- 1Department of Marine Sciences, Kristineberg Marine Research Station, University of Gothenburg, Fiskebäckskil, Sweden
- 2Department of Geology and Geoenvironment, University of Athens, Athens, Greece
Microplastics in the marine environment have been the focus of intense research recently, however little attention has been given to boat paint sources, despite its direct influence on the marine ecosystem. This is largely due to the lack of established analytical methods. Microplastics from boating sources may originate from antifouling paints on the underwater body, surface coatings on the top sides, deck, and superstructure, as well as plastic parts of the boat construction. Their release can occur during construction, operation (leisure boats and commercial ships), service, and maintenance, from the materials themselves or used chemicals (e.g., abrasive detergents). Most importantly, boat paint microplastics containing biocides, such as the metals copper and zinc, and particles containing tin (residues from old or current use of tributyl-tin ship hull paints) should raise higher concern on potential environmental impacts. This study aims to provide practical insight on methods for the quantification of boat paint microplastics in marine waters and provide a baseline survey on their occurrence. Sampling and analysis methods are applied on case studies, i.e., marinas on the Swedish coast. A multi-method approach for identifying and characterizing boat paint microplastics based on visual and chemical characteristics is presented. In general, the measured content of biocide-containing microplastics was remarkably high in all marinas, with concentration levels of copper-rich particles >10 μm between 400 and 1400 particles per L. Given that biocide paint particles are manufactured to be toxic, it is particularly important to take into account field measurements in future environmental status assessments. This work underlines the importance of monitoring data in the action work between relevant authorities and stakeholders.
Introduction
Microplastics is a form of solid pollution that has caused high concern, especially but not exclusively in the marine environment, because of its ubiquitous occurrence and distribution, and size distributions overlapping with that of natural particles. Since it has been proposed that microplastics pose a potential hazard and impact on aquatic ecosystems this has spurred intense research on potential physical and chemical effects (Takahashi et al., 2012; Galloway et al., 2017; Corinaldesi et al., 2021). Microplastic particles are however made up of a large variety of chemical compositions (polymers, additives and other ingredients), and physical forms and sizes (Hartmann et al., 2019). In an effort to address this issue, recent regulations target microplastics that are intentionally produced components of consumer products, such as cosmetics, personal care products, detergents, etc., thus aiming to limit their release in the environment (Comitology Register, 2021). However, unintentionally produced microplastics resulting from the degradation of larger plastics are much more difficult to regulate, because the use of polymers is widely intertwined with the global economy. Degradation-produced microplastics are the majority of microplastics polluting the environment and although toxicological studies are not always conclusive about the risk posed by various types, microplastics from paints, tyres, and fabrics are considered the main sources of potentially hazardous microplastics. Antifouling paint particles in particular, are likely to pose a substantial risk to marine ecosystems, since antifouling paint is manufactured to be toxic in order to prohibit biological growth on the ship’s hull. Paint comprises largely of carbon polymers and its contribution to the global plastic pollution has been largely overlooked; of an estimated 7.4 Mt paint leakage in the environment, 63% is microplastics and 37% is released in oceans and waterways, making paint the largest source of microplastics in the oceans (Gaylarde et al., 2021; Muller-Karanassos et al., 2021; Turner, 2021; Paruta et al., 2022). It is therefore imperative to develop analytical methods to detect and characterize paint microplastics in the marine environment.
Paint microplastics are generated from environmental weathering, maintenance, or decommissioning operations, and can come from both land-based (houses, buildings, constructions), and maritime sources (leisure boats, ships, wrecks, and maritime constructions). In coastal waters, leisure boats and commercial ships are likely dominating sources (Paruta et al., 2022). There are many different formulations for paint, varnish and coatings used on boats and ships, depending on their construction material and use profile. The most common material for leisure boat production is glass fibre reinforced polyester composites (GRP) (Graner, 1982) that in itself can generate microplastics, and is often coated with a cross linked polyester paint (gel coat). Metal boats and ships are typically protected by various epoxy coatings, while wooden boats are often applied with varnish or paint (alkyde or polyurethane based). On the topsides, outside the epoxy paint for appearance and protection different hardened polyurethane (PU) topcoats are often applied. On underwater surfaces some kind of antifouling (AFC) or foul release coatings (FRC) are often applied over the protective coatings. The antifouling paints typically contain biocide ingredients, which per definition are designed to be harmful, not necessarily to non-target fouling species, but with well documented such side effects, e.g., (Karlsson et al., 2010; Muller-Karanassos et al., 2021). That makes antifouling paint particles key microplastic sources to be studied for occurrence, transport, fate, and effects. There are some rare exceptions, e.g., glass flake reinforced polyester, so called ice breaker paint, or other non-biocidal containing hard paints intended for mechanical cleaning, as well as different silicone or silane based, and fluoropolymer containing FRC, that are intended for release of the biofouling agent while in motion through water or by mechanical cleaning (Watermann and Eklund, 2019). For commercial ships, there are permits given in designated areas where underwater cleaning operations are conducted by divers or robots (Song and Cui, 2020), and for leisure boats there are increasing numbers of underwater boat washing stations built in harbors around the world. These underwater brushing operations are clearly generating high amounts of paint particles while removing the hard and soft biofouling coating, and therefore regulating authorities need to pay close attention to what kind of coatings are allowed to be cleaned, and which containment measures and efficacy this equipment may have (Scianni and Georgiades, 2019).
In Sweden there is a very high number of leisure boats per capita, with close to one million leisure boats and according to the Swedish transport agency 700.000 L of boat bottom paint of different types are applied annually (Transportstyrelsens Rapport Båtlivsundersökningen 2020, 2021). The most common biocidal ingredients are different copper-based substances, primarily cuprous oxide (Cu2O), and copper thiocyanate (CuSCN), in a micronized powder form in the formulations. The biocide tributyl tin (TBT) was a very common AFC biocide for many years, even long after it was discovered to have severe side effects, especially on marine snails (Sousa et al., 2014) and is still sold in some markets (Uc-Peraza et al., 2022). After its gradual ban in the nineties and early two thousand there was a number of other biocides introduced, such as cuprous oxide, and a large number of organic biocides. TBT is still a priority pollutant in the water framework directive of large concern in many harbors and coastal sediments, and boat maintenance and storage areas on land (EC report SWD (2019) 439 final). TBT can be resuspended by dredging, boat traffic or construction work, and additionally there are still boats with old TBT paint layers under newer AFC applications that can be mobilized, therefore this study also paid attention to tin containing particles. In Europe, the active biocidal ingredients are regulated under the biocide directive, and implemented with national law by respective competent authority, which in this case is the Swedish Chemical Agency, KEMI. The use of boats and service maintenance give rise to biocide accumulation, and paint particles in harbor sediments, on boat service and storage areas (Fauser et al., 2019; Fauser et al., 2022; Turner, 2022; Turner et al., 2022). However, the understanding of generation, spread, transport and fate of boat paint particles, have received very little attention, despite that some of those may be the most toxic of microplastics types due to the biocide composition. This is partly due to lack of established measurement methods.
The aim of this study was twofold; i) to investigate the fitness for purpose of conventional particle analysis methods for the detection and identification of maritime paint microplastics, with focus on AFC and ii) with these methods estimate a baseline study on leisure boat harbors on the Kattegat Sea and Baltic Sea coasts of Sweden.
Microplastics research is a relatively new scientific field and several variations and discrepancies have surfaced as a result of scientists from different backgrounds working on this transdisciplinary topic. A typical example is the definition of microplastics in terms of composition and physical properties. Since the study presented here is one of the very early studies on the quantification of paint microplastics in the marine environment, the definition of target materials follows the definition and categorization framework proposed by (Hartmann et al., 2019) (Figure 1). Paint microplastics are solid composites with a synthetic polymer as an essential ingredient, that are either insoluble in seawater or exhibit slow solubility that allows them to persist for months or years. They occur as nanoplastics (1–1,000 nm), microplastics (1–1,000 µm), and mesoplastics (1–10 mm) with irregular shape and various colors. Hartmann et al. acknowledge paint particles as plastics presenting them as a special case due to the lack of a threshold on the polymer content that would allow straightforward categorization as a plastic. Nevertheless, paint particles are currently widely accepted as an integral part of the global microplastics pollution challenge and one of its’ most hazardous and overlooked aspect (IMO, 2019; Gaylarde et al., 2021; Torres and De-la-Torre, 2021; Turner, 2021; Paruta et al., 2022).
Given the wide use of antifouling paints from large ships to small boats and building facades, it is indeed interesting how little is known about the distribution and fate of paint microplastics in the marine environment. This is partly due to the analytical challenges posed by the currently available analytical methods and physical-chemical properties of the particles, which hamper monitoring efforts of paint microplastics (Mattsson et al., 2021a). Here, a novel analytical method is used to quantify and characterize these particles in a broad size range, i.e., from 0.1 µm to several millimeters. In order to identify target particles from other anthropogenic or natural particles, two parameters were used: visual characteristics and metal content. In the first case, colors blue and red are used to identify paint microplastics, due to the scarcity of naturally occurring particles of the same color. Brown color in combination with shape is used as an additional handle. In the second case, metal content of copper, zinc, or tin are used, due to their use (current and past) in antifouling paints. Finally, we discuss challenges and possibilities of other methods and present the case for a correlative microscopy approach that offers a holistic characterization methodology.
Materials and methods
Description of sampling sites
Sampling was carried out in six marinas (Supplementary Figure S1; Table 1), two on the west coast of Sweden (Smögen and Stenungsund), two in Öresund strait between Sweden and Denmark (Råå and Limhamn), and two on the east coast of Sweden (Bosön and Fisksätra). In addition to covering geographical locations and seawater of different composition (Skagerak Sea, Öresund strait, and Baltic Sea), these marinas were selected to include differences in regional regulations regarding use and services on coastal waters. Maintenance activities were offered in these marinas, however, sampling took place early in the morning, before such works started. The number of berths in each marina (Table 1) is expected to impact the amounts of paint released in the seawater, but also local weather conditions, water currents, and daily activities are likely influencing parameters.
Sampling methods and controls
In order to cover a wide range of particle sizes, the following sampling techniques were used.
· Manta trawl, integrating about 100 m in each direction to 1,000 m total length (particle size >300 μm, surface water)
· Water samples filtered with vacuum pump (particle size >10 μm, 0.5 m depth)
· Collection of filtrates through 10 µm membrane to analyze for copper nanoparticles (particle size 0.1–10 μm, 0.5 m depth)
Sampling was first conducted using a manta trawl (Supplementary Figure S2), with a mesh size of 300 μm. In order to be able to statistically sample large particles (>300 μm), a manta trawl was used and dragged alongside a small-size motorboat with outboard cruising at 1-2 knots. In order to avoid possible contamination from the boat used for sampling, the manta sampler was diverted 2 m out from the boat with a boom on the side and outside the wake of the boat. The distance trawled in each direction was measured in advance from aerial photography, and the trawling was repeated so that 1,000 m was covered at each sampling location. This corresponds to about 65 m3 sample volume on each occasion passing through the 300 μm mesh. As the trawl samples had a lot of natural organic matter (both marine and terrestrial) that made direct visual analysis impossible, which is usually possible in other periods of the year and/or further out in open sea, it became necessary to develop a pre-processing method. First, a mild chemical degradation method was tested on pump-filtered plankton samples where both efficiency on decomposition of organic matter and non-destructive impact on plastics and paint flakes were investigated. Hydrogen peroxide (30%) was effective for the decomposition of natural organic matter, but at the same time bleached the paint flakes. Therefore, a method used for the decomposition of fish for microplastic analysis (Enders et al., 2017) was tested, and it was found to be both effective on marine plankton and not affecting the tested plastic particles. However, it turned out that the trawl samples were not degraded as efficiently due to the high presence of plant seeds, and other terrestrial organic matter. Therefore, the degradation treatment was also supplemented by a density separation where the sample was treated with a heavy liquid (zinc chloride solution) that causes the natural organic material to float while the very heavy paint flakes and small seashell residues sink (Mattsson et al., 2022). Then the trawl filtration samples were filtered on a new 100 µm mesh filter for further microscope analysis.
Water samples were taken at a depth of 0.5 m using Ruttner collectors for filtration through 10 µm pore size membranes and the filtrate was collected for analysis of metal-containing particles. Filtration was done directly from the water sampler, on a pre-assembled setup with 10 μm filters (Supplementary Figure S3) and the sample was initially gravity filtered. The first 200 mL filtrate volume was collected into acid-washed plastic bottles for analysis of copper-containing particles in solution with spICPMS. The filter was then connected to a vacuum pump and approx. 700 mL were additionally filtered (volume measured) before rinsing with ultrapure water. The sample volume collected was much lower than with the manta trawl and contained insignificant amounts of marine and terrestrial organic matter, thus further processing was not necessary in this case.
Analysis methods and controls
Different methods of analysis were applied to the different sample methods, and include visual counting of larger color flakes, automated counting of colored microparticles, automated scanning electron microscopic element-specific particle analysis, primarily for the identification of copper, zinc, and tin-rich particles. In addition, the water sample filtrates (<10 μm) were analyzed for copper-containing nanoparticles.
§ visual identification of particles >300 μm with light microscopy (LM)
Filters from the trawl samples were visually identified under a light microscope (Zeiss AxioImager M2), photographed and classified into morphological classes (color, shape, size, etc.) following Karlsson et al. (Karlsson et al., 2020). One image field at a time was acquired, if any particle existed, a photo was taken, then each particle was measured and classified into the color and size classes.
§ visual identification of particles >10 μm with LM
Particles sampled on 10 μm filters were visually identified using an automated method with light microscopy (Zeiss AxioImager M2, darkfield, 20 × lens). First, the entire filter was imaged and then analyzed with AxioVison Particle Analyzer for red, blue, brown, as well as other color particles revealing each particle’s size in combination with their color.
§ automated scanning electron microscopy (SEM) with X-ray spectroscopic (EDX) element analysis
SEM with EDX element analysis is a well-established method for identifying and characterizing particles. Here, SEM (Sigma, Carl Zeiss Microscopy GmbH) was used with EDX (Xflash 6|100, Bruker Nano GmbH) analysis, applying an automated method with Smart Particle Investigator (SmartPI) by Carl Zeiss. Analysis parameters were EHT: 20 kV, Aperture: 60, Variable Pressure: 40 Pa, Brightness: 45.1, Contrast 21.9, Scan Speed: 7, Magnification: 325, Pixel Size: 1.145 µm, Field Overlap: 5%. The sample area was divided in 1,341 square fields (Supplementary Figure S3) and an automated calibration of the Brightness was scheduled every 400 fields. Particles that were partly detected on the edge of a field with a minimum size of 1 μm2 were stitched together as part of the automated procedure. The backscattered electron detection (BSD) was used, which has higher contrast for heavier elements (Hassellöv and Kaegi, 2009). It is thus possible to select which particles are analyzed based on their elemental contrast. Here, the detection threshold was first adjusted on an Aluminum-rich particle. Next, a small area was scanned using various threshold values (Supplementary Figure S4) and threshold 150 was selected for further analysis, based on the number of copper-rich particles detected, fraction of copper in the total inorganic content, and time efficiency. Thus, priority is given to particles with high copper content, avoiding particles with traces of copper that may also occur naturally. Using these parameters, no time is spent on measuring plankton particles and lighter minerals, but only the ones containing heavier elements. The selected particles are then automatically analyzed for element content and morphological parameters (size, shape). The method is based on an automated scanning of the filter without operator bias, which guarantees both objectivity and good statistical data in the analysis, as hundreds of thousands of particles were analyzed in this study. After sample analysis, all particles were sorted first based on copper, zinc, or tin content and selected particles were then mapped manually for elemental content (ESPRIT software, Bruker). For correlative microscopy with LM and SEM-EDX, the ZenCore software (Zeiss) was used with the instruments described above.
§ single particle ICPMS
Copper-rich particles were detected and their number concentration (number per L) and size (from the mass of copper in the particle) quantified, using an ICP-MS on single-particle mode (Tuoriniemi, 2013). A NexIon 350D (PerkinElmer, Inc.) was used to measure 63Cu, with dwell time 5 ms and measurement time per sample of 180 s. Samples were diluted 20 times in ultra-pure water less than 1 min prior to analysis, in order to reduce the total dissolved solids of seawater. Quantification was done using certified element standards of copper and gold. The transport efficiency was calculated (3.55%) using standard gold nanoparticles with nominal size 30 nm (PerkinElmer). A rinsing procedure consisting of sequential wash with solutions 1% nitric acid, mixture of 2% hydrochloric and 1% nitric acid, and then ultra-pure water was applied between samples. Blanks containing only ultrapure water were analyzed between samples to check for carry-over. Using this method, only one element can be measured per particle; copper was selected due to its’ high content in paint, low background, and established methods with spICPMS (Navratilova et al., 2015).
Results
The first procedure applied was a combination of sampling for large microplastics (>300 µm) and mesoplastics and an automated light microscopy analysis. Manta trawl samples were analyzed using an automated light microscopy procedure, which involves scanning the whole filter area, identifying particles based on color contrast, and recording color, size, and shape. Examples of blue, red, and brown particles detected are shown in Figure 2. Red and blue particles are likely boat paint particles, because these colors do not occur naturally and their shape resembles paint flakes. Additional colors, such as white and black were identified in significant amounts, however it was not possible to distinguish between anthropogenic and natural sources based on these colors. Occasional yellow particles were also identified. Further analysis was conducted on the readily distinguishable fraction, i.e., blue, red, and brown particles.
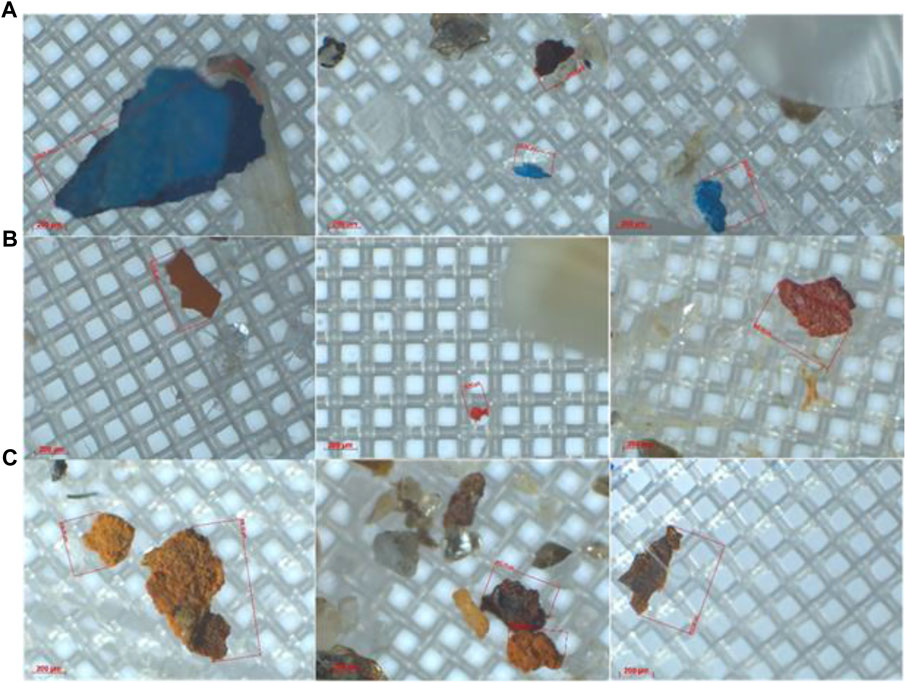
FIGURE 2. Examples of blue (A), red (C), and brown (B) particles detected with LM in manta trawl samples.
Total number concentrations ranged from 0.1 to 4.4 particles per cubic meter and the size distribution exhibited one peak at 0.2–0.3 mm (maximum dimension) and in some cases a second peak at 0.5–0.7 mm ( Figure 3A). Seawater from Eastern marinas contain a variety of particles, due to the high levels of suspended particles and organic matter in the Baltic Sea, compared to the clearer waters of the Kattegat Sea. There is no clear trend between marinas located on the East or West coast, or the Öresund strait, however, an increased variability is observed for the East locations (Bosön and Fisksätra), while higher concentrations were observed in the Öresund strait locations (Limhamn and Råå). A factor that may explain the amounts of boat paint particles released in the seawater is the size of the marina, expressed as the maximum number of boats that it may serve (number of berths). Indeed, the number concentration of color particles increases with the number of available berths in the marinas (Figure 3B), albeit with large variability. Correlation coefficients for number of berths with blue, red, and brown particles are 0.64, 0.58, and 0.39, respectively. The exact number of boats docked in the marinas, local currents, and winds are expected to influence these trends.
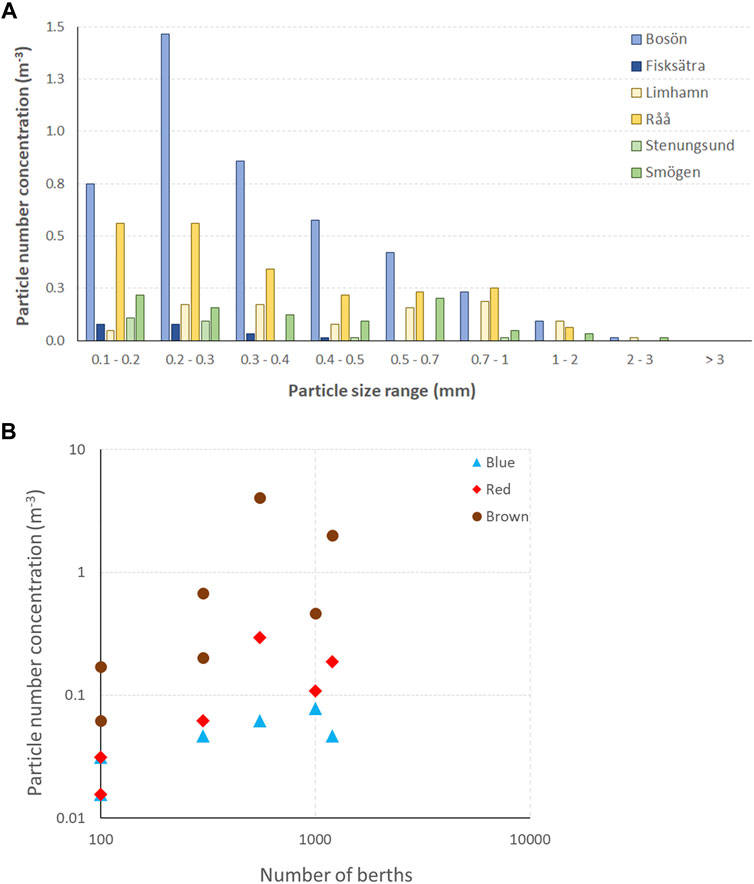
FIGURE 3. (A): Size distribution of particles collected with manta trawl (300 µm) and detected with automated LM method. (B): Particle number concentration plotted against the number of berths in the marinas sampled (axes are plotted on log scale).
The detection and characterization of particles becomes more challenging with decreasing particle size; in addition, number concentrations increase with decreasing particle size (Mattsson et al., 2021b). Thus, the second procedure applied was more complicated and targeted small microplastics and nanoplastics. Seawater collected in the marinas was immediately filtered on membranes with 10 µm pore size, collecting both the membrane and the filtrate for further analysis. The filter was processed by two automated methods: a procedure with light microscopy utilizing color and shape for identification (similar to the manta trawl samples) and a procedure with SEM-EDX, utilizing metal content to distinguish boat paint particles. The filtrate was further analyzed for copper content using spICPMS, targeting boat paint nanoplastics.
Several blue and red particles were detected using the automated light microscopy method (Figure 4). Yellow, black, and brown particles were also detected, but not recorded due to the scarcity or ambiguity of their origin (natural or anthropogenic). Significantly more particles were detected in this smaller size range. Total number concentrations ranged from 9 to 42 thousand particles per cubic meter with monomodal or bimodal size distributions (Figure 5). Samples in the Öresund strait exhibited higher concentrations of blue and red particles, consistent with the number of berths in these marinas. These data indicate that boat paint particles are released in substantial numbers in marinas. However, color alone is not a sufficient source indicator and compositional information is also necessary.
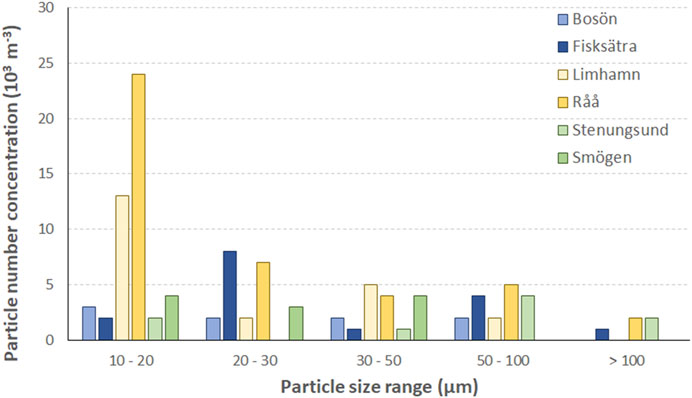
FIGURE 5. Size distribution of particles collected with Ruttner sampler, filtered (10 µm), and detected with automated LM method.
An automated SEM-EDX method was applied to identify particles containing metals that are common in antifouling or hull paints, such as copper, zinc, or tin. Tributyl tin (TBT) has been banned from boat paints but may persist in the harbors from contaminated land runoff, or from sanding or blasting of older paint layers during boat maintenance operations (Turner et al., 2015). Copper and zinc are common constituents of natural particles. In order to distinguish boat paint particles from such natural particles, a meta-analysis was conducted on the SEM-EDX data, counting only particles containing copper and zinc, both higher than 5% of the total inorganic content of the particle (since polycarbonate membranes were used, carbon was not included in the recorded compositional data). The 5% threshold was considered high enough to exclude most Cu-containing organic matter and low enough to include boat paint microplastics that have aggregated with suspended particles or decreased metal content due to leaching. A particle maximum Feret diameter higher than the 10 µm pore size of the membranes was added as a criterion, to improve the quantitative analysis. Results showed an increasing trend of both red/blue particles and copper/zinc containing particles with increasing number of berths in the marina (Figure 6), providing further evidence of the release of boat paint microplastics in the seawater. Correlation coefficients for number of berths with blue/red and Cu/Zn/Sn containing particles are 0.86 and 0.05, respectively, indicating that other anthropogenic sources of metal-containing particles may reduce specificity of the SEM-EDX method. Particles containing tin were also detected scattered and in small amounts (Supplementary Figure S5). Daily and weekly variations occur, due to local conditions and visiting boats, which was demonstrated in Fisksätra, where two samples were collected 1 day apart and in Stenungsund, where two samples were collected 1 week apart (Figure 6; Supplementary Figure S5).
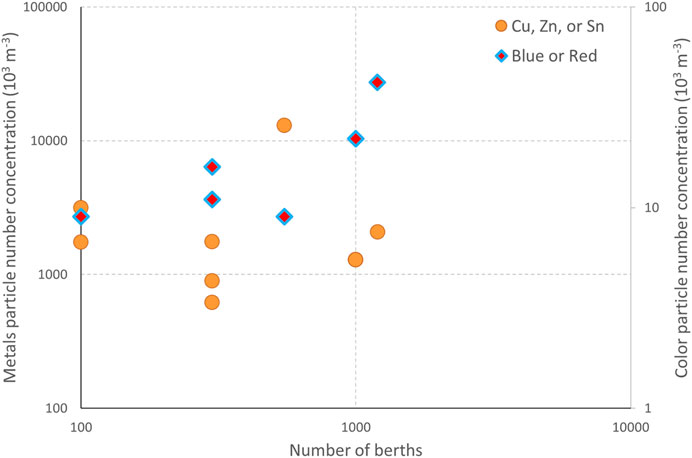
FIGURE 6. Number concentration of the sum of particles containing copper, zinc, or tin above the threshold (5% of the inorganic content) are plotted along the sum of blue and red particles in samples collected with Ruttner sampler, filtered (10 µm), and detected with automated SEM-EDX and LM methods.
Beyond state of the art
The development of automated methods has enabled the analysis of the whole membrane surface, thus overcoming the limitation of analyzing only part of a membrane by time-consuming manual inspection methods, which also hampers extrapolation of results to the whole sample. Thus, the results presented justify the use of automated LM or SEM-EDX methods for monitoring the release of boat paint microplastics close to point sources, e.g., in-water boat washing, where the visual and compositional properties can be determined in advance, e.g., taking samples from the boat prior to washing to establish target properties. When monitoring boat paint microplastics collectively released by boats, it is obvious that a single approach based on visual appearance (color selection) or elemental composition (other sources of metal-containing particles) is not sufficient to identify boat paint microplastics in the marine environment and complementary information from multiple analytical techniques is necessary. In this study, analytical methods were applied separately on the whole particle population of a sample, using state-of-the-art instrumentation. Advancing beyond the state-of-the-art, the application of multiple analytical methods on a single particle basis (i.e., characterizing the same particle with different instruments) offers clear advantages and the way forward in terms of analysis. This work is in progress both from scientists and instrument manufacturers. Here, we demonstrate the applicability of a correlative microscopy approach on selected samples. Scanning the sample with SEM-EDX, we were able to identify particles enriched in copper and zinc, which were then reprocessed to produce elemental maps (Figures 7A–C). Using appropriate sample holders that allow matching coordinates between the SEM and LM, these particles were analyzed for color using LM (Figure 7D). The example shown in Figure 7 shows a blue paint particle with high copper and zinc content. The inverse approach, i.e., first categorizing particles based on color with LM and then acquiring compositional information with SEM-EDX (e.g., Supplementary Figures S6, S7) may also prove advantageous, especially considering the beam damage on the carbon-rich membranes from elemental mapping procedure, which may interfere with color analysis in LM (see yellow background on Figure 7D).
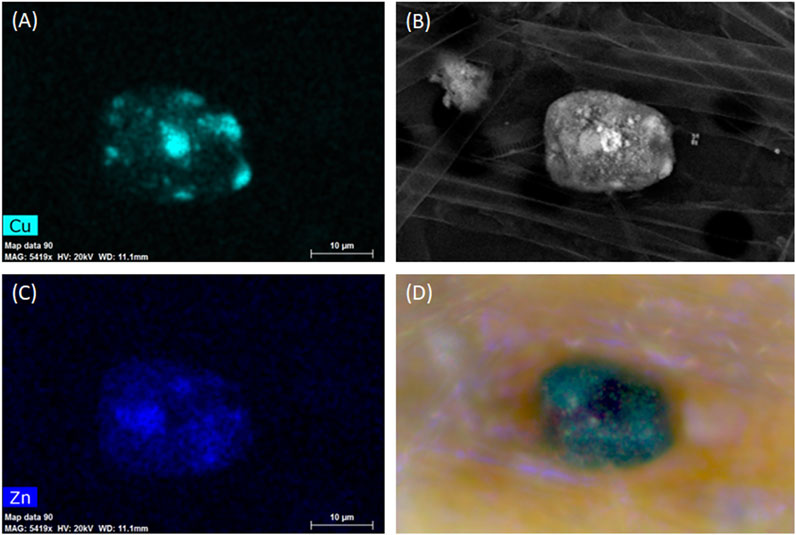
FIGURE 7. A blue particle, as determined by LM (D) with high zinc and copper content, measured with SEM-EDX (B) and shown in elemental maps (A,C), is an example of the correlative microscopy approach using first SEM-EDX with elemental mapping for elemental content and LM for color identification.
Analysis of particles in the nanoscale is challenging with SEM-EDX and impossible with LM automated methods. Using spICPMS, copper content was used as a criterion to detect particles smaller than 10 µm passing through the membrane. Number concentrations of copper-containing nanoparticles in the order of millions per cubic meter were detected (Supplementary Figure S6). Only one element can be measured with this method, thus several assumptions need to be made to derive the particle size. As an indication of particle size, the particle size distribution was derived from the measured mass of copper per particle, assuming spherical shape and copper oxide mineral structure. These assumptions are a simplification of the true composition and shape of particles in seawater and include both anthropogenic and naturally occurring particles. The majority of particles detected with this procedure correspond to equivalent particle sizes of 45–100 nm (Supplementary Figure S6).
Discussion
The methods used in this study target boat paint particles, using color, shape, and metal content to distinguish them from other suspended matter. Since number concentration increases logarithmically as particle size decreases, two sampling methods were successfully applied. A manta trawl for sampling large volumes of seawater targeting particles larger than 300 μm, which are more scarce and a filtration setup for sampling smaller volumes and targeting particles larger than 10 μm, with higher abundance. Particles smaller than 10 µm also were detected in the filtrate. These sampling methods were designed to facilitate analysis with LM, SEM-EDX, and spICPMS. Using color as the main criterion, the risk of false positives is high except for “exotic” colors, such as bright blue and red that were used in this study. In addition, non-paint plastic particles or paint particles from other sources (e.g., houses) may be mistaken for the target particles. Size, shape, and textural characteristics that correspond to paint flakes may be used to strengthen the decision-making process. Using metal content as the main criterion poses an advantage in identifying antifouling paint particles, which pose the highest risk for the marine ecosystem due to their inherent toxicity. However, non-paint particles (e.g., metal-rich friction byproducts, or engine residues) may be mistakenly categorized as boat paint microplastics due to their metal composition. Using combinations of metals as a compositional “fingerprint”, such as the copper-zinc combination used in this study appears to be a promising way forward, however, additional criteria are needed to exclude other natural or anthropogenic particles. Analysis with spICPMS provides information on the smallest particles, which are more likely to diffuse outside the limits of a marina, in the open sea or ocean. However, the information acquired is limited to one element, providing partial information about the particle composition. Using a time-of-flight spICPMS (Praetorius et al., 2017) is likely to provide a more holistic picture of the particle composition and allow using combinations of elements (e.g., Cu, Zn, and Sn) for identifying boat paint particles.
A comparison of these methods (Table 2) highlights that the detection and quantification of boat paint microplastics poses additional challenges than most microplastics. Paint particles contain, in general, more additives than plastics, thus hampering analysis using instruments typically used for microplastics, such as Raman spectroscopy and Fourier-Transform Infrared spectroscopy (FTIR). Analysis of paint particles or pigments with FTIR spectroscopy can be challenging for many reasons. Due to the nature of the particles, it is not suitable to use transmission mode (high energy throughput and thereby high sensitivity), and in reflectance mode, if the surface is not totally reflecting the resulting output spectra has a low quality. Moreover, the spectral range is typically 600–4,000 cm−1 excluding many bands from pigments, and sulfide pigments such as red pigments cannot be analyzed with FTIR (Castro et al., 2003). FTIR spectra of particle samples may be compared with reference spectra from products in the market or from specific ships [e.g., (Y. K. Song et al., 2014)], however, obtaining a complete reference library is a challenging task. Finally, the limits of detection of particle size with FTIR is relatively high (approx. 10 µm). The main challenge with Raman spectroscopy is to overcome the fluorescence interference. However, when a quality spectrum is achieved, it reveals the chemical composition of the paint color (Figure 8). For larger particles, visible through a light microscope, Raman analysis to verify a color is unnecessary. However, a colored particle may not necessarily be paint, it could be a colored plastic particle as well. Spectral analysis of colored plastics often reveals both the chemical composition of the color itself and the polymer which is not the case for paint particles. The challenge of differentiating the polymer from the color in paint particles is demonstrated in Figure 8 and applies to both particle-specific analysis and chemical mapping. For that reason, scientists have used Raman to classify paint particles based on the absence of a polymer signal (e.g., (Imhof et al., 2016; de Haan et al., 2019)).
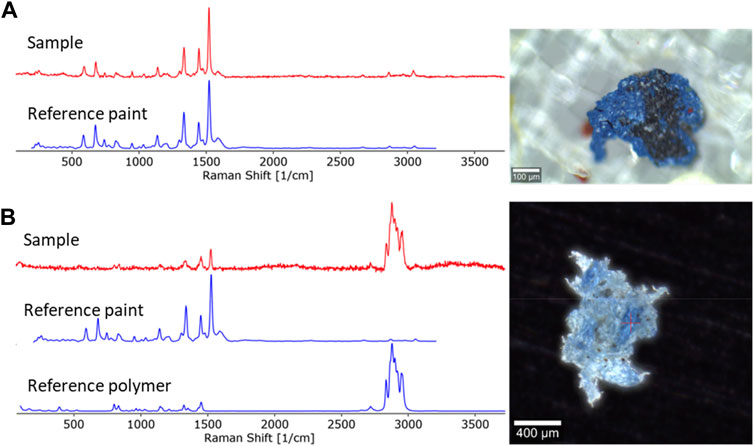
FIGURE 8. Example of a blue paint particle (A) and blue plastic particle (B), with spectra acquired using Raman microscopy (red color) compared to spectra of reference materials blue paint and polypropylene polymer.
Overall, our work demonstrates the applicability of standalone and correlative approaches for tackling challenges posed by boat paint microplastic detection and quantification in the marine environment. Advantages and disadvantages are outlined for LM, SEM-EDX, and spICPMS and beyond state-of-the-art correlative microscopy is demonstrated with promising results. In addition, we demonstrate that boat paint particles are present in the seawater inside marinas, including antifouling paints, which contain tin, most likely persisting from historical use of paints containing TBT (banned since 2008), either resuspended from sediments or freshly released from blasting older paint layers of ship hulls (Eklund and Watermann, 2018). This study was conducted after the introduction of boat washing stations in marinas. In order to persuade leisure boat owners to stop painting and instead get into the habit of brushing the boats in the cleaning stations, it was initially allowed to also brush boats with hard antifouling coatings. Although it is clear in the scientific literature that metals used in AFCs impact the marine ecosystem (Singh and Turner, 2009; Parks et al., 2010; Turner, 2010; Turner and Radford, 2010; Turner, 2013; Hasan et al., 2014; Muller-Karanassos et al., 2021), the spread and fate of boat paint microplastics is largely unknown. Further research on the release mechanisms and fate processes of boat paint microplastics is necessary to help guide regulatory efforts to address recent (e.g., microplastics) and historical (e.g., TBT paint) environmental issues. Developing appropriate analytical procedures for sampling and analysis is crucial in this respect.
Data availability statement
The raw data supporting the conclusion of this article will be made available by the authors, without undue reservation.
Author contributions
MH conceived the project idea, did sampling and data analysis, AG and KM did sample analysis and data analysis, all authors wrote the paper.
Funding
Swedish Agency for Water and Marine Management, the Swedish Environmental Protection Agency, FRAM: the Centre for Future Chemical Risk Assessment and Management Strategies, and JPI-OCEANS project ANDROMEDA.
Acknowledgments
The authors would like to thank Per-Olof Samuelsson for assistance with sampling, the reviewers for the insightful comments, and the Swedish Agency for Water and Marine Management, the Swedish Environmental Protection Agency, FRAM: the Centre for Future Chemical Risk Assessment and Management Strategies, and JPI-OCEANS project ANDROMEDA for financial support.
Conflict of interest
The authors declare that the research was conducted in the absence of any commercial or financial relationships that could be construed as a potential conflict of interest.
Publisher’s note
All claims expressed in this article are solely those of the authors and do not necessarily represent those of their affiliated organizations, or those of the publisher, the editors and the reviewers. Any product that may be evaluated in this article, or claim that may be made by its manufacturer, is not guaranteed or endorsed by the publisher.
Supplementary material
The Supplementary Material for this article can be found online at: https://www.frontiersin.org/articles/10.3389/fenvc.2023.1090704/full#supplementary-material
References
Castro, K., Pérez, M., Rodríguez-Laso, M. D., and Madariaga, J. M. (2003). Peer reviewed: FTIR spectra database of inorganic art materials. Anal. Chem. 75 (9), 214–221. doi:10.1021/AC031320E
Comitology Register (2021). Commission regulation (eu). Avaliable At: https://ec.europa.eu/transparency/comitology-register/screen/documents/083921/1/consult?lang=en.
Corinaldesi, C., Canensi, S., Dell’Anno, A., Tangherlini, M., di Capua, I., Varrella, S., et al. (2021). Multiple impacts of microplastics can threaten marine habitat-forming species. Commun. Biol. 4 (11), 431–513. doi:10.1038/s42003-021-01961-1
de Haan, W. P., Sanchez-Vidal, A., and Canals, M. (2019). Floating microplastics and aggregate formation in the western mediterranean sea. Mar. Pollut. Bull. 140, 523–535. doi:10.1016/J.MARPOLBUL.2019.01.053
Eklund, B., and Watermann, B. (2018). Persistence of TBT and copper in excess on leisure boat hulls around the Baltic Sea. Environ. Sci. Pollut. Res. 25 (15), 14595–14605. doi:10.1007/s11356-018-1614-1
Enders, K., Lenz, R., Beer, S., and Stedmon, C. A. (2017). Extraction of microplastic from biota: Recommended acidic digestion destroys common plastic polymers. ICES J. Mar. Sci. 74 (1), 326–331. doi:10.1093/ICESJMS/FSW173
Fauser, P., Bach, L., Daugaard, A. E., Vollertsen, J., Murphy, F., Koski, M., et al. (2019). Risk assessment of harmful types of plastics in the marine environment. Aarhus University, DCE—Danish Centre for Environment and Energy. Scientific Report from DCE—Danish Centre for Environment and Energy No. 329.
Fauser, P., Vorkamp, K., and Strand, J. (2022). Residual additives in marine microplastics and their risk assessment – a critical review. Mar. Pollut. Bull. 177, 113467. doi:10.1016/J.MARPOLBUL.2022.113467
Galloway, T. S., Cole, M., and Lewis, C. (2017). Interactions of microplastic debris throughout the marine ecosystem. Nat. Ecol. Evol. 1 (55), 0116–0118. doi:10.1038/s41559-017-0116
Gaylarde, C. C., Neto, J. A. B., and da Fonseca, E. M. (2021). Paint fragments as polluting microplastics: A brief review. Mar. Pollut. Bull. 162, 111847. doi:10.1016/J.MARPOLBUL.2020.111847
Graner, W. R. (1982). Marine applications. Handb. Compos. 1982, 699–721. doi:10.1007/978-1-4615-7139-1_27
Hartmann, N. B., Hüffer, T., Thompson, R. C., Hassellöv, M., Verschoor, A., Daugaard, A. E., et al. (2019). Are we speaking the same language? Recommendations for a definition and categorization framework for plastic debris. Environ. Sci. Technol. 53 (3), 1039–1047. doi:10.1021/acs.est.8b05297
Hasan, C. K., Turner, A., Readman, J., and Frickers, T. (2014). Environmental risks associated with booster biocides leaching from spent anti-fouling paint particles in coastal environments. Water Environ. Res. 86 (12), 2330–2337. doi:10.2175/106143014X14062131178835
Hassellöv, M., and Kaegi, R. (2009). Analysis and characterization of manufactured nanoparticles in aquatic environments. Environ. Hum. Health Impacts Nanotechnol. 2009, 211–266. doi:10.1002/9781444307504.CH6
Imhof, H. K., Laforsch, C., Wiesheu, A. C., Schmid, J., Anger, P. M., Niessner, R., et al. (2016). Pigments and plastic in limnetic ecosystems: A qualitative and quantitative study on microparticles of different size classes. Water Res. 98, 64–74. doi:10.1016/J.WATRES.2016.03.015
IMO (2019). Hull scrapings and marine coatings as a source of microplastics. Avaliable At: https://wwwcdn.imo.org/localresources/en/OurWork/Environment/Documents/Hull%20Scrapings%20final%20report.pdf.
Karlsson, J., Ytreberg, E., and Eklund, B. (2010). Toxicity of anti-fouling paints for use on ships and leisure boats to non-target organisms representing three trophic levels. Environ. Pollut. 158 (3), 681–687. doi:10.1016/J.ENVPOL.2009.10.024
Karlsson, T. M., Kärrman, A., Rotander, A., and Hassellöv, M. (2020). Comparison between manta trawl and in situ pump filtration methods, and guidance for visual identification of microplastics in surface waters. Environ. Sci. Pollut. Res. 27 (5), 5559–5571. doi:10.1007/s11356-019-07274-5
Mattsson, K., Björkroth, F., Karlsson, T., and Hassellöv, M. (2021a). Nanofragmentation of expanded polystyrene under simulated environmental weathering (thermooxidative degradation and hydrodynamic turbulence). Front. Mar. Sci. 7, 1252. doi:10.3389/fmars.2020.578178
Mattsson, K., da Silva, V. H., Deonarine, A., Louie, S. M., and Gondikas, A. (2021b). Monitoring anthropogenic particles in the environment: Recent developments and remaining challenges at the forefront of analytical methods. Curr. Opin. Colloid & Interface Sci. 56, 101513. doi:10.1016/J.COCIS.2021.101513
Mattsson, K., Ekstrand, E., Granberg, M., Hassellöv, M., and Magnusson, K. (2022). Comparison of pre-treatment methods and heavy density liquids to optimize microplastic extraction from natural marine sediments. Sci. Rep. 12 (11), 15459–9. doi:10.1038/s41598-022-19623-5
Muller-Karanassos, C., Arundel, W., Lindeque, P. K., Vance, T., Turner, A., and Cole, M. (2021). Environmental concentrations of antifouling paint particles are toxic to sediment-dwelling invertebrates. Environ. Pollut. 268, 115754. doi:10.1016/J.ENVPOL.2020.115754
Navratilova, J., Praetorius, A., Gondikas, A., Fabienke, W., von der Kammer, F., and Hofmann, T. (2015). Detection of engineered copper nanoparticles in soil using single particle ICP-MS. Int. J. Environ. Res. Public Health 12 (12), 15756–15768. doi:10.3390/ijerph121215020
Parks, R., Donnier-Marechal, M., Frickers, P. E., Turner, A., and Readman, J. W. (2010). Antifouling biocides in discarded marine paint particles. Mar. Pollut. Bull. 60 (8), 1226–1230. doi:10.1016/J.MARPOLBUL.2010.03.022
Paruta, P., Pucino, M., and Bucher, J. (2022). Plastic paints the environment. Avaliable At: https://www.e-a.earth/plasticpaintstheenvironment.
Praetorius, A., Gundlach-Graham, A., Goldberg, E., Fabienke, W., Navratilova, J., Gondikas, A., et al. (2017). Single-particle multi-element fingerprinting (spMEF) using inductively-coupled plasma time-of-flight mass spectrometry (ICP-TOFMS) to identify engineered nanoparticles against the elevated natural background in soils. Environ. Sci. Nano 4 (2), 307–314. doi:10.1039/c6en00455e
Scianni, C., and Georgiades, E. (2019). Vessel in-water cleaning or treatment: Identification of environmental risks and science needs for evidence-based decision making. Front. Mar. Sci. 6, 467. doi:10.3389/fmars.2019.00467
Singh, N., and Turner, A. (2009). Trace metals in antifouling paint particles and their heterogeneous contamination of coastal sediments. Mar. Pollut. Bull. 58 (4), 559–564. doi:10.1016/J.MARPOLBUL.2008.11.014
Song, C., and Cui, W. (2020). Review of underwater ship hull cleaning technologies. J. Mar. Sci. Appl. 19 (3), 415–429. doi:10.1007/s11804-020-00157-z
Song, Y. K., Hong, S. H., Jang, M., Kang, J. H., Kwon, O. Y., Han, G. M., et al. (2014). Large accumulation of micro-sized synthetic polymer particles in the sea surface microlayer. Environ. Sci. Technol. 48 (16), 9014–9021. doi:10.1021/es501757s
Sousa, A. C. A., Pastorinho, M. R., Takahashi, S., and Tanabe, S. (2014). History on organotin compounds, from snails to humans. Environ. Chem. Lett. 12 (1), 117–137. doi:10.1007/s10311-013-0449-8
Takahashi, C. K., Turner, A., Millward, G. E., and Glegg, G. A. (2012). Persistence and metallic composition of paint particles in sediments from a tidal inlet. Mar. Pollut. Bull. 64 (1), 133–137. doi:10.1016/J.MARPOLBUL.2011.10.010
Torres, F. G., and De-la-Torre, G. E. (2021). Environmental pollution with antifouling paint particles: Distribution, ecotoxicology, and sustainable alternatives. Mar. Pollut. Bull. 169, 112529. doi:10.1016/J.MARPOLBUL.2021.112529
Transportstyrelsens rapport Båtlivsundersökningen 2020 (2021). En undersökning om båtlivet i Sverige. Avaliable At: https://www.transportstyrelsen.se.
Tuoriniemi, J. (2013). “New single particle methods for detection and characterization of nanoparticles in environmental samples,” in Jani tuoriniemi (Gothenburg, Sweden: Gothenburg University). Avaliable At: http://hdl.handle.net/2077/33677.
Turner, A., Comber, S., Rees, A. B., Gkiokas, D., and Solman, K. (2015). Metals in boat paint fragments from slipways, repair facilities and abandoned vessels: An evaluation using field portable XRF. Talanta 131, 372–378. doi:10.1016/J.TALANTA.2014.08.012
Turner, A. (2010). Marine pollution from antifouling paint particles. Mar. Pollut. Bull. 60 (2), 159–171. doi:10.1016/J.MARPOLBUL.2009.12.004
Turner, A. (2022). Metal contamination of intertidal sediment and macroalgae in an area impacted by paint from abandoned boats. Mar. Pollut. Bull. 182, 113958. doi:10.1016/J.MARPOLBUL.2022.113958
Turner, A. (2013). Metal contamination of soils, sediments and dusts in the vicinity of marine leisure boat maintenance facilities. J. Soils Sediments 13 (6), 1052–1056. doi:10.1007/s11368-013-0686-2
Turner, A., Ostle, C., and Wootton, M. (2022). Occurrence and chemical characteristics of microplastic paint flakes in the North Atlantic Ocean. Sci. Total Environ. 806, 150375. doi:10.1016/J.SCITOTENV.2021.150375
Turner, A. (2021). Paint particles in the marine environment: An overlooked component of microplastics. Water Res. X 12, 100110. doi:10.1016/J.WROA.2021.100110
Turner, A., and Radford, A. (2010). Bioaccessibility of trace metals in boat paint particles. Ecotoxicol. Environ. Saf. 73 (5), 817–824. doi:10.1016/J.ECOENV.2010.02.022
Uc-Peraza, R. G., Castro, Í. B., and Fillmann, G. (2022). An absurd scenario in 2021: Banned TBT-based antifouling products still available on the market. Sci. Total Environ. 805, 150377. doi:10.1016/J.SCITOTENV.2021.150377
Keywords: paint, microplastics, marine, antifouling, correlative microscopy, electron microscopy, light microscopy, single particle ICPMS
Citation: Gondikas A, Mattsson K and Hassellöv M (2023) Methods for the detection and characterization of boat paint microplastics in the marine environment. Front. Environ. Chem. 4:1090704. doi: 10.3389/fenvc.2023.1090704
Received: 05 November 2022; Accepted: 11 January 2023;
Published: 26 January 2023.
Edited by:
Massoud Kaykhaii, Gdansk University of Technology, PolandReviewed by:
Gabriel Enrique De-la-Torre, Saint Ignatius of Loyola University, PeruKristian Wende, Leibniz Institute for Plasma Research and Technology e.V. (INP), Germany
Copyright © 2023 Gondikas, Mattsson and Hassellöv. This is an open-access article distributed under the terms of the Creative Commons Attribution License (CC BY). The use, distribution or reproduction in other forums is permitted, provided the original author(s) and the copyright owner(s) are credited and that the original publication in this journal is cited, in accordance with accepted academic practice. No use, distribution or reproduction is permitted which does not comply with these terms.
*Correspondence: Martin Hassellöv, bWFydGluLmhhc3NlbGxvdkBndS5zZQ==