- 1Univ. Lille, CNRS, Univ. Littoral Côte D’Opale, IRD, UMR 8187—LOG—Laboratoire d’Océanologie et de Géosciences, Wimereux, France
- 2Centre d’Innovation et de Recherche des Matériaux et Polymères (CIRMAP), Service des Matériaux Polymères et Composites (SMPC), Université de Mons, France, Belgium
- 3UMR 8516, CNRS, LASIRE—Laboratoire de Spectroscopie pour les interactions, la Réactivité et l’Environnement, Lille, France
This study investigates several methods to identify microplastics (MPs) of small size ranges (<10 µm) in the copepod Eurytemora affinis collected in the Seine estuary (France) and identified using epifluorescence microscopy and Raman microspectroscopy. In order to calibrate the methodology, copepods obtained from cultures were used. Firstly, we labelled three types of MPs (i.e., Polystyrene, Polyethylene, and Polylactic acid) with Nile Red and confirmed their ingestion by E. affinis with epifluorescence microscopy. Considering the convenient detection of Nile Red labelled MPs using epifluorescence observation, we tried to pair this method with Raman microspectroscopy. For this, we developed an enzymatic digestion method consisting of copepods digestion using Proteinase K followed by sonication in order to fragment their cuticle. The lysate was then vacuum filtered on black polycarbonate membrane filters that were the most appropriate for epifluorescence microscopy. Potential MPs were dyed with Nile Red directly on the filter, which allowed a relatively rapid visual detection. However, results showed that black polycarbonate membrane filters induced a significant background fluorescence during Raman identification of MPs and hence particles smaller than 10 µm could not be characterized. In this case, we were not able to link staining method with micro-Raman for the size range of MPs targeted in this study. Thus, aluminum oxide filters were tested, and staining method was replaced by a classical observation with stereomicroscopic magnifier to delimit areas of observation for Raman microscopic identification. Aluminum oxide filters induced less fluorescence, allowing the detection of MPs (as small as 1 µm diameter) on copepods from laboratory cultures exposed with MPs. We applied this method on copepods collected in the natural environment. Within a pool of 20 copepods of three replicates, we identified 17 MPs (average of 0.28 MPs/copepod) composed of eight different polymer types and six colors. These MPs corresponded to 59% of fibres with 14.1 ± 9.4 µm diameter and 391.6 ± 600.4 µm length along with 41% of fragments with an average diameter of 13.2 µm ± 9.5 µm. This study reports a novel approach to detect the presence of small particles of MPs ingested by copepods in the natural environment.
1 Introduction
The first sampling of plastic polymers in the marine environment was performed in 1972 (Carpenter et al., 1972). In the decades following this discovery, several researchers have warned about the ecological impact of macroscale plastic pollution on several higher trophic levels of marine animals like sea-birds (Blight and Burger, 1997; Tanaka et al., 2013), marine turtles (Campani et al., 2013), fishes (Romeo et al., 2015) or marine mammals (Laist, 1997). Studying the impact of small size fragments of plastics on almost all components of marine food-webs has been recently documented (Jeong et al., 2017; Li et al., 2020). A significant fraction of plastic debris is channeled by rivers and estuaries to marine environments. For example, in the Seine River, plastic pollution is observed in environmental samples since 1965 on sites under tidal influence (Tramoy et al., 2019). Whereas, the large size of plastic debris is visible in aquatic habitats and called macro-plastic, the smallest fraction called microplastic, arbitrary defined as plastic fragments of less than 5 mm in size represent the hidden part (Arthur et al., 2008) and majority (Lindeque et al., 2020) of plastic pollution. MPs can originate from the degradation of larger plastic debris by physical and chemical mechanisms during their migration in ecosystems (Andrady, 2011). Thus, when the size of MPs decreases, their number inevitably increases (Maes et al., 2017). In addition, a significant part of microplastics is also directly released in the environment through synthetic fibers from textile unleashed by domestic washing machines (Napper and Thompson, 2016) or from cosmetic products like facial cleansers (Napper et al., 2015).
Because of their small size range and diverse properties (abundance, variety of density, and colors), MPs have a great bioavailability for a large variety of vertebrates and invertebrates at lower trophic levels (Wright et al., 2013). Researchers focusing on MPs topic used wide methodologies to detect and characterize this xenobiotic in different environmental compartments (Renner et al., 2018). Even if most studies used visual microscope examination, this method used alone, remains controversial (Song Y. K. et al., 2015) because of the wide variations between observers that can exist and so lead to overestimation or underestimation of MPs (Prata et al., 2019). To remedy this problem, more analytical methods tend to be used to obtain more accurate chemical characterization. Among them, Fourier transform infrared spectroscopy (FTIR) (Frias et al., 2014) and Raman spectroscopy (Lenz et al., 2015) are popular methods. According to literature, FTIR represent a good alternative to visual analysis as it is less time-consuming compared to Raman spectroscopy. However, FTIR could lead to underestimation of MPs < 20 µm compared to Raman spectroscopy because of the diffraction limit of IR spectroscopy (Käppler et al., 2016) and detection limit is fixed to 10 µm (Sun et al., 2018a)
Copepods is a widely distributed group in all aquatic ecosystems (Mauchline, 1998). Several studies showed that both large sized copepods, such as the calanoid Calanus helgolandicus, and the small cyclopoid Paracyclopina nana have the ability to ingest MPs in the size range of their natural preys, using fluorescent beads of one single polymer, generally Polystyrene (Cole et al., 2014; Cole et al., 2015; Cole et al., 2016; Jeong et al., 2017). Ingestion of non-metabolizable particles induce stresses measurable at individual scale including energetic lack for development, decrease of reproductive success and lipid reserves (Cole et al., 2015) but also at molecular levels with induction of oxidative stress (Jeong et al., 2017). The ingestion rate of MPs depends on the food selectivity of individuals which itself depends on the capacity to distinguish natural preys from MPs (Setälä et al., 2014). Furthermore, copepods have mechano- and chemoreceptors that allow them to handle properly their environment and discriminate non-food from food items (Strickler, 1982). However, in the environment, MPs can be really similar to food items and confuse copepods because they: have different shapes like fibers or fragments, come from different polymers, and are generally biofouled. In fact, some studies showed that ingestion rates varied with polymer nature and shape (Desforges et al., 2015; Coppock et al., 2019) and also if MPs are biofouled compared to virgin and pristine ones (Vroom et al., 2017).
Although important, studies showing the interaction between fluorescent pristine MPs and copepods are difficult to extrapolate to field situation since most in situ MPs are not fluorescent and have large variety of shapes. Consequently, investigation of copepods and non-fluorescent MPs interactions remains limited and needs adequate methodological development. To circumvent this issue, some studies used visual detection using Nile Red staining of MPs and observation of whole copepods with epifluorescence microscopy when trying to observe the non-fluorescent MPs in digestive tract of copepods (Cole et al., 2019; Coppock et al., 2019). Although effective, staining of MPs were performed before ingestion of MPs by copepods. Therefore, we don’t know if this method is suitable to study MPs impregnation of environmental samples of copepods. Another bottleneck when trying to detect and characterize the non-fluorescent MPs ingested by copepods is the elaboration of new methods able to « expel » MPs from copepod matrix, notably cuticle, and minimize their alteration. Studies focus on MPs contamination of natural environment copepods used acid digestion of copepods based on a previous work (Desforges et al., 2015). However, this method could least to digestion of low pH tolerance polymers and underestimate MPs contamination in individuals. For MPs characterization, visual detection and µ-FTIR were the two methods used with a lower limit of 7 µm for MPs characterization (Sun et al., 2018a).
To our knowledge, there is no published study that developed an adequate analytical method for the identification and chemical characterization of small-size MPs (∼1–10 µm) ingested by copepods in the field. Thus, we developed a complete methodology using mass culture adults of Eurytemora affinis to correctly digest copepods without altering low pH tolerance polymers by using an enzymatic digestion with Proteinase K and reached characterization limit of MPs to 1 µm with Raman microspectroscopy using aluminum oxide filters. Once robust methodology was validated, it was applied to adult copepods of the same species collected from the Seine estuary allowing a better quantification of MPs ingested by copepods. MPs contamination of these copepods showed greater MPs contamination levels compared to literature which could be indicative of an underestimation of MPs levels in copepod model in literature because of previous MPs detection limit.
2 Materials and Methods
2.1 Microplastics Tested on Experimental Copepods
Firstly, three types of commercial plastics were, separately, considered during this study: 1) two non-compostable petro-based plastics (i.e., polystyrene (PS) (d < 1 g cm−3) and polyethylene (PE) (d < 1 g cm−3); 2) a compostable bio-based plastics (i.e., polylactic acid (PLA)(d > 1 g cm−3). PS beads were supplied by Polysciences Inc., United States. This Polybead Microspheres are monodispersed polystyrene microspheres with 6 µm of diameter. PE were prepared by Prof. J. Cachot (University of Bordeaux, France) using crushing and cryogenic grinding protocol leading to MPs with 4–6 µm of diameter. PLA MPs, with 3–9 µm of diameter, were prepared by Resinex (Belgium) from Ingeo™ Biopolymer 4043D provided by NatureWorks LLC (United States).
To test our methods on a combination of polymers, we used a mixture of macroplastics collected from the Seine estuary, that was crushed and cryo-grinded into microplastics used for studies within the French Plastic-Seine project. Samples were composed in weight of 65% of PE (40% LDPE and 25% HDPE), 25% of Polypropylene (PP) and 10% of PS. These three polymers are representative of global contamination by MPs because they are massively produced every year and are common in marine environment (Avio et al., 2017). Overall, 7% of MPs in this sample had a size in our targeted size range <10 µm.
2.2 Copepods
2.2.1 Experimental Copepods
The culture of the copepod E. affinis used in our experiments comes from wild individuals sampled in September 2014 from the oligohaline zone of the Seine estuary and maintained since that date at the Marine Station of Wimereux at a constant temperature of 19°C with 12:12 photoperiod at salinity 15 PSU (seawater from the English Channel adjusted to salinity with deionized water) (Michalec et al., 2017) using the multi-generations protocol developed by our group (Souissi et al., 2016; Das et al., 2020). This mass culture provides standardized individuals for ecotoxicological studies. In this work, we focused on adult stages by using both genders for the MPs exposures.
Copepods were fed with the Rhodomonas sp. microalga which is a good nutritional source for copepods, allowing high performance of the culture (Dayras et al., 2021). Copepods were starved for 1 week before exposure to MPs, both to render MPs more attractive for feeding but also to facilitate observation with fluorescence microscope because of the relative auto-fluorescence of Rhodomonas sp. cells.
2.2.2 Copepods Sampled From the Field
To validate our methods on environmental samples, 60 adults of E. affinis were isolated from “La Bouille” (GPS coordinates: 49.35407849415815, 0.9293737816503634) station by the laboratory of Functional ecology and Environment of Toulouse (EcoLab–UMR CNRS-UPS-INPT 5245) using a plankton net in the context of SENTINELLES project (https://www.seine-aval.fr/projet/sentinelles/). Copepods were sampled at two different points by filtering 50 L of water on a 50 µm net. Next, each zooplankton sample was maintained in 200 ml formaldehyde 4% solution. This station is located in the upstream zone of the Seine estuary.
Adult individuals of E. affinis, were sorted to study their MPs contamination. Zooplankton samples were first sieved and rinsed abundantly to remove formaldehyde and then placed in ethanol in a homogenous way with a Motoda box. E. affinis individuals were then isolated with Pasteur pipette and put in a closed glass jar with 70% ethanol solution for future studies of polymers potentially ingested.
During copepod isolation, an open glassware filled with MilliQ water was placed near the operator during all handling in order to be used as control for airborne MPs contamination. This MilliQ water was then vacuum filtered and MPs were counted to evaluate the airborne contamination that could affect filters with digested copepods.
2.3 Ingestion Treatment on Experimental Copepods
For the tested MPs (i.e., PS, PE, PLA, and in situ MPs) in cultured copepods, the ingestion protocol was the same. One control and triplicates of 20 adults of E. affinis were isolated by using Pasteur pipette under binocular magnifier (Olympus SZX2-ILLK, Olympus, Japan) and then maintained in a 80 ml beaker with 15 PSU water (estuarine salinity) under constant aeration. Estuarine salinity was obtained by mixing MilliQ water with 0.2 µm filtered sea water from English Channel.
For each sample of MPs above-mentioned, a concentration of 3 mg/L of MPs (size range described in Section 2.1) was added in each beaker for 12 h in the dark to keep fluorescence of MPs when they were stained with Nile Red. For these conditions, the quantity of Nile Red adsorbed on MPs was low enough to avoid any negative effect on copepods during exposure.
2.4 Microplastics Identification Methods on Cultured Copepods
2.4.1 Staining of Microplastics
For the three staining methods described below, a stock solution of 500 μg/ml of Nile Red was prepared in acetone and stored at 4°C in the dark for future needs of the experiments.
2.4.1.1 Staining of Microplastics Before Exposure
This first staining method was performed as preliminary work. The aim of this experiment was to demonstrate the capacity of adults of E. affinis from mass culture to ingest MPs samples in a size range in accordance with our targeted objective for MPs (<10 µm) from wild individuals. MPs were placed in a micro-tube with few drops of Nile Red stock solution for 10 min in the dark. After that, stained microplastics were vacuum filtered on a Whatman™ Nuclepore polycarbonate membrane (0.2 µm, 47 mm), briefly rinsed with acetone to remove excess dye then abundantly rinsed with MilliQ water. Finally, MPs were re-suspended for the ingestion experiment previously described (Section 2.3).
2.4.1.2 Staining of Microplastics After Exposure Without Digestion Step
Staining of MPs before ingestion is not applicable for wild copepods. Thus, in order to study the possibility of detecting MPs on whole copepods using epifluorescence microscopy, we tried different staining protocols. First, we stained MPs in digestive tract after copepods ingestion without digesting organisms. To make sure individuals had MPs in the digestive tract, a total of 20 copepods for which MPs were detected in the digestive tract by using Nile Red before ingestion were left for 2 h on daylight in a Petri dish to halt the staining from the colorant. Next, copepods were put in a beaker with 20 ml of MilliQ water and then 10 µl of the Nile Red stock solution was added. Copepods were left in the dark for 10 min before the observation with epifluorescence microscope (Nikon Eclipse TE2000-S, Nikon, Japan).
2.4.1.3 Staining of Microplastics After Exposure and Digestion of Individuals
After testing the possibilities offered by staining with Nile Red of MPs on whole copepods, the same techniques were directly applied to a filter, after digestion of individuals contaminated by MPs and vacuum filtration. The hypothesis of this method was to still use the advantage of staining with Nile Red and making applicable a Raman microspectroscopy identification. As Polycarbonate filters were used, a second solution of Nile Red (5 μg/ml) in n-hexane solvent was prepared by diluting the stock solution made in acetone. N-hexane was used to not modify Polycarbonate filter properties following a previous study (Shim et al., 2016). This lowest concentration of Nile Red was used to still stain MPs without inducing too much background fluorescence.
After digestion of individuals and vacuum filtration of the lysate, the solution was briefly vortexed and 1 ml was added on filter and then left for 10 min to induce total evaporation of the solvent. The same staining protocol was performed for our tests on aluminum oxide filters.
2.4.2 Preparation of Copepods for Microplastics Identification
For the mass cultured copepods, at the end of the ingestion protocol, individuals were sieved, fixed with ethanol 70% and then gently rinsed with MilliQ water to remove MPs on external parts of copepods like cuticle and appendices.
Then, the next step for the copepods preparation depended on the final analytical method used:
For the two first staining protocols, that did not require digestion of copepods as previously described, individuals were directly placed on microscopy glass slides and observed with the epifluorescence microscope.
For the tests on digested copepods, a pool of 20 individuals contaminated with MPs was placed in a 100 ml Erlenmeyer flask with 20 ml MilliQ water and 160 µl (0.2 mg/ml) of Proteinase K solution (AppliChem GmbH, Germany). Digestion of copepods was performed overnight at 50°C (optimum temperature for Proteinase K activity) under agitation, followed by 20 min sonication. Lysate was vacuum filtered on Whatman™ Nuclepore polycarbonate black membrane (0.2 µm, 47 mm) or Whatman™ Anodisc™ aluminum oxide filter (0.2 µm, 47 mm) depending on the experiments.
Cellulose filters were not used because of the high Raman signal emitted by cellulose (Wright et al., 2019). Paper, and fiberglass were used in our preliminary tests but quickly eliminated from our final protocol because of their relative thickness that don’t allow to easily identify MPs on the filter.
Filters were finally placed in Petri dishes covered with Parafilm applied to minimize external contamination by airborne MPs, and then placed in a desiccator with silica gel until future analysis.
For Raman microspectroscopy analysis, the same digestion protocol was followed.
2.4.3 Identification of Microplastics With Epifluorescence Microscopy
Samples were analyzed with an epifluorescence microscope at an excitation wavelength of 455 nm and an emission wavelength of 494 nm with objectives ×10, ×20, and ×40 (Olympus, Japan), when necessary. For the digested samples, during observation, zones of potential presence of MPs were delimited with a pencil to take less observation time for the micro-Raman manual detection and identification.
2.4.4 Identification With Raman Microspectroscopy
Filters were analyzed using a micro-Raman Xplora Plus (HORIBA Scientific®, France) equipped with a closing chamber that prevent filters from external airborne contamination. Zones of presence of potential MPs marked previously were observed with an objective ×10 and then ×100 by using a microscope (Olympus, France). Particles detected were analyzed with two lasers with a wavelength of 532 nm and 785 nm depending on particle on the range of 200–3,300 cm−1 to obtain spectra of main polymers (Käppler et al., 2016). For each suspected MP, Raman spectrum was compared to a polymer identification database (KnowItAll, BioRad®) and a personal library made with standard polymers obtained from Goodfellow (France). For the samples prepared from copepods collected in the Seine estuary, photos of items were taken using Labspec 6 software (Horiba Scientific, Japan). Moreover, their size, shape and color were recorded.
2.5 Application of Validated Method to Copepods Collected From the Field
A pool of 60 individuals sampled from the Seine estuary was analyzed by using the most effective method develop from our conclusions on cultured copepods. Briefly, individuals were digested and then filtered on Whatman™ aluminum oxide filters. Furthermore, our results on experimental copepods showed the need to use aluminum oxide filter for chemical identification of small MPs (<10 µm) with Raman microspectroscopy. However, staining method was impossible with this filter type. In order to minimize the observation time of potential MPs on filter during Raman analysis, staining of MPs and epifluorescence observation was replaced by preliminary binocular magnifier observation (Olympus SZX10, Olympus, Japan) during which zones of potential MPs presence were delimited with a pencil and then particles were analyzed with Raman microspectroscopy as described above.
3 Results
3.1 Staining Methods of Microplastics on Whole Copepods
Our preliminary tests of staining with Nile Red MPs before ingestion allowed us to stain and observe monomeric polymers (Figure 1) and in situ MPs using fluorescence microscopy. Thanks to the solvatochromic nature of Nile Red, the different observed polymers had a different staining pattern depending on their surface polarity characteristics (Maes et al., 2017). This characteristic allowed the detection of each polymer type separately (PS, PE or PLA) in the digestive tract of the individuals of E. affinis and also in their feces.
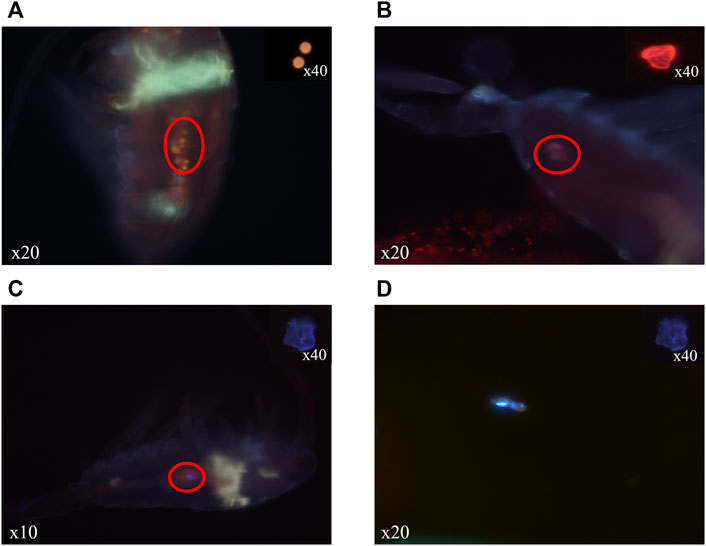
FIGURE 1. Microplastics of PS (A), PLA (B) and PE (C), (D) stained with Nile Red and detected (red circles) in digestive tracts (A–C) and faeces (D) after ingestion treatment of individuals of E. affinis with epifluorescence microscope (crime light: 455–494 nm).
For the in situ MPs mixture, we compared the relative abundance of each staining color with the sample to determine which polymer matches which each color (Figure 2A) and confirmed the results with micro-Raman. Contrary to PS and PP, particles surfaces of PE were composed in variable proportions of two staining colors. This characteristic arises because polyethylene was an assembly of HDPE and LDPE which changes the solvatochromic nature of Nile Red. This quick staining method confirmed that E. affinis adults are able to ingest MPs fragments and beads in the size range targeted to calibrate our methods and similar to their common food items. We noted that when ingested and visible in the digestive tract of the copepods, only the major staining color of the sample, given by most PE particles in the in situ MPs mixture, is visible with epifluorescence microscope (Figure 2B). For all the studied MPs types, we noted that this method, based on epifluorescence microscopy observation of stained with Nile Red MPs before ingestion, is limited by the natural fluorescence of copepods, notably the cuticles and the lipid droplets. The consequences are that MPs can be missed and quantification is difficult.
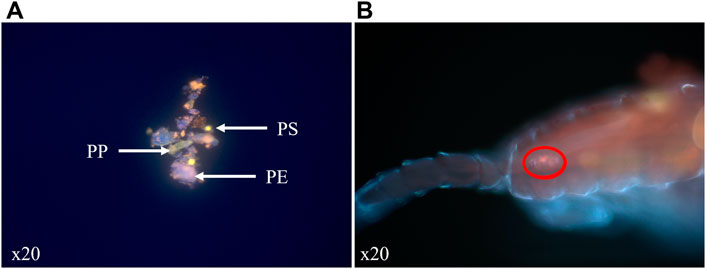
FIGURE 2. Sample of in situ MPs mixture stained with Nile Red and observed with epifluorescence microscope (crime light: 455–494 nm) (A). (B) Observation of in situ MPs ingested and visible (red circle) as a compact orange orb in the digestive tract of an adult copepod of E. affinis.
After ingestion by copepods, MPs were stained using Nile Red. This approach could have led to a methodology applicable for environmental samples of copepods with no digestion step. But, during observation, we noticed that staining with Nile Red was not applicable because it only stained lipid droplets of copepods and did not interact with the MPs in the digestive tract. At this point, digestion of individuals was a mandatory step to find a method based on fluorescence microscopy and Raman microspectroscopy operable on wild copepods.
3.2 Digestion of Copepods and Fluorescence Observations of the Stained Microplastics on Filters Resulting From Vacuum Filtration of the Lysate
By visual inspection, we noted that digestion method was effective to digest all organic materials of the copepod and break down chitin materials into small pieces recognizable as remaining translucent green fragments (Figures 3A,B), after staining with Nile Red and epifluorescence microscopy on black polycarbonate membrane filter. During epifluorescence observation, we detected that PE (Figure 3A) and PS (Figure 3B) had the same colorations as showed by our previous method when MPs were stained before ingestion without digestion of individuals.
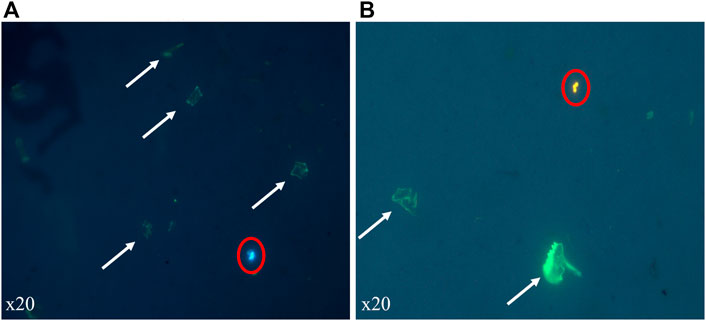
FIGURE 3. Observation of experimental copepods contaminated with MPs (red circles) of LDPE (A) and PS (B) and digested on black polycarbonate membrane filters. Filters were stained with Nile Red and observed with epifluorescence microscopy (crime light: 455–494 nm). Residual’s cuticle fragments are present as green translucent particles (white arrows).
Regarding staining method on aluminum oxide filters, much more background noise was observed probably due to the interaction between Nile Red and nature of the filter. Particles on the filter were much less noticeable (Figure 4). No critical differences were observed between MPs and cuticle fragments that permit to distinguish them.
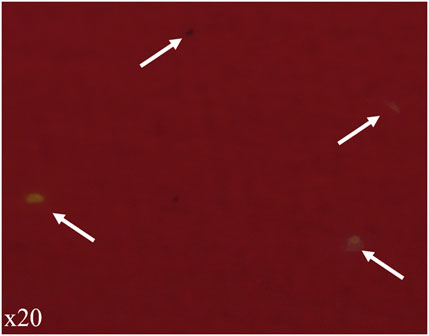
FIGURE 4. Observation of experimental copepods contaminated with MPs of LDPE and digested on aluminum oxide filter. Filter was stained with Nile Red and observed with epifluorescence microscopy (crime light: 455–494 nm). Ingested MPs and cuticle fragments were present on filter but undistinguishable.
3.3 Raman Microspectroscopy on Experimental Copepods
The first hypothesis was to couple the staining method that permit a quick detection of MPs on filter with a chemical identification with Raman microspectroscopy. But, over these advantages, we had a limit of polymer identification with micro-Raman relatively high (>10 µm) for polycarbonate black membrane filter compared to aluminum oxide one for which several ingested MPs of 1 µm were successfully characterized. Furthermore, the Raman background emitted by the polycarbonate filter didn’t allow to analyze plastic particle in the target size of 1–10 µm we fixed. Consequently, aluminum oxide filter was selected for the detection of MPs on in situ collected individuals (Table 1).
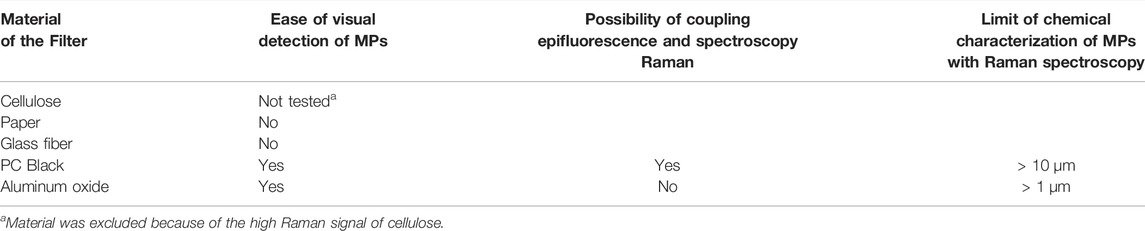
TABLE 1. Summary table of all tested filters (except for Cellulose) and possibilities it offers for Nile Red staining with epifluorescence microscopy and Raman spectroscopy to detect and characterize MPs ingested by copepods from the natural environment.
3.4 First Impression of Microplastics Contamination of Eurytemora affinis in the Seine Estuary
In total, 17 microplastics for an average of 0.28 MPs/individuals were found from the 60 (3 replicates of 20 individuals) analyzed copepods (Table 2). Eight different types of polymers were identified except for the polymer nature of one fiber due to the intensity of the fluorescence that masked the Raman signal. Polystyrene (PS) was the most common polymer found with five items, followed by High-density Polyethylene (HDPE), polypropylene (PP), polycarbonate (PC), Polyethylene terephthalate (PET) with two particles for each type. Finally, we found one particle for a copolymer composed of Polyamide (PA) and Polyvinyl acetate (PVAC) (Supplementary Figure S1), and also one particle for Polyamide and Low-density Polyethylene (LDPE). Regarding to the shape of items, no microbeads were observed. Fiber was the predominant shape (59%) followed by the fragment one (41%). Blue, black, and pink were the most identified colors with respectively six, five and three particles, followed by red, orange and translucent with one particle for each.

TABLE 2. Average (±SD), minimum and maximum of size of ingested MPs found in the copepod Eurytemora affinis collected in the area “La Bouille” in the French Seine estuary.
4 Discussion
4.1 Detection of Microplastics on Cultured Copepods Using Nile Red Staining
The staining method using Nile Red in acetone, previously developed in the literature (Cole, 2016), allowed us to stain and observe with blue light polymers when they were alone or in mixture in the digestive tract of adult individuals of E. affinis. This showed the physiological capacity of this species to ingest MPs of size ranged between 1 and 10 µm. Our observations confirmed the solvatochromic nature of Nile Red that allowed us to discriminate the most common polymers (PE, PS, and PP) as well as the PLA based on surface polarity characteristics (Maes et al., 2017). This method of staining MPs before ingestion treatment on copepods gave crucial information like lipid accumulation, feeding, food selectivity or fecal density when individuals from other species interact with MPs (Cole et al., 2019; Coppock et al., 2019) and could be clearly applied in the future to E. affinis (or other similar species) to better quantify the interaction between MPs and this species in the aquatic environment. Moreover, we demonstrate the capacity of Nile Red to stain a biobased polymer (PLA) and detect it in copepods gut. This could lead to the study of other biobased or biodegradable polymers impacts on copepods life traits.
However, our results highlighted that staining MPs with Nile Red cannot be used to accurately quantify the plastic fragments in the gut of copepods. In addition, staining of MPs after ingestion by copepods was not effective due to the high affinity of Nile Red for copepod’s lipid droplets (Song Y. S. et al., 2015) and so couldn’t be applied directly on whole copepods from the natural environment. Thus, we suggest that digestion of copepod is a necessary step when quantitative results or chemical characterization of polymers are needed like for wild sampled individuals.
4.2 Digestion Efficacy and Special Case of Polylactic Acid
Regarding to studies on biotic or abiotic compartments, there is no standardized protocol for the digestion of organic material and the isolation of MPs. Hydrogen peroxide (H2O2) and KOH have been the most used chemical treatments for this purpose (Renner et al., 2018; Kazour et al., 2019). In case of copepods, most studies used a digestion treatment with nitric acid (HNO3) developed by Desforges et al. (2015). In this study, we used an enzymatic digestion with Proteinase K because of its capacity to mineralize biological tissues without causing damages on small microplastics (Cole et al., 2014). Furthermore, Claessens et al. (2013) showed that strong mineral acids as HNO3 can oxidize and damage or destroy MPs from polymers with a low pH tolerance as PS or Polyamide (PA) and so can lead to an under estimation of MPs.
After heated incubation of samples with Proteinase K, 100% of the organic material of copepod was digested. Despite the constant agitation during this step, cuticles appeared intact for 90% of individuals and engendered an additional difficulty to detect the MPs released from the gut of the copepods. However, the additional step of sonication for 20 min solved this problem and cuticles were present as small fragments on filter after vacuum filtration that permitted to identify MPs directly on it with fluorescence microscopy or Raman microspectrometry.
It is important to keep in mind that our experiments for digesting copepods contaminated with PLA microplastics showed complete digestion of particles of this polymer. Previous study on copolymer’s films (10 mm × 10 mm × 0.4 mm) showed the capacity of proteinase K to degrade L-lactyl units compared to D-lactyl ones (Li et al., 2000). Because PLA represents one of the best alternatives to replace non-biodegradable plastics, particularly polypropylene (European Bioplastics, 2018), future research is needed to correctly study the fate of this plastic on wild biota samples.
4.3 Polycarbonate Black Membrane Filter and Microplastics Identification Possibilities
This type of filter coupled with Nile Red staining in n-hexane has been used in the past to detect microplastics in water and sand samples collected from the field (Shim et al., 2016). Authors reported the relative facility of detection during epifluorescence observation because of the flat surface and the black background of this filter. In our study, this method was effective to detect MPs of contaminated copepods. Contrary to the tests done before on Amphipod carapace (Shim et al., 2016), the cuticles fragments in our study were stained, which showed a clear green fluorescence pattern during the microscopic observation. In the case of remaining chitin fragments that could represent an issue for future identifications, chitinase enzyme can be used (Cole et al., 2014).
This first step of microplastics detection cannot precisely give the polymeric nature of microplastics. It can be considered as a useful and simple visual method to quickly detect suspected microplastics and directly target areas of their presence for further chemical identification with Raman microspectroscopy. Indeed, our results showed that polycarbonate black membrane filter can represent a good option for coupling Nile Red staining, epifluorescence microscopy and Raman spectroscopy but this filter is limited by the relatively high limit of detection of MPs. Nevertheless, in our case the use of black polycarbonate filter led to a lower micro-Raman identification limit of 10 µm due to high fluorescence emitted by filter material that didn’t meet our main objective.
4.4 Aluminum Oxide Filter: Lowering the Detection Limit of Microplastics for Micro-Raman Spectroscopy Identification
Aluminum oxide filter was tested in the past on pristine microplastics (4–50 µm) to detect airborne contamination. It showed a globally low intensity Raman signal, a clear visual detection of suspected microplastics on filter but didn’t permit authors to map microplastics using Streamline mode, contrary to silver membrane and mixed cellulose filters (Wright et al., 2019) with a 785 nm laser. Contrary to this last conclusion, we were able to identify pristine microplastics of PS and PE of 1–10 µm size range with this filter. This could be due to the relatively high wavelength used in this study. In fact, during our analysis, we saw that lowering the wavelength from 785 nm to 532 nm generally leads to an identifiable spectrum result with much less background noise for MPs of few microns.
Wright et al. (2019) also reported weaker intensity bands of PS microplastics due to interference when cellulose filters are used. This can lead to an under estimation of PS microplastics when Raman microspectroscopy is used to identify them, especially to detect microplastics ingested by copepods in the field (Sun et al., 2018a).
4.5 Validation of Full Method on Copepods From the Natural Environment
Our results on mass cultured copepods showed that despite the technical impossibility to detect MPs with staining and epifluorescence microscopy with aluminum oxide filter, this method represented the best option to identify microplastics of few microns with Raman microspectroscopy as a final method. To try to save analysis time, fluorescence method was replaced by a visual inspection with a stereomicroscope magnifier.
To validate our methodology on wild copepods, 60 individuals were digested and studied. With 94.1% of microplastics for which polymer was clearly identified with a minimum size of 2.1 µm, we can assume that aluminum oxide filter represents a robust solution for manual Raman microspectroscopy MPs identification proposing a methodology that lowers the restraint of detecting microplastics ingested by copepods observed in the literature (7 µm) (Sun et al., 2018a).
Gasperi and Cachot (2021) studied the levels of MPs contamination of the Seine estuary in the same sampling station of copepods studied in this paper. Even though they focused their research on the fraction >50 µm, they reported in the sampled station studied a concentration of 5 MP/m3 with three shapes: predominantly fragments, microbeads, and fibers. In our study, we showed that fibers were the most ingested shape followed by fragments, respectively 59% and 41%. The difference of abundance of fiber shape in abiotic compartment compared to our results on biota can be explained by the relatively high mesh size of 300 µm used that don’t permit to capture small fibers properly. Furthermore, punctual sampling with an 80 µm mesh size showed higher density of fiber shape MPs in this study. Additionally, most studies on MPs contamination of field copepods also reported fiber shape as the predominant ingested one (Sun et al., 2017; Sun et al., 2018a; Sun et al., 2018b; Zheng et al., 2021).
About identified polymers, our results are consistent regarding polymers identified in the Seine estuary (Gasperi and Cachot, 2021). Furthermore, this study and ours demonstrated a dominance of Polyethylene, Polystyrene, Polypropylene, and Polyethylene terephthalate to other polymers.
With an average retention of 0.28 MPs per individual, our results showed higher concentration of ingested MPs in copepods from Seine estuary compared to other similar studies where the concentrations ranged from 0.03 MPs/individual in northeast Pacific Ocean (Desforges et al., 2015) to 0.26 MPs/individual in Jiaozhou Bay — Yellow Sea (Zheng et al., 2021). For these two studies, MPs contamination of water column was not studied, so it is difficult to conclude if this difference can be explained by the level of MPs impregnation of water column or by the limit of detection of MPs. Nevertheless, Md Amin et al. (2020), performed MPs contamination analysis in both water with sampling conditions close to Gasperi and Cachot (2021) and copepods. Results of MPs in water ranged from 0.3 to 1.45 MPs/L and a mean of 0.13 MPs/individual were found in calanoid copepods with a limit of detection of 100 µm with FTIR. We can make the hypothesis that with concentrations between 60–290 times higher in water, ingested MPs concentration could have been higher as measurements were also on calanoid species.
In conclusion, we developed in this study a new methodology to identify MPs ingested by copepods with a new size limit of detection of 1 µm. Enzymatic digestion was the first critical point developed to ensure a correct degradation of organisms without a doubt to degrade potential polymers. Our results on cultured and wild copepods indicate the ability of Proteinase K coupled with ultra-sonification to correctly digest copepods for MPs characterization without causing damages on low pH tolerance classic polymers. However, the use of this enzyme led to a total digestion of PLA fragments in our conditions. Future studies should be achieved to better know the tolerance of other biobased or biodegradable polymers to chemical or enzymatic digestion protocols used to study MPs biota or environmental contamination.
We demonstrate that using aluminum oxide filter for Raman microspectroscopy analysis allow reaching a size limit for MPs detection in copepods down to 1 µm, which is far lower than found in current literature. Our approach is promising to better estimate MPs impregnation in key organisms, like copepods.
Data Availability Statement
The raw data supporting the conclusion of this article will be made available by the authors, without undue reservation.
Author Contributions
SS, SM, SB, MK, J-MR, and RA helped in drafting the manuscript and approved the current version. JT conceived and performed the experiments. CB and DD contributed to experiments. MK and MM helped for Raman microspectroscopy analyses. All authors contributed to the article and approved the submitted version.
Funding
This study is a part of the Ph.D. thesis of JT from the University of Mons and University of Littoral Côte d’Opale, funded in equal parts by the two Universities. This work is also a contribution to the CPER MARCO 2014-2020 and CPER 2021-2027 IDEAL projects funded by the region Hauts-de France, the French government, Europe (FEDER) and IFREMER. This study is also a contribution to the Plastic-Seine project funded by GIP Seine-Aval and to the International Associated Laboratory between the Université de Lille and the National Taiwan Ocean University (IAL MULTIFAQUA).
Conflict of Interest
The authors declare that the research was conducted in the absence of any commercial or financial relationships that could be construed as a potential conflict of interest.
Publisher’s Note
All claims expressed in this article are solely those of the authors and do not necessarily represent those of their affiliated organizations, or those of the publisher, the editors and the reviewers. Any product that may be evaluated in this article, or claim that may be made by its manufacturer, is not guaranteed or endorsed by the publisher.
Acknowledgments
We are grateful to Michele Tackx from EcoLab laboratory for the environmental samples of copepods and Jérôme Cachot from EPOC laboratory for the samples of microplastics. We also thank Urania Christaki from LOG laboratory to let us use her epifluorescence microscope for our experiments. SB acknowledge supports by the European Community (FEDER) for general support in the frame of LCFM-BIOMAT. J-MR is a FRS-FNRS Research Associate. We are grateful to the past and current members of the group of SS for maintaining the continuous plankton cultures during 2 decades. We are thankful to Shagnika Das for her help with the English and for her comments on the MS.
Supplementary Material
The Supplementary Material for this article can be found online at: https://www.frontiersin.org/articles/10.3389/fenvc.2022.905303/full#supplementary-material
References
Andrady, A. L. (2011). Microplastics in the Marine Environment. Mar. Pollut. Bull. 62 (8), 1596–1605. doi:10.1016/j.marpolbul.2011.05.030
Arthur, C., Baker, J. E., and Bamford, H. A. (2008). Proceedings of the International Research Workshop on the Occurrence, Effects, and Fate of Microplastic Marine Debris. Dissertation. Tacoma, WA: University of Washington.
Avio, C. G., Gorbi, S., and Regoli, F. (2017). Plastics and Microplastics in the Oceans: From Emerging Pollutants to Emerged Threat. Mar. Environ. Res. 128, 2–11. doi:10.1016/j.marenvres.2016.05.012
Blight, L. K., and Burger, A. E. (1997). Occurrence of Plastic Particles in Seabirds from the Eastern North Pacific. Mar. Pollut. Bull. 34 (5), 323–325. doi:10.1016/S0025-326X(96)00095-1
Campani, T., Baini, M., Giannetti, M., Cancelli, F., Mancusi, C., Serena, F., et al. (2013). Presence of Plastic Debris in Loggerhead Turtle Stranded along the Tuscany Coasts of the Pelagos Sanctuary for Mediterranean Marine Mammals (Italy). Mar. Pollut. Bull. 74 (1), 225–230. doi:10.1016/j.marpolbul.2013.06.053
Carpenter, E. J., Anderson, S. J., Harvey, G. R., Miklas, H. P., and Peck, B. B. (1972). Polystyrene Spherules in Coastal Waters. Science 178 (4062), 749–750. doi:10.1126/science.178.4062.749
Claessens, M., Van Cauwenberghe, L., Vandegehuchte, M. B., and Janssen, C. R. (2013). New Techniques for the Detection of Microplastics in Sediments and Field Collected Organisms. Mar. Pollut. Bull. 70 (1-2), 227–233. doi:10.1016/j.marpolbul.2013.03.009
Cole, M., Webb, H., Lindeque, P. K., Fileman, E. S., Halsband, C., and Galloway, T. S. (2014). Isolation of Microplastics in Biota-Rich Seawater Samples and Marine Organisms. Sci. Rep. 4, 4528. doi:10.1038/srep04528
Cole, M., Lindeque, P., Fileman, E., Halsband, C., and Galloway, T. S. (2015). The Impact of Polystyrene Microplastics on Feeding, Function and Fecundity in the Marine Copepod Calanus helgolandicus. Environ. Sci. Technol. 49 (2), 1130–1137. doi:10.1021/es504525u
Cole, M., Lindeque, P. K., Fileman, E., Clark, J., Lewis, C., Halsband, C., et al. (2016). Microplastics Alter the Properties and Sinking Rates of Zooplankton Faecal Pellets. Environ. Sci. Technol. 50 (6), 3239–3246. doi:10.1021/acs.est.5b05905
Cole, M., Coppock, R., Lindeque, P. K., Altin, D., Reed, S., Pond, D. W., et al. (2019). Effects of Nylon Microplastic on Feeding, Lipid Accumulation, and Moulting in a Coldwater Copepod. Environ. Sci. Technol. 53 (12), 7075–7082. doi:10.1021/acs.est.9b01853
Cole, M. (2016). A Novel Method for Preparing Microplastic Fibers. Sci. Rep. 6, 34519. doi:10.1038/srep34519
Coppock, R. L., Galloway, T. S., Cole, M., Fileman, E. S., Queirós, A. M., and Lindeque, P. K. (2019). Microplastics Alter Feeding Selectivity and Faecal Density in the Copepod, Calanus helgolandicus. Sci. Total Environ. 687, 780–789. doi:10.1016/j.scitotenv.2019.06.009
Das, S., Ouddane, B., Hwang, J.-S., and Souissi, S. (2020). Intergenerational Effects of Resuspended Sediment and Trace Metal Mixtures on Life Cycle Traits of a Pelagic Copepod. Environ. Pollut. 267, 115460. doi:10.1016/j.envpol.2020.115460
Dayras, P., Bialais, C., Sadovskaya, I., Lee, M.-C., Lee, J.-S., and Souissi, S. (2021). Microalgal Diet Influences the Nutritive Quality and Reproductive Investment of the Cyclopoid Copepod Paracyclopina Nana. Front. Mar. Sci. 8, 697561. doi:10.3389/fmars.2021.697561
Desforges, J.-P. W., Galbraith, M., and Ross, P. S. (2015). Ingestion of Microplastics by Zooplankton in the Northeast Pacific Ocean. Arch. Environ. Contam. Toxicol. 69, 320–330. doi:10.1007/s00244-015-0172-5
European Bioplastics (2018). Bioplastics Market Data Report. Available at: https://www.european-bioplastics.org/wp-content/uploads/2016/02/Report_Bioplastics-Market-Data_2018.pdf (Accessed October 10, 2021).
Frias, J. P. G. L., Otero, V., and Sobral, P. (2014). Evidence of Microplastics in Samples of Zooplankton from Portuguese Coastal Waters. Mar. Environ. Res. 95, 89–95. doi:10.1016/j.marenvres.2014.01.001
Gasperi, J., and Cachot, J. (2021). Flux et impacts des microplastiques dans l’estuaire de la Seine. Rouen: GIP Seine-Aval.
Jeong, C.-B., Kang, H.-M., Lee, M.-C., Kim, D.-H., Han, J., Hwang, D.-S., et al. (2017). Adverse Effects of Microplastics and Oxidative Stress-Induced MAPK/Nrf2 Pathway-Mediated Defense Mechanisms in the Marine Copepod Paracyclopina Nana. Sci. Rep. 7, 41323. doi:10.1038/srep41323
Käppler, A., Fischer, D., Oberbeckmann, S., Schernewski, G., Labrenz, M., Eichhorn, K.-J., et al. (2016). Analysis of Environmental Microplastics by Vibrational Microspectroscopy: FTIR, Raman or Both? Anal. Bioanal. Chem. 408, 8377–8391. doi:10.1007/s00216-016-9956-3
Kazour, M., Jemaa, S., Issa, C., Khalaf, G., and Amara, R. (2019). Microplastics Pollution along the Lebanese Coast (Eastern Mediterranean Basin): Occurrence in Surface Water, Sediments and Biota Samples. Sci. Total Environ. 696, 133933. doi:10.1016/j.scitotenv.2019.133933
Laist, D. W. (1997). “Impacts of Marine Debris: Entanglement of Marine Life in Marine Debris Including a Comprehensive List of Species with Entanglement and Ingestion Records,” in Marine Debris. Editors J. M. Coe, and D. B. Rogers (New York: Springer), 99–139. doi:10.1007/978-1-4613-8486-1_10
Lenz, R., Enders, K., Stedmon, C. A., Mackenzie, D. M. A., and Nielsen, T. G. (2015). A Critical Assessment of Visual Identification of Marine Microplastic Using Raman Spectroscopy for Analysis Improvement. Mar. Pollut. Bull. 100 (1), 82–91. doi:10.1016/j.marpolbul.2015.09.026
Li, S., Tenon, M., Garreau, H., Braud, C., and Vert, M. (2000). Enzymatic Degradation of Stereocopolymers Derived from L -, Dl - and Meso-Lactides. Polym. Degrad. Stab. 67 (1), 85–90. doi:10.1016/S0141-3910(99)00091-9
Li, L.-L., Amara, R., Souissi, S., Dehaut, A., Duflos, G., and Monchy, S. (2020). Impacts of Microplastics Exposure on Mussel (Mytilus edulis) Gut Microbiota. Sci. Total Environ. 745, 141018. doi:10.1016/j.scitotenv.2020.141018
Lindeque, P. K., Cole, M., Coppock, R. L., Lewis, C. N., Miller, R. Z., Watts, A. J. R., et al. (2020). Are We Underestimating Microplastic Abundance in the Marine Environment? A Comparison of Microplastic Capture with Nets of Different Mesh-Size. Environ. Pollut. 265 (A), 114721. doi:10.1016/j.envpol.2020.114721
Maes, T., Jessop, R., Wellner, N., Haupt, K., and Mayes, A. G. (2017). A Rapid-Screening Approach to Detect and Quantify Microplastics Based on Fluorescent Tagging with Nile Red. Sci. Rep. 7, 44501. doi:10.1038/srep44501
Mauchline, J. (1998). “The Biology of Calanoid Copepods,” in Advances in Marine Biology. Editor J. Mauchline (London, New York: Academic Press), 170.
Md Amin, R., Sohaimi, E. S., Anuar, S. T., and Bachok, Z. (2020). Microplastic Ingestion by Zooplankton in Terengganu Coastal Waters, Southern South China Sea. Mar. Pollut. Bull. 150, 110616. doi:10.1016/j.marpolbul.2019.110616
Michalec, F.-G., Fouxon, I., Souissi, S., and Holzner, M. (2017). Zooplankton Can Actively Adjust Their Motility to Turbulent Flow. Proc. Natl. Acad. Sci. U.S.A. 114 (52), 11199–11207. doi:10.1073/pnas.1708888114
Napper, I. E., and Thompson, R. C. (2016). Release of Synthetic Microplastic Plastic Fibres from Domestic Washing Machines: Effects of Fabric Type and Washing Conditions. Mar. Pollut. Bull. 112 (1-2), 39–45. doi:10.1016/j.marpolbul.2016.09.025
Napper, I. E., Bakir, A., Rowland, S. J., and Thompson, R. C. (2015). Characterisation, Quantity and Sorptive Properties of Microplastics Extracted from Cosmetics. Mar. Pollut. Bull. 99 (1-2), 178–185. doi:10.1016/j.marpolbul.2015.07.029
Prata, J. C., da Costa, J. P., Duarte, A. C., and Rocha-Santos, T. (2019). Methods for Sampling and Detection of Microplastics in Water and Sediment: A Critical Review. Trends Anal. Chem. 110, 150–159. doi:10.1016/j.trac.2018.10.029
Renner, G., Schmidt, T. C., and Schram, J. (2018). Analytical Methodologies for Monitoring Micro(nano)plastics: Which Are Fit for Purpose? Curr. Opin. Environ. Sci. Health 1, 55–61. doi:10.1016/j.coesh.2017.11.001
Romeo, T., Pietro, B., Pedà, C., Consoli, P., Andaloro, F., and Fossi, M. C. (2015). First Evidence of Presence of Plastic Debris in Stomach of Large Pelagic Fish in the Mediterranean Sea. Mar. Pollut. Bull. 95 (1), 358–361. doi:10.1016/j.marpolbul.2015.04.048
Setälä, O., Fleming-Lehtinen, V., and Lehtiniemi, M. (2014). Ingestion and Transfer of Microplastics in the Planktonic Food Web. Environ. Pollut. 185, 77–83. doi:10.1016/j.envpol.2013.10.013
Shim, W. J., Song, Y. K., Hong, S. H., and Jang, M. (2016). Identification and Quantification of Microplastics Using Nile Red Staining. Mar. Pollut. Bull. 113 (1-2), 469–476. doi:10.1016/j.marpolbul.2016.10.049
Song Y. K., Y. K., Hong, S. H., Jang, M., Han, G. M., Rani, M., Lee, J., et al. (2015). A Comparison of Microscopic and Spectroscopic Identification Methods for Analysis of Microplastics in Environmental Samples. Mar. Pollut. Bull. 93 (1-2), 202–209. doi:10.1016/j.marpolbul.2015.01.015
Song Y. S., Y. S., Won, Y. J., and Kim, D. Y. (2015). Time-lapse In Situ Fluorescence Lifetime Imaging of Lipid Droplets in Differentiating 3T3-L1 Preadipocytes with Nile Red. Curr. Appl. Phys. 15 (12), 1634–1640. doi:10.1016/j.cap.2015.09.006
Souissi, A., Souissi, S., and Hwang, J. S. (2016). Evaluation of the Copepod Eurytemora Affinis Life History Response to Temperature and Salinity Increases. Zool. Stud. 55, e4. doi:10.6620/ZS.2016.55-04
Strickler, J. R. (1982). Calanoid Copepods, Feeding Currents, and the Role of Gravity. Science 218 (4568), 158–160. doi:10.1126/science.218.4568.158
Sun, X., Li, Q., Zhu, M., Liang, J., Zheng, S., and Zhao, Y. (2017). Ingestion of Microplastics by Natural Zooplankton Groups in the Northern South China Sea. Mar. Pollut. Bull. 115, 217–224. doi:10.1016/j.marpolbul.2016.12.004
Sun, X., Liu, T., Zhu, M., Liang, J., Zhao, Y., and Zhang, B. (2018a). Retention and Characteristics of Microplastics in Natural Zooplankton Taxa from the East China Sea. Sci. Total Environ. 640-641, 232–242. doi:10.1016/j.scitotenv.2018.05.308
Sun, X., Liang, J., Zhu, M., Zhao, Y., and Zhang, B. (2018b). Microplastics in Seawater and Zooplankton from the Yellow Sea. Environ. Pollut. 242, 585–595. doi:10.1016/j.envpol.2018.07.014
Tanaka, K., Takada, H., Yamashita, R., Mizukawa, K., Fukuwaka, M.-a., and Watanuki, Y. (2013). Accumulation of Plastic-Derived Chemicals in Tissues of Seabirds Ingesting Marine Plastics. Mar. Pollut. Bull. 69 (1-2), 219–222. doi:10.1016/j.marpolbul.2012.12.010
Tramoy, R., Colasse, L., Gasperi, J., and Tassin, B. (2019). Plastic Debris Dataset on the Seine River Banks: Plastic Pellets, Unidentified Plastic Fragments and Plastic Sticks are the Top 3 Items in a Historical Accumulation of Plastics. Data Brief 23, 103697. doi:10.1016/j.dib.2019.01.045
Vroom, R. J. E., Koelmans, A. A., Besseling, E., and Halsband, C. (2017). Aging of Microplastics Promotes Their Ingestion by Marine Zooplankton. Environ. Pollut. 231 (1), 987–996. doi:10.1016/j.envpol.2017.08.088
Wright, S. L., Thompson, R. C., and Galloway, T. S. (2013). The Physical Impacts of Microplastics on Marine Organisms: A Review. Environ. Pollut. 178, 483–492. doi:10.1016/j.envpol.2013.02.031
Wright, S. L., Levermore, J. M., and Kelly, F. J. (2019). Raman Spectral Imaging for the Detection of Inhalable Microplastics in Ambient Particulate Matter Samples. Environ. Sci. Technol. 53 (15), 8947–8956. doi:10.1021/acs.est.8b06663
Keywords: copepods, Eurytemora affinis, microplastics, digestion protocol, nile red staining, Raman spectroscopy
Citation: Thery J, Bialais C, Kazour M, Moreau M, Dufour D, Benali S, Amara R, Monchy S, Raquez J-M and Souissi S (2022) A New Method for Microplastics Identification in Copepods. Front. Environ. Chem. 3:905303. doi: 10.3389/fenvc.2022.905303
Received: 26 March 2022; Accepted: 22 April 2022;
Published: 11 May 2022.
Edited by:
Javier Jimenez-Lamana, Institut des Sciences Analytiques et de Physico-Chimie pour l’Environnement et les Matériaux (IPREM), FranceReviewed by:
Kyari Yates, Robert Gordon University, United KingdomSong Cui, Northeast Agricultural University, China
Copyright © 2022 Thery, Bialais, Kazour, Moreau, Dufour, Benali, Amara, Monchy, Raquez and Souissi. This is an open-access article distributed under the terms of the Creative Commons Attribution License (CC BY). The use, distribution or reproduction in other forums is permitted, provided the original author(s) and the copyright owner(s) are credited and that the original publication in this journal is cited, in accordance with accepted academic practice. No use, distribution or reproduction is permitted which does not comply with these terms.
*Correspondence: Sami Souissi, c2FtaS5zb3Vpc3NpQHVuaXYtbGlsbGUuZnI=