2019; Konyashin et al., 2022a). Thanks to their composite nature, HMs exhibit an excellent combination of wear resistance and toughness, making them the natural choice for metal forming, cutting tools, and wear parts for decades (Ortner et al., 2015). The wide and growing industrial use of cobalt and its limited deposits worldwide, localized in a few countries, makes it a critical metal of strategic importance (Shemi et al., 2018). Specifically, in 2021, a 9.2 kt Co demand for hard-metal production (5.3% of global demand of Co) was recorded, of which around 60% was supplied by the Democratic Republic of the Congo (DRC) (García et al., 2019; U.S. Geological Survey, 2022). On account of this aforementioned scarcity and socio-political concerns related to the most relevant cobalt-supplying countries, methods addressed to limit the use of natural reserves by recovering them from secondary raw materials like waste are stimulated, in line with worldwide directives addressed to circular economy models (The Royal Society of Chemistry Hunt, 2013; Directorate-General for Internal Market et al., 2018).
Currently, a variety of methods for the enhancement of cobalt-containing waste generally exploit the use of dangerous reagents (primarily strong oxidizing acids) and/or aggressive operating conditions (high temperatures) (Zeiler et al., 2021; Konyashin et al., 2022b). In the last decade, the use of bio-derived organic acids (OAs) as leaching agents has gained increasing attention for being safe, renewable, and, in some cases, cheap substances (Burckhard et al., 1995; Musariri et al., 2019; Cera et al., 2023). Thanks to their capability to dissolve low-reduction potential metals, some of them have been recently proposed as suitable leaching agents for the transition and recovery of rare earth1 elements from several kinds of waste. As an example, citric acid has been proven to work as a powerful and green agent for Cu-based slag enhancement, effectively and selectively removing Co, Ni, and Fe metal impurities from the composite material (Meshram et al., 2017). Similarly, citric and acetic acids were satisfactorily employed as effective leaching agents for NbFeB magnets–where also Co, Ni, and several other REEs in low amounts are present in mild conditions, achieving material dissolution in a short time (Gergoric et al., 2018). Recently, we demonstrated the selectivity and efficacy of a new solvometallurgical Co-leaching method from HM powders based on the use of diluted maleic acid (H2Mal) ethanolic solutions working under gentle operative conditions (Oumarou Amadou et al., 2021). Specifically, H2Mal (0.5 M, EtOH) selectively and quantitatively leached Co trapped within WC-Co powders within 4 h at room temperature, leaving WC unreacted and ready for re-employment in HM manufacturing.
In these processes, the organic acid can work as a complexing and oxidizing—by H+— agent toward elemental state metals. More specifically, it will be able to couple complexing and oxidizing actions when its pKa and/or the Kdiss of the formed complex (Complex dissociation constant, Kdiss = 1/complex formation constant, Kf) are very low. Differently, the role played by the acid is mainly related to the complexing properties of its deprotonated (anionic) form; thus, an external oxidizing agent is necessary for the metal(0) leaching, as observed in the case of the use of acetic acid solutions (pKa = 4.7) toward WC-Co wastes, which requires forced O2 addition to the leaching system for driving the Co-dissolution reaction (Edtmaier et al., 2005). Other examples are related to the use of OAs for metal leaching from oxidized materials. Due to the amount of waste we will soon have to deal with, the case attracting the most attention recently is the application of OAs like acetic, ascorbic, citric, lactic, maleic, malic, and succinic, in the presence of H2O2, for lithium and cobalt recovery from spent Li-ion batteries (LIBs) (Li et al., 2013; Golmohammadzadeh et al., 2018). Furthermore, several examples of Co recovery from oxidized HM scraps were also proposed, as in the case of the use of malic acid/H2O2 for Co leaching from CoWO4 (Seo and Kim, 2016). In these cases, the role of the organic acid is mainly related to its coordinative behavior toward the metal ion and the formation of metal complexes soluble in the leaching solvent. In some cases, lower effectiveness in leaching reactions carried out by weak organic acids compared to strong inorganic acids is observed. Against this, the use of weak coordinating acids may increase selectivity, limit by-product formation, and make the process safer for operators and the environment. It is noteworthy that a good exposure of metal values to the chemical etching results in good improvements in the leaching rate.
On this basis, the present paper introduces a fundamental study of the reactions between several selected bio-derived organic acids and cobalt metal in water. OAs were selected among complexing carboxylic acids based on their easy availability, renewability, and eco-friendliness, taking into account their acidity in water, as summarized in Table 1. The systematic approach allowed us to highlight, through experimental evidence and calculations, how the complexing capability of the leaching agent, together with its Ka1, may affect the reduction potential of both the metal and the proton. The role of the acid in the reaction, the most appropriate leaching conditions, and the obtained complexes are here systematically investigated, allowing the suitability of the different acids for HM waste enhancement applications to be assessed. Then, the manuscript describes a full process of material recovery from WC-Co waste powders by using diluted aqueous HLac, identified as the most promising for practical applications due to its low cost, high efficiency toward both Co powder and Co contained as a binder in the real WC-Co powders, and large availability through organic substrate fermentation (Oumarou Amadou et al., 2023).
2 Materials and methods
All chemicals were used as purchased without any purification (Sigma-Aldrich: succinic acid, 99%. TCI: maleic acid, >99.0%. Carlo Erba: citric acid monohydrate RPE and acetic acid glacial RPE. Acros Organics: itaconic acid, 99+% and lactobionic acid, 97%. ACEF: lactic acid FU-BP E 270). Cobalt powder was used as purchased from Umicore (Extrafine, >99.5 wt. %, 1.3 μm). WC-Co powders (RC-627C and RC-631L) were provided by F.I.L.M.S. SpA, Anzola D’Ossola, VB (IT).
2.1 Test specimen definition
The WC-Co powders studied throughout this work, namely, RC-627C and RC-631L, are by-products of HM manufacturing generated during machining operations by F.I.L.M.S. SpA.
As detailed in the study by Oumarou Amadou et al. (2023), both powders show an approximate 20 wt. % of Co binder amount in the mixture, while mainly differing for the WC grain size and the mean free path value with RC-631L, which shows a wider grain size distribution than RC-627C. Table 2 summarizes the main elements’ composition of the test specimens, and the experimental procedures are given in Sect. S1.2.
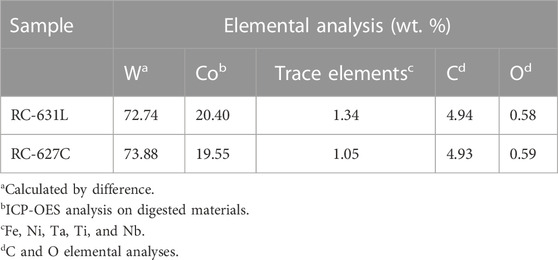
TABLE 2. Metal composition (wt. %) of the recovery powders (RC-631L and RC-627C) used throughout the present study (see Section 1.2 for experimental procedures) (Oumarou Amadou et al., 2021).
The morphology and WC particle size of these powdered samples were determined using scanning electron microscopy (SEM), while optical microscopy (OM) was used to characterize the corresponding sintered samples.
2.2 Characterization of samples and products
Unless expressly indicated, the analytical data are reported considering the standard deviation of +/−1 on the last significant figure.
2.2.1 Chemical characterization of leachates
Leachate aliquots spanning from 0.1 to 5 mL were introduced in TFM vessels and dried. Mixtures of HNO3 (65%, 4 mL), H2SO4 (96%, 4 mL), and H2O (deionized, 2 mL) were then added to the dried materials and submitted to a digestion cycle in a Milestone Ethos 1 Microwave digester, equipped with an HPR1000/10S high-pressure segmented rotor, an ATC-400CE automatic temperature control, and a Terminal 640 with easyCONTROL software. The treatment was performed by applying a microwave program consisting of two steps lasting 10 min each at 200°C and microwave power up to 1000 W. The samples were prepared for analysis by diluting the digested solutions with 1% HNO3, and then Co was determined by ICP-OES on a Perkin Elmer Optima DV 7000, with respect to five-point calibration plots in the 0.01–10 ppm range.
2.2.2 Chemical characterization of solid samples
A total of 0.5 g WC-based powder aliquots were reacted with 1 L of H2O2 30 vol. % in the presence of 10 mL of concentrated nitric acid and 10 mL of hydrofluoric acid by heating at around 150°C for 15 min until the reaction became vigorous and then left to react at room temperature. 15 mL of tartaric acid solution was added to the mixture for preventing the precipitation of tungstic acid. 10 mL of H2O2 was also added before dilution with deionized water. The freshly prepared solutions were analyzed for Co, Fe, Ni, Ta, Ti, Nb, Cr, Cu, Mo, and V via inductively coupled plasma-optical emission spectrometry (ICP-OES, Horiba Jobin Yvon JY 2000 series, Thermo Scientific).
C and O contents in the solid samples were determined by using Leco WC 230 and Leco RO400 analyzers, respectively.
2.2.3 Compositional and morphological characterization of solid samples
Physical–chemical characterization of the solid samples, before and after treatments, was performed by powder X-ray diffraction using a Rigaku Geigerflex D-Max diffractometer operating with a Cu tube at 30 kV and 30 mA (mineral identification was carried out by comparison with the JCPDS File 1985) (Library of Congress, 2023) and by scanning electron microscopy combined with energy dispersive spectroscopy using SEM/EDS JEOL JSM-7800F (Supplementary Figure S3) and JEOL JSM 5500LV (Supplementary Figure S5) equipment. C and O contents in the solid samples were determined by using Leco WC 230 and Leco RO400 analyzers, respectively.
2.2.4 Co powder
Co metal powders (0.020 g, 0.34 mmol) were reacted with 0.050 L of a 0.5 M aqueous leaching solution of the selected acids in an open flask under room conditions (approximately 20°C and 1 atm) and magnetic bar stirring (300 rpm). The solutions turned readily from colorless to bright pink, and the disappearance of Co powder occurred in a short time. The obtained cobalt complexes were isolated in the solid state from the leaching solution through the diffusion of acetone in the aqueous solution. The dried solids were subsequently characterized as in Sects. S1. Similar experiments using Co powder (0.160 g) and OA (aq) (0.5 M, 400 mL) were run into capped 1 L plastic bottles in the attrition mode (Heidolph REAX 20 rotating system, 5 rpm) with the view to highlight gas formation/consumption during the leaching process through a corresponding volume expansion/shrinkage of the container.
2.2.5 WC-Co test specimen
Co-leaching experiments on test specimens were carried out by reacting 500 mg of the sample with an aqueous solution of each of the respective acids (250 mL, 0.5M) in an open flask at room temperature under magnetic bar stirring (300 rpm). In each case, the leaching process was monitored over 1–12 h in 1 h increments. Co-recovery yields were determined incrementally based on the elemental analysis (ICP-OES) of the leachate aliquots after digestion (see Section 2.2.1).
2.2.6 Scale-up process
A 650 g aliquot of RC-631L was reacted with HLac(aq) (1 M, 7 L, L/S = 14 L/kg) at room temperature (20°C) and pressure in the presence of forced aeration in a 30 L mixing vessel. Stirring was provided by bubbling air from the bottom of the vessel through spherical, porous stones connected to an air compressor. The leaching reaction was monitored from 1 to 24 h by sampling the leachate and analyzing the digested aliquots for Co by ICP-OES. The Recovered WC-Co powder was, hence, characterized as described in sections 2.2.2 and 2.2.3.
2.2.7 Process demand of oxygen (respirometry)
The respirometer is a system typically used for the measurement of the biochemical oxygen demand (BOD) in water or the amount of oxygen that is consumed during the decomposition of organic matter in soils or composts. The system (Sapromat apparatus, H+P Labortechnik AG, Germany) consists of a 500 mL glass reaction bottle containing the sample connected to a sensor and an O2 generator. At the time when there is net oxygen consumption (i.e., in the absence of any other gas production due to the specific reaction involved), an electrode system detects the depression in the glass reaction bottle and supplies a parceled amount of oxygen. Flasks are placed in a thermostated bath at 20°C under magnetic bar stirring.
3 Results and discussion
3.1 Leaching experiments on Co powder
With the view to investigate the chemical reaction occurring between the selected lixiviants and cobalt in water, Co metal powders were reacted with an excess of diluted aqueous leaching solutions of the selected acids in an open flask under room conditions, as detailed in Section 2.3.1. Effective reactions took place in all cases under study, providing bright pink colored solutions, and the full disappearance of Co powder occurred in short periods (3–12 h). The leaching reactions occur by concerted oxidation and complexation of the metal in a single stage, forming the corresponding Co complex, which was isolated, in most cases, in the solid state by concentration and selective precipitation. The recovered compounds were, hence, characterized as detailed in Supplementary Material, Sect. S1, and most of them were recognized as already well known, even if typically reported in the literature as the products of complexing reactions.
Table 3 summarizes the Co-leaching reactions occurring with the different acids in water and the corresponding primary products (see Sects. S1 for characterization).
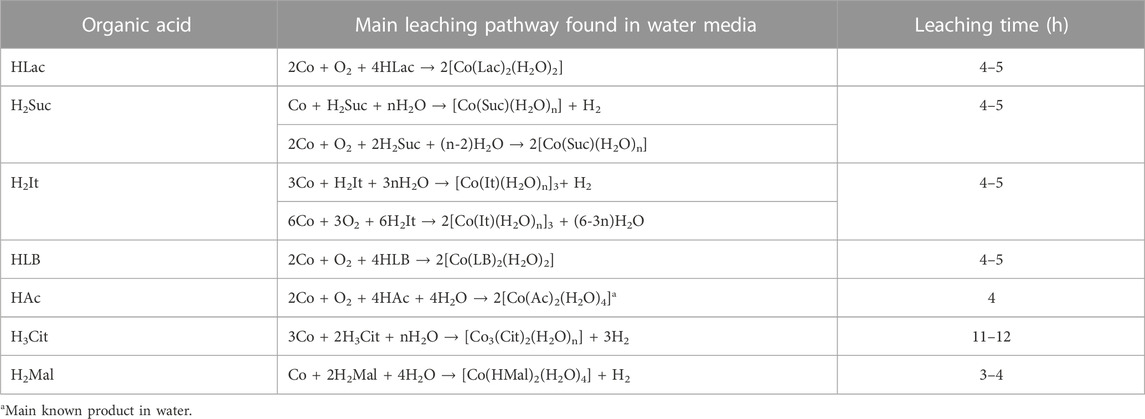
TABLE 3. Co-leaching reactions occurring with different acids in water. The leaching time refers to the complete disappearance of Co powder by leaching in a 0.5 M OA solution.
Among the different compounds isolated by the listed reactions, well-shaped crystals for [Co(It)(H2O)2]3 suitable for X-ray measurements provided the following described crystal structure.
Figure 1 summarizes the crystal structure and parameters of [Co(It)(H2O)2]3 detailed in Sect. S2, similar to those described in the study by Zhong-Yi et al. (2023).
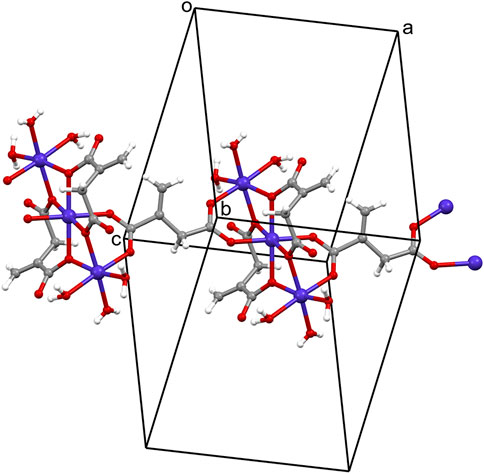
FIGURE 1. Molecular structure with a portion of crystal packing for the [Co(It)(H2O)2]3 complex. Crystal system: orthorhombic; space group: Pbca; a, b, and c (Å): 9.9809(13), 14.5548(14), and 15.3513(19); and α = β = γ (°): 90. Color legend: blue, Co; red, O; gray, C; and white, H. Disordered fragments were omitted for clarity.
Even if an extensive description of the crystal structure of the products overcomes the scope of this work, it is worth noting that all the compounds reported here are characterized as bearing Co(II) in an octahedral environment, where the anionic form of the acid coordinates the metal center as monodentate or bidentate, chelating and/or bridging ligand, and the coordination sphere is completed by water molecules according to the literature (Frutos et al., 1997; Zheng and Lin, 2000; Chandran et al., 2015; Wang et al., 2018). Specifically, the [Co(IT)(H2O)2]3 compound shows a peculiar trimeric structure, where two It2- anions behave as bidentate chelating ligands on the central Co atom and are bridged with the two peripheral Co atoms. Furthermore, two It2- anions act as monodentate on the central Co atom and behave as bridging ligands between two adjacent trimeric structures. The coordination sphere of the two peripheral Co atoms is completed by water molecules.
As summarized, leaching reactions, in addition to Co complexation, may involve the oxidizing action of the organic acid (through H+ reduction), which would result in H2 gas evolution and/or the oxidizing action of dissolved O2, which would instead be accompanied by O2 consumption and H2O formation. Specifically, using maleic, citric, succinic, and itaconic acids, leaching was accompanied by a noticeable H2 gas evolution. However, no gas formation was observed by leaching cobalt with lactic, lactobionic, and acetic acid solutions. These results were supported by running the reactions into capped plastic bottles in the attrition mode: where noticeable H2 evolution in an open flask under magnetic bar stirring was observed, a visible bottle expansion in the rotating closed system occurred; where there was no evidence of gas formation in the open flask, evident gas consumption in the closed vessel (visible bottle shrink) occurred. However, in the case of succinic and itaconic acid leaching, despite a noticeable gas evolution present in the open-flask reaction, no significant effects on the volume of the bottle were observed when performed in a closed environment. This evidence suggested that, in addition to H2 production, O2 consumption may occur at comparable rates.
Despite the occurrence of these two possible pathways, which can be considered obvious, identifying the parameters able to drive the system to preferentially follow one specific pathway over the other may give relevant hints to designing the leaching system appropriately for applicative purposes.
As for a more formal description, a prediction of the spontaneity of the redox reaction between the metal and the H+ as oxidant has been made by comparing the normal (or apparent) reduction potential of the two species involved, taking into account the competitive complexation and acid dissociation equilibria occurring in water. With specific reference to Co leaching, as detailed in Supplementary Material Sect. S3, we took into account that the ligands are involved in two different competing equilibria. Actually, complex formation equilibria such as Co + L ⇌ [CoL] (L = organic acid in deprotonated form) operate simultaneously with the protonation equilibrium H + L ⇌ HL. To keep into account both processes in the interpretation of the redox behavior of Co2+, we have, therefore, considered the following equilibrium:
The Nernst equation for the redox potential of the reduction Co2+ + 2 e- ⇌ Co,
can be, therefore, rearranged to obtain the apparent reduction potential for semi-reaction 1.
where
E°’’Co2+/Co differs from the expression of the typical E°’Co2+/Co (potentials corrected for the complex formation equilibria) (Serpe, 2018) for a term dependent on pKa. By evaluating the expression of E°’’Co2+/Co, it is clear that its value decreases (metallic Co becomes more oxidizable) with increasing stability of [CoL] species (higher Kf) and with increasing strength of the acid (decreasing pKa). The relative magnitude of these two parameters for an organic acid under examination contributes to defining the capacity of an organic ligand to form complexes with the cobalt ion. This, therefore, justifies the use of E°’’Co2+/Co as a better parameter than E°’Co2+/Co to evaluate the redox behavior of cobalt in our conditions.
The calculations of the speciation of the Co2+/ligand systems for the ligands examined (see Table 4) performed using the Hyss software (Alderighi et al., 1999) have shown that under the experimental conditions used in this work, the proton dissociation is also limited for polyprotic ligands to the first ionization equilibrium. In addition, the formation of complexes is limited to 1:1 metal:ligand species, even for acids that can form complexes with different stoichiometries (see Supplementary Table S2). These results justify the use of only pKa1 and β1 (hereafter β1 = Kf) as thermodynamic parameters.
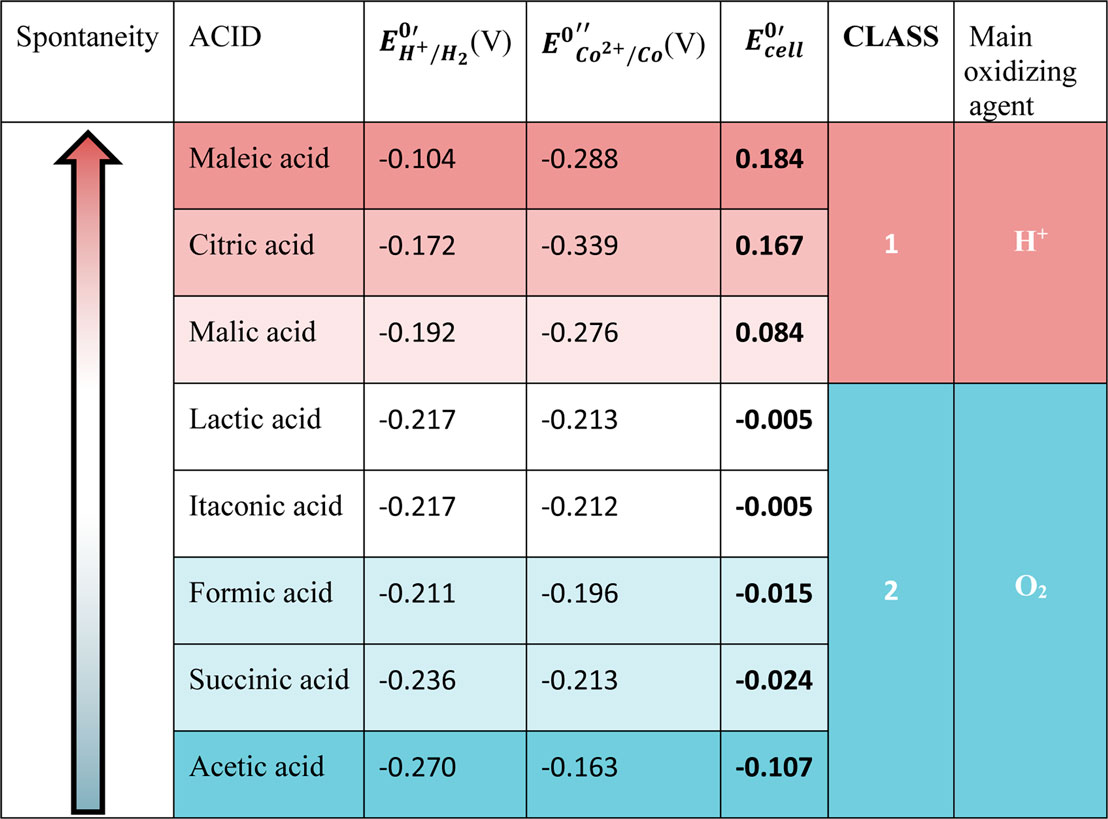
TABLE 4. E °’H+/H2, E °’’Co2+/Co, and E °’cell values. The pKa values used are those reported at 25°C (Smith et al., 2004). As for the ionic strength, the values reported for I = 0.1 M are used for homogeneity.
As for the reduction potential of H+, we took into account the protonation equilibria of the ligands, which led to the Nernst equation (see Supplementary Material, Sect. S3):
where
By evaluating the expression of the two standard normal reduction potentials 4 and 6, it is found that the process of oxidation of metallic cobalt by an organic acid pivots principally around the pKa value. Indeed, a low pKa value results in a higher H+ concentration and a lower HL concentration or possibly in the formation of [CoL] species, in turn making metallic cobalt easier to oxidize.
The combination of the two semi-reactions leads to the process
and to the related standard potential of the cell
Table 4 reports the E°’’Co2+/Co and E°’H+/H2 values calculated for the organic acids studied here and the corresponding calculated E°’cell. The E°’’Co2+/Co and E°’H+/H2 values are also reported in a graphic form in Figure 2. Those acids (points) that are in the bottom-right region of the plot have a significant tendency to oxidize metallic cobalt with the formation of H2, while those in the upper-left region are not prone to this process, and the presence of a stronger oxidant such as dioxygen is required. Acids that are on or close to the dotted line are those for which the process is not significantly favored on a thermodynamic basis, but it can occur if the actual concentrations of the components in the system allow it. Just as an example, in the presence of solely metallic cobalt, the process may occur and then rapidly stop because the redox cell potential reaches zero. Our calculations of the normal (or apparent) reduction potential under standard conditions E°’’Co2+/Co open a window on the rationalization of the relative occurrence of the observed redox reactions. As pointed out, their occurrence is related to the relative reduction potential of the interacting species (ECo2+/Co vs EH+/H2 and EO2/H2O) and the relative concentration of the oxidizing species in solution. Indeed, on the one hand, the stability of the formed metal complex lowers the reduction potential of the metal, making it easier to be oxidized, as well as the reduction potential of the H+/H2 couple when weak acids (high pKa1 values) are involved. On the other hand, despite the higher reduction potential of O2 with respect to H+, the low solubility and diffusion rate of gaseous O2 in water solution make it a low competitive oxidizing agent. For this reason, H+ oxidation is reasonably the main reaction toward low-potential elements in the case of strong acids (low pKa1) able to provide highly acidic solutions and/or when highly coordinative polydentate anionic species are formed by metal ion complexation, liberating low-acidic H+ ions in the solution. Based on the experimental evidence well supported by the described more formal studies, the classification of metal lixiviants into class 1 and 2 based on weak OAs is proposed in Table 4 depending on the thermodynamic spontaneity of the metal oxidation reaction by H+. OAs providing E cell only slightly negative for the described process may combine the two reactions, even more so in non-standard conditions.
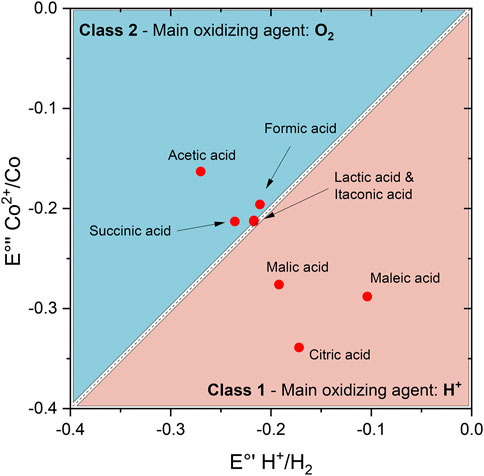
FIGURE 2. Plot of the E°’H+/H2 and E°’’Co2+/Co values calculated for the organic acids studied. Acids that refer to points in the bottom-right region are those expected to oxidize metallic cobalt.
3.2 Leaching experiments on test specimens of HM powders
Leaching experiments on real WC-Co powders were carried out in the same conditions applied to Co metal powder. Specifically, 250 mL of 0.5 M aqueous solutions of H2Mal and H3Cit class 1 OAs, as well as HLac, H2Suc, H2It, HLB and HAc class 2 OAs, were reacted in an open flask with 0.500 g aliquots of RC-627C and RC-631L test specimens at room temperature under magnetic bar stirring. Figure 3 shows the Co-leaching profiles in terms of yield of leached cobalt (wt. %) vs. time (h), obtained by monitoring the amount of cobalt in the leachates of the test specimens in time (1 h steps). The Co amount in the leachate was determined by ICP-OES measurements on digested measured aliquots of the solution, as detailed in Sect. S1.1.
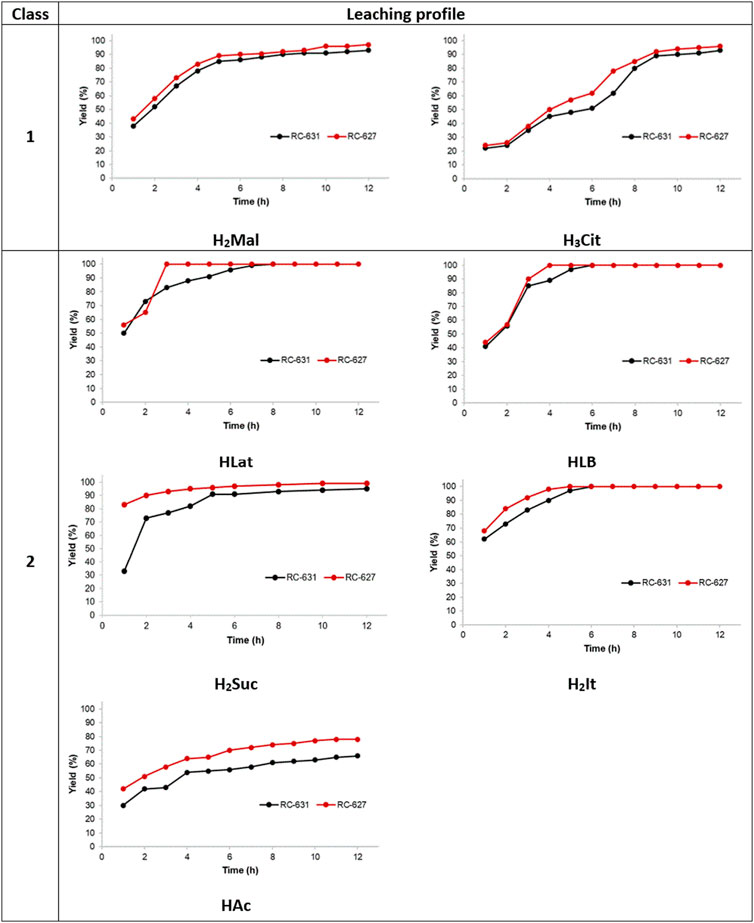
FIGURE 3. Co-leaching profiles from RC-627C and RC-631L recovery powders (0.5 g) on varying the leaching agent and times. Experimental conditions: [Acid] = 0.5 M; r.T. and pressure; and stirring. Results are reported as the average values of two different experiments.
As shown and expected under these experimental conditions, all the used acids demonstrated to be effective in cobalt dissolution from RC-627C, achieving in most cases an almost complete Co dissolution in short times, specifically 3 h with HLac, 4 h with HLB, H2Suc, and H2It, and 10 h with H2Mal and H3Cit solutions, respectively. In the reported conditions, HAc demonstrated that it provided the less-effective leaching solution, achieving around 90% Co dissolution on RC-627C in 12 h. Co leaching on RC-631L was demonstrated to be slightly less efficient than observed on RC-627C but still satisfactory, obtaining over 90% Co dissolution in 5 h with HLac, HLB, and H2It, 7 h with H2Suc, 8 h with H2Mal, and 9 h with H3Cit solutions, respectively, while the HAc solution dissolves around 65% of cobalt in 12 h. This difference can be reasonably attributed to the lower values of the WC particles’ mean grain size and the metallic binder mean free path of the RC-631L test specimen, as found in previous studies on solvometallurgical processes on the same samples (Oumarou Amadou et al., 2021). Additional characterization was performed on the solid residues after leaching to point out the stage of Co-dissolution and the state of the remaining W-based phases. In particular, powder X-ray diffraction (p-XRD) and SEM/EDS analysis were performed on the RC-631L sample before and after leaching with several of the systems under study. These techniques, despite having a lower sensitivity with respect to the chemical analysis, demonstrated themselves to be very diagnostic and fast tools for monitoring the reactions, being able to point out the progress of the Co leaching through the lowering of Co peaks without requiring any additional sample treatment for analysis. Furthermore, p-XRD is also diagnostic of the chemical form of the species.
Figure 4 and Supplementary Figure S3 show, respectively, the p-XRD and SEM-EDS patterns recorded for the RC-631 L sample before and after leaching representatively with solutions of HLac and H2Suc for 4 and 6 h under the previously reported conditions. The XRD patterns of the sample before (Figure 4A) and after leaching (Figure 4B and Figure 4C) clearly show the disappearance of Co metal peaks after treatment, highlighting the highly efficient dissolution of cobalt in HLac and H2Suc solutions after 4 and 6 h of leaching, respectively. Furthermore, the patterns collected after treatment showed no changes in the other parts of the spectrum in terms of the disappearance of existing species or the appearance of new peaks related to newly formed species. This evidence demonstrates the capability of the solutions of these acids to dissolve Co selectively, leaving W and WC unreacted. In agreement, SEM-EDS characterization highlights an almost complete Co dissolution, preserving both W and C presence.
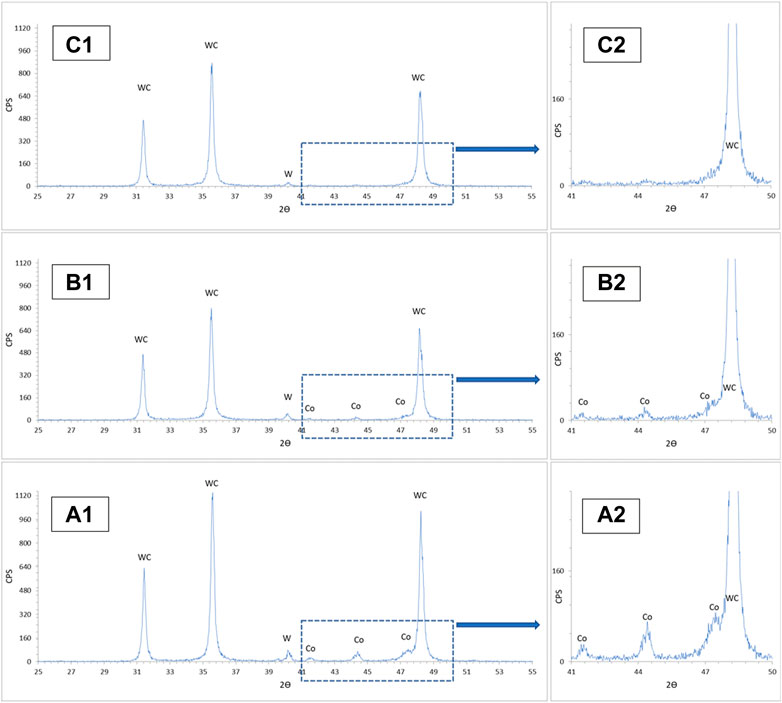
FIGURE 4. P-XRD patterns of (A1) RC-631L before Co leaching; (B1) RC-631L after 6 h of leaching with H2Suc; (C1) RC-631L after 4 h of leaching with HLac, and corresponding magnification of the Co-peaks range (A2, B2, C2). Peak attribution: W metal (JCPDS card 04–0806); WC (JCPDS cards 65–8828 and 51–0939); and Co metal (JCPDS card 5–0727).
These experiments demonstrate that the proposed method can tune the percentage of Co in the treated powders by tailoring appropriately the leaching time based on the leaching profiles meeting the compositional requirements of the industrial hard-metal manufacturing process. P-XRD and/or SEM-EDS characterization of the solid residue demonstrated an appealing and expeditious tool for the monitoring of the process evolution, even if a chemical analysis by ICP-OES is required to quantitatively determine the final sample composition.
3.3 HLac case study: setup of operative conditions
3.3.1 Liquid/solid ratio
With the view of meeting industrial requirements for technological transfer and improving the sustainability of the process, experiments limiting the amount of the leaching agent and solvent were performed on solutions of the most promising bio-derived acid, namely HLac. Specifically, attempts to fix the Co: acid molar ratio to lower values than the previous 1:74 (0.5 g of test specimen, approximately 20 wt. % Co; [Acid] = 0.5 M; volume = 0.250 L), but providing the cobalt with a calculated excess of lixiviant and the acid concentration to a still low 1 M, were performed. The experiments were first carried out on 0.375 g of Co powder with a 1 M solution at room temperature under magnetic bar stirring, monitoring the time of complete disappearance of Co powder from the reaction vessel. Table 5 summarizes the Co-leaching time and experimental conditions applied to Co-powder.
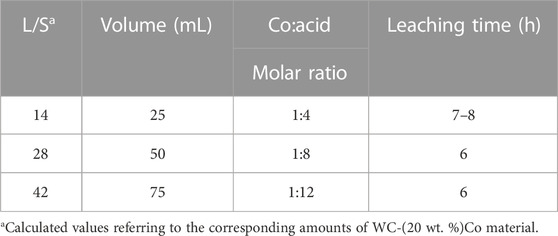
TABLE 5. Complete Co-leaching time of 0.375 g Co powder in an open flask by varying the Co:acid molar ratio under magnetic bar stirring. [HLac] = 1 M.
As shown, keeping all other conditions fixed, as the excess of reagent increases, the leaching times decrease. Co:acid 1:8 molar ratio seemed to guarantee the minimum excess of acid that allowed the leaching to be almost quantitative in a short time. A further increase to 1:12 does not show a significant time reduction.
On these bases, 18.8 g of the RC-631L sample was treated in an open vessel with 250 and 500 mL of HLac 1 M at room temperature under mechanical stirring. Figure 5 shows the Co-leaching profiles of the sample under the reported conditions (detailed Co-leaching yields are reported in Sect. S5, Supplementary Table S3).
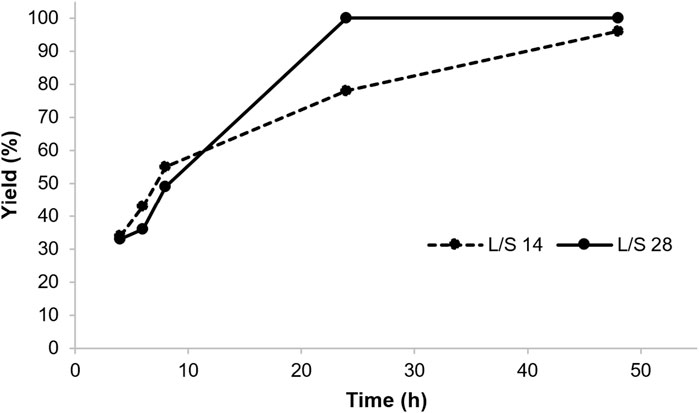
FIGURE 5. Co-leaching profiles on the RC-631L sample shown as Co-dissolution yield (%) vs. time (h) by varying the L/S ratio (14 and 28 L/kg) under mechanical stirring at room conditions.
As shown and expected based on the previous results, a 28 L/kg ratio, corresponding to a 1:8 Co:HLac molar ratio, allows a complete Co dissolution in a shorter time (within 24 h), while longer times (at least 48 h) are required when a 14 L/kg ratio, corresponding to a 1:4 molar ratio, is applied. Nevertheless, if an industrial point of view is considered, using a more favorable 14 L/S ratio may represent the best compromise for achieving appropriately tuned composition materials. Indeed, under the reported conditions, a recovered powder with a WC-(5%)Co composition can be achieved in 24 h. It is worth noting that the leaching times in these experiments were found to be longer than those found on Co powder under the same conditions. Such an increase in the leaching time with the amount of starting material (0.375 g of Co powder vs. 3.75 g of Co in 18.8 g RC-631L) supported the hypothesis of a key role of dissolved oxygen in the reaction rate.
3.3.2 Studying the oxygenation effect on Co leaching by aqueous HLac solutions
To make the leaching process as efficient as possible, tests were carried out to identify the most kinetically favored reaction, specifically by the following trials:
Trial 1: Determination of the H2 produced by gas chromatography (GC) measurement in the absence (a) and presence (b) of oxygen;
Trial 2: Effect of forced oxygenation on leaching times of metallic cobalt by blowing air into the reaction environment.
The tests were carried out using a commercial HLac aqueous solution at room temperature and under magnetic stirring. In test a of Trial 1, the leaching solution was previously de-aerated using a flow of gaseous N2. In test b, the reaction was allowed to proceed in the presence of dissolved oxygen and the oxygen present in the head space. During test a, conducted in the absence of oxygen, a quantity of H2 is produced that is more than 60% higher than that produced during test b, conducted in the presence of O2. This indicates that in limiting conditions, characterized by little or no presence of oxygen, the reaction with the production of hydrogen is favored. It also indicates that oxygen has some influence on the reaction. This influence was investigated in trial 2, in which the time associated with the quantitative leaching of the metal cobalt powder in the absence and presence of forced oxygenation and maintaining the same operating conditions as in trial 1 were compared (Table 6). The test in natural oxygenation conditions was carried out in the absence of air blowing; the forced oxygenation reaction was carried out by bubbling pressurized air through a thin tube into the reaction medium. The complete disappearance of the Co powder indicates the end of the reaction.

TABLE 6. Effect of oxygenation (forced aeration by blowing air through a thin glass tube) on the metallic cobalt leaching time.
As shown, significantly shorter times were found for Co-leaching experiments performed through forced aeration, confirming the relevant effect of O2 on the reaction.
On these bases, additional experiments addressed to point out the entity and kind of interaction of oxygen with the components of the real WC-Co powder were carried out on Co, W, and WC powders using a Sapromat respirometer, as detailed in Section 2.3.4.
Figure 6 shows the profiles of oxygen consumption vs. leaching time determined by respirometry of the O2-dependent HLac leaching reactions.
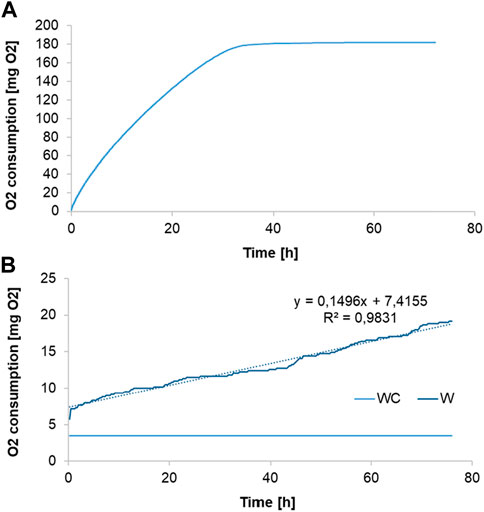
FIGURE 6. Leaching reaction with 0.1 L of HLac 1 M under Sapromat respirometer conditions: oxygen consumption curve of (A) Co metal (0.714 g); (B) WC and W powders (2.86 g).
As shown, cobalt powder consumes oxygen during the leaching reaction. The monitoring of oxygen consumption by Co allows estimating the end of the reaction, represented by the achievement of the plateau of the curve in Figure 6A (HLac solution does not consume oxygen). Despite the longer leaching times (approximately 30 h) found using this method,2 it points out some relevant aspects of the leaching reaction. Specifically, the amount of oxygen consumption (mg O2) during the reaction almost reflected the stoichiometric need: in the face of 193 mg O2 theoretically required by the reaction (0.27 g O2/g Co), 180 mg of O2 were consumed during the experiment, confirming the primary role of O2 in the leaching reaction. Experiments carried out at various pH values, obtained by adding a controlled amount of NaOH(aq) to the leaching solution, pointed out that at pH above 4.5, the formation of abundant precipitations (oxides and hydroxides of Co(II) and (III)) occurred, in agreement with the study by Edtmaier et al. (2005). pH < 4.5 prevented the formation of oxides and hydroxides, allowing the reaction to occur efficiently toward [Co(Lac)2(H2O)2] complex formation. This is a key point because the formation of insoluble by-products is undesirable in selective leaching processes. Moreover, as shown in Figure 6B, tungsten does not consume oxygen while tungsten carbide consumes a small and linearly increasing amount of O2, reaching a 12.5 mg O2 value (0.145 mg O2/g WC h) after 30 h (the average leaching time found for cobalt powder). This finding, which should be taken into account when assessing global O2 consumption during the process on WC-Co real material, confirms the knowledge of slow but occurring oxidative phenomena involving WC.
Finally, further leaching tests were performed for defining the best oxygenation system. Jar test reactions were carried out on metallic cobalt powder (1.47 g Co, 1 M HLac 0.2 L, r.T, mechanical stirring) with air blowing through the solutions by a thin glass tube providing large bubbles and, for comparison, a porous stone providing fine bubbles, both powered by a small air compressor. Table 7 summarizes conditions and leaching yields for the two systems after 4 h. Each experiment was performed twice.
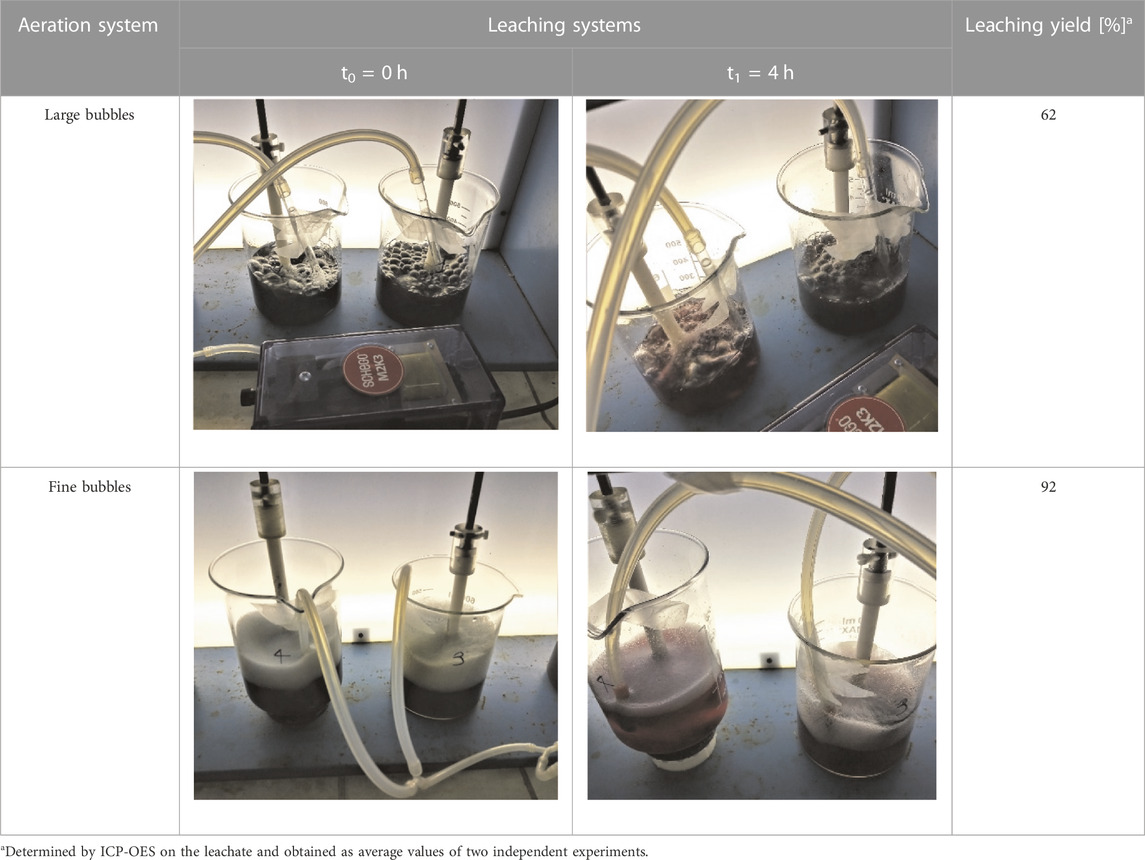
TABLE 7. Cobalt (1.47 g) leaching efficiency with HLac (1 M, 0.2 L) under large and fine bubble aeration.
As shown and expected, the fine bubble system is significantly more efficient and achieves almost complete leaching of Co in the observation time. These results were also confirmed by increasing the scale of the experiment, obtaining an almost quantitative Co dissolution in 4–5 h by treating 2.94 g and 5.89 g of Co powder with 0.4 and 0.8 L of HLac 1 M, respectively. This result suggests that the fine bubble diffuser provides available oxygen at a rate compatible with its consumption by the reaction.
3.3.3 pH effect on the leaching reaction
In addition to oxygenation, the leaching reaction is influenced by pH as it affects the Co and O2 reduction potential and the species produced by the reaction. For this reason, an experiment was conducted to compare the leaching behavior of metallic Co powder at different pH values. The experiments were carried out using a Co:HLac molar ratio of 1:8, and it was observed that
• the leaching reaction does not take place in a strongly alkaline environment
• at weakly acidic pH values (>4.5), the leaching reaction is accompanied by the formation of abundant precipitates (Co(II) and (III) oxides and hydroxides), as known from the study by Badawy et al. (2000)
• at pH ≤ 4, the reaction takes place without the formation of poorly soluble by-products.
Based on these, leaching cobalt for HM in the presence of fine bubble oxygenation and an acid environment with a pH ≤ 4 were selected as the optimal conditions for applicative purposes.
3.4 Scale-up of the leaching process by HLac on WC-Co residues
A leaching experiment on 650 g of RC-631L powder in the presence of forced aeration was performed with an HLac solution (1 M, 7 L, L/S = 14 for limiting the amount of solution) into a 30 L mixing vessel. Stirring was provided by bubbling air from the bottom of the vessel through spherical, porous stones connected to an air compressor. The leaching reaction was monitored for 24 h by sampling the leachate and analyzing the cobalt content of the digested aliquots by ICP-OES, as detailed in Section 2.2.3. The Co-leaching setup and profile of this experiment are shown in Figure 7. Leaching yields vs. time data are provided in Supplementary Table S4.
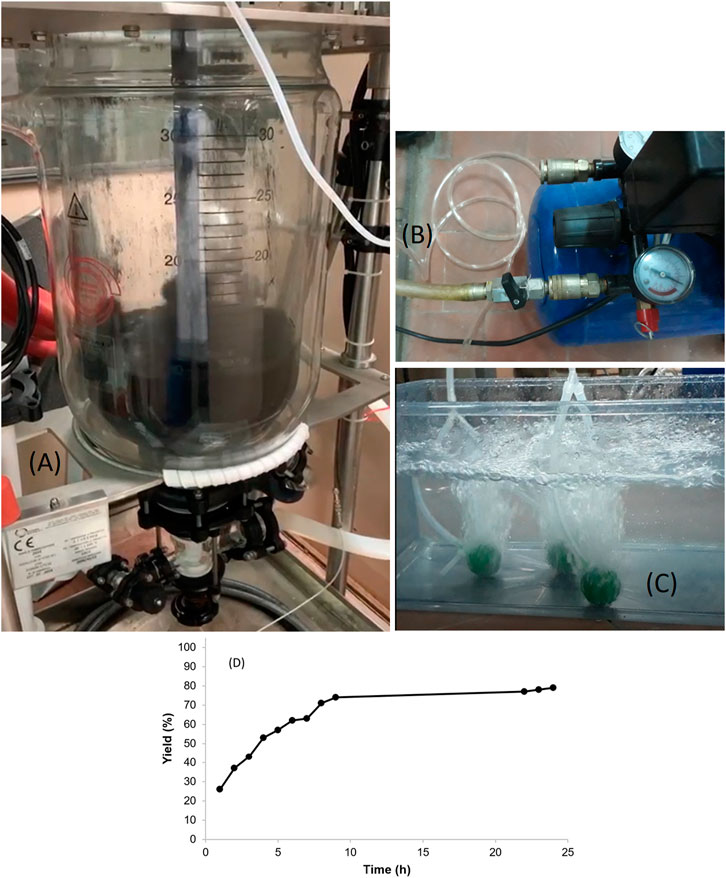
FIGURE 7. Co-leaching scale-up experiment on RC-631L powder (650 g) with HLac (1 M, 7 L) under aerated conditions. Thermostated double-wall 30 L mixing vessel equipped with a motovariator for mechanical stirring, pH control, and distillation line (A); forced aeration setup of the experiment based on the use of an air compressor (B); a four-way aerating system generating fine bubbles through spherical porous stones (C); and Co-leaching profile (D) (see Supplementary Table S4 for data).
Under the aforementioned conditions, approximately 80% yield of Co dissolution was achieved in 24 h. These data fully agreed with the corresponding ICP-OES characterization of the digested solid sample, where a WC-(5.7%) Co composition was found, as detailed in Sect. 2.2.2. Furthermore, these results were supported by XRD and SEM/EDS solid-state characterization of the recovered powder, which highlighted the lowering of cobalt peaks and a residual amount of cobalt in the final mixture (see Supplementary Figures S4, S5).
3.4.1 Quality assessment of the WC-Co recovered powder
C and O content in the WC-Co powders suitable to be directly employed in HM manufacturing is a critical parameter for obtaining HM tools with performing properties. Indeed, it is well known that the co-presence of C and O in a WC-based powder lowers the C content of the mixture during the sintering phase due to COx formation, affecting the final properties of the HM material due to the occurrence of the so-called η-phase in the final material (García et al., 2019). C and O elemental analyses were, hence, performed as detailed in Sect. S1.2 on the solid residue of the leaching experiment (Section 3.4), with the view of checking the quality of the recovered material for applicative purposes. The measured C and O compositions—6.24 and 1.60 wt. %, respectively—were found to be higher than the optimal 5.70 and ≤0.40 wt. % values. The most critical aspect is the significantly high amount of oxygen typically found in WC-Co powders treated in water, where hydration phenomena of the WC surface may occur along with the oxidative phenomena pointed out through respirometry experiments. Moreover, the retention of unwashed organic leaching agents/complexes may occur, affecting C–O elemental analysis. Thus, a thermal treatment under an inert environment (1,000°C, 2 h, N2) was attempted for improving the quality of the powder. The thermal treatment was demonstrated to be able to drive powder composition within the desired ranges, as the new material showed C 5.65 wt. % and 0.19 wt. % compositions, and to make it suitable for HM production.
4 Conclusion
A selection of OAs were tested as leaching agents for Co metal, both in the form of metal powder and in a composite material like WC-based cemented carbide where it plays the role of the metallic binder. All the selected OAs, namely, H2Mal, H3Cit, HLat, H2Suc, H2It, HLB, and HAc, demonstrated to work well, achieving quite fast and selective, almost quantitative, Co dissolution in an aqueous solution at low concentration levels and room conditions. Furthermore, a relevant role of oxygen in the leaching reaction with several of these acids was found, highlighting different capabilities among the selected OAs to oxidize the metal. Experimental evidence and computational calculations of the normal reduction potentials of cobalt and H+, taking into account the Ka1 of the acid and the Kf of the corresponding Co complex, agreed in identifying two classes of leaching agents: Class 1, which consists of OAs where H+ is thermodynamically favored to work as an oxidant toward Co; class 2, which instead consists of OAs where the metal oxidation is mainly carried out by external oxidants, e.g., dissolved O2, the oxidation through H+ being thermodynamically unfavored. Due to the high appeal related to the use of bio-derived, eco-friendly OAs in hydrometallurgy, these insights are crucial in the selection of the most appropriate OA for the specific application and in designing the leaching process. Furthermore, this model is, in principle, applicable to all metal–OA combinations, making it a key tool for OA leaching behavior prediction. Herein, the application of OA leaching solutions to Co-containing hard-metal powders was used as a successful case study that addressed the industrial recovery of critical raw materials. Among the selected acids, particular attention was devoted to the use of HLac in hydrometallurgy because of its effectiveness, low cost, sustainability, and large availability through biotechnological fermentative processes. A systematic study of the process conditions was carried out with satisfactory results, showing the capability of the leaching solution to provide, in mild conditions, good-quality recovered products suitable for re-employment in HM manufacturing.
Data availability statement
The datasets presented in this study can be found in online repositories. The names of the repository/repositories and accession number(s) can be found in the article/Supplementary Material.
Author contributions
AO, ST, and MC contributed to the experimental activity and writing. AS contributed to the conception, design, and writing of the study. GPD, ASM, and GDG tutored part of the experimental activities. LM and SC contributed to the XRD structural characterization of chemical compounds. MT performed modeling and calculations. GDG and AM contributed to writing sections of the manuscript. All authors contributed to the article and approved the submitted version.
Acknowledgments
The authors thank the Italian Ministry of University and Research (Dottorati innovativi con caratterizzazione industriale, Azione I.1 (AO’s scholarship); Dottorati su tematiche green, Azione IV.5 (MC’s scholarship)–PON R&I 2014–2020, N. DOT1304527) and ERASMUS+ Placedoc 2018–2019 (AO’s mobility) and 2021–22 action (MC’s mobility); the Italian Ministry for the Environment, Land, and Sea Protection (CoW_aste project, 2019); Fondazione di Sardegna (“Precious metal-free complexes for catalytic CO2 reduction” project, 2017); and the FILMS corp. (“The selective extraction of excess cobalt from the recovery powders of the “hard metal” production process” project, 2018; “The selective extraction of excess cobalt from the powders produced by carbothermal regeneration of “hard metal” scrap” project, 2019).
Conflict of interest
The authors declare that the research was conducted in the absence of any commercial or financial relationships that could be construed as a potential conflict of interest.
Publisher’s note
All claims expressed in this article are solely those of the authors and do not necessarily represent those of their affiliated organizations, or those of the publisher, the editors, and the reviewers. Any product that may be evaluated in this article, or claim that may be made by its manufacturer, is not guaranteed or endorsed by the publisher.
Supplementary material
The Supplementary Material for this article can be found online at: https://www.frontiersin.org/articles/10.3389/fenvc.2023.1216245/full#supplementary-material
Footnotes
1Rare earth elements (REEs), as defined by the International Union of Pure and Applied Chemistry, involve lanthanoides together with scandium and yttrium. Despite their name, their abundance in Earth’s crust is relatively high.
2Sapromat respirometer works in a “static” mode, suppling parceled amounts of oxygen in the head space of the closed reactor. This delivering mode does not favor, from a kinetical point of view, the oxygen availability for the leaching reaction, resulting in longer leaching times with respect to those recorded even just in an open flask.
References
Ackerman, L. K. G., Anka-Lufford, L. L., Naodovic, M., and Weix, D. J. (2015). Cobalt Co-catalysis for cross-electrophile coupling: Diarylmethanes from benzyl mesylates and aryl halides. Chem. Sci. 6 (2), 1115–1119. doi:10.1039/C4SC03106G
Alderighi, L., Gans, P., Ienco, A., Peters, D., Sabatini, A., and Vacca, A. (1999). Hyperquad simulation and speciation (HySS): A utility program for the investigation of equilibria involving soluble and partially soluble species. Coord. Chem. Rev. 184 (1), 311–318. doi:10.1016/S0010-8545(98)00260-4
Alonso, S., Rendueles, M., and Díaz, M. (2013). Bio-production of lactobionic acid: current status, applications and future prospects. Biotechnol. Adv. 31 (8), 1275–1291. doi:10.1016/j.biotechadv.2013.04.010
Badawy, W. A., Al-Kharafi, F. M., and Al-Ajmi, J. R. (2000). Electrochemical behaviour of cobalt in aqueous solutions of different PH. J. Appl. Electrochem. 30 (6), 693–704. doi:10.1023/A:1003893122201
Barbosa, L. P., Takiishi, H., and Faria, R. N. (2004). The effect of cobalt content on the microstructure of Pr–Fe–Co–B–Nb alloys and magnetic properties of HDDR magnets. J. Magn. Magn. Mat. 268 (1–2), 132–139. doi:10.1016/S0304-8853(03)00487-6
Burckhard, S. R., Schwab, A. P., and Banks, M. K. (1995). The effects of organic acids on the leaching of heavy metals from mine tailings. J. Hazard. Mat. 41 (2), 135–145. doi:10.1016/0304-3894(94)00104-O
Cera, M., Trudu, S., Oumarou Amadou, A., Asunis, F., Farru, G., De Gaudenzi, G. P., et al. (2023). Trends and perspectives in the use of organic acids for critical metal recycling from hard-metal scraps. Int. J. Refract. Met. Hard Mat. 114, 106249. doi:10.1016/j.ijrmhm.2023.106249
Chandran, S., Jagan, R., Paulraj, R., and Ramasamy, P. (2015). Spectral, mechanical, thermal, optical and solid state parameters, of metal-organic bis(hydrogenmaleate)-CO(II) tetrahydrate crystal. J. Solid State Chem. 230, 135–142. doi:10.1016/j.jssc.2015.06.035
Chen, X., Guo, C., Ma, H., Li, J., Zhou, T., Cao, L., et al. (2018). Organic reductants based leaching: A sustainable process for the recovery of valuable metals from spent lithium ion batteries. Waste Manag. 75, 459–468. doi:10.1016/j.wasman.2018.01.021
Directorate-General for Internal Market, I., Bobba, S., Claudiu, P., Huygens, D., Alves Dias, P., Gawlik, B., et al. (2018). Report on critical raw materials and the circular economy. LU: Publications Office of the European Union.
Edtmaier, C., Schiesser, R., Meissl, C., Schubert, W. D., Bock, A., Schoen, A., et al. (2005). Selective removal of the cobalt binder in WC/Co based hardmetal scraps by acetic acid leaching. Hydrometallurgy 76 (1), 63–71. doi:10.1016/j.hydromet.2004.09.002
Frutos, A. A., Escandar, G. M., Salas Peregrin, J. M., Gonzalez Sierra, M., and Sala, L. F. (1997). Complex Formation between D-lactobionate and bivalent metal ions. Studies in solution and in the solid state. Can. J. Chem. 75 (4), 405–413. doi:10.1139/v97-046
García, J., Collado Ciprés, V., Blomqvist, A., and Kaplan, B. (2019). Cemented carbide microstructures: A review. Int. J. Refract. Met. Hard Mat. 80, 40–68. doi:10.1016/j.ijrmhm.2018.12.004
Gergoric, M., Ravaux, C., Steenari, B.-M., Espegren, F., and Retegan, T. (2018). Leaching and recovery of rare-earth elements from neodymium magnet waste using organic acids. Metals 8 (9), 721. doi:10.3390/met8090721
Golmohammadzadeh, R., Faraji, F., and Rashchi, F. (2018). Recovery of lithium and cobalt from spent lithium ion batteries (LIBs) using organic acids as leaching reagents: A review. Resour. Conserv. Recycl. 136, 418–435. doi:10.1016/j.resconrec.2018.04.024
Konyashin, I., and Ries, B. (2022b). “Chapter 13 - recycling cemented carbides,” in Cemented carbides. Editors I. Konyashin, and B. Ries (Amsterdam, Netherlands: Elsevier), 313–325. doi:10.1016/B978-0-12-822820-3.00013-6
Konyashin, I., and Ries, B. (2022a). “Chapter 5 - structure and properties of binder phases in cemented carbides,” in Cemented carbides. Editors I. Konyashin, and B. Ries (Amsterdam, Netherlands: Elsevier), 51–63. doi:10.1016/B978-0-12-822820-3.00005-7
Li, L., Dunn, J. B., Zhang, X. X., Gaines, L., Chen, R. J., Wu, F., et al. (2013). Recovery of metals from spent lithium-ion batteries with organic acids as leaching reagents and environmental assessment. J. Power Sources 233, 180–189. doi:10.1016/j.jpowsour.2012.12.089
Library of Congress (2023). JCPDS--International Centre for diffraction data - LC linked data service: Authorities and vocabularies | library of congress, from LC linked data service: Authorities and vocabularies. Virginia: Library of Congress.
Meshram, P., Bhagat, L., Prakash, U., Pandey, B. D., and Abhilash, B. (2017). Organic acid leaching of base metals from copper granulated slag and evaluation of mechanism. Can. Metall. Q. 56 (2), 168–178. doi:10.1080/00084433.2017.1293900
Musariri, B., Akdogan, G., Dorfling, C., and Bradshaw, S. (2019). Evaluating organic acids as alternative leaching reagents for metal recovery from lithium ion batteries. Min. Eng. 137, 108–117. doi:10.1016/j.mineng.2019.03.027
Ortner, H. M., Ettmayer, P., Kolaska, H., and Smid, I. (2015). The history of the technological progress of hardmetals. Int. J. Refract. Met. Hard Mat. 49, 3–8. doi:10.1016/j.ijrmhm.2014.04.016
Oumarou Amadou, A., Cera, M., Trudu, S., Piredda, M., Cara, S., Gaudenzi, G. P. D., et al. (2023). A comparison among bio-derived acids as selective eco-friendly leaching agents for cobalt: The case study of hard metals waste enhancement. ChemRxiv April. 28, 2023. doi:10.26434/chemrxiv-2023-9l4mp-v2
Oumarou Amadou, A., De Gaudenzi, G. P., Marcheselli, G., Cara, S., Piredda, M., Spiga, D., et al. (2021). A new facile solvometallurgical leaching method for the selective Co dissolution & recovery from hard metals waste. Int. J. Refract. Met. Hard Mat. 98, 105534. doi:10.1016/j.ijrmhm.2021.105534
Seo, B., and Kim, S. (2016). Cobalt extraction from tungsten carbide-cobalt (WC-Co) hard metal scraps using malic acid. Int. J. Min. Process. 151, 1–7. doi:10.1016/j.minpro.2016.04.002
Serpe, A. (2018). “Green chemistry for precious metals recovery from WEEE,” in Waste electrical and electronic equipment recycling. Editors Francesco Vegliò, and I. Birloaga (Amsterdam, Netherlands: Elsevier Ltd), 271–332.
Shemi, A., Magumise, A., Ndlovu, S., and Sacks, N. (2018). Recycling of tungsten carbide scrap metal: A review of recycling methods and future prospects. Min. Eng. 122, 195–205. doi:10.1016/j.mineng.2018.03.036
Smith, R. M., Martell, A. E., and Motekaitis, R. J. (2004). NIST critically selected stability constants of metal complexes database, Version 8.0. Editor Standard Reference Data Program (National Institute of Standards and Technology (U.S.)) (Gaithersburg, MD: NIST standard reference database; Standard Reference Data Program, National Institute of Standards and Technology, U.S. Dept. of Commerce).
Srivastava, R. R., Lee, J., Bae, M., and Kumar, V. (2019). Reclamation of tungsten from carbide scraps and spent materials. J. Mat. Sci. 54 (1), 83–107. doi:10.1007/s10853-018-2876-1
Tang, Y., Liu, Z., Guo, W., Chen, T., Qiao, Y., Mu, S., et al. (2016). Honeycomb-like mesoporous cobalt nickel phosphate nanospheres as novel materials for high performance supercapacitor. Electrochimica Acta 190, 118–125. doi:10.1016/j.electacta.2016.01.042
The Royal Society of Chemistry (2013). in Element recovery and sustainability. Editor A. Hunt (London, United Kingdom: The Royal Society of Chemistry). doi:10.1039/9781849737340
U.S. Geological Survey (2022). “Mineral commodity summaries 2022,” in Mineral commodity summaries; USGS numbered series 2022 (Reston, VA: U.S. Geological Survey), 2022, 202. doi:10.3133/mcs2022
Wang, L., Xu, N., Pan, X., He, Y., Wang, X., and Su, W. (2018). Cobalt lactate complex as a hole cocatalyst for significantly enhanced photocatalytic H2 production activity over CdS nanorods. Catal. Sci. Technol. 8 (6), 1599–1605. doi:10.1039/C8CY00067K
Zeiler, B., Bartl, A., and Schubert, W.-D. (2021). Recycling of tungsten: Current share, economic limitations, technologies and future potential. Int. J. Refract. Met. Hard Mat. 98, 105546. doi:10.1016/j.ijrmhm.2021.105546
Zheng, Y.-Q., and Lin, J.-L. (2000). Crystal structure of catena-tetraaquasuccinato-O,O’-cobalt(II), CO(C4H4O4)(H2O)4. Z. Für Krist. - New Cryst. Struct. 215 (1), 159–160. doi:10.1515/ncrs-2000-0180
Keywords: critical raw materials, organic acids, leaching, recycling, green processes, coordination chemistry, apparent reduction potential
Citation: Oumarou Amadou A, Cera M, Trudu S, Piredda M, Cara S, De Gaudenzi GP, Matharu AS, Marchiò L, Tegoni M, Muntoni A, De Gioannis G and Serpe A (2023) A comparison among bio-derived acids as selective eco-friendly leaching agents for cobalt: the case study of hard-metal waste enhancement. Front. Environ. Chem. 4:1216245. doi: 10.3389/fenvc.2023.1216245
Received: 03 May 2023; Accepted: 02 June 2023;
Published: 22 June 2023.
Edited by:
Elza Bontempi, University of Brescia, ItalyReviewed by:
Jiawei Wen, China University of Petroleum, ChinaChiara Ferrara, University of Milano-Bicocca, Italy
Copyright © 2023 Oumarou Amadou, Cera, Trudu, Piredda, Cara, De Gaudenzi, Matharu, Marchiò, Tegoni, Muntoni, De Gioannis and Serpe. This is an open-access article distributed under the terms of the Creative Commons Attribution License (CC BY). The use, distribution or reproduction in other forums is permitted, provided the original author(s) and the copyright owner(s) are credited and that the original publication in this journal is cited, in accordance with accepted academic practice. No use, distribution or reproduction is permitted which does not comply with these terms.
*Correspondence: Angela Serpe, c2VycGVAdW5pY2EuaXQ=
†These authors have contributed equally to this work and share first authorship