- 1Department of Structures and Environmental Engineering, University of Agriculture, Faisalabad, Pakistan
- 2Department of Plant Pathology, University of Agriculture, Faisalabad, Pakistan
- 3School of Engineering and Technology, National Textile University, Faisalabad, Pakistan
Recent advances in graphene research have enabled the utilization of its nanocomposites for numerous energy-based and environmental applications. Recently, the advancement in graphene-based polymer nanocomposites has received much attention with special emphasis on synthesis and application. Graphene-based nanocomposites show astonishing electrical, mechanical, chemical, and thermal characteristics. Graphene nanocomposites (GNCs) are synthesized using a variety of methods, including covalent and non-covalent methods, a chemical-based deposition approach, hydrothermal growth, electrophoresis deposition, and physical deposition. Chemical methods are the most viable route for producing graphene in small quantities at low temperatures. The technique can also produce graphene films on a variety of substrate materials. The use of artificial intelligence (AI) for the synthesis of AI-created nanoparticles has recently received a lot of attention. These nanocomposite materials have excellent applications in the environmental, energy, and agricultural sectors. Due to high carrier mobility, graphene-based materials enhance the photocatalytic performance of semiconductor materials. Similarly, these materials have high potential for pollutant removal, especially heavy metals, due to their high surface area. This article highlights the synthesis of graphene-based nanocomposites with special reference to harnessing the power of modern AI tools to better understand GNC material properties and the way this knowledge can be used for its better applications in the development of a sustainable future.
1 Introduction
Carbon is abundantly present on Earth’s surface and is a major component of human life. Elements of biological life and fossil fuels all contain carbon as a major proportion. This shows the overall versatility of this element and its broad range of applications in daily life (Lam and Luong, 2014; Zeng et al., 2020). Carbon’s unique ability to create multiple bonds with each other allows it to create various forms of arrangements, ranging from linear sheet-like layers to tetrahedral structure formations (Serp and Figueiredo, 2009). This unique arrangement of carbon atoms allows the formation of various iso-forms, each possessing its own unique electrical, physical, thermal, and chemical properties. As a result, this carbon-based material can be utilized to make various kinds of products, ranging from nanofibers to carbon material sheets to more advanced stage carbon nanotube materials. In recent years, graphene has emerged as a nascent isoform of carbon and is getting heavily integrated into everyday life. This is attributed to the unique physio-chemical properties of graphene, which include a relatively higher surface area, thermal stability, mechanical strength, and high electric mobility (Gopiraman et al., 2013a).
Graphene is the basic building block for all available graphitic allotropes of carbon. It has several unique properties that make it stand out from all other allotropic forms of carbon. Ever since the groundbreaking research at the University of Manchester in 2007, it has gained great attention for application in multiple fields (Kravets et al., 2010). It has a two-dimensional structure and is often observed to be the thinnest existing material with only a single atom thickness. Inside the single layer, these atoms are interconnected in a honey comb like structure via sp2 atomic hybridization. Its unique properties include a theoretical surface area of 2,630 m2/g, a young modulus of around 1 TPa with high breaking strength, and a thermal conductivity of 5,000 W per meter per kelvin, making it a hot material for various novel applications in various fields of life (Zhu et al., 2010; Yang et al., 2013). Although it is composed of a single 2-D layer of carbon atoms, which are closely packed in a hexagonal structure, graphene is a highly flexible material and can stretch to a great extent from its original shape. Graphene is lighter than any form of existing steel, yet it is stronger than the strongest known form of steel. Although graphene materials mostly possess unique and favorable properties, allowing their wide-scale applications, a few of their attributes have some negative impacts as well. For instance, graphene layers have a lower light absorption rate and, due to their transparent nature, are utilized for the synthesis of various optoelectronic devices. However, this weak light absorption makes them less popular for the synthesis of solar energy absorption panels (Kravets et al., 2010). Collectively, this rapidly developing field of graphene applications has envisioned great potential in electronics, optoelectronics, and electrochemical and biomedical applications due to its unique structure and properties.
The conductivity of graphene is due to a single free electron in a covalent sp2 bond between carbon atoms. As a radical nanomaterial with extraordinary physical features, such as remarkably high heat conductivity and excellent electrical conductivity, graphene is gaining a lot of interest from the physical, chemical, and biomedical areas (Blake et al., 2007; Potts et al., 2011; Singh et al., 2011; Young et al., 2012) due to its biocompatibility, surface-to-volume ratio, and excellent mechanical strength (Yasmin and Daniel, 2004; Novoselov et al., 2005; Lee et al., 2008; Compton and Nguyen, 2010; Zhu et al., 2010; Chandrasekaran et al., 2014). Graphene is the most thermally conductive material known, and it has unusual electronic properties. At normal temperature, graphene possesses remarkably high electron mobility, according to experiments, and has been proposed as a transistor circuitry option. Graphene has over 200 times the electron mobility of Si and over 4 times that of III–V semiconductors (Chen et al., 2008; Pallecchi et al., 2014; Lamichhane and Ravindra, 2020). As a result, graphene would be a very appealing material for high-speed transistors.
Graphene layers are more commonly used in various structures in order to harness the potential properties of the compound. However, most recently, graphene-based nanocomposites have found a relatively quick rise in their application for numerous electrical, environmental, and biological purposes. In general, nanocomposites are materials that are made up of multiple (two or more) layers with engineered high-density interfaces and with hierarchical geometries. Nanocomposites have such physical and mechanical properties that they are higher than the corresponding single-layered structures of the same compound. Various nanocomposites are also formed by the combination of alternate layers of two different kinds of materials, which exhibit ultra–high-level strength (Mara et al., 2008). All of these characteristics of graphene and its nanocomposites were reported by different researchers along with the preparation methods and applications in the fields of the environment, energy, and agriculture. There is still a technological gap in the preparation and applications of these composites, which provides room for advancements in this domain. This article mainly highlights the conventional and AI-based preparation methods for graphene and its nanocomposites and the advanced applications of these composites in different fields.
Conclusively, this review makes use of previous research and presents a comprehensive insight into the manufacturing methods and applications of graphene-based composites. Previous review articles lack the combined approach toward conventional and AI-based synthesis of GNCs. Some articles discuss only the conventional methods of synthesis (Gorga and Cohen, 2004; Yuan et al., 2009; Rourke et al., 2011; Goenka et al., 2014; Jayasena and Melkote, 2015; Jaleh et al., 2017). Moreover, some articles mentioned the applications of GNCs in the fields of the environment sector (Hao et al., 2012; Cao and Li, 2014; Latha et al., 2016; Luo and Yang, 2017), energy sector (Choi et al., 2010; Kamat, 2010; Dimakis et al., 2015), and agricultural sector (Gomez De Arco et al., 2010; Nuvoli et al., 2011; Fan et al., 2018; Mohan and Panicker, 2019) separately. This review article, however, explored AI-based synthesizing methods and also, a combined approach toward applications in all three sectors is evaluated.
2 Structural analysis of graphene nanocomposites
In general, nano-materials are divided into various categories based on the dimension of the particles, i.e., zero-dimension, one dimension, and up to three-dimension graphene materials, which are used for a number of purposes (Tiwari et al., 2012). These materials are prepared by inter-mixing several layers of graphene and other compounds (i.e., ZnO, TiO2, etc.) (Chang et al., 2016). The chemical structure of GO is redrawn as shown in Figure 1 (Xiang et al., 2021).
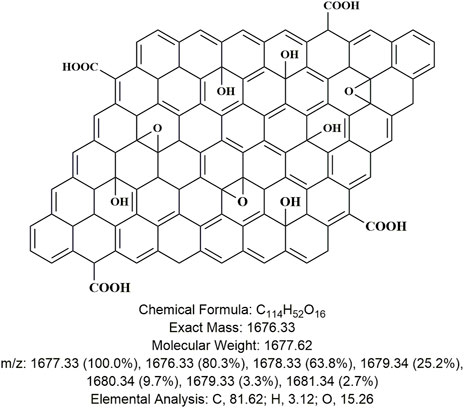
FIGURE 1. Chemical structure of graphene oxide with its chemical analysis redrawn using ChemDraw ultra 12 (Xiang et al., 2021).
Graphene comes in three different forms: graphene powder, graphene film, and graphene liquid. Mechanical stripping and SiC epitaxial growth are the most common graphene powder fabrication methods; chemical vapor deposition (CVD), electrochemical, and rapid heating methods are the most common graphene film fabrication methods; and graphene liquids are mostly graphene derivatives (Yap and Liu, 2020). Excellent mechanical strength, high electrical conductivity, strong flexibility, more surface area, and having light weight are among the features which provide it with the ability to store electric charge, ions, or hydrogen (Mahmood et al., 2014; Hu et al., 2018; Wang et al., 2019a). Despite this, the area is still in its infancy, and many experimental findings defy the theoretical explanation. For example, graphene, which is originally extremely conductive, can become a heat-proofing material when the environment is neutral (Cao et al., 2018). The oxidation of graphite in fuming nitric acid using potassium chlorate is the basis to produce GO (Green and Hersam, 2010; Jankovský et al., 2017; Khan et al., 2018; Yap and Liu, 2020).
Graphene has become the focus of numerous scientific sectors since its discovery in 2004 (Novoselov et al., 2004; Hernandez et al., 2008; Nagashio et al., 2009; Aristov et al., 2010; Bonanni and Pumera, 2011; Paredes et al., 2011; Avouris and Xia, 2012; Brownson et al., 2012; Mattevi et al., 2012; Prasai et al., 2012; Song et al., 2013; Bolotin, 2014). Because of its 2-D structure, every atom in this form of carbon is accessible for chemical reactions. It is the strongest material, with a stiffness value of 1 TPa (Lee et al., 2008). Other notable properties of graphene include a hundred times more electron mobility than silicon and a thousand times higher electrical conductivity than diamonds. Because of new technologies to make and examine graphene, research on it has exploded in the last decade. Many applications, including ultra-thin flexible displays, smart phones, chemical sensors, super-fast transistors, hydrogen storage, and biosensors, have successfully exploited and functionalized graphene (Bonaccorso et al., 2010; Wang et al., 2010; Lerner et al., 2014; El-Kady et al., 2016).
Ruan et al. (2018) used Hummer’s approach to prepare for GO. Initially, a beaker containing graphite powder and NaNO3 was filled with H2SO4 and continuously stirred. Following that, during stirring, KMnO4 in a measured quantity was added. After that, H2O2 was mixed with the reaction solution and washed with distilled water (Shi et al., 2017; Fan et al., 2018; Hou et al., 2020). According to Chang et al. (2016), the Staudenmaier method was used to prepare GO. Graphite was treated with H2SO4 and HNO3. Potassium chlorate was used to control the temperature, filtered, and then rinsed with HCL. Tan and Pumera, (2017) proved that graphite oxide can be made using the Hoffman method. The mixture of H2SO4 and HNO3 was cooled to 0°C and graphite was added rapidly. Chlorine gas was released by adding KCLO3. The temperature was brought to ambient conditions and 3 L of water was added. Then, 5% HCL was added in the reaction mixture. Centrifuge was used to separate the solid from the liquid. A vacuum oven was used to dry it for 48 h.
Many studies have been published regarding the creation of graphene-based nanocomposites, for example, to generate graphene/ZnO nanocomposites films, ZnO nanoparticles can be distributed in distilled water over graphene (Chang et al., 2016). Zhang et al. (2016) demonstrated the production of TiO2-graphene aerogel hybrids. The GO solution was first treated with anhydrous ethanol. The reactant was then sonicated after adding tetra-butyl-titanate. The combination was then moved to a Teflon-lined stainless-steel autoclave, where the TiO2-graphene hydrogel was hydrothermally treated. Han et al. (2019) used a simple sono-chemical approach to synthesize SnO2/rGO nanocomposites from SnCl2 and GO precursors. Sn2+ was homogeneously disseminated on the GO surface in the first step, and then GO was reduced by using ultrasound and adding SnO2.
To prepare graphene using the electrochemical approach, the choice of suitable electrolyte and electrolysis conditions is an important factor (Yap and Liu, 2020). The electrochemical exfoliations of charcoal and graphite were investigated using various electrolytes. The cathode was copper foil, while the anode was graphene. Electrolytes were used to soak both electrodes. Ma et al. (Ma et al., 2018) reported the mechanical exfoliation and stirring of graphite powder into honey formulating mono-and-multi–layered structures of graphene. To make graphene quantum dots, top-down and bottom-up approaches are typically utilized (Ding et al., 2018). Large-scale resources are sliced to obtain carbon-based structures and especially graphene, e.g., G-Quantumdots using the top-down method. The chemical approach is used to make graphene quantum dots from small molecular carbon sources in the bottom-up process. Because the preparation and post-treatment of graphene quantum dots can be manipulated, it is vital to carefully optimize the preparation procedure. Graphene quantum dots can be surface-passivated, functionalized, heteroatom doped, or recombined to fulfill the needs of various applications (Chen et al., 2019). The growth mechanism can be classified into two kinds (Han et al., 2017; Jo, 2018):
1) For metal substrates with a large carbon capacity, such as nickel, the mechanism of carburizing and carbon deposition is the same. When the temperature is decreased, the carbon atoms (created by the breaking of the carbon source) penetrate through the metal substrate at a high temperature, and nucleation is precipitated from inside.
2) The surface growth method nucleates graphene with high-temperature growth, in which carbon atoms are adsorbed on metal surfaces by copper and other low-carbon metal. Rapid thermal treatment (RTT) is used to make first-class graphene from silicon carbide in amorphous form (a-SiC). The SiO2/Si composite is first coated with an a-SiC film, followed by Ni and Cu films.
Carbon exists in numerous allotropes based on the type of chemical bonding. The electronic and mechanical properties of each allotrope differ. Carbon nanotubes (CNTs), graphene, and fullerenes are emerging new materials with superior properties (Potts et al., 2011; Singh et al., 2011). Carbon materials’ adaptability stems from the fact that their physical properties are highly dependent on the ratio of sp2 graphite-like to sp3 diamond-like links (Hu et al., 2018). From microcrystalline graphite to glassy carbon, there are numerous carbon structures with different hybridizations. As a result of their excellent mechanical and electrical capabilities, these materials have been extensively studied.
3 Conventional and AI-based synthesis of graphene
Due to its unique characteristics of high thermal and electrical strength, graphene has a bright future in environmental and energy applications (Potts et al., 2011; Singh et al., 2011). These applications necessitate vast amounts of graphene in different forms, e.g., low-cost nanoparticles, nanoplatelets, and nanocomposites.
3.1 Conventional preparation methods
Graphene has been prepared in a variety of ways. The electrical characteristics of graphene may be harmed by structural faults, imperfections, and wrinkles inside the material (Goenka et al., 2014). Different researchers reported many methods to produce several graphene-based nanocomposites.
Jaleh et al. (2017) utilized the co-precipitation method at low temperatures for the formation of graphene oxide/Fe3O4 (GO/Fe3O4) nanocomposites. Use of energy dispersive X-ray analysis, XRD and FT-IR, and TEM and SEM were used to determine the particle size and morphology of the prepared nanocomposites. Particle size was found to be smaller than 20 nm. GO/Fe3O4 nanocomposites were prepared using different amounts of FeCl2.4H2O and FeCl3.6H2O.
Qian et al. (2020) prepared lignin-poly (N-methylanine)-reduced graphene oxide hydrogel using a two-step method. Characteristically, a modified hummer’s method was utilized to prepare reduced-GO (Wang et al., 2018). Firstly, polymerization of NMA in the presence of an aqueous lignin solution is carried out to produce microspheres of lignin-PNMA. Secondly, the reduction-induced self-assembly method was incorporated to encapsulate the already prepared GO hydrogel nanosheets.
Nasrollahzadeh et al., (2014) reported the synthesis of GO/ZnO nanocomposites. Various tetrazoles were reported to be manufactured using this heterogeneous catalyst. Characterization was done by XRD, SEM, EDS, TEM, and UV-Vis spectroscopies. The employed method gave a high yield and provided simplicity in operation.
Depending on the desired use, graphene can be produced in following ways:
• Mechanical exfoliation (ME)
• Chemical vapor deposition (CVD)
• Liquid-phase exfoliation (LPE)
Each approach has various advantages and limits. The following is a quick summary of various strategies.
3.1.1 Exfoliation
Most of the graphene used in research during the last decade was made by mechanical exfoliation (Rourke et al., 2011; Jayasena and Melkote, 2015; Yi and Shen, 2015). In this method, adhesive tape is pressed on a specified substrate surface, and graphite samples are speeded on the tape, then the tape is peeled of (Figure 2A). Graphene fragments are spread on the substrate (Novoselov et al., 2005). The scotch tape approach produces exceptionally high-quality graphene that allows investigators to study its mechanical and physical characteristics. However, this approach is not feasible for large-scale graphene production in industrial applications.
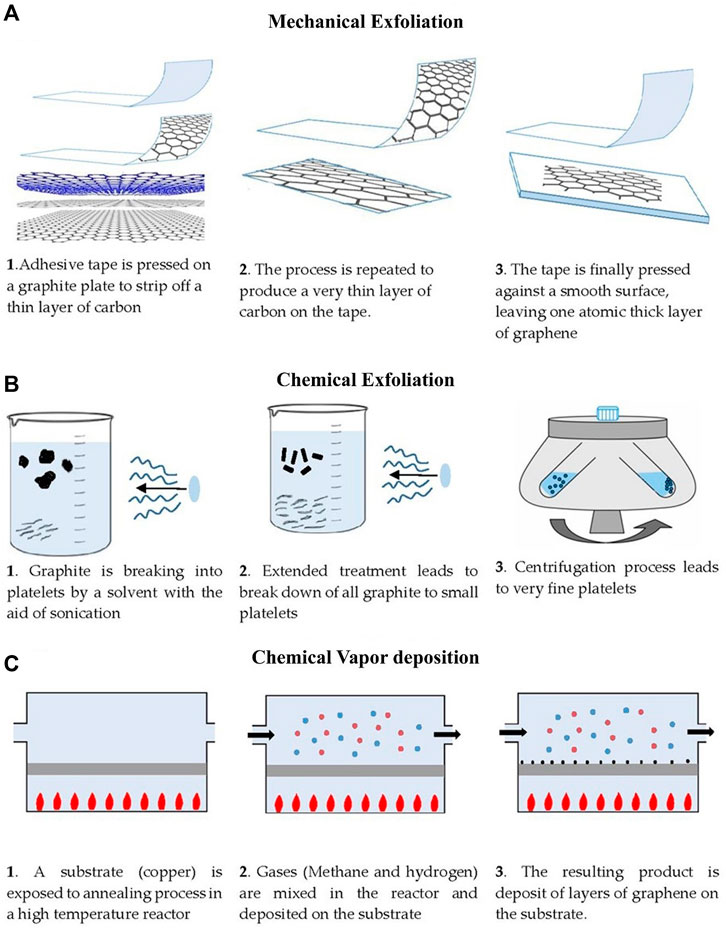
FIGURE 2. The main graphene production strategies are depicted in this diagram. (A) Exfoliation by mechanical means, (B) Liquid-phase chemical exfoliation (C) Chemical vapour deposition (CVD).
3.1.2 Liquid-phase exfoliation
This approach is extremely adaptable and can be used in a large diversity of settings and on numerous substrates. A combination of sonication and wet chemical dispersion using an appropriate solvent is the most viable route for LPE (Zhou et al., 2014). It consists of three steps: (Lam and Luong, 2014): solvent dispersion, (Zeng et al., 2020), exfoliation, and (Serp and Figueiredo, 2009) separation of the exfoliated material through cleansing as mentioned in Figure 2B. Ultrasonic force and solvent interaction are the key steps for the exfoliation processes. LPE use makes the separation of graphene layers easier. They are bound together in graphite through strong attractions, and an enormous amount of mechanical energy is required to break these bonds apart. Disrupting the attraction forces that hold the graphene layers together is one approach to lowering this energy input. This is accomplished by submerging the bulk material in a specific solvent and then exfoliating it. Nuvoli et al., (2011) used LPE of graphite in an ionic liquid to acquire highly concentrated, layered graphene sheets. This is an excellent strategy for creating fine graphene particles. High yield with low cost and scale-up skill are main advantages of the LPE processes.
3.1.3 Chemical vapor deposition
Carbon-based composites, such as graphene quantum dots and carbon nanostructures (CNTs), were preferably prepared by CVD (Eletskii et al., 2011; Zhu et al., 2013). Figure 2C depicts the thermo-catalytic breakdown of gaseous HC on a metal surface to produce graphene. It is a relatively new process for generating films of continuous 2D graphene structures with a huge surface area. In this process, a metal is placed in a furnace. Gases are being pumped into the furnace (methane and hydrogen). Hydrogen (H2) then catalyzes a reaction between methane and the surface metal, causing the methane’s carbon atoms to settle on the metal’s outer layer. A layer of graphene is deposited on the substrate as a result of the process. Different metals can be used in the CVD method, e.g., Ni, Co. A number of layers generated can be precisely controlled using the CVD process (Gopiraman et al., 2013a; Khan et al., 2018).
Seo et al. (2018) used an ambient-air CVD technique to produce permeable graphene for water desalination. No nanopores in graphene microstructures were observed, unlike earlier studies in which post-treatment procedures created nanopores on the graphene surface. Instead, they found a multilayer graphene film with multiple grain borders resulting from tiny domain widths and numerous overlapping regions of graphene. These results support graphene’s status as a 2-D nanomaterial with substantial thermal anisotropy, with great thermal conductivity in the x–y direction due to sp2 bonding in the graphene lattice, and low thermal conductivity in the z direction due to weak van der Waals interaction.
3.2 Graphene-based nanocomposites/nanopolymers
Due to its outstanding material characteristics, such as toughness, yield strength, and thermal conductivity, polymer nanocomposite (PNC) manufacturing has increased significantly over the last decade (Chang et al., 2013; Arash et al., 2014; Mahmood et al., 2014; Li and Wang, 2016; Wang and Zhi, 2016; Zope et al., 2016; Wang et al., 2019b; Khatun et al., 2020; Ibrahim et al., 2021). Traditional composite structures typically have a high proportion of filler bound (Nguyen and Nguyen, 2016). However, it has been demonstrated that micron-sized graphene can be scaled up for mass production. As a result, graphene-based composite materials are appropriate for a wide variety of applications (Lerner et al., 2014).
Significant amounts of graphene can be created by oxidizing graphite precursors. As a result, graphene-based polymer nanocomposites have piqued researchers’ curiosity all over the world. Graphene polymer nanocomposites have been made using a variety of polymers as matrices, including epoxy (Gervasoni, 2016; Wu et al., 2020a), PMMA (Ramanathan et al., 2007; Jang et al., 2009), HDPE (Zheng et al., 2004), polystyrene (Yuan et al., 2009), and nylon (Frontini and Pouzada, 2011; Goodfellow et al., 2016; Moore et al., 2018; Pierson et al., 2019; Qi et al., 2019; Schwarzer et al., 2019; Lin et al., 2022). The use of graphene-supported nanocomposites for the development of novel materials is becoming more common.
Nanocomposites have matrices consisting of polymer, metal, or ceramic, as well as fillers like nanotubes (CNTs), clay minerals, and graphene nanodots. These inclusions improve the material’s properties (Haraguchi, 2011; Mittal et al., 2015). Polymeric nanocomposites are the most versatile, with applications in a wide range of industries including energy, electronics, biomedical, and others. Polymer composites are separated into three groups based on the dispersion of nano-scale layers: intercalated nanocomposites, micro-composites, and exfoliated nanocomposites (Costantino et al., 2012).
Chemically, the compatible nature of the matrix and filler material, the concentration of the filler, and the dispersion of the filler impart different properties to graphene nanocomposites. Appropriate fabrication procedures must be used to produce the best outcomes. Furthermore, the degree of dispersion has a substantial relationship with the performance quality of nanocomposites (Frontini and Pouzada, 2011; Unalan et al., 2014; Fu et al., 2019). In situ polymerization, exfoliation adsorption, and melt intercalation are the three types of polymer nanocomposites.
3.2.1 In situ polymerization
In situ polymerization is a very effective approach during the formation of diversified graphene in a polymer matrix. In this process, the monomers join through polymerization with the stacking components. The filler is immersed in a solution of monomers. The filler expands and monomers pass through the monomer spaces. A sonicator is used to sonicate and stir the mixed solution. After an even distribution, polymerization is carried out by direct heating, a catalyst, or radiation. By incorporating additional functional groups, in situ polymerization offers higher compatibility with polymers and the dispersion of graphene on the polymers. Unlike previous processes, thermoset polymers are employed. The ability to generate strong bonds between a nano-filler and matrix is a fundamental benefit of this approach (Unalan et al., 2014; Fu et al., 2019).
3.2.2 Melt blending
Inorganic-based hybrid polymers at nano levels are preferably prepared through melt blending (Avouris and Xia, 2012). It has been determined that it is cheap and environmentally friendly. In these processes, graphene or modified graphene can be obtained without the use of any solvent. This is the traditional approach to graphene. The fundamental drawback of this approach is the graphene’s poor dispersion in the polymer matrix, particularly at higher filler loadings. This is because composites have a higher viscosity.
Melt intercalation or melt blending is the most frequent method of creating polymer nanocomposites (Gorga and Cohen, 2004; Bao et al., 2012). Thermally stable fillers are required to be used to avoid filler degradation during the process (Uhl et al., 2005). (Khvan et al., 2007) synthesized graphene/polylactic acid (PLA) nanocomposites with exceptional characteristics. PLA nanocomposites with well-dispersed graphene have dramatically increased crystallinity, mechanical properties, and electrical conductivity. The method’s main disadvantages include greater stickiness at large filler portions and a lower intercalation degree than other approaches (Jung et al., 2015).
3.2.3 Exfoliation adsorption
The use of exfoliation adsorption to produce highly adhesive forces between the matrix solution and the filler materials is a very effective approach (Vazquez et al., 2012; Unalan et al., 2014). This technique includes solution mixing and demands for the filler and matrix solvent. The filler swells as it allows the intercalation of polymer chains and increases the interlayer space. Uniform distribution is achieved through proper stirring and sonication. A multilayered structure is then achieved after removing the solvent. This approach is used to manufacture nanocomposites using polymers of low polarity. However, it is not suited for industrial use because it requires large amounts of solvents (Khvan et al., 2007; Jayasena and Melkote, 2015; Yap and Liu, 2020). Polymer nanocomposites can be made by solution mixing with a variety of polymers, including polymethylmethacrylate (PMMA) (Zhang et al., 2012a), polyurethane (PU) (Kim et al., 2010a), and polyvinyl alcohol (PVA) (Kim et al., 2010b).
Many researchers explored these techniques and similar approaches for the manufacturing of GNCs depending upon the diverse nature of graphene-based materials, their structural properties and their ultimate usage. In order to provide a more accurate and in-time evaluation of the structural integrity of graphene-based compounds, researchers are now gradually shifting toward AI-based modeling for attaining a higher design efficiency.
3.3 AI-based modeling and preparation of graphene
Recent advanced research in the chemical and material science field has highlighted the usefulness of applying various kinds of nano-materials to enhance the structural integrity and strength of compounds. Among these, graphene-based nano-materials have received great attention. Research has shown that an addition of about 0.05% graphene in concrete materials has a significant impact on the enhancement of their mechanical properties (Isfahani et al., 2016). For better understanding, binding and formation analyses of nanocomposites, image analysis of the compound is an effective process. The data gathered can then be used to improve the predictions and designs for new nanocomposite materials. In this regard, Scrivener (2004) utilized the back-scattered electron (BSE) method to visualize the structure of such compounds at the 100 nm level. It also gave crucial information regarding various size factors and presented a fractal analysis for such nanocomposites (Ruan et al., 2018). However, this approach often faced technical difficulties in observing the full-scale impact of nano-materials on the structural enhancement at the micro level. In this regard, modern artificial intelligence (AI) approaches are now providing a new view regarding the evaluation of nanocomposites. Lin et al. (2022) has used convolutional neural networks (CNN), an advanced AI approach, to evaluate the mechanism of nanomaterial reinforcements using data image processing. Conventional approaches involving such studies often required a higher degree of technicality and sophistication, making the entire process more time-consuming and laborious. In comparison to this, using an AI approach eliminates such complexities as programmed algorithms are able to analyze and learn from a given dataset and ultimately perform wide-scale image analyses and extract important findings from the image study to better understand nanocomposites (Goodfellow et al., 2016). Furthermore, these AI methods exhibit their superiority in mining features regarding spatial correlation and other dominant factors in material microstructures. Ultimately, this provides a pathway for the microstructural characterization of nano-reinforced composite materials.
The use of AI models to assess nanocomposite structures has resulted in significantly higher levels of accuracy, and the overall predictive accuracy increases with the size of the dataset provided to the program. The increase in the image dataset provided more information for the program to study and enabled it to capture and analyze information regarding the material. At a micro level, the AI extracts higher-dimensional information such as porosity, solid material shape, and the arrangement of numerous phases, providing a better understanding of its structural dynamics. This enables a better interpretation of the potential mechanism behind nano-reinforcement of composite materials, aiding the design of such nanocomposites (Lin et al., 2022).
The unique features of AI, which tend to reduce the overall computational time for analysis and preparation of high-fidelity modeling of materials, make it an effective tool in material sciences (Qi et al., 2019). Cracking or fracturing is an important phenomenon occurring in different materials and is caused by the breakage of bonds at an atomic level. Understanding the behavior of cracking in materials is important from a scientific as well as industrial point of view in order to manipulate the integrity and durability of materials. In this regard, the development of such AI models that can predict the cracking phenomenon of materials based on the atomic level bond breaking data can provide numerous possibilities during nano-scale material designing. Various AI models have been developed to detect and study cracks in materials. Moore et al. (2018) developed a random forest-based advanced AI model using data from a finite–discrete element mode in order to predict vibrant fractures in materials (Moore et al., 2018). Similarly, certain models have been developed for the detection of microscopic 3D crack structures in brittle materials and alloys at microscopic levels (Pierson et al., 2019; Schwarzer et al., 2019). Furthermore, using the data obtained from molecular dynamics, a model was constructed to predict brittle fractures in general, with another related model developed for fracture mechanism prediction in graphene as a function of its crystal orientation (Lew et al., 2021).
Elapolu et al. (2022) developed an AI model for the prediction of brittle fractures occurring in polycrystalline graphene under certain tensile stress. The model uses convolution neural networks (CNN) to predict crack growth in realistic polycrystalline graphene by processing spatial and sequential datasets. The recorded spatial features included grain boundaries as well as their orientation, whereas their sequential features were linked with crack growth. Novel image processing data were collected and fed in to the model which carried out the predictive analysis. The final results obtained via AI model analysis depict a close agreement with the molecular dynamic simulation, which is used for obtaining information related to the fracture process in pre-cracked polycrystalline graphene sheets subjected to tensile load. The corresponding model gave accurate predictions regarding the crack path of graphene with high accuracy. It also provides instantaneous results, providing an option to bypass high-cost computational dynamic simulations. These AI-based modelings are now proving to be an effective method for a quick structural evaluation and can be a vital tool in graphene-based product developments.
3.4 Characterization of graphene-based nanocomposites
Various analyses may be used to determine the physicochemical features of graphene-based nanocomposites, e.g., particle size and size distribution, shape, valence state, specific surface area, etc. As shown in Table 1, there are different techniques that can be used. As shown previously, graphene and its composites have a wide range of unique properties, making them ideal candidates for use in clean energy materials to reduce pollution and promote humanity’s long-term development.
4 Applications of graphene and its nanocomposites
Carbon-based agents are extremely adaptable and can be employed in a variety of applications (Zeng et al., 2019; Zeng et al., 2020). In the realm of heterogeneous catalysis, graphene and graphene-based composites have received a lot of attention in recent years (Gopiraman et al., 2013a; Gopiraman et al., 2013b; Gopiraman et al., 2014; Vinoth et al., 2015; Carreño et al., 2017; Wu et al., 2020a). These composites offer a lot of flexibility when it comes to tailoring the catalytic capabilities to specific needs. For example, a large surface area aids in the dispersion of nanoparticles, and so, increases the performance (Gopiraman et al., 2015a; Vuong Hoan et al., 2016; Yi et al., 2017). Furthermore, due to its high thermal stability under decreasing conditions, it is an excellent substrate for metal nano-particles (Dhand et al., 2013; Gopiraman et al., 2015b). Similarly, graphene’s high-speed electron mobility boosts the electrocatalytic activity while also improving the photocatalytic behavior by reducing the electron–hole pair recombination (Ramaswamy and Mukerjee, 2012; Ganesh Babu et al., 2015). Defect sites in graphene-based materials, on the other hand, act as nucleation locations for nanomaterials, preventing them from sintering throughout the catalytic process (Babu et al., 2016).
Graphene-based materials are categorically promising as energy and environmental support materials. This claim is backed up by the instances provided. Photovoltaics, wastewater treatment through photocatalysis, and water splitting could all benefit from graphene’s outstanding electron mobility (Hassanpour et al., 2017; Monsef et al., 2018). Graphene’s thermal and chemical resilience also makes it a good support material for nano-catalysts in a variety of applications (Blake et al., 2007; Lalwani et al., 2013). It is almost translucent to visible light, ultraviolet light, and infrared light. Graphene is a truly multidisciplinary substance that is being studied in a variety of sectors for a variety of extraordinary applications. The optical properties of graphene are promising research and application areas (Mohan et al., 2021).
Graphene oxide (GO), due to its exceptional thermal, electrical, and mechanical characteristics has attracted multiple disciplinary fields, and it has been applied in the fields of energy production, environmental rehabilitation, and water treatment/decontamination (Dreyer et al., 2010; Eda and Chhowalla, 2010; Zhou and Bongiorno, 2014). GO is being applied in different forms. Previously, computational simulation approaches were used to examine Fe adsorption on graphene (Hu et al., 2010; Nakada and Ishii, 2011; Dimakis et al., 2015; Dimakis et al., 2017). Furthermore, reduced graphene oxide (rGO) has high chemical stability, making it a viable support option.
Graphene and its composites are extensively employed in several catalyst-supported reactions, such as the oxygen reduction reaction (ORR) (Kamat, 2010; Lei et al., 2014), due to the aforementioned unique features, Fischer–Tropsch synthesis (FTS) (Berger et al., 2004; Pereira et al., 2004; Marforio et al., 2019), oxidative dehydrogenation (ODH) of ethylbenzene to styrene, oxidation of SO2 gas to SO3 (Long et al., 2011), oxidative dehydrogenation (ODH), (Lu et al., 2016), water splitting (Choi et al., 2010; Guo and Dong, 2011), selective hydrogenation (Srivastava et al., 2010), oxidative dehydrogenation (ODH) of ethylbenzene to styrene (Mestl et al., 2001; Su et al., 2005; Hass et al., 2008; Wang et al., 2019a), NOx abatement (Kim et al., 2009), catalytic purification of VOCs (Peng et al., 2010), hydrogen fuel cells (Seger and Kamat, 2009), and wastewater treatment (Dadvar et al., 2017) are only a few examples of graphene applications. There are wide applications of graphene nanomaterials, which is two-dimensional lattice structure with a hexagonal comb composed of carbon atoms and sp2 hybrid orbitals.
4.1 Pollutant degradation through photocatalysis
Global industrialization has played a critical part in the modern world’s economic growth and urbanization (Hu and Teng, 2007; Sur, 2012; Wu and Hung, 2015). The expansion of a large number of enterprises resulted in pollution, mostly water contamination, as industrial waste was dumped into the aquatic environment (Karthik et al., 2015; Kumar et al., 2016; Latha et al., 2016). As a result of the wastewater generated by industries, major difficulties have risen in aquatic living areas. Preventing industrial wastewater from mixing into the aquatic environment is a difficult undertaking, and it is preferable to minimize the effluents before they are mixed into an aquatic environment. For the degradation of pollutants generated by companies, several technologies are available (Luo and Yang, 2017; Wang et al., 2019b; Longxing et al., 2019; Zhao et al., 2019). Photocatalysis is one of them, and it has the ability to degrade/decompose wastewater–contaminated water, primarily organic contaminants (Zhang et al., 2012b; Xiang et al., 2012). A range of metal composites and carbon-based materials have been used since the introduction of photocatalysis employing semiconductor nanoparticles (Liu et al., 2017a; Zhang et al., 2017a; Zheng et al., 2019), extending the applicability of graphene to the photocatalysis processes by tuning its electronic characteristics by inserting heteroatoms or functionality (Liu et al., 2011). As a result, the photocatalytic behavior of graphene-based nanomaterials is studied in this section.
Babu et al. (2015) used nanostructured rGO-supported CuO–TiO2 for the photocatalyzed removal of methyl orange (MO). A modified Hummer’s method was used to make graphitic oxide from graphite powder. Sono-chemical techniques were utilized in preparing ex-foliateded graphene and reduced graphene oxide (rGO). Under ultrasonication, rGO is coated with a CuO–TiO2 nanocomposite was also loaded onto the surface of rGO. The authors went over to determine the photocatalytic activity of rGO. The authors compare the photocatalytic performance of several photocatalysts, including CuO/TiO2, CuO, TiO2, and rGO-loaded CuO/TiO2 nanocomposites. It was assumed that rGO-loaded CuO/TiO2 had a higher photocatalytic proficiency than other photocatalysts. They also tested the photocatalytic response in a variety of settings, including darkness, ultrasound, light, diffused sunlight, and a combination of both visible and ultrasound. According to the findings, combining ultrasound with diffused sunlight resulted in better productivity. Monsef et al. (2021) tested carbon nano-composites in lanthanide oxides showing a high degree of proficiency for electrochemical activities and are more super capacitive than either of these oxides alone.
Liu et al. (2017a) have reported the photocatalytic degradation of phenol using the composites of TiO2–G and ZnO–G. It was examined using SEM and TEM that both ZnO and TiO2 were properly imparted on a graphene surface. TEM images were also used to assess the size of these nanoparticles. When compared to pure TiO2 and ZnO nanoparticles, the rate was raised by up to 30 percent. This showed that graphene conducts electrons and suppresses recombination, while linking two composites created a more effective charge separation and extended charge carrier life, which improved the photocatalytic efficiency. Experimentation was optimized by controlling pH, intensity of light, phenol concentration, and catalyst dose. Using coupled ZnO–G/TiO2–G photocatalysts, the full degradation of a 40-ppm phenol solution took just 60 min at the optimum conditions. The fact that graphene boosts the photocatalytic performance of semiconductors is well demonstrated by these examples in the literature.
Bhunia and Jana, (2014) developed a straightforward method for synthesizing nanocomposites of rGO with a loading of silver (rGO–Ag), which showed a high removal of atrazine, phenol, and A-bisphenol in visible light. The creation of pure rGO–Ag was firmly shown by XPS and XRD studies, as well as greater crystallinity. Furthermore, the authors used TEM analysis to establish the composite nature of rGO–Ag, demonstrating the homogeneous decorating of Ag nanoparticles over the rGO surface. Furthermore, Ag nanoparticles were calculated to have an average particle size of roughly 5 nm. Experimenters experimented with different catalyst loadings e.g., 1: ½ and 1: ¼. Furthermore, the authors carried out a reusability test and, using photoluminescence spectroscopy, proposed a mechanism for photocatalytic degradation.
4.2 Heavy metal and heavy metal ion removal
Heavy metal contamination is an intensified environmental and aquatic system problem in today’s industrial world. The flow of these pollutants into the soil, water systems, and atmosphere severely pollutes ecosystems. Heavy metal ions are soluble in aquatic environments and also tend to bond with important biological constituents (i.e. proteins and nucleic acids), posing a danger to human health directly or indirectly (Jin et al., 2014). The mining sector, sewage irrigation, metal plating, electronic industry, pesticides, plastics, and fertilizers are all major sources of heavy metals (Ebrahimi et al., 2013; Maleki et al., 2015; Li et al., 2019; Saljnikov et al., 2019). Because of the increased use of heavy metals in businesses, the amount of heavy metals released into the ecosystem has recently increased (Zhong et al., 2016). Arsenic (As), cadmium (Cd), copper (Cu), chromium (Cr), lead (Pb), nickel (Ni), Mercury (Hg), and zinc (Zn) are the most frequent heavy metals found in the environment. These pollutants can cause major health problems in humans, including emphysema, kidney damage, hypertension, and cancer, even at low concentrations (Jacob et al., 2000; Cao et al., 2012; Gao et al., 2017).
Detection and elimination of these heavy metal ions from aquatic systems is important as even a trace quantity of these contaminants can cause major health problems (Motahari et al., 2015). Heavy metals are removed using a variety of technologies, including ion exchange, coagulation, reverse osmosis (RO), solvent extraction, and adsorption (Lesmana et al., 2009; Bashir et al., 2019). Among these, the adsorption approach is the most effective for removing heavy metal ions from aquatic systems.
There are numerous adsorbents in the market today, including activated carbon (Moreno-Fernandez et al., 2017), polymeric adsorbents, clay (Cantuaria et al., 2016), and palm shell (Ismaiel et al., 2013). However, the task is made more difficult by the limited performance against metal ion removal. Due to its excellent properties, e.g. greater surface area, graphene and its oxide derivatives have been efficaciously employed as adsorbents (Chowdhury and Balasubramanian, 2014; Cortés-Arriagada and Toro-Labbé, 2016). Graphene oxide (GO) has also been reported as a versatile adsorbent for the removal of heavy metal ions e.g., Au(III) and Pt (IV) (Liu et al., 2012), Pb(II) (Huang et al., 2011), Cu(II) (Yang et al., 2010), Zn(II) (Niu et al., 2013), Cd(II) (Bian et al., 2015), and Co(II) (Gao et al., 2011). Due to aggregation, Pure GO has a huge surface area, making it ineffective for heavy metal removal. As a result, the chemical treatment of GO surface was necessary to remove the heavy metal ion (Cao and Li, 2014).
4.2.1 Chromium (Cr) removal
Cr (III) and Cr (VI) are the two mostly existing oxidation states of chromium. The trivalent chromium Cr (III) is required for the insulin hormone to function properly. Cr (VI), on the other hand, is extremely harmful, being about 500 times deadlier than Cr (III). Similarly, Cr ions can exist as chromate or dichromate ions depending on the pH of the medium. These ions are both extremely soluble in water and extremely poisonous in nature. Cr (VI) is the most dangerous pollutant to the environment, according to the US Environmental Protection Agency (EPA). As a result, Cr ions must be removed from the aquatic system (Song et al., 2020). The adsorption behavior of graphene-based catalysts toward Cr removal is better than that of conventional adsorbents.
Besharat et al. (2021) used reduction techniques to explore the effectiveness of graphene catalysts include electroreduction, photoreduction, adsorption-reduction, chemical reduction and bio-reduction. Because of chromium’s high carcinogenicity to humans and marine habitats, hexavalent chromium Cr(VI)) toxicity in water resources is among the most serious environmental concerns. Because Cr(VI) is discharged into water from a variety of industrial sources, such as alloy fabrication, mining, and so on, eliminating it from water-based solutions is a major focus. Reducing Cr(VI) is said to be highly effective in removing it from aqueous systems, and has attracted a lot of attention in recent years because the end product is Cr(III), which is a non-toxic chemical and nutrient. Novel catalysts founded on nanostructures of carbon - containing materials, particularly graphene and its derivatives, have gained significant interest in heavy metal reduction and water purification due to their enormous surface area and excellent conductivity. Reduction techniques used to examine graphene catalysts include electroreduction, photoreduction, adsorption-reduction, chemical reduction (formic acid aided methods), and bio-reduction. Graphene-based catalysts have been found to have improved the photocatalytic activity and protracted stability when compared to a variety of other commercially available catalysts.
Seongpil et al. (An et al., 2017) projected a mechanism for removing heavy metals from water using graphene and its composites. Graphite was modified to make it more hydrophilic, which has a narrow water contact range. Another researcher discovered that SnS2/rGO composites lowered K2CrO7 by approximately 90%, but pure SnS2 only reduced K2CrO7 by 36% under similar conditions. For the large-scale manufacture of highly mechanically stable graphene, the authors adopted a roll-to-roll fabrication approach. Furthermore, this approach led to the creation of nano-porous GO stacking, which increased filtration. Because of their increased size and electrostatic interactions, these nanoporous graphene membranes rejected the salts (Zhou et al., 2013; Perreault et al., 2015).
4.2.2 Arsenic (As) removal
Arsenic is involved in a variety of critical physiological processes. It has an easy time attaching to proteins and enzymes, resulting in delayed metabolic abnormalities. Arsenite (As3+) and arsenate (As5+) are two oxidation states of arsenic. However, As3+ ions are shown to be more hazardous than As5+ ions (Lizama et al., 2011). Pourbeyram et al. (2016) produced graphene oxide–zirconium nanocomposites (GO–Zr) for removing both (As3+) and (As5+) from synthetic solutions. They exposed that As5+ ions are absorbed rapidly in the first 5 min, then gradually increased until equilibrium was reached after 10 min. When compared to As5+ ions, the elimination of As3+ ions took longer under similar conditions. However, As5+ has a higher removal capacity than As3+, and they were able to accomplish a complete removal with the catalyst dose of 2 mg/L in only 20 min. Zhang et al. (2010) used a series of composites of GO, iron hydroxide, and arsenic ions. Authors discovered that as-prepared composites enhanced the effectiveness of arsenic ion removal. The elimination of arsenate was most effective when the pH was between 4 and 8. At increasing pH values, however, the effectiveness of arsenate elimination decreased.
4.2.3 Cadmium (Cd), copper (Cu), and lead (Pb) removal
Li et al. developed a DTPA/magnetic graphene oxide (MGO) composite for the removal of copper, lead, and cadmium ions at low pH. In comparison to MGO, DTPA/MGO presented higher removal rates of metal ions. It is also slightly affected by pH levels (Li et al., 2017). Similarly, Hao et al. (2012) developed a metal ion solution containing copper, lead, and cadmium for competitive adsorption. It was perceived that the electrostatic interaction between lead cations and the negative surface charge of the SiO2/GO composite enhances its removal. The ability to remove other ions, on the other hand, declines, meaning that this developed composite behaves selectively. It was discovered that heavy metal ion reduction is independent of the ionic radii of ions but dependent on the electronegativity of metal ions (Gu et al., 2015; Duru et al., 2016).
4.3 Water desalination
Membranes’ inability to withstand a wide range of contaminants is a significant unsolved problem in water treatment. Recent improvements have been seen in water purification through membrane technology by constructing modified membranes with graphene films (Garaj et al., 2010; Surwade et al., 2015; Abraham et al., 2017). On the other hand, these techniques include a succession of highly concentrated and controlled, resource-conserving, sophisticated operations that are challenging to perform uniformly at greater densities. As a result, CVD graphene sheets’ potential to purify and desalinate water is limited to small-scale experiments (Surwade et al., 2015). Furthermore, while CVD synthesis allows for the precise control of graphene sheet formation, in addition, the hydrophobic characteristics of CVD-G materials typically cause further barriers to their use in water purification membranes. As a result, the economic practicability of these sheets for water filtration is hampered by these technical constraints (Sun et al., 2016).
MD is a new thermally driven water purification technology with a lot of promise for treating highly salty water especially seawater, such as reverse osmosis (Drioli et al., 2015). In the MD process, the pressure differential over a porous/hydrophobic membrane drives water purification. Because of these benefits, MD is a green technology in purification processes with zero liquid discharge (Tijing et al., 2015). MD has a few major limitations: a huge amount of energy requirement, temperature maintenance, and the MD membrane’s inability to tolerate a wide range of pollutant combinations (Drioli et al., 2015; Tijing et al., 2015). Furthermore, conduction in typical MD membranes results in poor water vapor flow and performance degradation over time, which remains a serious concern (Camacho et al., 2013). For antifouling membranes to properly handle these difficulties, new materials for antifouling membranes are required. Several approaches, such as electrospinning and phase, have been used to create improved MD membranes. However, getting large amounts of filtered water without fouling the membrane is still a problem (Lin et al., 2014; Boo et al., 2016). The use of graphene flakes in membranes has recently been shown to improve the efficacy of water purification processes (Woo et al., 2016). However, the vast potential and promises of 2D graphene films for water purification have yet to be fully employed.
Yuyang et al. (Wang et al., 2021) used an AI approach to synthesize G-based membranes for water purification. Because of their exceptional physical properties, 2-D nanomaterials, mainly graphene, have been intensively explored. The nanopore morphology and topology of such materials can have a big impact on how well they work in real-world problems. However, finding the most efficient nanopores frequently necessitates expensive and time-consuming experimentations. A study suggested a data-driven artificial intelligence framework for determining the most efficient graphene nanopore for its application in water treatment. When compared to typical circular nanopores, the MD simulations of prospective AI-created graphene nanopores show a high ion rejection rate with higher water flux as compared to other membranes. The irregular shape of AI-created pores with rough edges has been found to be a key factor in their high water desalination performance. Finally, this study shows that artificial intelligence can be a useful tool for creating and screening nanomaterials.
According to Dong et al. (Seo et al., 2018), the samples were then wet transferred to a PTFE/MD membrane. Unlike traditional CVD procedures, ambient-air graphene manufacturing does not necessitate the use of costly and potentially explosive purified compressed gases (Ruan et al., 2011). Growth source is replaced with soybean oil, which is a cheap, safe, and sustainable bio-agent. On a polycrystalline-Ni substrate, ambient-air CVD technique allows the formation of continuous graphene sheets that are suitable as water vapor-permeable channels. The PTFE membrane is used as supporting component. At the same time, the membrane can reject both salt and hazardous water-borne contaminants including surfactants and oils. Real seawater is processed through the developed membrane to demonstrate its practical applicability under real desalination circumstances. A commercial PTFE-based MD membrane was fouled during the processing of saltwater, causing a continuous decline in the water vapor flux and a small decrease in salt rejection over 72 h. A permeable graphene-based membrane, on the other hand, demonstrated 100 percent salt rejection while maintaining a high water flow and long-term stability over 72 h. Long-term resilience of permeable graphene-based membrane under actual seawater feed was also established. This study demonstrated water desalination using a graphene membrane containing nanochannels of multilayers. Graphene-based membranes had much greater water vapor flux retention and salt rejection rates than the standard distillation membranes, as well as a superior antifouling performance under a mixture of saline water containing pollutants such as oils and surfactants.
4.4 Energy production in hydrogen fuel cells
For hydrogen fuel cells, water splitting is one of the basic units that are used for hydrogen production which takes place according to the following reactions (Ali et al., 2021):
For the production of electricity directly from the chemical energy of oxygen and hydrogen, HFCs are more efficient power generation devices from which more than 50% of power can be generated efficiently (Durbin and Malardier-Jugroot, 2012). Reverse reaction of electrolyzing water is the working principle of HFCs. See Equations 1–3. Through the catalyst, hydrogen ions are generated at the anode by hydrogen when oxygen and hydrogen are supplied at the cathode and anode, respectively, from which, lost electrons generate electricity through an external circuit when they reach the cathode. On the other hand, hydrogen ions on the anode side through a polymer electrolyte membrane (PEM) reach the cathode side to generate water after the reaction with oxygen. High power, long driving distance, no noise, low emissions of pollutants, and an environmentally friendly behavior are some of the advantages of the HFCs (Xin et al., 2019). Table 2 summarizes the different catalytic reactions involved in the energy production through fuel cell.
In a study about the fabrication of GO/Nafion composite membrane, Choi et al. (Choi and Lee, 2012) explained methanol permeability, which is greatly reduced by the addition of GO as a filler. The reason for the modification of the microstructure of hydrophilic and hydrophobic PEM regions is the interaction of GO (hydrophilic nature) with non-polar main chain and cluster of polar ions. Thus, in the presence of water, phase separation of the membrane is reduced while it is improved in the case of permeability of proton exchange membrane.
In a study by Hu et al. (2014), they reported the characteristics of graphene-based membrane materials for the transportation of protons and found that good conducting membranes for protons, graphene, and boron nanocomposites could be used, which are two-dimensional and consist of a single layer of atoms.
4.5 Energy production in a polymer solar cell
Including possible solutions, flexibility, low cost, light weight, and large area coatings are all the considerable advantages that have gained interest regarding solar cells which are based on bulk heterojunction (BHJ) (Günes et al., 2007; Li et al., 2012). BHJ absorbs solar light efficiently because it consists of a mixture of polymer materials that are electron donors and acceptors, which dissociate into free charge carriers while generating excitons (van Hal et al., 2003; Xiao et al., 2018; Marforio et al., 2019).
In PSCs, photoactive blends are the most widely used acceptors that are based on poly (3,4-ethylenedioxythiophene) (P3HT) and fullerene. To increase efficiency, recently, polymers with low bandgaps have been developed which are able to absorb a wide range of solar spectra (Peet et al., 2007; Ma et al., 2015). However, commercialization of PSCs is greatly affected by many factors, which include chemical instability, low efficiency of power conversion, fast degradation of photoactive layers, and an insufficient separation of charges (Lipomi et al., 2012; Savagatrup et al., 2015).
Because of its unusual physicochemical properties and numerous applications in fuel cells, solar cells, sensors, batteries, and photocatalysis, graphene sheets with a two-dimensional structure have piqued the interest of researchers (Stoller et al., 2008; Loh et al., 2010; Chen et al., 2013; Mahmood et al., 2014; Liu et al., 2017b; Abdullah and Hashim, 2019). For PSC applications, graphene proves to be an inspiring candidate because of its tunable work function, optical transparency, and outstanding electrical conductivity (Eletskii et al., 2011; Seo et al., 2018).
Different components in PSCs have been presented in recent research studies, which are mainly based on the utilization of graphene and graphene/nanocomposites (Liu et al., 2014). Graphene offers a wide range of opportunities because of its active edges and large surface area to develop a highly efficient PSC while incorporating various polymeric, organic, and inorganic materials (Vinoth et al., 2017).
4.6 Applications in the hole-transporting layer
A successful implication of graphene oxide (GO) was carried out by Li et al. (2010) with electron donor polymers and an anode as a hole-transporting layer (HTL) due to its proper function with it. Furthermore, researchers motivated the work based on the GO buffer layers in PSC. The work function of poly (3-hexylthiophene) [P3HT] closely matches the calculated work function of GO (−4.7 eV), facilitating the hole transport from the donor polymer (P3HT) to anode. A suitable work function and an effective separation of charges mainly enhance the performance of GO sheets. Likewise, GO was represented as a HTL in a PSC inverted-type device with the architecture of ITO/ZnO/C60-SAM/P3HT: PCBM (Gao et al., 2010).
4.7 Applications in electrodes
In PSC, graphene sheets have been widely used, which are few-layered or single in nature, transparent and flexible (He et al., 2012). Graphene with the device structure of CVD-grown graphene/PEDOT: PSS/CuPc/C60/Al was used as an anode in PSC (Gomez De Arco et al., 2010). Maximum PCE displayed by the electrode of the resultant CVD-grown graphene was comparable with devices such as the PCE of ITO-based PSC. In PSC-inverted devices, a top electrode was demonstrated by CVD-grown graphene in earlier research studies (Lee et al., 2011). Remarkably, as compared to the widely used ITO anode, CVD-grown graphene exhibited better mechanical stability and also increased the PCE.
On the other hand, the bottom electrode was constructed in PSC based on P3HT: PCBM on the basis of chemically reduced graphene (rGO). The polyethylene terephthalate (PET) substrate, which is flexible, was spin-coated with rGO sheets. Under the optimized rGO thick films, almost 0.78% of the highest PCE was achieved. A graphene as the electrodes for both the anode and cathode for flexible PSC has been designed by Park et al. (2014). PSC devices significantly enhanced the PCE from 6.1% to 7.1%, which is based on the graphene anode and cathode, respectively. Recently, for PSC, a possible solution was reported by Zhang et al. rGO/silver nanowires (AgNWs)/rGO, which is based on the transparent electrode (Zhang et al., 2017b). At 550 nm, a 90% transparency was shown by the hybrid electrode rGO/AgNWs/rGO. As compared to control devices that are fabricated with an ITO electrode, the electrode rGO/AgNWs/rGO demonstrated excellent mechanical flexibility and improved photovoltaic performance.
4.8 Applications in the agricultural sector
The agriculture sector is of great importance as it provides a great deal of the materials necessary to ensure food, feed, and fiber for human life. Satisfying these basic needs of mankind in the face of climate change and rising global population is a major challenge. In order to combat such issues, an integrated technological approach is necessary to ensure global food security. Since the last decade, the discovery of graphene materials has resulted in the advancement in various fields of life, including the field of agriculture.
4.8.1 Water treatment membranes
Water is a necessary component for life sustenance throughout the world, as it supports health and hygiene on one hand and agriculture and food production on the other. According to an estimate, in order to achieve per capita water security, an annual demand of 1,200 cubic meters is required, and owing to shrinking fresh water resources around the world, questions regarding future water security around the globe are raised. During the early 1970’s, water was heavily utilized to ensure optimum soil moisture levels. This approach resulted in increased food production and, at the same time, caused various negative effects in the form of ground water depletion, river water resource depletion, and pollution of water bodies. Managing water resources is crucial from a consumption point of view, as well as maintaining the minimum fresh water bodies required for sustainable aquatic life (Fan et al., 2018).
Despite the early success in the green revolution, many parts of the world with dry climates and limited water resources experienced severe malnutrition, exposing the relative unsustainability of this approach. Water treatment for the removal of pollutants and desalination of ocean water is crucial in this perspective as ocean bodies form the largest proportion of the hydrosphere. Graphene-based layers are an effective way to reduce water pollution and increase the availability of water sources for consumption. Thin layers that are developed are called “Graphair,” and they enable the filtering of compounds through membrane distillation. Prior to this development, a wide-scale purification of water bodies by removing different pollutants was a key challenge in water treatment efforts. Graphair includes thin, multi-layered graphene which filters water passing through it by enabling permeation via nano-channels developed using graphene grains in a layer form. In contrast to ordinary filters, graphene-based filters enable the filtration of highly toxic compounds while allowing pure water with a neutral pH through them. Narrow channel systems allow smaller water molecules to pass but hinder the movement of larger dissolved molecules and ions through its channels, all while dealing with high concentrations of acid or alkaline that rapidly destroy other filtering membranes. This can allow the filtration of water and stable water supplies for drinking and agricultural purposes at remote and deserted locations (Seo et al., 2018).
4.8.2 Antimicrobial applications
Graphene has been reported to possess significant anti-microbial properties, making it a suitable candidate for the development of new anti-fungal and anti-bacterial chemicals (Wang et al., 2014; Mohan and Panicker, 2019). These microbes are responsible for causing some severe disease infections in plant species resulting in significant yield losses (Mansfield et al., 2012). Graphene oxide tends to control fungal growth by inhibiting the growth of mycelium, disrupting cell membranes, distorting electron and ion transport chains, and introducing oxidative stress (Sawangphruk et al., 2012). Graphene sheets have great anti-bacterial activity, with smaller sheets exhibiting a higher degree of anti-microbial activity. Graphene oxide nanocomposites have shown a great degree of cytotoxicity for various bacterial species, including E. coli, R. solanacearum and P. aeruginosa (Akhavan and Ghaderi, 2010; Sawangphruk et al., 2012; Mohan and Panicker, 2019). These compounds tend to rupture the cell membranes at various growth levels, resulting in the release of cellular content from the bacteria. This germicidal and preservative action of the graphene composites makes them suitable for disease-control applications, plant preservation, and promoting plant growth.
4.8.3 Smart sensors
Water is a basic necessity of life and is required by every kind of cellular life form, including plants, as they utilize water for various processes to maintain their health and vigor. In this regard, monitoring and assessment of soil moisture levels is of great significance as the optimum levels of moisture tend to promote soil health by ensuring a better uptake of nutrients required for ideal growth (Palaparthy et al., 2013). For this purpose, moisture estimation sensors are used for large field applications. However, these sensors are often rendered faulty due to fluctuating temperatures and changing concentrations of ions that manipulate soil conductivity, resulting in sensor degradation (Kabiri et al., 2017). For this reason, the development of low-cost, effective sensors that can be deployed on a large scale and are resistant to temperature drift and salt fluctuations is necessary.
In this regard, graphene oxide-based capacitive sensors have been developed which are highly sensitive to soil moisture content. These sensors have the ability to detect moisture changes in a fast detection method (response time range of 100–120 s) with relative ease. It was also observed that these graphene-based sensors showed an output change of 6% with temperature variations from 25°C to 65 °C, which leads to just 3% discrepancies for soil moisture measurements (Palaparthy et al., 2013).
4.8.4 Smart Fertilizer release system
Fertilizers are essential in ensuring growth in agriculture, and there is a need to enhance their uptake efficiency and reduce the losses to the environment. In this regard, the application of graphene in the development of new slow-release fertilizers is viewed as an important alternative to reduce these losses. It is known that, in covering fertilizer granules, a graphene layer tends to induce their physical resistance, preventing them from friction-related damages and degradation during the manufacture, transport, and application phases (Kabiri et al., 2017). In this process, the graphene oxide is composed of layers that are negatively charged. This enables it to retain cationic micronutrients including zinc (Zn), copper (Cu), and anions such as negatively charged phosphate groups. In comparison to commercial fertilizers, these graphene fertilizer mixtures reduce the possibility of soluble nutrients leaching. The addition of graphene can provide a great deal of benefits by preventing wastage and overdose of fertilizer applications and can result in a great yield of gains for high-value crops such as fruits and vegetables (Andelkovic et al., 2018).
5 Conclusion
This article begins with a brief discussion of the fundamental structure and composition of graphene materials, then, it moves on to its synthesis and application. The role of modern AI technologies has been discussed for better understanding the structure and synthesis of graphene materials in the development of more efficient nanomaterials in the near future. Graphene materials have come a long way since their discovery and are now integrated into many walks of life. These materials present a high potential as support materials in energy, agriculture, and environmental conservation, and this is evident from many of the examples discussed. As graphene-based materials provide exceptional electron mobility, they can be highly useful in photovoltaic, photocatalytic hydrolysis, and photocatalytic removal of pollutants from wastewater. A higher degree of chemical stability makes graphene suitable as a support material for nano-catalytic applications. The higher surface area of graphene materials makes them an excellent adsorbent and enable them to remove heavy metals and other related pollutants from water. These massive applications indicate the potential of graphene materials and the possibility of further integrating them into energy, water, and agriculture applications in the near future.
Author contributions
All authors listed have made a substantial, direct, and intellectual contribution to the work and approved it for publication.
Conflict of interest
The authors declare that the research was conducted in the absence of any commercial or financial relationships that could be construed as a potential conflict of interest.
Publisher’s note
All claims expressed in this article are solely those of the authors and do not necessarily represent those of their affiliated organizations, or those of the publisher, the editors, and the reviewers. Any product that may be evaluated in this article, or claim that may be made by its manufacturer, is not guaranteed or endorsed by the publisher.
Abbreviations
AI, artificial intelligence; BSE, back-scattered electron; CVD, chemical vapor deposition; CNN, convolutional neural network; CNTs, carbon nanotubes; DMFC, direct methanol fuel cell; GO, graphene oxide; GNCs, graphene nanocomposites; FTS, fischer–tropsch synthesis; HDPE, high density poly ethylene; H2SO4, sulfuric acid; HNO3, nitric acid; KMnO4, potassium permanganate; LPE, liquid-phase exfoliation; ME, mechanical exfoliation; ORR, oxygen reduction reaction; ODH, oxidative dehydrogenation; SnO2, tin oxide; RTT, rapid thermal treatment; TiO2, titanium oxide; PMMA, polymethyl methacrylate; PLA, polylactic acid; PU, polyurethane; PVA, polyvinyl alcohol; MD, membrane distillation; PEMs, proton exchange membranes; PTFE, polytetrafluoroethylene; P3HT, poly-3,4-ethylenedioxythiophene; ZnO, zinc oxide.
References
Abdullah, M. F., and Hashim, A. M. (2019). Review and assessment of photovoltaic performance of graphene/Si heterojunction solar cells. J. Mat. Sci. 54 (2), 911–948. doi:10.1007/s10853-018-2947-3
Abraham, J., Vasu, K. S., Williams, C. D., Gopinadhan, K., Su, Y., Cherian, C. T., et al. (2017). Tunable sieving of ions using graphene oxide membranes. Nat. Nanotechnol. 12 (6), 546–550. doi:10.1038/nnano.2017.21
Akhavan, O., and Ghaderi, E. (2010). Toxicity of graphene and graphene oxide nanowalls against bacteria. ACS Nano 4 (10), 5731–5736. doi:10.1021/nn101390x
Ali, M., Riaz, R., Anjum, A. S., Sun, K. C., Li, H., Jeong, S. H., et al. (2021). Graphene quantum dots induced porous orientation of holey graphene nanosheets for improved electrocatalytic activity. Carbon N. Y. 171, 493–506. doi:10.1016/j.carbon.2020.09.031
An, S., Joshi, B. N., Lee, J-G., Lee, M. W., Kim, Y. I., Kim, M-w., et al. (2017). A comprehensive review on wettability, desalination, and purification using graphene-based materials at water interfaces. Catal. Today 295, 14–25. doi:10.1016/j.cattod.2017.04.027
Andelkovic, I. B., Kabiri, S., Tavakkoli, E., Kirby, J. K., McLaughlin, M. J., Losic, D. J., et al. (2018). Graphene oxide-Fe (III) composite containing phosphate–A novel slow release fertilizer for improved agriculture management. J. Clean. Prod. 185, 97–104. doi:10.1016/j.jclepro.2018.03.050
Arash, B., Wang, Q., and Varadan, V. (2014). Mechanical properties of carbon nanotube/polymer composites. Sci. Rep. 4 (1), 6479. doi:10.1038/srep06479
Aristov, V. Y., Urbanik, G., Kummer, K., Vyalikh, D. V., Molodtsova, O. V., Preobrajenski, A. B., et al. (2010). Graphene synthesis on cubic SiC/Si wafers. Perspectives for mass production of graphene-based electronic devices. Nano Lett. 10 (3), 992–995. doi:10.1021/nl904115h
Avouris, P., and Xia, F. (2012). Graphene applications in electronics and photonics. MRS Bull. 37 (12), 1225–1234. doi:10.1557/mrs.2012.206
Babu, S. G., Gopiraman, M., Deng, D., Wei, K., Karvembu, R., Kim, I. S, et al. (2016). Robust Au–Ag/graphene bimetallic nanocatalyst for multifunctional activity with high synergism. Chem. Eng. J. 300, 146–159. doi:10.1016/j.cej.2016.04.101
Babu, S. G., Vijayan, A. S., Neppolian, B., and Ashokkumar, M. J. (2015). SnS2/rGO: An efficient photocatalyst for the complete degradation of organic contaminants. Mat. focus 4 (4), 272–276. doi:10.1166/mat.2015.1247
Bao, C., Song, L., Xing, W., Yuan, B., Wilkie, C. A., Huang, J., et al. (2012). Preparation of graphene by pressurized oxidation and multiplex reduction and its polymer nanocomposites by masterbatch-based melt blending. J. Mat. Chem. 22 (13), 6088. doi:10.1039/c2jm16203b
Bashir, A., Malik, L. A., Ahad, S., Manzoor, T., Bhat, M. A., Dar, G., et al. (2019). Removal of heavy metal ions from aqueous system by ion-exchange and biosorption methods. Environ. Chem. Lett. 17 (2), 729–754. doi:10.1007/s10311-018-00828-y
Berger, C., Song, Z., Li, T., Li, X., Ogbazghi, A. Y., Feng, R., et al. (2004). Ultrathin epitaxial graphite: 2D electron gas properties and a route toward graphene-based nanoelectronics. J. Phys. Chem. B 108 (52), 19912–19916. doi:10.1021/jp040650f
Besharat, F., Ahmadpoor, F., and Nasrollahzadeh, M. J. (2021). Graphene-based (nano) catalysts for the reduction of Cr (VI): A review. J. Mol. Liq. 334, 116123. doi:10.1016/j.molliq.2021.116123
Bhunia, S. K., and Jana, N. R. J. (2014). Reduced graphene oxide-silver nanoparticle composite as visible light photocatalyst for degradation of colorless endocrine disruptors. ACS Appl. Mat. Interfaces 6 (22), 20085–20092. doi:10.1021/am505677x
Bian, Y., Bian, Z-Y., Zhang, J-X., Ding, A-Z., Liu, S-L., Wang, H. J., et al. (2015). Effect of the oxygen-containing functional group of graphene oxide on the aqueous cadmium ions removal. Appl. Surf. Sci. 329, 269–275. doi:10.1016/j.apsusc.2014.12.090
Blake, P., Hill, E., Castro Neto, A., Novoselov, K., Jiang, D., Yang, R., et al. (2007). Appl. Phys. Lett. 91 (6), 063124. doi:10.1063/1.2768624
Bolotin, K. J. (2014). Graphene, 199–227. doi:10.1533/9780857099334.3.199Electronic transport in graphene: Towards high mobility
Bonaccorso, F., Sun, Z., Hasan, T., and Ferrari, A. C. (2010). Graphene photonics and optoelectronics. Nat. Photonics 4 (9), 611–622. doi:10.1038/nphoton.2010.186
Bonanni, A., and Pumera, M. (2011). Graphene platform for hairpin-DNA-based impedimetric genosensing. ACS Nano 5 (3), 2356–2361. doi:10.1021/nn200091p
Boo, C., Lee, J., and Elimelech, M. J. (2016). Omniphobic polyvinylidene fluoride (PVDF) membrane for desalination of shale gas produced water by membrane distillation. Environ. Sci. Technol. 50 (22), 12275–12282. doi:10.1021/acs.est.6b03882
Brownson, D. A., Kampouris, D. K., and Banks, C. E. (2012). Graphene electrochemistry: Fundamental concepts through to prominent applications. Chem. Soc. Rev. 41 (21), 6944. doi:10.1039/c2cs35105f
Calovi, M., Callone, E., Ceccato, R., Deflorian, F., Rossi, S., Dirè, S., et al. (2019). Effect of the organic functional group on the grafting ability of trialkoxysilanes onto graphene oxide: A combined nmr, XRD, and esr study. Materials 12 (23), 3828. doi:10.3390/ma12233828
Camacho, L. M., Dumée, L., Zhang, J., Li, J-d., Duke, M., Gomez, J., et al. (2013). Advances in membrane distillation for water desalination and purification applications. Water 5 (1), 94–196. doi:10.3390/w5010094
Cantuaria, M. L., de Almeida Neto, A. F., Nascimento, E. S., and MGJJocp, V. (2016). Adsorption of silver from aqueous solution onto pre-treated bentonite clay: Complete batch system evaluation. J. Clean. Prod. 112, 1112–1121. doi:10.1016/j.jclepro.2015.07.021
Cao, C-Y., Qu, J., Wei, F., Liu, H., and Song, W-G. (2012). Superb adsorption capacity and mechanism of flowerlike magnesium oxide nanostructures for lead and cadmium ions. ACS Appl. Mat. Interfaces 4 (8), 4283–4287. doi:10.1021/am300972z
Cao, Y., Fatemi, V., Demir, A., Fang, S., Tomarken, S. L., Luo, J. Y., et al. (2018). Correlated insulator behaviour at half-filling in magic-angle graphene superlattices. Nature 556 (7699), 80–84. doi:10.1038/nature26154
Cao, Y., and Li, X. J. (2014). Adsorption of graphene for the removal of inorganic pollutants in water purification: A review. Adsorpt. Bost. 20 (5), 713–727. doi:10.1007/s10450-014-9615-y
Carreño, N., Barbosa, A., Duarte, V., Correa, C., Ferrúa, C., Nedel, F., et al. (2017). Metal-carbon interactions on reduced graphene oxide under facile thermal treatment: Microbiological and cell assay, 2017.
Chandrasekaran, S., Sato, N., Tölle, F., Mülhaupt, R., Fiedler, B., Schulte, K., et al. (2014). Fracture toughness and failure mechanism of graphene based epoxy composites. Compos. Sci. Technol. 97, 90–99. doi:10.1016/j.compscitech.2014.03.014
Chang, H., Wu, H. J. E., and Science, E. (2013). Graphene-based nanocomposites: Preparation, functionalization, and energy and environmental applications. Energy Environ. Sci. 6 (12), 3483. doi:10.1039/c3ee42518e
Chang, W-C., Tseng, T-C., Yu, W-C., Lan, Y-Y., and GerM-D, (2016). Graphene/ZnO nanoparticle composite photoelectrodes for dye-sensitized solar cells with enhanced photovoltaic performance. J. Nanosci. Nanotechnol. 16 (9), 9160–9165. doi:10.1166/jnn.2016.12900
Chen, J-H., Jang, C., Xiao, S., Ishigami, M., and Fuhrer, M. S. (2008). Intrinsic and extrinsic performance limits of graphene devices on SiO2. Nat. Nanotechnol. 3 (4), 206–209. doi:10.1038/nnano.2008.58
Chen, L., Zhang, M., and Wei, W. (2013). Graphene-based composites as cathode materials for lithium ion batteries. J. Nanomater. 2013, 1–8. doi:10.1155/2013/940389
Chen, X., Yang, H., Wu, B., Wang, L., Fu, Q., Liu, Y., et al. (2019). Epitaxial growth of h‐BN on templates of various dimensionalities in h‐BN–graphene material systems. Adv. Mat. 31 (12), 1805582. doi:10.1002/adma.201805582
Cheng, D., Wu, P., Wang, J., Tang, X., An, T., Zhou, H., et al. (2019). Synergetic pore structure optimization and nitrogen doping of 3D porous graphene for high performance lithium sulfur battery. Carbon N. Y. 143, 869–877. doi:10.1016/j.carbon.2018.11.032
Choi, K., and Lee, W. (2012). Enhanced degradation of trichloroethylene in nano-scale zero-valent iron Fenton system with Cu (II). J. Hazard. Mat. 211, 146–153. doi:10.1016/j.jhazmat.2011.10.056
Choi, W., Lahiri, I., Seelaboyina, R., Kang, Y. S., and Sciences, M. (2010). Synthesis of graphene and its applications: A review. Crit. Rev. Solid State Mater. Sci. 35 (1), 52–71. doi:10.1080/10408430903505036
Chowdhury, S., and Balasubramanian, R. (2014). Recent advances in the use of graphene-family nanoadsorbents for removal of toxic pollutants from wastewater. Adv. Colloid Interface Sci. 204, 35–56. doi:10.1016/j.cis.2013.12.005
Compton, O. C., and Nguyen, S. T. (2010). Graphene oxide, highly reduced graphene oxide, and graphene: Versatile building blocks for carbon‐based materials. Small 6 (6), 711–723. doi:10.1002/smll.200901934
Cortés-Arriagada, D., and Toro-Labbé, A. J. (2016). Aluminum and iron doped graphene for adsorption of methylated arsenic pollutants. Appl. Surf. Sci. 386, 84–95. doi:10.1016/j.apsusc.2016.05.154
Costantino, A., Pettarin, V., Viana, J., Pontes, A., Pouzada, A., Frontini, P., et al. (2012). Microstructure of PP/clay nanocomposites produced by shear induced injection moulding. Procedia Mater. Sci. 1, 34–43. doi:10.1016/j.mspro.2012.06.005
Dadvar, E., Kalantary, R. R., Ahmad Panahi, H., and Peyravi, M. J. (2017). Efficiency of polymeric membrane graphene oxide-TiO2 for removal of azo dye. J. Chem. 2017, 1–13. doi:10.1155/2017/6217987
Devrim, Y., Arıca, E. D., and Albostan, A. J. (2018). Graphene based catalyst supports for high temperature PEM fuel cell application. Int. J. Hydrogen Energy 43 (26), 11820–11829. doi:10.1016/j.ijhydene.2018.03.047
Dhand, V., Rhee, K. Y., Ju Kim, H., and Jung, H. D. (2013). A comprehensive review of graphene nanocomposites: Research status and trends. J. Nanomater. 2013, 1–14. doi:10.1155/2013/763953
Dimakis, N., Flor, F. A., Salgado, A., Adjibi, K., Vargas, S., Saenz, J., et al. (2017). Density functional theory calculations on transition metal atoms adsorbed on graphene monolayers. Appl. Surf. Sci. 421, 252–259. doi:10.1016/j.apsusc.2016.10.144
Dimakis, N., Navarro, N. E., Velazquez, J., and Salgado, A. (2015). Electronic and vibrational properties of graphene monolayers with iron adatoms: A density functional theory study. Appl. Surf. Sci. 334, 2–6. doi:10.1016/j.apsusc.2014.06.126
Ding, Z., Li, F., Wen, J., Wang, X., and Sun, R. (2018). Gram-scale synthesis of single-crystalline graphene quantum dots derived from lignin biomass. Green Chem. 20 (6), 1383–1390. doi:10.1039/c7gc03218h
Drioli, E., Ali, A., and Macedonio, F. J. (2015). Membrane distillation: Recent developments and perspectives. Desalination 356, 56–84. doi:10.1016/j.desal.2014.10.028
Durbin, D., and Malardier-Jugroot, C. (2012). Theoretical investigation of the use of doped graphene as a membrane support for effective CO removal in hydrogen fuel cells. Mol. Simul. 38 (13), 1061–1071. doi:10.1080/08927022.2012.690876
Duru, İ., Ege, D., and Kamali, A. R. (2016). Graphene oxides for removal of heavy and precious metals from wastewater. J. Mat. Sci. 51 (13), 6097–6116. doi:10.1007/s10853-016-9913-8
Ebrahimi, R., Maleki, A., Shahmoradi, B., Daraei, H., Mahvi, A. H., Barati, A. H., et al. (2013). Elimination of arsenic contamination from water using chemically modified wheat straw. Desalination Water Treat. 51 (10-12), 2306–2316. doi:10.1080/19443994.2012.734675
Eda, G., and Chhowalla, M. (2010). Chemically derived graphene oxide: Towards large‐area thin‐film electronics and optoelectronics. Adv. Mat. 22 (22), 2392–2415. doi:10.1002/adma.200903689
El-Kady, M. F., Shao, Y., and Kaner, R. B. (2016). Graphene for batteries, supercapacitors and beyond. Nat. Rev. Mat. 1 (7), 16033. doi:10.1038/natrevmats.2016.33
Elapolu, M. S., Shishir, M. I. R., and Tabarraei, A. A novel approach for studying crack propagation in polycrystalline graphene using machine learning algorithms. 2022;201:110878. doi:10.1016/j.commatsci.2021.110878
Eletskii, A. V., Iskandarova, I. M., Knizhnik, A. A., and Krasikov, D. N. J. (2011), Graphene: fabrication methods and thermophysical properties. Phys. -Usp. 54 (3), 227–258. doi:10.3367/ufne.0181.201103a.0233
Fan, M., Hu, J., Cao, R., Ruan, W., and Wei, X. J. C. (2018). A review on experimental design for pollutants removal in water treatment with the aid of artificial intelligence, 200, 330–343.
Frontini, P. M., and Pouzada, A. S. (2011). Is there any chance for polypropylene/clay nanocomposites in injection molding? Express Polym. Lett. 5 (8), 661. doi:10.3144/expresspolymlett.2011.64
Fu, S., Sun, Z., Huang, P., Li, Y., and Hu, N. J. (2019). Some basic aspects of polymer nanocomposites: A critical review. Nano Mater. Sci. 1 (1), 2–30. doi:10.1016/j.nanoms.2019.02.006
Ganesh Babu, S., Vinoth, R., Surya Narayana, P., Bahnemann, D., and Neppolian, B. (2015). Reduced graphene oxide wrapped Cu2O supported on C3N4: An efficient visible light responsive semiconductor photocatalyst. Apl. Mat. 3 (10), 104415. doi:10.1063/1.4928286
Gao, W., Majumder, M., Alemany, L. B., Narayanan, T. N., Ibarra, M. A., Pradhan, B. K., et al. (2011). Engineered graphite oxide materials for application in water purification. ACS Appl. Mat. Interfaces 3 (6), 1821–1826. doi:10.1021/am200300u
Gao, Y., Yip, H-L., Hau, S. K., O’Malley, K. M., Cho, N. C., Chen, H., et al. (2010). Anode modification of inverted polymer solar cells using graphene oxide. Appl. Phys. Lett. 97 (20), 203306. doi:10.1063/1.3507388
Gao, Z., Cao, J., Yan, J., Wang, J., Cai, S., and Yan, C. (2017). Blood lead levels and risk factors among preschool children in a lead polluted area in Taizhou. China. BioMed Res. Int. 2017, 1–8. doi:10.1155/2017/4934198
Garaj, S., Hubbard, W., Reina, A., Kong, J., Branton, D., Golovchenko, J. J., et al. (2010). Graphene as a subnanometre trans-electrode membrane. Nature 467 (7312), 190–193. doi:10.1038/nature09379
Gervasoni, J. L. (2016). Straightforward routes for the preparation of graphene-based polymer nanocomposites. Graphene Sci. Handb., 193–208. CRC Press. doi:10.1201/b19488-22
Goenka, S., Sant, V., and Sant, S. J. JoC. R. (2014). Graphene-based nanomaterials for drug delivery and tissue engineering. J. Control. Release 173, 75–88. doi:10.1016/j.jconrel.2013.10.017
Gomez De Arco, L., Zhang, Y., Schlenker, C. W., Ryu, K., Thompson, M. E., Zhou, C. J., et al. (2010). Continuous, highly flexible, and transparent graphene films by chemical vapor deposition for organic photovoltaics. ACS Nano 4 (5), 2865–2873. doi:10.1021/nn901587x
Gopiraman, M., Babu, S. G., Khatri, Z., Kim, B-S., Wei, K., Karvembu, R., et al. (2015). Photodegradation of dyes by a novel TiO2/u-RuO2/GNS nanocatalyst derived from Ru/GNS after its use as a catalyst in the aerial oxidation of primary alcohols (GNS= graphene nanosheets). Reac. Kinet. Mech. Cat. 115 (2), 759–772. doi:10.1007/s11144-015-0861-0
Gopiraman, M., Babu, S. G., Khatri, Z., Wei, K., Endo, M., Karvembu, R., et al. (2013). Facile and homogeneous decoration of RuO 2 nanorods on graphene nanoplatelets for transfer hydrogenation of carbonyl compounds. Catal. Sci. Technol. 3 (6), 1485. doi:10.1039/c3cy20735h
Gopiraman, M., Bang, H., Babu, S. G., Wei, K., Karvembu, R., Kim, I. S., et al. (2014). Catalytic N-oxidation of tertiary amines on RuO 2 NPs anchored graphene nanoplatelets. Catal. Sci. Technol. 4 (7), 2099. doi:10.1039/c3cy00963g
Gopiraman, M., Deng, D., Ganesh Babu, S., Hayashi, T., Karvembu, R., Kim, I. S., et al. (2015). Sustainable and versatile CuO/GNS nanocatalyst for highly efficient base free coupling reactions. ACS Sustain. Chem. Eng. 3 (10), 2478–2488. doi:10.1021/acssuschemeng.5b00542
Gopiraman, M., Ganesh Babu, S., Khatri, Z., Kai, W., Kim, Y. A., Endo, M., et al. (2013). Dry synthesis of easily tunable nano ruthenium supported on graphene: Novel nanocatalysts for aerial oxidation of alcohols and transfer hydrogenation of ketones. J. Phys. Chem. C 117 (45), 23582–23596. doi:10.1021/jp402978q
Gorga, R. E., and Cohen, R. E. (2004). Toughness enhancements in poly (methyl methacrylate) by addition of oriented multiwall carbon nanotubes. J. Polym. Sci. B. Polym. Phys. 42 (14), 2690–2702. doi:10.1002/polb.20126
Green, A. A., and Hersam, M. C. (2010). Emerging methods for producing monodisperse graphene dispersions. J. Phys. Chem. Lett. 1 (2), 544–549. doi:10.1021/jz900235f
Gu, D., Fein, J. B. J. C., Physicochemical, S. A., and Aspects, E. (2015). Adsorption of metals onto graphene oxide: Surface complexation modeling and linear free energy relationships. Colloids Surfaces A Physicochem. Eng. Aspects 481, 319–327. doi:10.1016/j.colsurfa.2015.05.026
Günes, S., Neugebauer, H., and Sariciftci, N. S. (2007). Conjugated polymer-based organic solar cells. Chem. Rev. 107 (4), 1324–1338. doi:10.1021/cr050149z
Guo, S., and Dong, S. J. (2011). Graphene nanosheet: Synthesis, molecular engineering, thin film, hybrids, and energy and analytical applications. Chem. Soc. Rev. 40 (5), 2644. doi:10.1039/c0cs00079e
Han, D., Wang, X., Zhao, Y., Chen, Y., Tang, M., Zhao, Z. J., et al. (2017). High-quality graphene synthesis on amorphous SiC through a rapid thermal treatment. Carbon N. Y. 124, 105–110. doi:10.1016/j.carbon.2017.06.002
Han, X., Li, R., Qiu, S., Zhang, X., Zhang, Q., Yang, Y., et al. (2019). Sonochemistry-enabled uniform coupling of SnO 2 nanocrystals with graphene sheets as anode materials for lithium-ion batteries. RSC Adv. 9 (11), 5942–5947. doi:10.1039/c9ra00554d
Hao, L., Song, H., Zhang, L., Wan, X., Tang, Y., Lv, Y., et al. (2012). SiO2/graphene composite for highly selective adsorption of Pb (II) ion. J. Colloid Interface Sci. 369 (1), 381–387. doi:10.1016/j.jcis.2011.12.023
Haraguchi, K. J. (2011). Synthesis and properties of soft nanocomposite materials with novel organic/inorganic network structures. Polym. J. 43 (3), 223–241. doi:10.1038/pj.2010.141
Hass, J., De Heer, W., and Conrad, E. J. (2008). The growth and morphology of epitaxial multilayer graphene. J. Phys. Condens. Matter 20 (32), 323202. doi:10.1088/0953-8984/20/32/323202
Hassanpour, M., Safardoust-Hojaghan, H., and Salavati-Niasari, M. J. (2017). Degradation of methylene blue and Rhodamine B as water pollutants via green synthesized Co3O4/ZnO nanocomposite. J. Mol. Liq. 229, 293–299. doi:10.1016/j.molliq.2016.12.090
He, M., Jung, J., Qiu, F., and Lin, Z. J. (2012). Graphene-based transparent flexible electrodes for polymer solar cells. J. Mat. Chem. 22 (46), 24254. doi:10.1039/c2jm33784c
Hernandez, Y., Nicolosi, V., Lotya, M., Blighe, F. M., Sun, Z., De, S., et al. (2008). High-yield production of graphene by liquid-phase exfoliation of graphite. Nat. Nanotechnol. 3 (9), 563–568. doi:10.1038/nnano.2008.215
Hou, Y., Qi, J., Hu, J., Xiang, Y., Xin, L., Wei, X. J. P., et al. (2020). Mesoporous Mn-Doped Fe nanoparticle-modified reduced graphene oxide for ethyl violet elimination: Modeling and optimization using artificial intelligence. Process. (Basel). 8 (4), 488. doi:10.3390/pr8040488
Hu, C., Liu, D., Xiao, Y., and Dai, L. (2018). Functionalization of graphene materials by heteroatom-doping for energy conversion and storage. Prog. Nat. Sci. Mater. Int. 28 (2), 121–132. doi:10.1016/j.pnsc.2018.02.001
Hu, C-C., and Teng, H. J. (2007). Influence of structural features on the photocatalytic activity of NaTaO3 powders from different synthesis methods. Appl. Catal. A General 331, 44–50. doi:10.1016/j.apcata.2007.07.024
Hu, L., Hu, X., Wu, X., Du, C., Dai, Y., Deng, J., et al. (2010). Density functional calculation of transition metal adatom adsorption on graphene. Phys. B Condens. Matter 405 (16), 3337–3341. doi:10.1016/j.physb.2010.05.001
Hu, S., Lozada-Hidalgo, M., Wang, F., Mishchenko, A., Schedin, F., Nair, R. R., et al. (2014). Proton transport through one-atom-thick crystals. Nature 516 (7530), 227–230. doi:10.1038/nature14015
Huang, Z-H., Zheng, X., Lv, W., Wang, M., Yang, Q-H., Kang, F. J., et al. (2011). Adsorption of lead (II) ions from aqueous solution on low-temperature exfoliated graphene nanosheets. Langmuir 27 (12), 7558–7562. doi:10.1021/la200606r
Ibrahim, A., Klopocinska, A., Horvat, K., and Abdel Hamid, Z. J. P. (2021). Graphene-based nanocomposites: Synthesis, mechanical properties, and characterizations. Polym. (Basel). 13 (17), 2869. doi:10.3390/polym13172869
Iqbal, M. A., Liaqat, A., Hussain, S., Wang, X., Tahir, M., Urooj, Z., et al. (2020). Ultralow‐Transition‐energy organic complex on graphene for high‐performance shortwave infrared photodetection. Adv. Mat. 32 (37), 2002628. doi:10.1002/adma.202002628
Isfahani, F. T., Li, W., Redaelli, E. J. C., and Composites, C. (2016). Dispersion of multi-walled carbon nanotubes and its effects on the properties of cement composites. Cem. Concr. Compos. 74, 154–163. doi:10.1016/j.cemconcomp.2016.09.007
Ismaiel, A. A., Aroua, M. K., and Yusoff, R. J. (2013). Palm shell activated carbon impregnated with task-specific ionic-liquids as a novel adsorbent for the removal of mercury from contaminated water. Chem. Eng. J. 225, 306–314. doi:10.1016/j.cej.2013.03.082
Jacob, B., Ritz, B., Heinrich, J., Hoelscher, B., and Wichmann, H-E. (2000). The effect of low-level blood lead on hematologic parameters in children. Environ. Res. 82 (2), 150–159. doi:10.1006/enrs.1999.4011
Jaleh, B., Khalilipour, A., Habibi, S., Niyaifar, M., and Nasrollahzadeh, M. J. (2017). Synthesis, characterization, magnetic and catalytic properties of graphene oxide/Fe3O4. J. Mat. Sci. Mat. Electron. 28 (6), 4974–4983. doi:10.1007/s10854-016-6151-4
Jang, J. Y., Kim, M. S., Jeong, H. M., and Shin, C. M. (2009). Graphite oxide/poly (methyl methacrylate) nanocomposites prepared by a novel method utilizing macroazoinitiator. Compos. Sci. Technol. 69 (2), 186–191. doi:10.1016/j.compscitech.2008.09.039
Jankovský, O., Nováček, M., Luxa, J., Sedmidubský, D., Boháčová, M., Pumera, M., et al. (2017). Concentration of nitric acid strongly influences chemical composition of graphite oxide. Chem. Eur. J. 23 (26), 6432–6440. doi:10.1002/chem.201700809
Jayasena, B., and Melkote, S. N. J. (2015). An investigation of PDMS stamp assisted mechanical exfoliation of large area graphene. Procedia Manuf. 1, 840–853. doi:10.1016/j.promfg.2015.09.073
Jin, L., Zhang, G., and Tian, H. J. (2014). Current state of sewage treatment in China. Water Res. 66, 85–98. doi:10.1016/j.watres.2014.08.014
Jo, S. S. (2018). Chemical vapor deposition (CVD) growth and optimal transfer processes for graphene. Midsize City: Massachusetts Institute of Technology.
Jung, H., Yu, S., Bae, N-S., Cho, S. M., Kim, R. H., Cho, S. H., et al. (2015). High through-plane thermal conduction of graphene nanoflake filled polymer composites melt-processed in an L-shape kinked tube. ACS Appl. Mat. Interfaces 7 (28), 15256–15262. doi:10.1021/acsami.5b02681
Kabiri, S., Degryse, F., Tran, D. N., da Silva, R. C., McLaughlin, M. J., Losic, D. J., et al. (2017). Graphene oxide: A new carrier for slow release of plant micronutrients. ACS Appl. Mat. Interfaces 9 (49), 43325–43335. doi:10.1021/acsami.7b07890
Kamat, P. V. J. (2010). Graphene-based nanoarchitectures. Anchoring semiconductor and metal nanoparticles on a two-dimensional carbon support. J. Phys. Chem. Lett. 1 (2), 520–527. doi:10.1021/jz900265j
Karthik, P., Vinoth, R., Babu, S. G., Wen, M., Kamegawa, T., Yamashita, H., et al. (2015). Synthesis of highly visible light active TiO 2-2-naphthol surface complex and its application in photocatalytic chromium (vi) reduction. RSC Adv. 5 (50), 39752–39759. doi:10.1039/c5ra03831f
Khan, A., Habib, M. R., Kumar, R. R., Islam, S. M., Arivazhagan, V., Salman, M., et al. (2018). Wetting behaviors and applications of metal-catalyzed CVD grown graphene. J. Mat. Chem. A Mat. 6 (45), 22437–22464. doi:10.1039/c8ta08325h
Khatun, F., Aziz, A., and Sim, L. (2020). “Preparation of reduced graphene oxide (RGO) modified titanium dioxide nanotube (TNTs) as visible light effective catalyst for the conversion of CO2 to CH4,” in IOP Conference Series: Materials Science and Engineering (Ho Chi Minh City, Vietnam: IOP Publishing).
Khvan, S., Kim, J., and Lee, S-S. (2007). Fabrication of pre-exfoliated clay masterbatch via exfoliation-adsorption of polystyrene nanobeads. Macromol. Res. 15 (1), 51–58. doi:10.1007/bf03218752
Kim, H., Miura, Y., and Macosko, C. W. (2010). Graphene/polyurethane nanocomposites for improved gas barrier and electrical conductivity. Chem. Mat. 22 (11), 3441–3450. doi:10.1021/cm100477v
Kim, K. S., Zhao, Y., Jang, H., Lee, S. Y., Kim, J. M., Kim, K. S., et al. (2009). Large-scale pattern growth of graphene films for stretchable transparent electrodes. Nature 457 (7230), 706–710. doi:10.1038/nature07719
Kim, S., Do, I., and Drzal, L (2010). Thermal stability and dynamic mechanical behavior of exfoliated graphite nanoplatelets‐LLDPE nanocomposites. Polym. Compos. 31 (5), 755–761. doi:10.1002/pc.20781
Kravets, V., Grigorenko, A., Nair, R., Blake, P., Anissimova, S., Novoselov, K., et al. (2010). Spectroscopic ellipsometry of graphene and an exciton-shifted van Hove peak in absorption. Phys. Rev. B 81 (15), 155413. doi:10.1103/physrevb.81.155413
Kumar, P. S., Karuthapandian, S., Umadevi, M., Elangovan, A., and Muthuraj, V. J. (2016). Light induced synthesis of Sr/CdSe nanocomposite for the highly synergistic photodegradation of methylene blue dye solution. Mat. focus 5 (2), 128–136. doi:10.1166/mat.2016.1301
Lafforgue, C., Zadick, A., Dubau, L., Maillard, F., and Chatenet, M. J. (2018). Selected review of the degradation of Pt and Pd‐based carbon‐supported electrocatalysts for alkaline fuel cells: Towards mechanisms of degradation. Fuel Cells (Weinh). 18 (3), 229–238. doi:10.1002/fuce.201700094
Lalwani, G., Henslee, A. M., Farshid, B., Lin, L., Kasper, F. K., Qin, Y-X., et al. (2013). Two-dimensional nanostructure-reinforced biodegradable polymeric nanocomposites for bone tissue engineering. Biomacromolecules 14 (3), 900–909. doi:10.1021/bm301995s
Lam, E., and Luong, J. H. (2014). Carbon materials as catalyst supports and catalysts in the transformation of biomass to fuels and chemicals. ACS Catal. 4 (10), 3393–3410. doi:10.1021/cs5008393
Lamichhane, A., and Ravindra, N. M. (2020). Energy gap-refractive index relations in perovskites. Materials 13 (8), 1917. doi:10.3390/ma13081917
Latha, P., Dhanabackialakshmi, R., Kumar, P. S., Karuthapandian, S., and Technology, P. (2016). Synergistic effects of trouble free and 100% recoverable CeO2/Nylon nanocomposite thin film for the photocatalytic degradation of organic contaminants. Sep. Purif. Technol. 168, 124–133. doi:10.1016/j.seppur.2016.05.038
Lee, C., Wei, X., Kysar, J. W., and Hone, J. (2008). Measurement of the elastic properties and intrinsic strength of monolayer graphene. Science 321 (5887), 385–388. doi:10.1126/science.1157996
Lee, Y-Y., Tu, K-H., Yu, C-C., Li, S-S., Hwang, J-Y., Lin, C-C., et al. (2011). Top laminated graphene electrode in a semitransparent polymer solar cell by simultaneous thermal annealing/releasing method. ACS Nano 5 (8), 6564–6570. doi:10.1021/nn201940j
Lei, Y., Chen, F., Luo, Y., and Zhang, L. (2014). Three-dimensional magnetic graphene oxide foam/Fe3O4 nanocomposite as an efficient absorbent for Cr (VI) removal. J. Mat. Sci. 49 (12), 4236–4245. doi:10.1007/s10853-014-8118-2
Lerner, M. B., Matsunaga, F., Han, G. H., Hong, S. J., Xi, J., Crook, A., et al. (2014). Scalable production of highly sensitive nanosensors based on graphene functionalized with a designed G protein-coupled receptor. Nano Lett. 14 (5), 2709–2714. doi:10.1021/nl5006349
Lesmana, S. O., Febriana, N., Soetaredjo, F. E., Sunarso, J., and Ismadji, S. J. (2009). Studies on potential applications of biomass for the separation of heavy metals from water and wastewater. Biochem. Eng. J. 44 (1), 19–41. doi:10.1016/j.bej.2008.12.009
Lew, A. J., Yu, C-H., Hsu, Y-C., and Buehler, M. J. (2021). Deep learning model to predict fracture mechanisms of graphene. npj 2D Mater. Appl. 5 (1), 1–8. doi:10.1038/s41699-021-00228-x
Li, G., Zhu, R., and Yang, Y. (2012). Polymer solar cells. Nat. Photonics 6 (3), 153–161. doi:10.1038/nphoton.2012.11
Li, Q., and Wang, Q. (2016). Polymer nanocomposites. Springer, 139–163.Polymer nanocomposites for power energy storage
Li, S-S., Tu, K-H., Lin, C-C., Chen, C-W., and Chhowalla, M. J. (2010). Solution-processable graphene oxide as an efficient hole transport layer in polymer solar cells. ACS Nano 4 (6), 3169–3174. doi:10.1021/nn100551j
Li, X., Wang, S., Liu, Y., Jiang, L., Song, B., Li, M., et al. (2017). Adsorption of Cu (II), Pb (II), and Cd (II) ions from acidic aqueous solutions by diethylenetriaminepentaacetic acid-modified magnetic graphene oxide. J. Chem. Eng. Data 62 (1), 407–416. doi:10.1021/acs.jced.6b00746
Li, Y., Zhou, Q., Ren, B., Luo, J., Yuan, J., Ding, X., et al. (2019). Trends and health risks of dissolved heavy metal pollution in global river and lake water from 1970 to 2017. Rev. Environ. Contam. Toxicology,Reviews Environ. Contam. Toxicol. 251, 1–24. doi:10.1007/398_2019_27
Lin, J., Liu, Y., Sui, H., Sagoe-Crentsil, K., Duan, W. J. C., and Research, C. (2022). Microstructure of graphene oxide–silica-reinforced OPC composites: Image-based characterization and nano-identification through deep learning. Cem. Concr. Res. 154, 106737. doi:10.1016/j.cemconres.2022.106737
Lin, S., Nejati, S., Boo, C., Hu, Y., Osuji, C. O., Elimelech, M., et al. (2014). Environ. Sci. Technol. Lett. 1 (11), 443–447. doi:10.1021/ez500267p
Lipomi, D. J., Chong, H., Vosgueritchian, M., Mei, J., and Bao, Z. J. (2012). Toward mechanically robust and intrinsically stretchable organic solar cells: Evolution of photovoltaic properties with tensile strain. Sol. Energy Mat. Sol. Cells 107, 355–365. doi:10.1016/j.solmat.2012.07.013
Liu, B., Wang, Y., Jiang, H-W., and Zou, B-X. (2017). WO3 nanowires on graphene sheets as negative electrode for supercapacitors. J. Nanomater. 2017, 1–9. doi:10.1155/2017/2494109
Liu, H., Liu, Y., and Zhu, D. (2011). Chemical doping of graphene. J. Mat. Chem. 21 (10), 3335–3345. doi:10.1039/c0jm02922j
Liu, J., Durstock, M., Dai, L. J. E., and Science, E. (2014). Graphene oxide derivatives as hole-and electron-extraction layers for high-performance polymer solar cells. Energy Environ. Sci. 7 (4), 1297–1306. doi:10.1039/c3ee42963f
Liu, L., Li, C., Bao, C., Jia, Q., Xiao, P., Liu, X., et al. (2012). Preparation and characterization of chitosan/graphene oxide composites for the adsorption of Au (III) and Pd (II). Talanta 93, 350–357. doi:10.1016/j.talanta.2012.02.051
Liu, Y., Yang, D., Yu, R., Qu, J., Shi, Y., Li, H., et al. (2017). Tetrahedral silver phosphate/graphene oxide hybrids as highly efficient visible light photocatalysts with excellent cyclic stability. J. Phys. Chem. C 121 (45), 25172–25179. doi:10.1021/acs.jpcc.7b07848
Lizama, K., Fletcher, T. D., and Sun, G. J. (2011). Removal processes for arsenic in constructed wetlands. Chemosphere 84 (8), 1032–1043. doi:10.1016/j.chemosphere.2011.04.022
Loh, K. P., Bao, Q., Eda, G., and Chhowalla, M. J. (2010). Graphene oxide as a chemically tunable platform for optical applications. Nat. Chem. 2 (12), 1015–1024. doi:10.1038/nchem.907
Long, Y., Zhang, C., Wang, X., Gao, J., Wang, W., Liu, Y. J., et al. (2011). Oxidation of SO 2 to SO 3 catalyzed by graphene oxide foams. J. Mat. Chem. 21 (36), 13934. doi:10.1039/c1jm12031j
Longxing, H., Yuyao, Z., Wencong, L., Lu, Y., and Hu, H. J. (2019). Easily recyclable photocatalyst Bi 2 WO 6/MOF/PVDF composite film for efficient degradation of aqueous refractory organic pollutants under visible-light irradiation. J. Mat. Sci. 54 (8), 6238–6257. doi:10.1007/s10853-018-03302-w
Lu, X., Peng, Y., Ge, L., Lin, R., Zhu, Z., Liu, S. J., et al. (2016). Amphiphobic PVDF composite membranes for anti-fouling direct contact membrane distillation. J. Memb. Sci. 505, 61–69. doi:10.1016/j.memsci.2015.12.042
Luo, X., and Yang, J. (2017). Water pollution detection based on hypothesis testing in sensor networks. J. Sensors. 2017, 1–8. doi:10.1155/2017/3829894
Ma, T., Jiang, K., Chen, S., Hu, H., Lin, H., Li, Z., et al. (2015). Efficient low‐bandgap polymer solar cells with high open‐circuit voltage and good stability. Adv. Energy Mat. 5 (20), 1501282. doi:10.1002/aenm.201501282
Ma, Y., Bai, D., Hu, X., Ren, N., Gao, W., Chen, S., et al. (2018). Robust and antibacterial polymer/mechanically exfoliated graphene nanocomposite fibers for biomedical applications. ACS Appl. Mat. Interfaces 10 (3), 3002–3010. doi:10.1021/acsami.7b17835
Mahmood, N., Zhang, C., Yin, H., and Hou, Y. J. (2014). Graphene-based nanocomposites for energy storage and conversion in lithium batteries, supercapacitors and fuel cells. J. Mat. Chem. A 2 (1), 15–32. doi:10.1039/c3ta13033a
Maleki, A., Pajootan, E., and Hayati, B. J. (2015). Ethyl acrylate grafted chitosan for heavy metal removal from wastewater: Equilibrium, kinetic and thermodynamic studies. J. Taiwan Inst. Chem. Eng. 51, 127–134. doi:10.1016/j.jtice.2015.01.004
Mansfield, J., Genin, S., Magori, S., Citovsky, V., Sriariyanum, M., Ronald, P., et al. (2012). Mol. Plant Pathol. 13 (6), 614–629. doi:10.1111/j.1364-3703.2012.00804.x
Mara, N., Bhattacharyya, D., Dickerson, P., Hoagland, R., and Misra, A. J. (2008). Deformability of ultrahigh strength 5 nm Cu∕ Nb nanolayered composites. Appl. Phys. Lett. 92 (23), 231901. doi:10.1063/1.2938921
Marforio, T. D., Bottoni, A., Zerbetto, F., and Calvaresi, M. (2019). CNT‐Catalyzed oxidative dehydrogenation of ethylbenzene to styrene: DFT calculations disclose the pathways. ChemNanoMat 5 (4), 499–505. doi:10.1002/cnma.201800558
Mattevi, C., Colléaux, F., Kim, H., Lin, Y-H., Park, K. T., Chhowalla, M., et al. (2012). Nanotechnology 23 (34), 344017. doi:10.1088/0957-4484/23/34/344017
Mestl, G., Maksimova, N. I., Keller, N., Roddatis, V. V., and Schlögl, R. (2001). Carbon nanofilaments in heterogeneous catalysis: An industrial application for new carbon materials? Angew. Chem. Int. Ed. 40 (11), 2066–2068. doi:10.1002/1521-3773(20010601)40:11<2066::aid-anie2066>3.0.co;2-i
Mittal, G., Dhand, V., Rhee, K. Y., Park, S-J., Lee, W. R., and Chemistry, E. (2015). A review on carbon nanotubes and graphene as fillers in reinforced polymer nanocomposites. J. Ind. Eng. Chem. 21, 11–25. doi:10.1016/j.jiec.2014.03.022
Mohan, A. N., and Panicker, S (2019). Facile synthesis of graphene-tin oxide nanocomposite derived from agricultural waste for enhanced antibacterial activity against Pseudomonas aeruginosa. Sci. Rep. 9 (1), 4170. doi:10.1038/s41598-019-40916-9
Mohan, H., Ramalingam, V., Karthi, N., Malathidevi, S., Shin, T., Venkatachalam, J., et al. (2021). Enhanced visible light-driven photocatalytic activity of reduced graphene oxide/cadmium sulfide composite: Methylparaben degradation mechanism and toxicity. Chemosphere 264, 128481. doi:10.1016/j.chemosphere.2020.128481
Monsef, R., Ghiyasiyan-Arani, M., and Salavati-Niasari, M. J. (2021). Design of magnetically recyclable ternary Fe2O3/EuVO4/g-C3N4 nanocomposites for photocatalytic and electrochemical hydrogen storage. ACS Appl. Energy Mat. 4 (1), 680–695. doi:10.1021/acsaem.0c02557
Monsef, R., Ghiyasiyan-Arani, M., and Salavati-Niasari, M. J. (2018). Application of ultrasound-aided method for the synthesis of NdVO4 nano-photocatalyst and investigation of eliminate dye in contaminant water. Ultrason. Sonochem. 42, 201–211. doi:10.1016/j.ultsonch.2017.11.025
Moore, B. A., Rougier, E., O’Malley, D., Srinivasan, G., Hunter, A., Viswanathan, H. J., et al. (2018). Predictive modeling of dynamic fracture growth in brittle materials with machine learning. Comput. Mat. Sci. 148, 46–53. doi:10.1016/j.commatsci.2018.01.056
Moreno-Fernandez, G., Ibañez, J., Rojo, J. M., and Kunowsky, M. (2017). Activated carbon fiber monoliths as supercapacitor electrodes. Adv. Mater. Sci. Eng. 2017, 1–8. doi:10.1155/2017/3625414
Motahari, F., Mozdianfard, M. R., Salavati-Niasari, M. J., and Protection, E. (2015). Synthesis and adsorption studies of NiO nanoparticles in the presence of H2acacen ligand, for removing Rhodamine B in wastewater treatment. Process Saf. Environ. Prot. 93, 282–292. doi:10.1016/j.psep.2014.06.006
Muthumeenal, A., Saraswathi, M. S. A., Rana, D., and Nagendran, A. J. (2017). Fabrication and electrochemical properties of highly selective SPES/GO composite membranes for direct methanol fuel cells. J. Environ. Chem. Eng. 5 (4), 3828–3833. doi:10.1016/j.jece.2017.07.036
Nagashio, K., Nishimura, T., Kita, K., and Toriumi, A. (2009). “Metal/graphene contact as a performance killer of ultra-high mobility graphene analysis of intrinsic mobility and contact resistance,” in 2009 IEEE International Electron Devices Meeting (IEDM). (IEEE).
Nakada, K., and Ishii, A. (2011). DFT calculation for adatom adsorption on graphene. Graphene Simul.
Nasrollahzadeh, M., Jaleh, B., and Jabbari, A. J. (2014). Synthesis, characterization and catalytic activity of graphene oxide/ZnO nanocomposites. RSC Adv. 4 (69), 36713. doi:10.1039/c4ra05833j
Nguyen, B. H., and Nguyen, V. H. (2016). Adv. Nat. Sci. Nanosci. Nanotechnol. 7 (2), 023002. doi:10.1088/2043-6262/7/2/023002
Niu, Y., Qu, R., Sun, C., Wang, C., Chen, H., Ji, C., et al. (2013). Adsorption of Pb (II) from aqueous solution by silica-gel supported hyperbranched polyamidoamine dendrimers. J. Hazard. Mat. 244, 276–286. doi:10.1016/j.jhazmat.2012.11.042
Novoselov, K. S., Geim, A. K., Morozov, S. V., Jiang, D., Katsnelson, M. I., Grigorieva, I., et al. (2005). Two-dimensional gas of massless Dirac fermions in graphene. Nature 438 (7065), 197–200. doi:10.1038/nature04233
Novoselov, K. S., Geim, A. K., Morozov, S. V., Jiang, D-E., Zhang, Y., Dubonos, S. V., et al. (2004). Electric field effect in atomically thin carbon films. Science 306 (5696), 666–669. doi:10.1126/science.1102896
Nuvoli, D., Valentini, L., Alzari, V., Scognamillo, S., Bon, S. B., Piccinini, M., et al. (2011). High concentration few-layer graphene sheets obtained by liquid phase exfoliation of graphite in ionic liquid. J. Mat. Chem. 21 (10), 3428–3431. doi:10.1039/c0jm02461a
Palaparthy, V. S., Baghini, M. S., and Singh, D. N. (2013). Review of polymer-based sensors for agriculture-related applications. Emerg. Mater. Res. 2 (4), 166–180. doi:10.1680/emr.13.00010
Pallecchi, E., Lafont, F., Cavaliere, V., Schopfer, F., Mailly, D., Poirier, W., et al. (2014). High electron mobility in epitaxial graphene on 4H-SiC (0001) via post-growth annealing under hydrogen. Sci. Rep. 4 (1), 4558. doi:10.1038/srep04558
Paredes, J., Villar-Rodil, S., Fernández-Merino, M., Guardia, L., Martínez-Alonso, A., Tascon, J., et al. (2011). Environmentally friendly approaches toward the mass production of processable graphene from graphite oxide. J. Mat. Chem. 21 (2), 298–306. doi:10.1039/c0jm01717e
Park, H., Chang, S., Zhou, X., Kong, J., Palacios, Ts, Gradečak, S., et al. (2014). Flexible graphene electrode-based organic photovoltaics with record-high efficiency. Nano Lett. 14 (9), 5148–5154. doi:10.1021/nl501981f
Park, H-Y., Cha, I. Y., Chung, Y-H., Cho, M. K., Yoo, S. J., Kim, H-J., et al. (2015). Determination of phosphoric acid coverage on Pt/C for high temperature-polymer electrolyte membrane fuel cell (HT-PEMFC) using in-situ X-ray absorption spectroscopy. ECS Meet. Abstr. MA2015-01 (32), 1855. doi:10.1149/ma2015-01/32/1855
Peet, J., Kim, J. Y., Coates, N. E., Ma, W. L., Moses, D., Heeger, A. J., et al. (2007). Efficiency enhancement in low-bandgap polymer solar cells by processing with alkane dithiols. Nat. Mat. 6 (7), 497–500. doi:10.1038/nmat1928
Peng, C., Hu, W., Zhou, Y., Fan, C., and Huang, Q. J. S. (2010). Intracellular imaging with a graphene‐based fluorescent probe. Small 6 (15), 1686–1692. doi:10.1002/smll.201000560
Pereira, M. F. R., Figueiredo, J. L., Órfão, J. J., Serp, P., Kalck, P., Kihn, Y., et al. (2004). Catalytic activity of carbon nanotubes in the oxidative dehydrogenation of ethylbenzene. Carbon N. Y. 42 (14), 2807–2813. doi:10.1016/j.carbon.2004.06.025
Perreault, F., De Faria, A. F., and Elimelech, M. J. (2015). Environmental applications of graphene-based nanomaterials. Chem. Soc. Rev. 44 (16), 5861–5896. doi:10.1039/c5cs00021a
Pierson, K., Rahman, A., and Spear, A. D. Predicting microstructure-sensitive fatigue-crack path in 3d using a machine learning framework. JOM 2019;71(8):2680–2694. doi:10.1007/s11837-019-03572-y
Potts, J. R., Dreyer, D. R., Bielawski, C. W., and Ruoff, R. S. (2011). Graphene-based polymer nanocomposites. Polymer 52 (1), 5–25. doi:10.1016/j.polymer.2010.11.042
Pourbeyram, S., Alizadeh, S., and Gholizadeh, S. J. (2016). Simultaneous removal of arsenate and arsenite from aqueous solutions by graphene oxide-zirconium (GO-Zr) nanocomposite. J. Environ. Chem. Eng. 4 (4), 4366–4373. doi:10.1016/j.jece.2016.10.003
Prasai, D., Tuberquia, J. C., Harl, R. R., Jennings, G. K., and Bolotin, K. I. (2012). Graphene: Corrosion-inhibiting coating. ACS Nano 6 (2), 1102–1108. doi:10.1021/nn203507y
Qi, Z., Zhang, N., Liu, Y., and Chen, W. (2019). Prediction of mechanical properties of carbon fiber based on cross-scale FEM and machine learning. Compos. Struct. 212, 199–206. doi:10.1016/j.compstruct.2019.01.042
Qian, H., Wang, J., and Yan, L., (2020). Synthesis of lignin-poly (N-methylaniline)-reduced graphene oxide hydrogel for organic dye and lead ions removal. J. Bioresour. Bioprod. 5 (3), 204–210. doi:10.1016/j.jobab.2020.07.006
Ramanathan, T., Stankovich, S., Dikin, D., Liu, H., Shen, H., Nguyen, S., et al. (2007). Graphitic nanofillers in PMMA nanocomposites—An investigation of particle size and dispersion and their influence on nanocomposite properties. J. Polym. Sci. B. Polym. Phys. 45 (15), 2097–2112. doi:10.1002/polb.21187
Ramaswamy, N., and Mukerjee, S. J. (2012). Fundamental mechanistic understanding of electrocatalysis of oxygen reduction on Pt and non-Pt surfaces: Acid versus alkaline media. Adv. Phys. Chem. 2012, 1–17. doi:10.1155/2012/491604
Rourke, J. P., Pandey, P. A., Moore, J. J., Bates, M., Kinloch, I. A., Young, R. J., et al. (2011). The real graphene oxide revealed: Stripping the oxidative debris from the graphene‐like sheets. Angew. Chem. Int. Ed. 50 (14), 3173–3177. doi:10.1002/anie.201007520
Ruan, G., Sun, Z., Peng, Z., and Tour, J. M. (2011). Growth of graphene from food, insects, and waste. ACS Nano 5 (9), 7601–7607. doi:10.1021/nn202625c
Ruan, W., Hu, J., Qi, J., Hou, Y., Cao, R., Wei, X., et al. (2018). Removal of crystal violet by using reduced-graphene-oxide-supported bimetallic Fe/Ni nanoparticles (rGO/Fe/Ni): Application of artificial intelligence modeling for the optimization process. Materials 11 (5), 865. doi:10.3390/ma11050865
Saljnikov, E., Mrvić, V., Čakmak, D., Jaramaz, D., Perović, V., Antić-Mladenović, S., et al. (2019). Pollution indices and sources appointment of heavy metal pollution of agricultural soils near the thermal power plant. Environ. Geochem. Health 41 (5), 2265–2279. doi:10.1007/s10653-019-00281-y
Savagatrup, S., Printz, A. D., O'Connor, T. F., Zaretski, A. V., Rodriquez, D., Sawyer, E. J., et al. (2015). Mechanical degradation and stability of organic solar cells: Molecular and microstructural determinants. Energy Environ. Sci. 8 (1), 55–80. doi:10.1039/c4ee02657h
Sawangphruk, M., Srimuk, P., Chiochan, P., Sangsri, T., and Siwayaprahm, P. J. C. (2012). Synthesis and antifungal activity of reduced graphene oxide nanosheets. Carbon N. Y. 50 (14), 5156–5161. doi:10.1016/j.carbon.2012.06.056
Schwarzer, M., Rogan, B., Ruan, Y., Song, Z., Lee, D. Y., Percus, A. G., et al. (2019). Learning to fail: Predicting fracture evolution in brittle material models using recurrent graph convolutional neural networks. Comput. Mat. Sci. 162, 322–332. doi:10.1016/j.commatsci.2019.02.046
Seger, B., and Kamat, P. V. J. T. (2009). Electrocatalytically active graphene-platinum nanocomposites Role of 2-D carbon support in PEM fuel cells. J. Phys. Chem. C 113 (19), 7990–7995. doi:10.1021/jp900360k
Seo, D. H., Pineda, S., Woo, Y. C., Xie, M., Murdock, A. T., Ang, E. Y., et al. (2018). Anti-fouling graphene-based membranes for effective water desalination. Nat. Commun. 9 (1), 683. doi:10.1038/s41467-018-02871-3
Shi, X., Ruan, W., Hu, J., Fan, M., Cao, R., Wei, X. J. N., et al. (2017). Optimizing the removal of rhodamine B in aqueous solutions by reduced graphene oxide-supported nanoscale zerovalent iron (nZVI/rGO) using an artificial neural network-genetic algorithm (ANN-GA). Nanomater. (Basel). 7 (6), 134. doi:10.3390/nano7060134
Singh, V., Joung, D., Zhai, L., Das, S., Khondaker, S. I., Seal, S. J., et al. (2011). Graphene based materials: Past, present and future. Prog. Mat. Sci. 56 (8), 1178–1271. doi:10.1016/j.pmatsci.2011.03.003
Song, S., Wu, K., Wu, H., Guo, J., and Zhang, L. J. (2020). Synthesis of Z-scheme multi-shelled ZnO/AgVO3 spheres as photocatalysts for the degradation of ciprofloxacin and reduction of chromium (VI). J. Mat. Sci. 55 (12), 4987–5007. doi:10.1007/s10853-019-04316-8
Song, W., Ji, X., Deng, W., Chen, Q., Shen, C., Banks, C. E., et al. (2013). Graphene ultracapacitors: Structural impacts. Phys. Chem. Chem. Phys. 15 (13), 4799. doi:10.1039/c3cp50516b
Srivastava, A., Galande, C., Ci, L., Song, L., Rai, C., Jariwala, D., et al. (2010). Novel liquid precursor-based facile synthesis of large-area continuous, single, and few-layer graphene films. Chem. Mat. 22 (11), 3457–3461. doi:10.1021/cm101027c
Stoller, M. D., Park, S., Zhu, Y., An, J., and Ruoff, R. S. (2008). Graphene-based ultracapacitors. Nano Lett. 8 (10), 3498–3502. doi:10.1021/nl802558y
Su, D. S., Maksimova, N., Delgado, J. J., Keller, N., Mestl, G., Ledoux, M. J., et al. (2005). Nanocarbons in selective oxidative dehydrogenation reaction. Catal. Today 102, 110–114. doi:10.1016/j.cattod.2005.02.012
Sun, P., Wang, K., and Zhu, H. J. (2016). Recent developments in graphene‐based membranes: Structure, mass‐transport mechanism and potential applications. Adv. Mat. 28 (12), 2287–2310. doi:10.1002/adma.201502595
Sur, U. K. (2012). Graphene: A rising star on the horizon of materials science. Int. J. Electrochem. 2012, 1–12. doi:10.1155/2012/237689
Surwade, S. P., Smirnov, S. N., Vlassiouk, I. V., Unocic, R. R., Veith, G. M., Dai, S., et al. (2015). Water desalination using nanoporous single-layer graphene. Nat. Nanotechnol. 10 (5), 459–464. doi:10.1038/nnano.2015.37
Tan, S. M., and Pumera, M. J. C. A. E. J. (2017). Electrosynthesis of bifunctional WS3−x/reduced graphene oxide hybrid for hydrogen evolution reaction and oxygen reduction reaction electrocatalysis. Chem. Eur. J. 23 (35), 8510–8519. doi:10.1002/chem.201701722
Tijing, L. D., Woo, Y. C., Choi, J-S., Lee, S., Kim, S-H., Shon, H. K., et al. (2015). Fouling and its control in membrane distillation—a review. J. Memb. Sci. 475, 215–244. doi:10.1016/j.memsci.2014.09.042
Tiwari, J. N., Tiwari, R. N., and Kim, K. S. (2012). Zero-dimensional, one-dimensional, two-dimensional and three-dimensional nanostructured materials for advanced electrochemical energy devices. Prog. Mat. Sci. 57 (4), 724–803. doi:10.1016/j.pmatsci.2011.08.003
Uhl, F. M., Yao, Q., Nakajima, H., Manias, E., and Wilkie, C. A. (2005). Polym. Degrad. Stab. 89 (1), 70–84. doi:10.1016/j.polymdegradstab.2005.01.004
Unalan, I. U., Cerri, G., Marcuzzo, E., Cozzolino, C. A., and Farris, S. (2014). Nanocomposite films and coatings using inorganic nanobuilding blocks (NBB): Current applications and future opportunities in the food packaging sector. RSC Adv. 4 (56), 29393–29428. doi:10.1039/c4ra01778a
van Hal, P. A., Wienk, M. M., Kroon, J. M., Verhees, W. J., Slooff, L. H., van Gennip, W. J., et al. (2003). Photoinduced electron transfer and photovoltaic response of a MDMO‐PPV: TiO2 bulk‐heterojunction. Adv. Mat. 15 (2), 118–121. doi:10.1002/adma.200390022
Vazquez, A., Cyras, V. P., Alvarez, V. A., and Moran, J. I. (2012). Starch/clay nano-biocomposites. Environ. Silic. nano-biocomposites, Green Energy Technol., 287–321. Springer. doi:10.1007/978-1-4471-4108-2_11
Vinoth, R., Babu, S. G., Bahnemann, D., and Neppolian, B. (2015). Nitrogen doped reduced graphene oxide hybrid metal free catalyst for effective reduction of 4-nitrophenol. Sci. Adv. Mat. 7 (7), 1443–1449. doi:10.1166/sam.2015.2181
Vinoth, R., Babu, S. G., Bharti, V., Gupta, V., Navaneethan, M., Bhat, S. V., et al. (2017). Ruthenium based metallopolymer grafted reduced graphene oxide as a new hybrid solar light harvester in polymer solar cells. Sci. Rep. 7 (1), 43133. doi:10.1038/srep43133
Vuong Hoan, N. T., Anh Thu, N. T., Duc, H. V., Cuong, N. D., Quang Khieu, D., and Vo, V. (2016). Fe3O4/reduced graphene oxide nanocomposite: Synthesis and its application for toxic metal ion removal. J. Chem., 1–10. doi:10.1155/2016/2418172
Wang, C., Liu, W., Wei, S., Su, D., and Qi, W. J. C. (2019). Oxidative dehydrogenation on nanocarbon: Revealing the reaction mechanism via in situ experimental strategies. ChemCatChem 11 (1), 397–400. doi:10.1002/cctc.201801547
Wang, L., Liu, L., Chen, J., Mohsin, A., Yum, J. H., Hudnall, T. W., et al. (2020). Synthesis of honeycomb‐structured beryllium oxide via graphene liquid cells. Angew. Chem. Int. Ed. 59 (36), 15734–15740. doi:10.1002/anie.202007244
Wang, L., Sofer, Z., and Pumera, M. J. N. (2019). Catalytic hydrogen evolution reaction on “metal-free” graphene: Key role of metallic impurities. Nanoscale 11 (23), 11083–11085. doi:10.1039/c9nr03557e
Wang, X., Liu, X., Chen, J., Han, H., and Yuan, Z. J. C. (2014). Evaluation and mechanism of antifungal effects of carbon nanomaterials in controlling plant fungal pathogen. Carbon N. Y. 68, 798–806. doi:10.1016/j.carbon.2013.11.072
Wang, Y., Cao, Z., and Farimani, A. B. (2021). Efficient water desalination with graphene nanopores obtained using artificial intelligence. npj 2D Mater. Appl. 5 (1), 1–9. doi:10.1038/s41699-021-00246-9
Wang, Y., Shao, Y., Matson, D. W., Li, J., and Lin, Y. (2010). Nitrogen-doped graphene and its application in electrochemical biosensing. ACS Nano 4 (4), 1790–1798. doi:10.1021/nn100315s
Wang, Z., and Zhi, C. (2016). Thermally conductive electrically insulating polymer nanocomposites. Polym. Nanocomposites, 281–321. doi:10.1007/978-3-319-28238-1_11
Woo, Y. C., Kim, Y., Shim, W-G., Tijing, L. D., Yao, M., Nghiem, L. D., et al. (2016). Graphene/PVDF flat-sheet membrane for the treatment of RO brine from coal seam gas produced water by air gap membrane distillation. J. Memb. Sci. 513, 74–84. doi:10.1016/j.memsci.2016.04.014
Wu, C. W., and Hung, W. Z. J. (2015). Real national income average growth rate: A novel economic growth and social fair evaluation index. Econ. Res. Int. 2015, 1–7. doi:10.1155/2015/678927
Wu, X., Hu, J., Qi, J., Hou, Y., and Wei, X. J. (2020). Graphene-supported ordered mesoporous composites used for environmental remediation: A review. Sep. Purif. Technol. 239, 116511. doi:10.1016/j.seppur.2020.116511
Wu, Y., Mu, H., Cao, X., and He, X. (2020). Polymer-supported graphene–TiO2 doped with nonmetallic elements with enhanced photocatalytic reaction under visible light. J. Mat. Sci. 55 (4), 1577–1591. doi:10.1007/s10853-019-04100-8
Xiang, Q., Yu, J., and Jaroniec, M. J. (2012). Chem. Soc. Rev. 41 (2), 782–796. doi:10.1039/c1cs15172j
Xiang, Y., Xin, L., Hu, J., Li, C., Qi, J., Hou, Y., et al. (2021). Advances in the applications of graphene-based nanocomposites in clean energy materials. Cryst. (Basel). 11 (1), 47. doi:10.3390/cryst11010047
Xiao, Z., Duan, T., Chen, H., Sun, K., and Lu, S. (2018). The role of hydrogen bonding in bulk-heterojunction (BHJ) solar cells: A review. Sol. Energy Mat. Sol. Cells 182, 1–13. doi:10.1016/j.solmat.2018.03.013
Xin, C., Jinsong, Z., Donghua, L., and Kangning, Z. Development and future prospect of the hydrogen fuel cell vehicle at home and abroad. 2019;4:1671–7988.
Yang, K., Feng, L., Shi, X., and Liu, Z. J. (2013). Chem. Soc. Rev. 42 (2), 530–547. doi:10.1039/c2cs35342c
Yang, S-T., Chang, Y., Wang, H., Liu, G., Chen, S., Wang, Y., et al. (2010). Folding/aggregation of graphene oxide and its application in Cu2+ removal. J. Colloid Interface Sci. 351 (1), 122–127. doi:10.1016/j.jcis.2010.07.042
Yap, K., and Liu, W. (2020).Exfoliation of charcoal by electrochemical method to synthesize few layers graphene sheets, In” IOP Conference Series: Materials Science and Engineering”. Ho Chi Minh City, Vietnam: IOP Publishing.
Yasmin, A., and Daniel, I. M. (2004). Mechanical and thermal properties of graphite platelet/epoxy composites. Polymer 45 (24), 8211–8219. doi:10.1016/j.polymer.2004.09.054
Yi, G., Xing, B., Zeng, H., Wang, X., Zhang, C., Cao, J., et al. (2017). One-step synthesis of hierarchical micro-mesoporous SiO2/reduced graphene oxide nanocomposites for adsorption of aqueous Cr (VI). J. Nanomater. 2017, 1–10. doi:10.1155/2017/6286549
Yi, M., and Shen, Z. (2015). A review on mechanical exfoliation for the scalable production of graphene. J. Mat. Chem. A Mat. 3 (22), 11700–11715. doi:10.1039/c5ta00252d
Young, R. J., Kinloch, I. A., Gong, L., and Novoselov, K. S. (2012). The mechanics of graphene nanocomposites: A review. Compos. Sci. Technol. 72 (12), 1459–1476. doi:10.1016/j.compscitech.2012.05.005
Yuan, J-M., Fan, Z-F., Chen, X-H., Chen, X-H., Wu, Z-J., He, L-P. J. P., et al. (2009). Preparation of polystyrene–multiwalled carbon nanotube composites with individual-dispersed nanotubes and strong interfacial adhesion. Polymer 50 (14), 3285–3291. doi:10.1016/j.polymer.2009.04.065
Zeng, J., Mu, Y., Ji, X., Lin, Z., Lin, Y., Ma, Y., et al. (2019). N, O-codoped 3D graphene fibers with densely arranged sharp edges as highly efficient electrocatalyst for oxygen reduction reaction. J. Mat. Sci. 54 (23), 14495–14503. doi:10.1007/s10853-019-03743-x
Zeng, Z., Yi, L., He, J., Hu, Q., Liao, Y., Wang, Y., et al. (2020). Hierarchically porous carbon with pentagon defects as highly efficient catalyst for oxygen reduction and oxygen evolution reactions. J. Mat. Sci. 55 (11), 4780–4791. doi:10.1007/s10853-019-04327-5
Zhang, H-B., Zheng, W-G., Yan, Q., Jiang, Z-G., and Yu, Z-Z. (2012). The effect of surface chemistry of graphene on rheological and electrical properties of polymethylmethacrylate composites. Carbon N. Y. 50 (14), 5117–5125. doi:10.1016/j.carbon.2012.06.052
Zhang, J., Wang, X., Yu, C., Li, Q., Li, Z., Li, C., et al. (2017). A facile method to prepare flexible boron nitride/poly (vinyl alcohol) composites with enhanced thermal conductivity. Compos. Sci. Technol. 149, 41–47. doi:10.1016/j.compscitech.2017.06.008
Zhang, J., Zhou, Y., Zheng, G., Huang, Q., Zheng, X., Liu, P., et al. (2016). Novel assembly and electrochemical properties of anatase TiO2-graphene aerogel 3D hybrids as lithium-ion battery anodes. Chem. Phys. Lett. 662, 214–220. doi:10.1016/j.cplett.2016.09.044
Zhang, K., Dwivedi, V., Chi, C., and Wu, J. (2010). Graphene oxide/ferric hydroxide composites for efficient arsenate removal from drinking water. J. Hazard. Mat. 182 (1-3), 162–168. doi:10.1016/j.jhazmat.2010.06.010
Zhang, N., Zhang, Y., and Xu, Y-J. (2012). Recent progress on graphene-based photocatalysts: Current status and future perspectives. Nanoscale 4 (19), 5792. doi:10.1039/c2nr31480k
Zhang, X., Wu, J., Liu, H., Wang, J., Zhao, X., Xie, Z. J., et al. (2017). Efficient flexible polymer solar cells based on solution-processed reduced graphene oxide–Assisted silver nanowire transparent electrode. Org. Electron. 50, 255–263. doi:10.1016/j.orgel.2017.07.055
Zhao, W., Cao, H., Wang, S., Xie, L., and Huang, W. J. (2019). Solution-processable GO/RGO: Preparation, functionalization, self-assembly and applications in smart information devices. Chin. Sci. Bull. 64 (26), 2689–2702. doi:10.1360/tb-2019-0060
Zheng, W., Lu, X., and Wong, S. C. (2004). Electrical and mechanical properties of expanded graphite‐reinforced high‐density polyethylene. J. Appl. Polym. Sci. 91 (5), 2781–2788. doi:10.1002/app.13460
Zheng, Y., Yang, Y., Zhang, Y., Zou, W., Luo, Y., Dong, L., et al. (2019). Facile one-step synthesis of graphitic carbon nitride-modified biochar for the removal of reactive red 120 through adsorption and photocatalytic degradation. Biochar 1 (1), 89–96. doi:10.1007/s42773-019-00007-4
Zhong, W-S., Ren, T., and Zhao, L-J. (2016). Determination of Pb (Lead), Cd (Cadmium), Cr (Chromium), Cu (Copper), and Ni (Nickel) in Chinese tea with high-resolution continuum source graphite furnace atomic absorption spectrometry. J. Food Drug Anal. 24 (1), 46–55. doi:10.1016/j.jfda.2015.04.010
Zhou, L., Deng, H., Wan, J., Shi, J., and Su, T. J. (2013). A solvothermal method to produce RGO-Fe3O4 hybrid composite for fast chromium removal from aqueous solution. Appl. Surf. Sci. 283, 1024–1031. doi:10.1016/j.apsusc.2013.07.063
Zhou, M., Tian, T., Li, X., Sun, X., Zhang, J., Cui, P., et al. Production of graphene by liquid-phase exfoliation of intercalated graphite. 2014;9(2):810–820.
Zhou, S., and Bongiorno, A. (2014). Density functional theory modeling of multilayer “epitaxial” graphene oxide. Acc. Chem. Res. 47 (11), 3331–3339. doi:10.1021/ar400288h
Zhu, H., He, R., Mao, J., Zhu, Q., Li, C., Sun, J., et al. (2018). Discovery of ZrCoBi based half Heuslers with high thermoelectric conversion efficiency. Nat. Commun. 9 (1), 2497. doi:10.1038/s41467-018-04958-3
Zhu, L., Zhao, X., Li, Y., Yu, X., Li, C., Zhang, Q. J. M. C., et al. (2013). High-quality production of graphene by liquid-phase exfoliation of expanded graphite. Mat. Chem. Phys. 137 (3), 984–990. doi:10.1016/j.matchemphys.2012.11.012
Zhu, Y., Murali, S., Cai, W., Li, X., Suk, J. W., Potts, J. R., et al. (2010). Graphene and graphene oxide: Synthesis, properties, and applications. Adv. Mat. 22 (35), 3906–3924. doi:10.1002/adma.201001068
Keywords: graphene, nanocomposites, AI modeling, environmental remediation, energy, agricultural applications
Citation: Tariq W, Ali F, Arslan C, Nasir A, Gillani SH and Rehman A (2022) Synthesis and applications of graphene and graphene-based nanocomposites: Conventional to artificial intelligence approaches. Front. Environ. Chem. 3:890408. doi: 10.3389/fenvc.2022.890408
Received: 05 March 2022; Accepted: 04 July 2022;
Published: 31 August 2022.
Edited by:
David Lokhat, University of KwaZulu-Natal, South AfricaReviewed by:
Masoud Salavati-Niasari, University of Kashan, IranMahmoud Nasrollahzadeh, University of Qom, Iran
Ananthakumar Ramadoss, Central Institute of Petrochemical Engineering and Technology, India
Copyright © 2022 Tariq, Ali, Arslan, Nasir, Gillani and Rehman. This is an open-access article distributed under the terms of the Creative Commons Attribution License (CC BY). The use, distribution or reproduction in other forums is permitted, provided the original author(s) and the copyright owner(s) are credited and that the original publication in this journal is cited, in accordance with accepted academic practice. No use, distribution or reproduction is permitted which does not comply with these terms.
*Correspondence: Waheed Tariq, V2FoZWVkdGFyaXE0NzI4QGdtYWlsLmNvbQ==