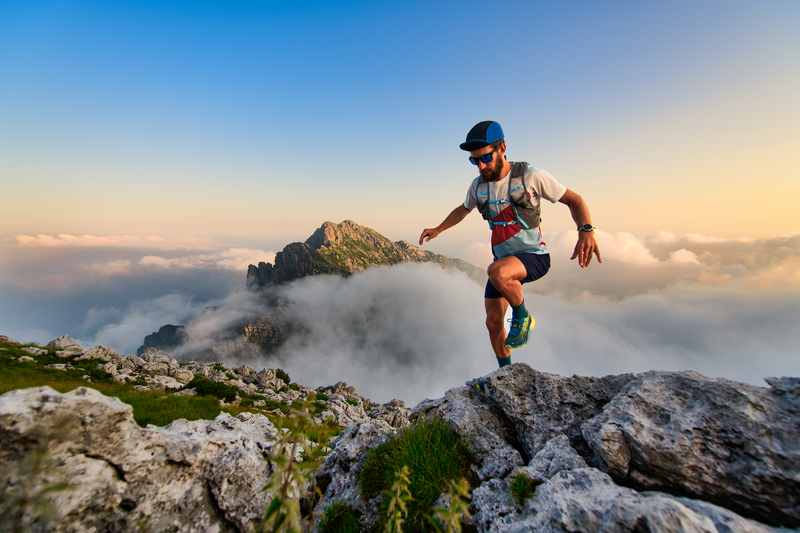
95% of researchers rate our articles as excellent or good
Learn more about the work of our research integrity team to safeguard the quality of each article we publish.
Find out more
ORIGINAL RESEARCH article
Front. Environ. Chem. , 02 March 2023
Sec. Chemical Treatments
Volume 4 - 2023 | https://doi.org/10.3389/fenvc.2023.1114807
This article is part of the Research Topic Women In Environmental Chemistry View all 6 articles
Most researchers assume minimal impact of pretreatment on strontium isotope ratios (87Sr/86Sr) for bones and teeth, and methods vary tremendously. We compared 14 pretreatment methods, including no prep other than powdering enamel, ashing, soaking in water, an oxidizing agent (bleach or hydrogen peroxide) or acetic acid (0.1 M, 1.0 M, and 1.0 M buffered with calcium acetate), and a combination of these steps. We prepared and analyzed aliquots of powdered molar enamel from three proboscideans (one modern captive Indian elephant, Elephas maximus indicus; one Pleistocene mastodon, Mammut americanum; and one Miocene gomphothere, Afrochoerodon kisumuensis). Each pretreatment was performed in triplicate and we measured 87Sr/86Sr, Sr concentration, and uranium (U) concentration, using the same lab space and instrumentation for all samples. Variability in 87Sr/86Sr and Sr and U concentrations was considerable across pretreatments. Mean 87Sr/86Sr across methods ranged from 0.70999 to 0.71029 for the modern tooth, 0.71458 to 0.71502 for the Pleistocene tooth, and 0.70804 to 0.70817 for the Miocene tooth. The modern tooth contained the least Sr and negligible U. The Pleistocene tooth contained slightly more Sr and measurable amounts of U, and the Miocene tooth had approximately 5x more Sr and U than the Pleistocene tooth. For all three teeth, variance in 87Sr/86Sr, Sr concentrations, and U concentrations among replicates was statistically indistinguishable across pretreatments, but there were apparent differences among pretreatments for the modern and Pleistocene teeth. Both contained relatively little Sr, and it is possible that small amounts of exogenous Sr from reagents, building materials or dust affected some replicates for some pretreatments. For the modern tooth, median 87Sr/86Sr varied considerably (but statistically insignificantly) across pretreatments. For the Pleistocene tooth, variability in median 87Sr/86Sr was also considerable; some pretreatments were statistically distinct but there were no obvious patterns among methods. For the Miocene tooth, variability in median 87Sr/86Sr was much smaller, but there were significant differences among pretreatments. Most pretreatments yielded 87Sr/86Sr and Sr concentrations comparable to, or lower than, untreated powder, suggesting selective removal of exogenous material with high 87Sr/86Sr. Further evaluation of the mechanisms driving isotopic variability both within and among pretreatment methods is warranted. Researchers should clearly report their methods and avoid combining data obtained using different methods. Small differences in 87Sr/86Sr could impact data interpretations, especially in areas where isotopic variability is low.
Strontium isotope ratios (87Sr/86Sr) are widely used by archeologists, paleoecologists, ecologists, and forensic scientists to evaluate origins and movement of animals, people, and traded goods (see recent reviews by Britton et al., 2021; Wathen et al., 2022). Thanks to decreased analytical costs, increased availability of instrumentation, and the development of numerous geospatial models that allow researchers to predict bioavailable 87Sr/86Sr for specific regions and localities, applications of this geochemical tool have surged in recent years (Figure 1).
FIGURE 1. Summary of citations for four different search strings on Google Scholar within 5-year time bins. Results were similar, but overall counts were slightly lower if British English was used (i.e., archaeology, palaeontology, palaeoecology). Data compiled on 13 September 2022.
Despite such advances, there is still room for additional work. In particular, the methods used to prepare tooth and bone for isotopic analysis (called sample pretreatments) vary tremendously among researchers, and there has been remarkably little work comparing them. As noted by others (e.g., Pellegrini and Snoeck, 2016; Snoeck and Pellegrini, 2015; Wathen et al., 2022), this is overdue. We compared 14 different sample pretreatments for enamel hydroxyapatite (Ca10(PO4)6(OH)2), which are representative of published methods (Table 1). These range from no preparation (aside from mechanically cleaning and powdering a sample), to using a variety of chemicals to remove secondary minerals and organics, combusting samples to remove organics, or a combination of these approaches. We prepared homogenized powdered molar enamel from one modern Indian elephant, one Pleistocene mastodon, and one Miocene gomphothere, and measured 87Sr/86Sr, strontium (Sr) concentration, and uranium (U) concentration, using the same lab space and instrumentation for all samples. We included concentration data to help monitor selective removal or addition of material (e.g., diagenetic carbonates) with more or less Sr or U. We anticipated finding significant differences in both isotopic and elemental results among pretreatments, especially for the older teeth, which had been buried for thousands to millions of years.
TABLE 1. Summary details for each of the 14 sample pretreatments. See Table 2 for additional details regarding specific reagents.
Wathen et al. (2022) published a comprehensive review of the history of sample pretreatment approaches for Sr isotope analysis and their evolution over time. Here we briefly recap what the main approaches are and why they are used. The primary purpose of pretreating osseous tissues prior to analysis is to remove exogenous minerals (chiefly diagenetic carbonates that either fill pores or adsorb onto crystal surfaces; Krueger, 1991), and to isolate biogenic isotope values. Some pretreatment methods also remove organic matter. Ideally, chemical pretreatment will remove exogenous non-lattice-bound material without affecting the isotopic composition of the biogenic mineral or introducing contamination or secondary minerals.
Despite a regular stream of methodological research regarding sample pretreatment for isotopic analysis since the mid-80’s (reviewed in Wathen et al., 2022), few researchers have explicitly considered strontium, particularly Sr isotopes in enamel (see Hoppe et al., 2003; Trickett et al., 2003). The approaches used to pretreat osseous tissues for 87Sr/86Sr analysis have their roots in three foundational studies: Sillen (1986); Krueger and Sullivan (1984); and Nelson et al. (1986). Sillen (1986) was interested in using the relative amounts of strontium and calcium in fossil bones (Sr/Ca) to evaluate diet. He developed a method, known as solubility profiling, which involves repeatedly rinsing powdered samples in sodium acetate-buffered acetic acid (pH ∼4.5), and analyzing the leachate after each wash to progressively track the removal of soluble diagenetic mineral, and isolation of biogenic Ca and Sr. During each rinse, samples are sonicated in buffered acid for 1 minute, centrifuged, and the supernatant is removed and analyzed. Krueger and Sullivan (1984) introduced a method for isolating biogenic carbonate (which substitutes for phosphate in hydroxyapatite) in bone for carbon (δ13C) isotope analysis. This method involves a two-step process of 1) soaking overnight in Alconox (an alkaline detergent that removes organics), and 2) soaking overnight in unbuffered 1 M acetic acid to remove secondary carbonates. Although this method was developed specifically for δ13C analysis, it has been extremely influential in strontium isotope research. Lastly, Nelson et al. (1986) were explicitly interested in strontium isotopes. Following methods from much earlier work on the Sr content of human bones (Hodges et al., 1949; Turekian and Kulp, 1956), they ashed cleaned bone samples at 800°C for 24 h to remove organics and then analyzed the remaining residue.
Over the years, solubility profiling has only been used sparingly (e.g., Tuross et al., 1989; Hoppe et al., 2003; Trickett et al., 2003). There are several reasons for this. First, this approach requires multiple analyses, which inflates the per-sample cost. Second, the isotopic composition of “clean” washes and ultimate remaining powder (which should both reflect biogenic Sr) may be isotopically similar (Price et al., 1992; Trickett et al., 2003), and it is far easier to work with a single, dried, powdered sample than multiple liquid samples (particularly for researchers who do not have a mass spectrometer at their home institution). Lastly, repeated acid washing may not be effective at isolating biogenic strontium in ancient bone or dentine (particularly if the diagenetic Sr is incorporated into the mineral matrix), and single rinses may be just as effective for enamel (Tuross et al., 1989; Lambert et al., 1990; Trickett et al., 2003).
In contrast, acetic acid and ashing have both remained widely used. Chemical pretreatment with acetic acid (sometimes combined with a step that removes organics), is the most commonly used sample pretreatment approach for δ13C and oxygen (δ18O) as well as Sr isotope research. Initially, researchers interested in C and O isotopes used relatively concentrated (1 M) acetic acid to remove exogenous minerals (e.g., Krueger and Sullivan, 1984; Lambert et al., 1990; Price et al., 1992). However, soaking powdered bone or tooth in 1 M acetic acid has been repeatedly shown to lead to ion exchange and sample recrystallization (e.g., Lambert et al., 1990; Koch et al., 1997; Garvie-Lok et al., 2004). Because ion exchange and recrystallization both impact δ13C and δ18O, many researchers who are specifically working with these two isotopes have switched to using more dilute acid (i.e., 0.1 M) or advocated for using 1 M acetic acid buffered with either sodium (C2H3NaO2) or calcium acetate (C4H6CaO4), which have a higher pH (ca. 4.5 vs. 2.5 to 3 for unbuffered acetic acid), and thus reduces sample loss and the potential for recrystallization (Sillen, 1986; Lambert et al., 1990; Christoffersen et al., 1997; Koch et al., 1997; Yoder and Bartelink, 2010; Crowley and Wheatley, 2014; Snoeck and Pellegrini, 2015). The possibilities of leaching and isotopic exchange have not been previously considered in strontium isotope research but may be important, especially for older specimens that contain diagenetic Sr.
The length of time that a sample is allowed to react in acid is also important. Shorter reaction times (4 hours or less) may not be sufficient for removing diagenetic carbonates, while longer reaction times (12 + h) may increase the chances that initially removed diagenetic mineral gets reintegrated back into a sample due to chemical equilibrium or recrystallization (e.g., Lee-Thorp and van der Merwe, 1991; Garvie-Lok et al., 2004; Yoder and Bartelink, 2010). Strontium ions can replace Ca2+ in hydroxyapatite (Ca10(PO4)6(OH)2), as well as exogenous calcium-bearing minerals, like calcite (CaCO3), dolomite (CaMg(CO3)2, or brushite (CaHPO4.2H2O), even at neutral pH in water (e.g., Christoffersen et al., 1997; Littlewood et al., 2017; Alshaaer et al., 2020). Moreover, the amount of strontium in hydroxyapatite or a reagent can impact both dissolution and adsorption of Sr. The degree to which leaching of biogenic Sr, ion exchange, or recrystallization could impact 87Sr/86Sr has not been previously evaluated, and researchers continue to use a wide variety of acid concentrations and reaction times when preparing samples for 87Sr/86Sr analysis.
Removal of organics from a sample, either by soaking in a dilute oxidizing solution (e.g., 2%–3% sodium hypochlorite/bleach, NaOCl; 30% hydrogen peroxide, H2O2), or combusting (ashing), may also be beneficial because organics can inhibit complete dissolution of apatite during isotopic analysis (Zazzo et al., 2006). Koch et al. (1997) determined that when combined with an acetic acid soak, both NaOCl and H2O2 effectively removed organic material without impacting carbonate C or O isotopes. However, Crowley and Wheatley (2014) explicitly compared the independent and combined effects of different oxidizers and acids, concluding that treatment with NaOCl resulted in the formation of a secondary carbonate, but treatment with H2O2 did not increase carbonate content. They also determined that treatment with H2O2 followed by Ca acetate-buffered acetic acid resulted in less sample loss and less extreme impacts on carbon and oxygen isotopes compared to acid alone. Snoeck and Pellegrini (2015), and Pellegrini and Snoeck (2016) subsequently confirmed that treatment with NaOCl increases carbonate content by reacting with atmospheric CO2 and inducing the adsorption of exogenous carbonates. However, they also found that H2O2 may not be effective at removing organics. They further argued that because H2O2 is acidic, it may remove structural carbonates, and advocated for using a different oxidizing agent (hydrazine hydrate, N2H4·H2O).
Ashing bones or teeth at a high temperature is an alternative approach for removing organic material that is widely used by strontium isotope researchers. However, published temperatures and times for ashing vary from 500 to 825°C and 8–12 h (e.g., Sealy et al., 1991; Grupe et al., 1997; Price et al., 2000; Alonzi et al., 2019). Some researchers have raised concerns about the higher temperatures leading to sample recrystallization, which could integrate exogenous material into the enamel’s crystal lattice (e.g., Sillen and Sealy, 1995). While this approach avoids adding any chemicals to the sample (which could potentially cause contamination; Snoeck and Pellegrini, 2015; Pellegrini and Snoeck, 2016), ashing also cannot remove any strontium from a sample. Consequently, some authors have added an additional short ca. 30 min soak in acetic acid (typically 5%, or 0.9 M) prior to ashing to help remove exogenous Sr (e.g., Bentley et al., 2003; Knudson et al., 2004; Schwartz et al., 2021).
Due to concerns regarding the susceptibility of bone and dentine to diagenesis, many researchers have stopped working with these more porous and organic-rich materials and instead work exclusively with enamel (reviewed in Wathen et al., 2022). However, some researchers also assume that enamel is impervious to diagenetic alteration. These researchers do not subject samples to chemical analysis or high temperatures. Most manually remove the outer tooth surface (e.g., (Glassburn et al., 2018; Moore et al., 2020), cementum, or dentine (e.g., Budd et al., 2000; Hedman et al., 2009; Pokutta et al., 2019; Rogers et al., 2019), and sometimes clean samples with deionized water or acetone (e.g., (Evans et al., 2006; Esker et al., 2019; Janzen et al., 2020). These approaches minimize damage to a specimen. However, modern enamel does contain a small amount of organics, and assuming enamel integrity for older specimens may be unwise (e.g., Lambert et al., 1990; Kohn et al., 1999). Simply cleaning a specimen’s surface, or removing the exterior layer of material, may not completely remove exogenous strontium, which can also fill cracks and voids, adsorb onto crystal surfaces, and even replace ions in the enamel hydroxyapatite.
We conducted a literature search using the search terms “strontium isotope,” “ecology,” “paleontology,” “archeology,” “bone” and “enamel” and recorded trends in the methodology used by different researchers. Many publications we reviewed did not provide sufficient details to be able to reproduce their work. This includes not specifying the duration of chemical soaks, the temperature at which these were done, the amount of sample or liquid used, or in some cases, even the type of acetate buffer used or the chemical concentration. There was also a tendency for authors to simply cite a previous publication (often a book chapter or dissertation). Nevertheless, we did our best to summarize the pretreatment methods we encountered, and to use these to create 14 different enamel pretreatments that represent a spectrum of what has been published (Table 1). Eleven of these are published, or a variant of sample pretreatment methods that are published. These included no pretreatment other than powdering enamel, ashing, soaking in water, an oxidizing agent (bleach or hydrogen peroxide) or a range of concentrations of acetic acid (0.1 M, 1.0 M, and 1.0 M buffered with Ca acetate), and a combination of these steps (oxidizer + acid; acid + ashing).
We worked with enamel from three proboscidean molars with varying ages (modern, Pleistocene, and Miocene) and preservation conditions (Figure 2). Our modern sample is a shed molar fragment from “Sabu,” a captive male Indian elephant, Elephas maximus indicus, from the Cincinnati Zoo (Cincinnati, Ohio, United States). The Pleistocene sample is a fragment of an isolated molar from a mastodon (Mammut americanum) from the Cincinnati Museum Center (VP 7468s). The specimen was found in Ohio but detailed age and provenience are unknown. It was most likely recovered from glacial till (Glenn Storrs personal communication). The Miocene sample is a molar fragment from a gomphothere (Afrochoerodon kisumuensis) from the Duke Primate Center’s Division of Fossil Primates collection (DPC 14544). This specimen comes from the Moghara (also referred to as the Moghra), a region in western Egypt comprised of approximately 400 m of siliciclastic sediments that have been variously interpreted as shallow marine, semi-continental, fluvial-tidal, and estuarine (reviewed in Georgalis et al., 2020). Researchers have previously used 87Sr/86Sr to develop a chronology for macrofossils from the Moghara (based on the global seawater curve) and determined that the site is 17–21 million years old (Hassan, 2013).
FIGURE 2. Photographs of the three specimens (all molar fragments) included in this study: (A) modern male Indian elephant from the Cincinnati Zoo (“Sabu”); (B) Pleistocene American mastodon from Ohio, USA (VP 7468); and (C) Miocene gomphothere from the Moghara of Western Egypt (DPC 14544). Blue bars indicate 1 cm.
We confirmed the biogenicity of hydroxyapatite using Ca/P (following Alonzi et al., 2019; Simpson et al., 2023). We scanned the surface of each tooth using a Bruker Tracer 5i handheld x-ray fluorescence spectrometer and determined that all three specimens yielded Ca/P within the range expected for biogenic hydroxyapatite (1.3–2.3).
Initial sample preparation and pretreatment were conducted in the Quaternary Paleoecology Laboratory in the Department of Geosciences at the University of Cincinnati. This lab, which is a single, undivided room exposed to the main ventilation system for the building, is typical of the kinds of workspaces available to most researchers (particularly those who process samples to be sent to other institutions for analysis). We manually cleaned a ca. 1.5 cm strip of the exterior surface of each tooth and removed the outer layer of enamel. We then collected ca. 2 g of enamel powder from the cleaned area using a Dremel handheld rotary tool equipped with a steel carbide dental bit. Powder from each tooth was stored in a scintillation vial that had been cleaned with trace metal grade nitric acid. We considered this bulk powder to be homogenous.
We prepared three replicate samples of powder from each tooth for each pretreatment method. For methods that required a wet chemistry step, aliquots of powder were weighed into 2-mL screw-top polypropylene microcentrifuge tubes that had also been cleaned using trace metal grade nitric acid. Samples were rinsed 5x with ultrapure Milli-Q water after all chemical soaks, and we also used Milli-Q water for all dilutions. Wet samples were freeze-dried. For additional details regarding pretreatment methods, including reagents used, see Table 2. Powder destined for ashing was weighed into ceramic crucibles and baked in a muffle furnace in a separate lab space in the same building. Ashed samples were allowed to cool overnight in the furnace. We weighed aliquots of all pretreated samples using wax paper and a microbalance and transferred them to new pre-cleaned microcentrifuge tubes for analysis at the University of Illinois Urbana-Champaign (UIUC).
TABLE 2. Sr and U concentration data for reagents and empty, pre-cleaned plastic microcentrifuge tubes used for sample pretreatment at the University of Cincinnati. All stock solutions and beakers were borosilicate glass. We report summary data for three replicates for MilliQ water squeeze bottles. BDL = Below Detection Limit (0.017 μg/L for Sr; 0.003 μg/L for U).
To check for contamination within the lab, we collected aliquots of parent and stock solutions of all reagents, including Milli-Q water used to rinse samples. We also monitored possible accumulation of dust or other particulates by placing open, empty, pre-cleaned microcentrifuge tubes next to the microbalance and centrifuge in the lab for 3 days while lab users conducted normal activities.
Pretreated samples, reagents, and empty tubes were sent to the Isotope Geochemistry Laboratory at UIUC and remaining sample preparation and analyses were conducted in a clean lab. We obtained 87Sr/86Sr as well as Sr and U concentrations for the samples, chemical reagents and empty “blank” vials that had been left open in the pretreatment lab.
The powder in each tube was dissolved in 0.5 mL of ultra-pure 3 M nitric acid at room temperature, overnight. All nitric acid used at UIUC for dissolving powders, performing ion exchange procedures, and diluting reagents was made from concentrated (14 M) reagent grade nitric acid that had been double-distilled in a Savillex DST-1000 sub-boiling still. To obtain Sr and U concentrations, 50 μL of each sample solution was transferred to a 4 mL polypropylene autosampler tube and mixed with 0.4 mL of Milli-Q water and 1.55 mL of 2% nitric acid.
Aliquots of aqueous reagents used during sample pretreatment were diluted with 2% distilled nitric acid by factors of 1.02, 10 or 100 to enable Sr and U concentration measurements. Acids and oxidizers were diluted 10x or 100x depending on molarity of the original solutions. Water was diluted by a factor of 1.02 (we added 40 μL of concentrated, 14 M, nitric acid to 2 mL of water to acidify them for analyses). The contents of empty vials were prepared by adding 2 mL of 2% distilled nitric acid.
Strontium and U concentrations were analyzed on a Thermo Scientific iCAP Qc quadrupole inductively coupled plasma mass spectrometer (ICP-MS). SRM-1643f was used as a check standard roughly every 20 samples. Analytical precision for Sr was estimated from repeated analyses of 1:100 dilutions of SRM-1643f, while analytical precision for U was estimated from a multi-element concentration standard. Precision for Sr and U was approximately 5% and 10%, respectively. For powdered samples, analytical detection limits for Sr and U were 0.68 and 0.12 μg/L, respectively. For reagents, analytical detection limits for Sr and U were 0.017 and 0.003 μg/L, respectively.
For 87Sr/86Sr analysis, the remaining dissolved pretreated powdered samples were loaded onto ion exchange columns filled with 300 μL bed volume Eichrom Sr-spec strontium-specific resin, which was then rinsed with 3 and 8 M ultrapure nitric acid to elute all elements aside from Sr. Sr was eluted with 0.05 M nitric acid into autosampler vials. Blanks consisting of 3 M nitric acid were prepared along with each batch of samples; these typically yielded 0.8 ng Sr.
Strontium isotope data were measured on a Nu Plasma High-Resolution MC-ICPMS (Wrexham, UK). Mass bias correction was done by internal normalization (assumed 86Sr/88Sr = 0.11940), and corrections were made for interferences from Kr, Rb, and Ba. Results were normalized using NIST SRM-987 (accepted 87Sr/86Sr = 0.710255), with generally one analysis per four unknowns. Precision and accuracy were monitored using measurements of the Eimer and Amend standard and an in-house standard solution derived from a modern marine coral. Analytical reproducibility for 87Sr/86Sr was nominally ± 0.00005, but we used a more conservative ± 0.0001 for the modern and Pleistocene teeth, which contained less Sr (<500 ng) and therefore had greater sensitivity to background Sr contamination.
We calculated Sr and U concentrations in the treated powders (ng Sr or U/mg sample) by multiplying measured Sr and U concentrations in the dissolved sample solution by their volume (0.5 mL) and then dividing by the mass of pretreated sample that was sent to UIUC for analysis. For most pretreatments, we weighed aliquots of pretreated powder for analysis. However, we were concerned about sample size for powdered enamel soaked in unbuffered 1 M acetic acid (pretreatments 5 and 13). We did not attempt to weigh aliquots of these pretreated samples and instead sent all remaining material to Illinois for analysis. Unfortunately, this meant we did not know the mass of material that was analyzed, which prevented calculating Sr and U concentrations. We attempted to estimate sample mass for these pretreatments using regressions between mass and Ca concentration for other pretreatments. However, we found that estimated masses for pretreatments 5 and 13 varied tremendously depending on which pretreatments were included in the regression calculation. Given the variability of estimated masses, we did not feel comfortable calculating Sr and U concentrations for these two pretreatments.
For each tooth, we confirmed homogeneity of variance in 87Sr/86Sr, Sr concentration, and U concentration among pretreatments using O’Brien tests, and we compared pretreatments using both one-way analysis of variance (ANOVA) coupled with post hoc Tukey’s tests and Kruskal-Wallis tests coupled with post hoc Steel-Dwass All Pairs tests. We also used Pearson’s correlation coefficients to check for relationships between 87Sr/86Sr, Sr concentration, and U concentration among pretreatments for each tooth. All statistical analyses were performed using JMP Pro 16.1 and significance was set at α = 0.05.
We found considerable variability in 87Sr/86Sr and Sr concentrations across sample pretreatments for all three teeth. Uranium concentrations were at or below detection limit for the modern tooth and two replicates of pretreatment 7 for the Pleistocene tooth, but varied among other pretreatments for the Pleistocene tooth, and also among pretreatments for the Miocene tooth (Figure 3). Mean 87Sr/86Sr for different pretreatments ranged from 0.70999 to 0.71029 for the modern tooth, 0.71458 to 0.71502 for the Pleistocene tooth, and 0.70804 to 0.70817 for the Miocene tooth (Table 3). The modern tooth contained the least Sr and negligible U. The Pleistocene tooth had similar Sr concentration but greater U concentrations than the modern tooth, and the Miocene tooth had approximately 5x more Sr and U than the Pleistocene tooth (Figure 3). Raw data are provided in Supplementary Table S1.
FIGURE 3. Box plots comparing (A) 87Sr/86Sr; (B) strontium concentrations, and (C) uranium concentrations across pretreatments for the three specimens. Results for both one-way ANOVA and Kruskal-Wallis analyses are provided. Letters within each panel indicate results of post hoc Tukey’s comparisons. Groups that share a letter were statistically indistinguishable. Uranium concentrations for all analyses for the modern specimen, and two replicates for pretreatment 7 for the Pleistocene tooth were at or below detection limit (0.12 μg/L). We plotted theses as 0.0 μg/L. We did not have good mass estimates for pretreatments 5 or 13, and therefore were not able to calculate elemental concentrations for these pretreatments. Analytical precision for 87Sr/86Sr was nominally ±0.00005, but we used a more conservative ±0.0001 for samples containing less than 500 ng Sr because of their greater sensitivity to background Sr contamination. We used the smaller value for the Miocene tooth and the more conservative value for the Modern and Pleistocene teeth. Analytical precision for Sr and U concentrations were 5% and 10%, respectively.
TABLE 3. Summary data for each experimental treatment for each tooth. N = 3 for each row. Treatments are explained in Table 1. BDL = Below Detection Limit (0.68 μg/L for Sr; 0.12 μg/L for U).
Variances in 87Sr/86Sr, Sr concentrations, and U concentrations among replicates were statistically indistinguishable across pretreatments for each tooth, but there were notable apparent differences (Figure 3). Variability in 87Sr/86Sr among replicates was rather pronounced for some pretreatments for both the modern and Pleistocene tooth, and in some cases was larger than our conservative analytical uncertainty estimate (±0.0001). In contrast, variance in 87Sr/86Sr among replicates for the Miocene tooth was smaller than our nominal analytical uncertainty of ± 0.00005. Pretreatment 6 (1 M Ca acetate-buffered acetic acid) had apparently more variable Sr concentrations for the modern tooth, while pretreatment 7 (2%–3% NaOCl) and pretreatment 2 (ultrapure water) had apparently more variable Sr concentrations for the Pleistocene and Miocene tooth, respectively. Pretreatment 14 (H2O + 5% acetic acid + ashing) had apparently more variable U concentrations than other pretreatments for the Pleistocene tooth.
There were few consistent trends in mean and median 87Sr/86Sr, Sr concentrations, or U concentrations among pretreatments for the three teeth. Most pretreatments were statistically distinguishable using both parametric ANOVA and non-parametric Kruskal-Wallis analyses. Yet Steel-Dwass all pairs post hoc comparisons failed to detect any pairwise differences. There were very obvious visual differences, and post hoc Tukey’s analyses detected quite a few pairwise differences among groups. We therefore report the results of the Tukey’s tests below and in Figure 3.
Strontium isotope ratios were statistically indistinguishable among pretreatments for the modern specimen. They differed significantly among pretreatments for both the Pleistocene and Miocene teeth, but no coherent patterns emerged (Figure 3). For the Pleistocene tooth, post hoc Tukey’s analyses indicated that pretreatment 4 (0.1 M Acetic acid) had significantly higher 87Sr/86Sr than pretreatments 6 (1 M buffered acetic acid) or 9 (30% H2O2 + 1 M buffered acetic acid); pretreatment 13 (2%–3% NaOCl + 1 M acetic) also had significantly higher 87Sr/86Sr than pretreatment 6. Untreated powder (pretreatment 1) was statistically indistinguishable from all other pretreatments for the Pleistocene tooth. Nevertheless, there were interesting apparent differences; most pretreatments had 87Sr/86Sr that was comparable to, or higher than, untreated powder.
For the Miocene tooth, overall variability in 87Sr/86Sr was much smaller (Table 3; Figure 3). Pretreatment 3 (ashed sample) had the highest 87Sr/86Sr (significantly higher than pretreatments 4–6 and 9–13), while Pretreatment 13 had the lowest 87Sr/86Sr (significantly lower than pretreatments 1–4, 7–9, and 12). Strontium isotopes for most pretreatments were comparable to, or lower than, untreated powder (pretreatment 1).
Strontium concentrations were statistically indistinguishable among pretreatments for the modern and Pleistocene teeth but differed significantly for the Miocene tooth (Table 3; Figure 3). Post-hoc Tukey’s tests indicate that pretreatment 3 (ashing sample) had significantly higher Sr concentrations than any of the other pretreatments for the Miocene tooth, and pretreatments 6 (1 M buffered acetic acid), 11 (2%-3% NaOCl + 1 M buffered acetic acid), and 12 (2%-3% NaOCl + 0.1 M acetic acid) had the lowest Sr concentrations for the Miocene tooth. Pretreatment 3 also had apparently higher Sr concentrations than all other methods for the Pleistocene tooth, with the exception of one replicate for pretreatment 7 (2%–3% NaOCl).
Uranium concentrations differed significantly among pretreatments for the Pleistocene and Miocene teeth. For the Pleistocene tooth, pretreatment 14 (H2O + 0.9 M acetic acid + ashing) had the highest U concentration, while pretreatments 1, 2, 6, 7, and 9, and 11 had the lowest U (Table 3; Figure 3). With the exception of one replicate for pretreatment 14, the overall range in U concentrations was small for the Pleistocene tooth (Figure 3). In contrast, there was a considerable range in U concentrations across pretreatments for the Miocene tooth. Tukey’s tests indicated that pretreatment 14 had the highest concentrations (roughly two times more than untreated powder), while pretreatments 2, 6 and 7 had the lowest U concentrations.
Including all data, there were no relationships between 87Sr/86Sr and Sr concentration for the modern specimen (Figure 4). There was also no relationship between 87Sr/86Sr, Sr concentration, or U concentration for the Pleistocene specimen; the single replicate of pretreatment 14 with elevated U was clearly distinct from all other datapoints, while the replicate for pretreatment 7 with an elevated Sr concentration did not stand out (Figure 5). Removing the outlier U datum for pretreatment 14, there was still no relationship between 87Sr/86Sr and U, but a weak significant positive relationship emerged between Sr and U concentrations (Figure 5). For the Miocene tooth, there was a significant positive relationship between 87Sr/86Sr and Sr concentration, and a significant negative relationship between 87Sr/86Sr and U concentration (Figure 6). Pretreatments 3 and 14 (with elevated Sr or U concentrations, respectively) were distinct, but given that all three replicates yielded similar data, we did not exclude them from the correlation analyses.
FIGURE 4. Bivariate plot showing relationships between 87Sr/86Sr and Sr concentration for the modern specimen. Uranium concentrations were below detection limit for all analyses (0.12 μg/L).
FIGURE 5. Bivariate plots showing relationships between 87Sr/86Sr, strontium concentrations, and uranium concentrations for the Pleistocene specimen. Strontium and U concentrations were not available for pretreatments 5 or 13. Uranium concentrations were below detection limit for two of the replicates for pretreatment 7 (0.12 μg/L), and are plotted as 0.0 μg/L. Unusual data are noted using different colored circles. We excluded the single replicate with an elevated U concentration for pretreatment 14, but included the replicate for pretreatment 7 with elevated Sr concentrations in correlation analyses.
FIGURE 6. Bivariate plots showing relationships between 87Sr/86Sr, strontium concentrations, and uranium concentrations for the Miocene specimen. Uranium concentrations were not available for pretreatments 5 or 13. Unusual data for pretreatments 3 and 14 are noted using different colored circles. All data were included in correlation analyses.
Comparing those pretreatments that should have removed organics from enamel (pretreatment 3: ashing; pretreatment 7: 2%–3% NaOCl; and pretreatment 8: 30% H2O2), we found that ashing resulted in apparently higher 87Sr/86Sr than pretreatment 7 for the modern tooth, pretreatment 8 for the Pleistocene tooth and both pretreatments for the Miocene tooth (Table 3; Figure 3). Ashed samples also had the highest Sr concentrations for all three teeth, and apparently higher U concentrations for the Pleistocene tooth. In contrast, pretreatments 7 and 8 were less distinct. There were small apparent differences in 87Sr/86Sr between the two methods for the modern and Pleistocene teeth, apparent differences in U concentration for the Pleistocene tooth, and significant differences in U concentrations for the Miocene tooth (Table 3; Figure 3). For all three teeth, both pretreatments 7 and 8 had statistically indistinguishable 87Sr/86Sr and Sr concentrations from the untreated powder (pretreatment 1).
Comparing pretreatments that involved acetic acid, which should remove exogenous carbonates, there were apparent, but statistically indistinguishable, differences in 87Sr/86Sr among 0.1 M acetic acid (pretreatment 4), 1.0 M acetic acid (pretreatment 5), and 1 M buffered acetic acid (pretreatment 6) for the modern tooth, and significant differences for both the Pleistocene and Miocene teeth (Figure 3; Table 3). We do not have elemental concentrations for pretreatment 5, but pretreatments 4 and 6 had statistically indistinguishable Sr concentrations for the modern and Pleistocene teeth, and U concentrations for the Pleistocene tooth. Pretreatment 4 had significantly higher U concentrations than pretreatment 6 for the Miocene tooth.
Looking at pretreatments that combined both an oxidizing and acid step (pretreatments 9–13), we found that methods that used 30% H2O2 as the oxidizing reagent (pretreatment 9: H2O2 + 1 M buffered acetic acid; and pretreatment 10: H2O2 + 0.1 M acetic acid) had similar 87Sr/86Sr for the modern and Miocene teeth, but diverged considerably for the Pleistocene tooth. Pretreatment 9 had low 87Sr/86Sr that was comparable to pretreatments 8 (30% H2O2), 6 (buffered acetic acid), and 11 (2%–3% NaOCl + 1 M buffered acetic acid), while pretreatment 10 had higher 87Sr/86Sr that was comparable to pretreatments that used unbuffered acetic acid: 4 (0.1 M acetic acid); 5 (1 M acetic acid); and 13 (2%–3% NaOCl + 1 M acetic). Strontium concentrations were indistinguishable among pretreatments for all three teeth, and U concentrations were indistinguishable for the Pleistocene and Miocene teeth (Table 3; Figure 3).
Comparing pretreatments that used 2%–3% NaOCl but differed in their acid step (11, 12, and 13), we observed that pretreatments 11 (NaOCl + 1 M buffered acetic) and 12 (NaOCl + 0.1 M acetic) had statistically indistinguishable 87Sr/86Sr, Sr concentrations, and U concentrations for all three teeth (Table 3; Figure 3). Pretreatment 12 had apparently higher 87Sr/86Sr than pretreatment 11 for the modern and Pleistocene teeth. Pretreatment 13 (NaOCl + 1 M acetic acid), had apparently higher 87Sr/86Sr for the Pleistocene tooth (comparable to pretreatments 4, 5 and 10, which also used unbuffered acetic acid), and low 87Sr/86Sr for the Miocene tooth (comparable to pretreatment 5; 1 M acetic). These smaller differences among pretreatments may be the result of shorter acid soaks for pretreatments 11 and 12 (only 4 h versus 24 h for pretreatments 9 and 10).
Pretreatments that used buffered 1 M acetic acid but differed in their oxidizing reagent (9 and 11) also had very similar and statistically indistinguishable results for most measurements (Table 3; Figure 3). However, for the Miocene tooth, pretreatment 9 (30% H2O2 + buffered acetic) had apparently larger Sr concentrations than pretreatment 11 (2%–3% NaOCl + buffered acetic), and both methods had U concentrations that were higher than just buffered acid or bleach (pretreatments 6, and 7), and comparable to just 0.1 M acetic acid (pretreatment 4) or 30% H2O2 (pretreatment 8).
Comparing pretreatments that used 0.1 M acetic acid but differed in their oxidizing reagent (10 and 12), we found that 87Sr/86Sr was again statistically indistinguishable for the modern and Miocene teeth (Table 3; Figure 3). Pretreatment 10 (30% H2O2+ 0.1 M acetic) had apparently higher 87Sr/86Sr than pretreatment 12 (2%–3% NaOCl + 0.1 M acetic) for the Pleistocene tooth (more similar to pretreatments 4, 5, and 13, which also involved unbuffered acetic acid). Mimicking trends observed for pretreatments 9 and 11, pretreatments 10 had apparently larger Sr concentrations than pretreatment 12 for the Miocene tooth. U concentrations for both methods were also higher than pretreatments 6 and 7, and comparable to pretreatments 4 and 8 for the Miocene tooth (Table 3; Figure 3).
Lastly pretreatment 14, which combined water, 5% acetic acid, and ashing, resulted in comparable 87Sr/86Sr to just water (pretreatment 2), just ashing (pretreatment 3), and just 1 M acetic acid (pretreatment 5) for the modern and Pleistocene teeth, but significantly lower 87Sr/86Sr than either pretreatments 2 or 3, and significantly lower Sr concentrations than pretreatment 3 for the Miocene tooth. Conversely, U concentrations for pretreatment 14 were very high for the Miocene tooth (Table 3; Figure 3). They were also elevated for one replicate for the Pleistocene tooth, although, as noted above, this datum was an anomaly.
Table 2 provides concentration data for reagents and empty “blank” tubes left open in high traffic areas of the main lab workspace at the University of Cincinnati. As expected for Ca-rich reagents, calcium acetate-buffered 1 M acetic acid contained a considerable amount of Sr (ca. 4,000 μg/L). The other reagents (non-buffered acetic, H2O2, and NaOH), contained much less Sr, although there was a measurable amount in both the 2%–3% NaOCl and the unbuffered 1 M acetic acid (Table 2). The other reagents and water contained less than 10 μg/L Sr. Uranium concentrations were negligible for all reagents except Ca acetate-buffered 1 M acetic acid (Table 2). For most reagents, elemental concentrations were similar for the parent stock solution and an aliquot poured into a beaker. However, H2O2 sampled from a beaker had a higher Sr concentration than the parent solution (Table 2). There was a detectable amount of Sr in several of the blank tubes, but U concentrations were at or below detection limit.
We set out to evaluate the degree to which sample pretreatment method might impact 87Sr/86Sr, Sr concentration, and U concentration of enamel hydroxyapatite. The lack of a “true” 87Sr/86Sr for each tooth makes it challenging to evaluate if one sample pretreatment method is universally better or worse than the others. Nevertheless, we may still be able to draw some conclusions regarding relative efficacy of different methods. Below, we discuss the possible reasons for variability both within and among pretreatments and provide some guidance for best practices and future research.
Isotopic data varied both among pretreatments and among replicates for some pretreatments for the modern and Pleistocene specimens. Variability in 87Sr/86Sr was particularly pronounced for the Pleistocene tooth. There were no obvious patterns in 87Sr/86Sr among pretreatments; untreated powder had both lower and higher 87Sr/86Sr than other methods for these teeth. Elemental concentrations were also variable, but considerably less so than 87Sr/86Sr. Relatively low Sr and U concentrations for both the modern and Pleistocene specimens, combined with similar elemental concentrations for untreated powder (pretreatment 1) and the various pretreatments (Table 3; Figure 3) suggest that neither specimen contained an appreciable amount of diagenetic Sr. This is unsurprising for the modern tooth, which was collected from a captive elephant living at a zoo. Enamel for the Pleistocene tooth was white and looked pristine. The exact provenance for this specimen is unknown, but we suspect that it came from Quaternary glacial till (isolated mammoth and mastodon teeth are frequently recovered from till in Ohio; Glenn Storrs personal communication).
In contrast, the Miocene tooth exhibited only minor isotopic variability among replicates for any given pretreatment. Trends in 87Sr/86Sr as well as Sr and U concentrations across pretreatments suggest the selective removal of exogenous material with high 87Sr/86Sr, an elevated Sr concentration, and low U concentration (Table 3; Figure 3). This specimen contained nearly five times more Sr and U than the Pleistocene tooth, and the enamel was visibly darkened (Figure 2). Slightly elevated U concentrations for most pretreatments compared to untreated powder could readily be explained if most of the observed U was incorporated into the hydroxyapatite matrix. Chemical pretreatments would not remove this U but would selectively remove other mineral (increasing the relative abundance of U in the samples).
The kind of acetic acid used to remove secondary minerals appears to have a measurable influence on both 87Sr/86Sr and elemental concentrations. 1 M acetic acid is rather aggressive, and therefore might be expected to remove the most mineral from samples. Because we did not have good estimates of sample mass for treatments 5 and 13 (1 M acetic acid, and 2%–3% NaOCl + 1 M acetic acid, respectively), we cannot evaluate elemental concentrations for pretreatments involving 1 M acetic acid. Both of these pretreatments had elevated 87Sr/86Sr for the Pleistocene tooth, and the lowest 87Sr/86Sr for the Miocene tooth (Table 3; Figure 3). Pretreatment 14 (H2O + 5% acetic acid + ashing) also had low 87Sr/86Sr for the Miocene tooth, and it did not have particularly low Sr concentrations. These patterns could be interpreted to indicate that the 1 M acetic acid was successful at removing diagenetic Sr from the Pleistocene and Miocene teeth. Extremely elevated U concentrations for pretreatment 14 for the Miocene tooth and one replicate for the Pleistocene tooth may also support removal of diagenetic minerals (assuming they contained minimal U and that most U was incorporated into the mineral matrix of the tooth enamel). However, these results might also indicate removal of some biogenic hydroxyapatite, or exchange of ions between hydroxyapatite, diagenetic minerals, and possibly the acid itself. We discuss these additional possibilities in more detail below.
Sample heterogeneity is a very unlikely explanation for the observed variation. The same parent vial of powdered enamel was used for all aliquots of a given tooth, and if heterogeneity were an issue, then isotopic variability among replicates should be somewhat random and scattered across pretreatments and teeth. We do not see this. Replicates for all pretreatments yielded identical values, within analytical uncertainty, for the Miocene tooth (Figure 3). Replicates for pretreatments 1 and 2 (untreated powder and soaking in ultrapure water) were also isotopically very similar for both the modern and Pleistocene teeth. Replicates for ashed samples (pretreatment 3) also had similar 87Sr/86Sr (within analytical error). It was only methods involving a chemical reagent and multiple rinses with ultrapure water that showed larger variability (Figure 3).
There was no evidence for any analytical issues with any of the analyses. However, some of the observed variability in elemental concentrations, particularly among replicates for the Pleistocene tooth, may reflect small differences between recorded and actual mass. For example, if we slightly overestimated mass due to incomplete transfer of weighed sample into pre-cleaned microcentrifuge tubes, this would have resulted in artificially low Sr or U concentration calculations. Low Sr concentrations for the modern and Pleistocene tooth could also have made them susceptible to possible contamination from reagents or the lab environment where pretreatment was conducted.
The degree to which contamination might have impacted samples in this study would depend on the 87Sr/86Sr, Sr concentration, and U concentration of both the contaminant and the sample. Contamination from a substance with Sr concentration, U concentration, or 87Sr/86Sr close to that of a given sample would have a smaller impact than a substance with strongly contrasting composition. We explicitly tested for the possibility of contamination in the lab where pretreatment was done. Empty tubes left open for multiple days in multiple places in the sample pretreatment lab contained negligible Sr and no detectable U (Table 2). We routinely wipe down lab surfaces, store glassware in a closed cabinet, and keep vials, boxes, and bottles closed, but the possibility of contamination during pretreatment persists. We anticipate that most contamination would have come from building materials containing calcium carbonates, oxides, hydroxides, and sulfates. These minerals likely all have 87Sr/86Sr between 0.708 and 0.709 (roughly comparable to seawater), but may vary slightly depending on when and where they formed, and the relative contribution of continental-derived Sr (reviewed in Capo et al., 1998). Contamination from dust or residue from other samples on work surfaces, tools, and equipment is also possible. The abundance and isotopic makeup of any of this contaminant would vary depending on what else was being processed in the lab at the same time.
87Sr/86Sr results may be especially sensitive to contamination as a result of their very small measurement uncertainties (e.g., ±0.00005). A speck of isotopically distinct dust could have had a measurable impact. For example, a 0.01 mg fragment of calcite could contain 10 ng of Sr, about 5% of the measured Sr mass for the most Sr-poor samples in this study. While this would have a minimal influence on Sr concentration, if 87Sr/86Sr for the contaminant Sr differed from that of the sample by 0.005, the resulting effect on measured 87Sr/86Sr would be 0.00025 (5x our nominal analytical precision for the modern and Pleistocene teeth). The variability in both 87Sr/86Sr and elemental concentrations among replicates for some pretreatments for the modern and Pleistocene teeth suggests that contamination may intermittently occur in the lab (Figures 3, 5).
It is also possible that some contamination was caused by the reagents themselves. Except for Ca acetate-buffered 1 M acetic acid, all of the parent solutions and stock reagents used contained minimal amounts of Sr and negligible U. Reagents should also have been fully rinsed from the powders with ultrapure water that contained negligible Sr and no U. Nevertheless, if there was adsorption of exogenous Sr or U onto samples, or exchange of Sr2+ ions between samples, exogenous mineral, and reagents, then this could have impacted measurements. It is also possible that some reagents removed some biogenic Sr. We discuss these possibilities in more detail below.
We were somewhat surprised that even sonication in ultrapure water (pretreatment 2) impacted both Sr and U concentrations for the Miocene tooth (Table 3; Figure 3). We suspect this is because hydroxyapatite dissolves (albeit slowly) in water (Christoffersen et al., 1997). Christoffersen and colleagues (1997) demonstrated that the more Sr in hydroxyapatite, the more readily the hydroxyapatite dissolves. This is likely because Sr is a larger atom than Ca, and when it substitutes into hydroxyapatite, it expands the crystal lattice, making the mineral more soluble (Rokita et al., 1993). Accordingly, pretreatment 2 might be expected to have selectively removed high-Sr material from the Miocene tooth, in particular, although there were no differences in 87Sr/86Sr between pretreatment 2 and untreated powder.
Researchers interested in C and O isotopes have repeatedly raised concerns about 1 M acetic acid leaching biogenic hydroxyapatite and enabling exchange of ions with secondary minerals (Lee-Thorp and van der Merwe, 1991; Koch et al., 1997; Garvie-Lok et al., 2004). Few researchers who prepare samples for δ13C and δ18O still use this reagent. However, the possibilities of leaching and isotopic exchange have not been previously considered in strontium isotope research. In addition to substituting for Ca2+ in hydroxyapatite (Ca10(PO4)6(OH)2), Sr2+ can readily substitute for Ca2+ in diagenetic and temporary secondary minerals formed during pretreatment, including calcite (CaCO3), dolomite (CaMg(CO3)2), and brushite (CaHPO4.2H2O), respectively forming Sr10(PO4)6(OH)2, SrCO3, SrMg(CO3)2, and SrHPO4*nH2O or SrHPO4 (Christoffersen et al., 1997; Littlewood et al., 2017; Alshaaer et al., 2020). If 1 M acetic acid liberates ions from diagenetic minerals, which may subsequently exchange with ions in biogenic hydroxyapatite, this would be problematic. This should be of most concern for older specimens that contain diagenetic material. However, if the acetic acid itself contained some Sr (which was the case in this study), there could also be the possibility of exchange of the Sr in the acid with biogenic Sr in hydroxyapatite, which could impact modern specimens. Moreover, if a pretreatment combines 1 M acetic acid and an oxidizer like NaOCl, which also contains some Sr and may form a secondary mineral, then there may be additional opportunity for exogenous Sr to get incorporated into a sample. Explicitly checking for the temporary formation of secondary minerals and exchange of Sr between samples and reagents would be useful research directions to pursue in a future study.
More dilute 0.1 M acetic acid should be less aggressive, and therefore have less opportunity to remove biogenic Sr or introduce exogenous Sr into enamel hydroxyapatite. Researchers interested in C and O isotopes have suggested that 0.1 M acetic acid is a good choice for removing diagenetic minerals from enamel (e.g., Yoder and Bartelink, 2010; Crowley and Wheatley, 2014), although Koch et al. (1997) noted that soaking modern dentine samples in 0.1 M acetic acid produced brushite, which they found troubling. In our study, the two pretreatments that used 0.1 M acetic (pretreatment 4: 0.1 M acetic acid; and pretreatment 10: H2O2 + 0.1 M acetic) did not have unusual 87Sr/86Sr or Sr concentrations for the modern tooth, or any of the measurements for the Miocene tooth. However, these pretreatments did have some of the highest 87Sr/86Sr for the Pleistocene tooth (similar to pretreatments 5 and 13, which involved 1 M acetic acid, and pretreatment 3, which involved ashing). Again, not knowing what “true” 87Sr/86Sr should be for this tooth makes it challenging to interpret if these results reflect removal of diagenetic Sr, incorporation of exogenous Sr, or some other factor, and we encourage additional work in this arena.
Using buffered 1 M acetic acid has previously been favored by researchers interested in C and O analyses because it helps reduce sample loss and also has a smaller influence on C and O isotopes than unbuffered acetic acid (Koch et al., 1997; Crowley and Wheatley, 2014). It may also help limit adsorption of exogenous Ca and Sr (Christoffersen et al., 1997). However, calcium acetate-buffered acetic acid also contains appreciable Sr (Table 2). Like calcium-rich building materials, we would anticipate that 87Sr/86Sr for Ca acetate, which is typically synthesized from limestone, should be around 0.708 or 0.709. This is lower than the 87Sr/86Sr for the Pleistocene and modern teeth but comparable to the Miocene tooth. Lower 87Sr/86Sr for pretreatments 6 (Ca acetate-buffered 1 M acetic acid) and 9 (30% H2O2 + buffered 1 M acetic) for the Pleistocene tooth, and apparently but insignificantly lower 87Sr/86Sr for pretreatment 6 for the modern tooth, could, therefore, conceivably reflect a small contribution of Sr from Ca acetate. However, these low values could also reflect successful removal of diagenetic Sr. Because we rinsed samples five times with ultrapure water after all chemical pretreatment steps, the amount of Sr remaining from Ca acetate-buffered acetic acid should have been infinitesimally small (on the order of 0.001% of the original reagent, and <<0.1 ng Sr). Low Sr concentrations for pretreatments involving buffered acetic acid also support a lack of contamination. Nevertheless, using sodium acetate-buffered acetic acid may be preferable as it presumably contains less Sr.
Concerns have also previously been raised about both NaOCl and H2O2 (e.g., Zazzo et al., 2006; Crowley and Wheatley, 2014; Pellegrini and Snoeck, 2016). Crowley and Wheatley (2014) found that carbonate content increased in enamel, dentine, and bone samples that were treated with 2%–3% NaOCl, and conjectured that this was due to the temporary formation of a secondary mineral (possibly brushite). The NaOCl that we used in the present study contained a measurable amount of Sr (Table 2); if a secondary mineral was formed and not subsequently removed by an acid step, then it could have impacted both elemental concentrations and 87Sr/86Sr. This would have been particularly concerning for the modern and Pleistocene teeth, which had little to no diagenetic minerals and had low Sr concentrations. We did observe small apparent (but statistically indistinguishable) differences in 87Sr/86Sr between pretreatments 7 (2%–3% NaOCl), and 8 (30% H2O2) for the modern and Pleistocene tooth, but Sr concentrations were similar and statistically indistinguishable (Table 3; Figure 3). Intriguingly, two replicates of pretreatment 7 for the Pleistocene tooth contained negligible U, and pretreatment 7 had significantly lower U concentrations than pretreatment 8 for the Miocene tooth. These results could reflect formation of a secondary mineral containing negligible U by NaOCl. However, they could also indicate dissolution of some mineral by H2O2, which is slightly acidic. Pellegrini and Snoeck (2016) argued that H2O2 does not effectively remove organics, and that this oxidizer has the potential to dissolve biogenic hydroxyapatite. However, other authors have argued that the acidity is actually beneficial and may help prevent adsorption of secondary minerals created during pretreatment (Crowley and Wheatley, 2014). Hydrogen peroxide may also help dissolve diagenetic oxy-hydroxides that are not removed by acetic acid (Voegelin and Hug, 2003; Rey et al., 2021). Disentangling these alternative possibilities will require further work.
Lastly, elevated Sr concentrations for pretreatment 3 (ashed sample) are not surprising given that ashing at high temperatures should be very effective at removing organics but cannot remove exogenous Sr. However, we were somewhat surprised that the differences between pretreatment 3 and other pretreatments was most pronounced for the Miocene specimen, which should have contained the least organic material, and least pronounced for the modern specimen, which should have contained the most organic material. We were also surprised that 87Sr/86Sr was apparently higher for pretreatment 3 than untreated powder (pretreatment 1) for all three teeth since removing organics from tooth enamel should not impact 87Sr/86Sr. We do not have an obvious explanation for this trend but suspect it might relate to the temperature at which the samples were ashed. While hydroxyapatite only starts to decompose above 1,000 °C, diagenetic carbonates start to break down around 750 °C (Liao et al., 1999; Karunadasa et al., 2019). If this breakdown impacted the solubility of diagenetic minerals in nitric acid, then it could, in turn, have impacted the measured 87Sr/86Sr of a sample (Sillen and Sealy, 1995). In general, both hydroxyapatite and diagenetic carbonates dissolve well in nitric acid (J.M.S. and T.M.J. personal observations). Nevertheless, it is possible that changes in the solubility of diagenetic minerals following ashing led to the release of more exogenous Sr into a sample. Elevated 87Sr/86Sr and Sr concentrations for the Miocene tooth, and apparently elevated 87Sr/86Sr, Sr concentrations, and U concentrations for the Pleistocene tooth, would thus reflect some contribution of both biogenic and diagenetic material. Comparable 87Sr/86Sr, and smaller differences in Sr concentrations between pretreatments 1 and 3 for the modern tooth support this explanation (Table 3; Figure 3). The fact that we did not observe elevated Sr concentrations for the Pleistocene or Miocene teeth for pretreatment 14, which ostensibly removes diagenetic material by including a short soak in 1 M acetic acid, further supports this possibility, although extremely elevated U concentrations for pretreatment 14 also suggest possible additional impacts of combining acetic acid and ashing.
In summary, our results suggest that sample pretreatment can influence enamel 87Sr/86Sr, but the specific impacts will depend on the sample and other variables. It is important to be aware that because of the very small analytical uncertainties, 87Sr/86Sr measurements are highly sensitive to small amounts of contamination. The variation we observed among replicate samples for the modern tooth, in particular, could have arisen from laboratory contamination. The laboratory used in this study was similar to those used by other groups for sample pretreatment (i.e., an ordinary laboratory; not a clean lab). In such a setting, small particles of common dust (e.g., calcite) may be expected to have large impacts if the amount of Sr in a sample is small, or if the dust and the sample have widely divergent 87Sr/86Sr. However, our results also show significant differences among pretreatments that were not caused by contamination. This is most evident for the high-Sr Miocene tooth, but also evident for the Pleistocene tooth. This is concerning, as small differences in 87Sr/86Sr (e.g., 0.0002), could impact data interpretations in settings where isotopic variation is small. While this is less of a concern for researchers working in isotopically heterogenous landscapes, there is more potential for cross-contamination between highly contrasting samples.
The possible impacts of sample pretreatment, and the possibility of contamination, both during sample preparation and analysis, have received little attention in Sr isotope research. We consider our study to be an important first step towards evaluating their importance. Our results suggest these factors should not be ignored, but the degree to which they matter will depend on the Sr content of a specimen, as well as burial and preservation conditions (which typically are also tied to the age of a sample). If a specimen has been buried for a long time and exposed to diagenetic carbonates and other minerals, there is the possibility of incomplete removal, exchange, or adsorption of diagenetic Sr during sample pretreatment. There is no diagenetic strontium in modern specimens, but Sr concentrations for modern material tend to be low, which may make modern specimens more susceptible to contamination from exogenous Sr during sample pretreatment.
In future work on this topic, larger sample sizes and additional specimens with known provenance would help clarify possible patterns among pretreatments. We acknowledge that we have only tested a subset of the sample pretreatment methods that are used (focusing on those chemicals and approaches most frequently referenced in the literature). There are additional reagents that we did not test, such as EDTA (Pokutta et al., 2019), sodium acetate-buffered acetic acid (e.g., Cox and Sealy, 1997), and hydrazine hydrate (Pellegrini and Snoeck, 2016). More work will be needed to confirm if any given pretreatment is universally better than another, but for now, we suggest using a method that has the least potential of causing contamination or exchange between biogenic and exogenous Sr. We also note that some pretreatments require more sample material than others, and some preclude other types of analysis (e.g., ashing requires considerably more sample than other pretreatments and also removes the possibility of analyzing C and O isotopes). Obtaining concentration data for Sr (and perhaps other elements, like U) is also a worthwhile investment. These analyses cost comparatively little and they add validity to isotopic results. We urge researchers to make sure they clearly describe their methods, including specifying reagent concentration and grade, as well as reaction temperature and duration. We also recommend avoiding combining data obtained using different pretreatment methods, as small differences in 87Sr/86Sr could impact data interpretations, especially in areas where variability in 87Sr/86Sr is low.
The original contributions presented in the study are included in the article/Supplementary Material, further inquiries can be directed to the corresponding author.
BC conceived of the project; BC, EB, and SH pretreated samples at the University of Cincinnati and JS and TJ prepared and analyzed the samples at the University of Illinois. BC, JS, and TJ analyzed the data; BC wrote the manuscript and all authors contributed editorial advice.
This project was funded by National Science Foundation Award No. BCS-1749676 (to BC).
We thank B. Hunda at the Cincinnati Museum Center, M. Campell at the Cincinnati Zoo, and M. Borths with the Division of Fossil Primates at the Duke Lemur Center for providing specimens. This is DLC Publication #1544. Thanks also to T. Lowell for use of his muffle furnace at UC, and K. Mazurkiewicz and C. Marks, for providing assistance with sample preparation and analysis at UIUC. Lastly, we thank X. Wang and the UC Statistical Consulting Center for data analysis advice.
The authors declare that the research was conducted in the absence of any commercial or financial relationships that could be construed as a potential conflict of interest.
All claims expressed in this article are solely those of the authors and do not necessarily represent those of their affiliated organizations, or those of the publisher, the editors and the reviewers. Any product that may be evaluated in this article, or claim that may be made by its manufacturer, is not guaranteed or endorsed by the publisher.
The Supplementary Material for this article can be found online at: https://www.frontiersin.org/articles/10.3389/fenvc.2023.1114807/full#supplementary-material
Alonzi, E., Daly, N., Gordon, G., Scott, R. E., and Knudson, K. J. (2019). Traveling monastic paths: Mobility and religion at medieval Irish monasteries. J. Anthropol. Archaeol. 55, 101077. doi:10.1016/j.jaa.2019.101077
Alshaaer, M., Afify, A. S., Moustapha, M. E., Hamad, N., Hammouda, G. A., and Rocha, F. (2020). Effects of the full-scale substitution of strontium for calcium on the microstructure of brushite:(CaxSr1–x) HPO4*nH2O system. Clay Miner. 55 (4), 366–374. doi:10.1180/clm.2021.5
Balasse, M., Ambrose, S. H., Smith, A. B., and Price, T. D. (2002). The seasonal mobility model for prehistoric herders in the south-Western Cape of South Africa assessed by isotopic analysis of sheep tooth enamel. J. Archaeol. Sci. 29, 917–932. doi:10.1006/jasc.2001.0787
Baumann, E., and Crowley, B. E. (2015). Stable isotopes reveal ecological differences amongst now-extinct proboscideans from the Cincinnati region, USA. Boreas 44 (1), 240–254. doi:10.1111/bor.12091
Bentley, R. A., Krause, R., Price, T. D., and Kaufmann, B. (2003). Human mobility at the early Neolithic settlement of Vaihingen, Germany: Evidence from strontium isotope analysis. Archaeometry 45 (3), 471–486. doi:10.1111/1475-4754.00122
Britton, K., Crowley, B. E., Bataille, C. P., Miller, J. H., and Wooller, M. J. (2021). Silver linings at the dawn of a “golden age”. Front. Ecol. Evol. 9, 748938. doi:10.3389/fevo.2021.748938
Budd, P., Montgomery, J., Barreiro, B., and Thomas, R. G. (2000). Differential diagenesis of strontium in archaeological human dental tissues. Appl. Geochem. 15 (5), 687–694. doi:10.1016/S0883-2927(99)00069-4
Capo, R. C., Stewart, B. W., and Chadwick, O. A. (1998). Strontium isotopes as tracers of ecosystem processes: theory and methods. Geoderma 82, 197–225. doi:10.1016/S0016-7061(97)00102-X
Christoffersen, J., Christoffersen, M. R., Kolthoff, N., and Bärenholdt, O. (1997). Effects of strontium ions on growth and dissolution of hydroxyapatite and on bone mineral detection. Bone 20 (1), 47–54. doi:10.1016/s8756-3282(96)00316-x
Cox, G., and Sealy, J. C. (1997). Investigating identity and life histories: Isotopic analysis and historical documentation of slave skeletons found on the Cape Town Foreshore, South Africa. Int. J. Hist. Archeol. 1 (3), 207–224. doi:10.1023/A:1027349115474
Crowley, B. E., and Wheatley, P. V. (2014). To bleach or not to bleach? Comparing treatment methods for isolating biogenic carbonate. Chem. Geol. 381, 234–242. doi:10.1016/j.chemgeo.2014.05.006
Esker, D., Forman, S. L., Widga, C., Walker, J. D., and Andrew, J. E. (2019). Home range of the Columbian mammoths (Mammuthus columbi) and grazing herbivores from the Waco Mammoth National Monument, (Texas, USA) based on strontium isotope ratios from tooth enamel bioapatite. Palaeogeogr. Palaeoclimatol. Palaeoecol. 534, 109291. doi:10.1016/j.palaeo.2019.109291
Evans, J. A., Chenery, C. A., and Fitzpatrick, A. P. (2006). Bronze age childhood migration of individuals near Stonehenge, revealed by strontium and oxygen isotope tooth enamel analysis. Archaeometry 48 (2), 309–321. doi:10.1111/j.1475-4754.2006.00258.x
Feranec, R. S., Hadly, E. A., and Paytan, A. (2007). Determining landscape use of Holocene mammals using strontium isotopes. Oecologia 153 (4), 943–950. doi:10.1007/s00442-007-0779-y
Garvie-Lok, S. J., Varney, T. L., and Katzenberg, M. A. (2004). Preparation of bone carbonate for stable isotope analysis: the effects of treatment time and acid concentration. J. Archaeol. Sci. 31, 763–776. doi:10.1016/j.jas.2003.10.014
Georgalis, G. L., Gawad, M. K. A., Hassan, S. M., El-Barkooky, A. N., and Hamdan, M. A. (2020). Oldest co-occurrence of Varanus and Python from africa—first record of squamates from the early Miocene of Moghra formation, western desert, Egypt. PeerJ 8, e9092. doi:10.7717/peerj.9092
Glassburn, C. L., Potter, B. A., Clark, J. L., Reuther, J. D., Bruning, D. L., and Wooller, M. J. (2018). Strontium and oxygen isotope profiles of sequentially sampled modern bison (Bison bison bison) teeth from interior alaska as proxies of seasonal mobility. Arctic 71 (2), 183–200. doi:10.14430/arctic4718
Grimstead, D. N., and Pavao-Zuckerman, B. (2016). Historical continuity in Sonoran Desert free-range ranching practices: Carbon, oxygen, and strontium isotope evidence from two 18th-century missions. J. Archaeol. Sci. Rep. 7, 37–47. doi:10.1016/j.jasrep.2016.03.009
Grupe, G., Price, T. D., Schröter, P., Söllner, F., Johnson, C. M., and Beard, B. L. (1997). Mobility of bell beaker people revealed by strontium isotope ratios of tooth and bone: a study of southern bavarian skeletal remains. Appl. Geochem. 12, 517–525. doi:10.1016/s0883-2927(97)00030-9
Hamilton, M., Nelson, S. V., Fernandez, D. P., and Hunt, K. D. (2019). Detecting riparian habitat preferences in “savanna” chimpanzees and associated fauna with strontium isotope ratios: Implications for reconstructing habitat use by the chimpanzee-human last common ancestor. Am. J. Phys. Anthropol. 170 (4), 551–564. doi:10.1002/ajpa.23932
Hassan, S. M. (2013). Sequence stratigraphy of the lower Miocene Moghra formation in the qattara depression. North Western Desert, Egypt: Springer Science & Business Media.
Hedman, K. M., Curry, B. B., Johnson, T. M., Fullagar, P. D., and Emerson, T. E. (2009). Variation in strontium isotope ratios of archaeological fauna in the midwestern United States: a preliminary study. J. Archaeol. Sci. 36, 64–73. doi:10.1016/j.jas.2008.07.009
Hodges, R. M., McArthur, C., Ezmirlian, F., MacDonald, N. S., Spain, P., Nusbaum, R., et al. (1949). The strontium content of human bones. Oak Ridge, TN: United States Atomic Energy Commission, Technical Information Division.
Hoppe, K. A., Koch, P. L., and Furutani, T. T. (2003). Assessing the preservation of biogenic strontium in fossil bones and tooth enamel. Int. J. Osteoarchaeol. 13, 20–28. doi:10.1002/oa.663
Janzen, A., Bataille, C., Copeland, S. R., Quinn, R. L., Ambrose, S. H., Reed, D., et al. (2020). Spatial variation in bioavailable strontium isotope ratios (87Sr/86Sr) in Kenya and northern Tanzania: Implications for ecology, paleoanthropology, and archaeology. Palaeogeogr. Palaeoclimatol. Palaeoecol. 560, 109957. doi:10.1016/j.palaeo.2020.109957
Karunadasa, K. S., Manoratne, C. H., Pitawala, H. M. T. G. A., and Rajapakse, R. M. G. (2019). Thermal decomposition of calcium carbonate (calcite polymorph) as examined by in-situ high-temperature X-ray powder diffraction. J. Phys. Chem. Solids 134, 21–28. doi:10.1016/j.jpcs.2019.05.023
Knudson, K. J., Price, T. D., Buikstra, J. E., and Blom, D. E. (2004). The use of strontium isotope analysis to investigate Tiwanaku migration and mortuary ritual in Bolivia and Peru. Archaeometry 46 (1), 5–18. doi:10.1111/j.1475-4754.2004.00140.x
Koch, P. L., Tuross, N., and Fogel, M. L. (1997). The effects of sample treatment and diagenesis on the isotopic integrity of carbonate in biogenic hydroxylapatite. J. Archaeol. Sci. 24, 417–429. doi:10.1006/jasc.1996.0126
Kohn, M. J., Schoeninger, M. J., and Barker, W. W. (1999). Altered states: effects of diagenesis on fossil tooth chemistry. Geochim. Cosmochim. Acta 63 (18), 2737–2747. doi:10.1016/S0016-7037(99)00208-2
Krueger, H. W., and Sullivan, C. H. (1984). “Models for carbon isotope fractionation between diet and bone,” in Stable isotopes in nutrition. Editors J. F. Turnland, and P. E. Johnson (Washington: American Chemical Society), 205–220.
Krueger, H. W. (1991). Exchange of carbon with biological apatite. J. Archaeol. Sci. 18, 355–361. doi:10.1016/0305-4403(91)90071-v
Laffoon, J. E., Davies, G. R., Hoogland, M. L. P., and Hofman, C. L. (2012). Spatial variation of biologically available strontium isotopes (87Sr/86Sr) in an archipelagic setting: A case study from the Caribbean. J. Archaeol. Sci. 39, 2371–2384. doi:10.1016/j.jas.2012.02.002
Laffoon, J. E., Hoogland, M. L., Davies, G. R., and Hofman, C. L. (2019). A multi-isotope investigation of human and dog mobility and diet in the pre-colonial Antilles. Environ. Archaeol. 24 (2), 132–148. doi:10.1080/14614103.2017.1322831
Lambert, J., Weydert, J., Williams, S., and Buikstra, J. (1990). Comparison of methods for the removal of diagenetic material in buried bone. J. Archaeol. Sci. 17, 453–468. doi:10.1016/0305-4403(90)90008-S
Lee-Thorp, J. A., and van der Merwe, N. J. (1991). Aspects of the chemistry of modern and fossil biological apatites. J. Archaeol. Sci. 18, 343–354. doi:10.1016/0305-4403(91)90070-6
Liao, C. J., Lin, F. H., Chen, K. S., and Sun, J. S. (1999). Thermal decomposition and reconstruction of hydroxyapatite in air atmosphere. Biomed. Sci. Instrum. 35, 99–104.
Littlewood, J. L., Shaw, S., Peacock, C. L., Bots, P., Trivedi, D., and Burke, I. T. (2017). Mechanism of enhanced strontium uptake into calcite via an amorphous calcium carbonate crystallization pathway. Cryst. Growth & Des. 17 (3), 1214–1223. doi:10.1021/acs.cgd.6b01599
Miller, J. H., Fisher, D. C., Crowley, B. E., Secord, R., and Konomi, B. A. (2022). Male mastodon landscape use changed with maturation (late Pleistocene, North America). Proc. Natl. Acad. Sci. 119 (25), 32118329119. doi:10.1073/pnas.2118329119
Moore, J., Rose, A., Anderson, S., Evans, J., Nowell, G., Gröcke, D. R., et al. (2020). A multi-isotope (C, N, O, Sr, Pb) study of Iron Age and Roman period skeletons from east Edinburgh, Scotland exploring the relationship between decapitation burials and geographical origins. J. Archaeol. Sci. Rep. 29, 102075. doi:10.1016/j.jasrep.2019.102075
Nelson, B. K., DeNiro, M. J., Schoeninger, M. J., De Paolo, D. J., and Hare, P. (1986). Effects of diagenesis on strontium, carbon, nitrogen and oxygen concentration and isotopic composition of bone. Geochim. Cosmochim. Acta 50, 1941–1949. doi:10.1016/0016-7037(86)90250-4
Pellegrini, M., and Snoeck, C. (2016). Comparing bioapatite carbonate pre-treatments for isotopic measurements: Part 2 — impact on carbon and oxygen isotope compositions. Chem. Geol. 420, 88–96. doi:10.1016/j.chemgeo.2015.10.038
Pokutta, D. A., Borodovskiy, A. P., Oleszczak, Ł., Tóth, P., and Lidén, K. (2019). Mobility of nomads in central asia: Chronology and 87 Sr/86 Sr isotope evidence from the pazyryk barrows of northern altai, Russia. J. Archaeol. Sci. Rep. 27, 101897. doi:10.1016/j.jasrep.2019.101897
Price, T. D., Blitz, J., Burton, J., and Ezzo, J. A. (1992). Diagenesis in prehistoric bone: Problems and solutions. J. Archaeol. Sci. 19 (5), 513–529. doi:10.1016/0305-4403(92)90026-Y
Price, T. D., Manzanilla, L., and Middleton, W. D. (2000). Immigration and the ancient city of teotihuacan in Mexico: A study using strontium isotope ratios in human bone and teeth. J. Archaeol. Sci. 27 (10), 903–913. doi:10.1006/jasc.1999.0504
Rey, L., Tacail, T., Santos, F., Rottier, S., Goude, G., and Balter, V. (2021). Disentangling diagenetic and biogenic trace elements and Sr radiogenic isotopes in fossil dental enamel using laser ablation analysis. Chem. Geol. In Press. doi:10.1016/j.chemgeo.2021.120608
Rogers, B., Gron, K. J., Montgomery, J., Rowley-Conwy, P., Nowell, G., Peterkin, J., et al. (2019). Isotopic analysis of the blick mead dog: A proxy for the dietary reconstruction and mobility of mesolithic British hunter-gatherers. J. Archaeol. Sci. Rep. 24, 712–720. doi:10.1016/j.jasrep.2019.02.022
Rokita, E., Hermes, C., Nolting, H. F., and Ryczek, J. (1993). Substitution of calcium by strontium within selected calcium phosphates. J. Cryst. Growth 130 (3-4), 543–552. doi:10.1016/0022-0248(93)90543-6
Schwartz, C. W., Somerville, A. D., Nelson, B. A., and Knudson, K. J. (2021). Investigating pre-Hispanic scarlet macaw origins through radiogenic strontium isotope analysis at Paquimé in Chihuahua, Mexico. J. Anthropol. Archaeol. 61, 101256. doi:10.1016/j.jaa.2020.101256
Sealy, J. C., van der Merwe, N. J., Sillen, A., Kruger, F. J., and Krueger, H. W. (1991). 87Sr/86Sr as a dietary indicator in modern and archaeological bone. J. Archaeol. Sci. 18 (3), 399–416. doi:10.1016/0305-4403(91)90074-y
Sillen, A., and Sealy, J. C. (1995). Diagenesis of strontium in fossil bone: a reconsideration of nelsonet al. (1986). J. Archaeol. Sci. 22 (2), 313–320. doi:10.1006/jasc.1995.0033
Sillen, A. (1986). Biogenic and diagenetic Sr/Ca in plio-pleistocene fossils of the omo shungura formation. Paleobiology 12 (3), 311–323. doi:10.1017/s0094837300013816
Simpson, E. M. B., Crowley, B. E., and Sturmer, D. M. (2023). Is the damage worth it? Testing handheld XRF as a non-destructive analytical tool for determining biogenic bone and tooth chemistry prior to destructive analyses. Front. Environ. Archaeol. 1. doi:10.3389/fearc.2022.1098403
Slater, P. A., Hedman, K. M., and Emerson, T. E. (2014). Immigrants at the mississippian polity of cahokia: strontium isotope evidence for population movement. J. Archaeol. Sci. 44, 117–127. doi:10.1016/j.jas.2014.01.022
Snoeck, C., and Pellegrini, M. (2015). Comparing bioapatite carbonate pre-treatments for isotopic measurements: Part 1—impact on structure and chemical composition. Chem. Geol. 417, 394–403. doi:10.1016/j.chemgeo.2015.10.004
Trickett, M., Budd, P., Montgomery, J., and Evans, J. (2003). An assessment of solubility profiling as a decontamination procedure for the 87Sr/86Sr analysis of archaeological human skeletal tissue. Appl. Geochem. 18, 653–658. doi:10.1016/S0883-2927(02)00181-6
Turekian, K., and Kulp, J. (1956). Strontium content of human bones. Science 124 (3218), 405–407. doi:10.1126/science.124.3218.405.b
Tuross, N., Behrensmeyer, A. K., and Eanes, E. D. (1989). Strontium increases and crystallinity changes in taphonomic and archaeological bone. J. Archaeol. Sci. 16, 661–672. doi:10.1016/0305-4403(89)90030-7
Voegelin, A., and Hug, S. J. (2003). Catalyzed oxidation of arsenic (III) by hydrogen peroxide on the surface of ferrihydrite: an in situ ATR-FTIR study. Environ. Sci. Technol. 37 (5), 972–978. doi:10.1021/es025845k
Wathen, A. C., Isaksson, S., and Lidén, K. (2022). On the road again—a review of pretreatment methods for the decontamination of skeletal materials for strontium isotopic and concentration analysis. Archaeol. Anthropol. Sci. 14 (45), 45. doi:10.1007/s12520-022-01517-2
Widga, C., Walker, J. D., and Stockii, L. D. (2010). Middle Holocene bison diet and mobility in the eastern Great Plains (USA) based on δ13C, δ18O, and 87Sr/86Sr analyses of tooth enamel carbonate. Quat. Res. 73, 449–463. doi:10.1016/j.yqres.2009.12.001
Widga, C., Hodgins, G., Kolis, K., Lengyel, S., Saunders, J., Walker, J., et al. (2021). Life histories and niche dynamics in late Quaternary proboscideans from midwestern North America. Quat. Res. 100, 224–239. doi:10.1017/qua.2020.85
Yoder, C. J., and Bartelink, E. J. (2010). Effects of different sample preparation methods on stable carbon and oxygen isotope values of bone apatite: a comparison of two treatment protocols. Archaeometry 52 (1), 115–130. doi:10.1111/j.1475-4754.2009.00473.x
Keywords: diagenenesis, acetic acid, hydroxyapatite, sodium hyplochlorite, hydrogen peroxide, MC-ICPMS, proboscidean
Citation: Crowley BE, Bruff Simpson EM, Hammer SJ, Smith JM and Johnson TM (2023) Comparison of powdered enamel sample pretreatment methods for strontium isotope analysis. Front. Environ. Chem. 4:1114807. doi: 10.3389/fenvc.2023.1114807
Received: 02 December 2022; Accepted: 17 February 2023;
Published: 02 March 2023.
Edited by:
Lorenzo Antonio Picos Corrales, Universidad Autónoma de Sinaloa, MexicoReviewed by:
Maciej Tomasz Krajcarz, Institute of Geological Sciences (Polska Akademia Nauk—PAN), PolandCopyright © 2023 Crowley, Bruff Simpson, Hammer, Smith and Johnson. This is an open-access article distributed under the terms of the Creative Commons Attribution License (CC BY). The use, distribution or reproduction in other forums is permitted, provided the original author(s) and the copyright owner(s) are credited and that the original publication in this journal is cited, in accordance with accepted academic practice. No use, distribution or reproduction is permitted which does not comply with these terms.
*Correspondence: Brooke Erin Crowley, YnJvb2tlLmNyb3dsZXlAdWMuZWR1
Disclaimer: All claims expressed in this article are solely those of the authors and do not necessarily represent those of their affiliated organizations, or those of the publisher, the editors and the reviewers. Any product that may be evaluated in this article or claim that may be made by its manufacturer is not guaranteed or endorsed by the publisher.
Research integrity at Frontiers
Learn more about the work of our research integrity team to safeguard the quality of each article we publish.