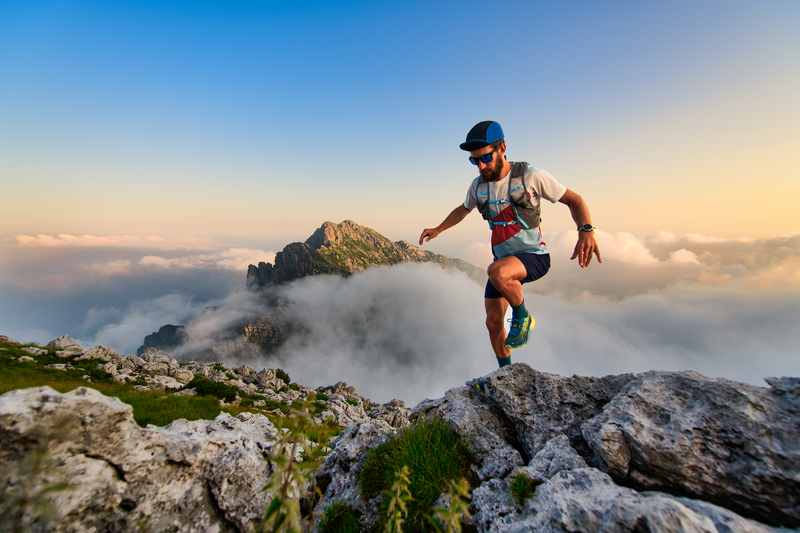
95% of researchers rate our articles as excellent or good
Learn more about the work of our research integrity team to safeguard the quality of each article we publish.
Find out more
ORIGINAL RESEARCH article
Front. Environ. Chem. , 14 February 2022
Sec. Inorganic Pollutants
Volume 3 - 2022 | https://doi.org/10.3389/fenvc.2022.767202
This article is part of the Research Topic Advances in Metals and Trace Elements Isotopes Measurements, Experiments and Application in Environmental Sciences View all 6 articles
Seafood has a great ecological and nutritional value for human and wildlife communities. However, accumulation of mercury (Hg) in fish is a concern to animal and human health. There is a crucial need to understand Hg speciation in marine organisms through controlled feeding experiments. This study represents a first assessment of the biological processes that may influence Hg bioaccumulation and dynamics in a marine predatory fish. We conducted a feeding experiment to investigate the dynamics of MeHg and iHg, as well as Hg isotopes in the liver and muscles of captive juvenile seabass (Dicentrarchus labrax). Three groups of juvenile seabass were fed in captivity during 3 weeks of acclimatization and 6 weeks of experiment. Each group was fed with pellets containing environmentally relevant MeHg concentrations (Control, 200 and 500 ng g−1 dw). We monitored the evolution of MeHg and iHg concentrations as well as Hg isotopic values in liver and muscle. We determined Hg dynamics with respect to the contamination level in the fish diet. Muscle δ202Hg and Δ199Hg turnover rates ranged between 33 and 14 days (Low diet) to 5 and 9 days (Mod diet). Liver δ202Hg and Δ199Hg turnover rates ranged between 3 and 7 days (Low diet) to 3 and 2 days (Mod diet), respectively. Hg species concentrations and δ202Hg varied over time between diet groups and tissues, showing the occurrence of internal mass-dependent fractionation (MDF). No significant intra-tissue and temporal Hg mass-independent fractionation (MIF) was observed. The results of our experiment are strongly in favor of the existence of MeHg demethylation in a coastal predatory fish exposed to low to moderate concentrations of environmental Hg. The decrease over time of δ202Hg in muscle of seabass from the most contaminated diet was accompanied by a temporal increase in iHg, pointing to possible Hg detoxification processes occurring in this tissue when dietary Hg exposure is high. The absence of Hg MDF and different turnover between muscle and liver in seabass exposed to 500 ng Hg g−1 confirmed that Hg speciation and bioaccumulation in juvenile fish are controlled by Hg levels and speciation in their diet.
Mercury (Hg) in the marine environment is of public health concern due to its persistence, toxicity, and bioaccumulation (UNEP, 2002; UNEP, 2013). Studies of sources and fate of Hg in marine and coastal ecosystems have grown extensively in the past decades, but there are still major gaps in the understanding of the cycle of Hg (Selin, 2009). Nowadays, in situ studies using the stable isotopic signature of Hg measured in environment and in biota are expanding (Bergquist and Blum, 2009). The analysis of stable isotopes of Hg is a promising technique that has already improved our understanding of the sources and biogeochemistry of Hg in ecosystems and particularly in the aquatic environment (Tsui et al., 2020). Several studies of stable isotopes of Hg in fish have successfully linked Hg sources to food webs (Gantner et al., 2009; Perrot et al., 2010; Senn et al., 2010; Tsui et al., 2012; Pinzone et al., 2021). However, the lack of controlled experiments limits the interpretation of Hg isotope ratios in fish tissues. Previous feeding experiments demonstrated that processes occurring in fish such as trophic transfer and in vivo demethylation of MeHg induce mass-dependent (δ202Hg) and mass-independent (Δ199Hg) fractionation (MDF and MIF, respectively), with contrasting results (Gonzalez et al., 2005; Perrot et al., 2012; Wang et al., 2013; Feng et al., 2015; Wang et al., 2017; Pinzone et al., 2021). Two studies concluded to no fractionation (Kwon et al., 2012; Kwon et al., 2013), and one long-term study on Pacific bluefin tuna (Thunnus orientalis) found a negative δ202Hg shift between fish and diet (Kwon et al., 2016). Yet, it has to be underlined that many experimental studies exposed fish to extremely elevated Hg levels (up to 10,000 ng g−1 dw) that might overcome or compromise the demethylating capacities of liver (Kwon et al., 2012; Wang et al., 2013; Feng et al., 2015; Gentès et al., 2015). Additionally, several processes were found to cause MDF in marine organisms such as Hg excretion and co-precipitation with thiol ligands or Se-containing molecules (Sherman et al., 2013; Bolea-Fernandez et al., 2019). Excretion of iHg and MeHg via urine and feces was found to enrich body tissues in the heavy 202Hg isotope, resulting in positive MDF values (Du et al., 2021). Conversely, Hg binding with affine molecules is believed to cause the bioaccumulation of inert Hg compounds with lighter isotopic composition, resulting in low to negative MDF values (Bolea-Fernandez et al., 2019). These observations have brought new light and at the same time complicated the interpretation of Hg isotopic differences between and within organisms.
Most studies focus on muscle tissue while Hg distribution and speciation in fish organs is not homogenous (Pentreath, 1976; Mieiro et al., 2011). We demonstrated in a previous study that muscle and liver of wild seabass (Dicentrarchus labrax) differed in both speciation and isotopic composition of Hg (Pinzone et al., 2021). Since methylation and demethylation cause fractionation of Hg isotopes (Bergquist and Blum, 2007), speciation and isotopy of Hg should be considered jointly. Through the comparison of Hg mass-dependent and independent isotopic fractionation between muscle and liver, we showed how several subpopulations of seabass around Europe all seem to present in vivo demethylation processes (Cransveld et al., 2017; Pinzone et al., 2021). To be able to link Hg contamination sources to Hg found in biota, it is fundamental to determine whether and how δ202Hg and Δ199Hg values are changed by internal processes in fish, and to what extent. Similarly, the applicability of Hg isotopes as natural ecological tracers would be improved by determining the time frames represented by the different fish tissues.
In the current study, we performed one controlled feeding experiment during 9 weeks: three of acclimatization and six of controlled feeding. Three groups of juvenile seabass were each fed with pellets containing environmentally relevant MeHg concentrations and distinct Hg isotopic signatures (Control, 200 and 500 ng g−1 dw). We monitored the evolution of THg, MeHg, and iHg concentrations as well as Hg isotopic values in liver and muscle, to determine the dynamics of mercury with respect to contamination level in the fish diet.
The fish were acquired from Aquanord Ichtus in Gravelines, France. 135 juveniles were raised in the Biology Department of Antwerp University. Experimental methods complied with the regulation of the Federation of European Laboratory Animal Science Associations and were approved by the Local Ethics Committee, University of Antwerp (Permit Number: 2014-23).
The food pellets were obtained directly from the fish supplier (Aquanord Ichtus) and are the same as those that the fish were raised on. This was to limit both stress to the fish and a possible bias that could be induced by a switch in diet. The pellets originally came from Coppens International B.V. and were made out of marine by-catch or waste products (the actual composition is undisclosed).
The food pellets were manipulated to three different MeHg concentrations (Table 1). We achieved three different total Hg (THg) concentration levels: 49 ± 5 ng g−1 dry weight (Control without added MeHg), 204 ± 19 ng g−1 and 506 ng g−1 ± 71 (pellets with added MeHg). These are referred to as Control, Low, and Mod treatments hereafter (Figure 1; Table 1). All food pellets were kept at −80°C prior to use.
TABLE 1. THg, MeHg, and iHg concentrations (as ng g−1 dw), percentages of MeHg and iHg (as %), and Hg isotopic composition (as ‰) of dietary pellets for the different exposure conditions.
FIGURE 1. Experimental design. Juvenile European seabass, Dicentrarchus labrax (body mass, 98 ± 17 g; standard length, 20 ± 2 cm at t0, n = 15) were simultaneously grown under different dietary conditions during 9 weeks (three of acclimatization t0 and six of feeding experiment, t2 to t6).
The pellets were soaked in solutions of ethanol (99% ethanol denatured with methanol GPR RECTAPUR from VWR Chemicals) containing the desired concentrations of MeHg as previously described (Gonzalez et al., 2005; Feng et al., 2015). First, the MeHg chloride dilution solution was prepared by dissolving 9.98 g of MeHg chloride (STREM chemicals USA, Newburyport, MA, United States) into 16.30 g of ethanol, resulting in a MeHg concentration of .486 mg ml−1. Then, 1 and 3 ml of the MeHg solution were diluted into two distinct boxes containing 2.5 L of ethanol each (to prepare low diet and moderate diet respectively). The pellets were soaked during 48 h in their respective boxes (closed by a lid). The Control diet was obtained by soaking pellets in MeHg-free ethanol. After 48 h, the lids were removed and ethanol was completely evaporated in the dark, under a fume hood.
Hg isotopic spiking of pellets was conducted following a reference protocol validated in several Hg exposure studies, previously published (Gonzalez et al., 2005). Using pellets instead of a diet composed by biological components (full organism or tissues) is considered as more efficient with regards to the bioavailability of Hg for the consumer (Feng et al., 2015; Gentès et al., 2015). In biological tissues Hg can accumulate and transform in different forms, which affect both the profile in Hg species (iHg versus MeHg) and Hg isotopic composition. Hg spiking of pellets allows to control the concentration of MeHg in the diet, its bioavailability for assimilation in exposed fish (ca. 100%) and its isotopic composition. This would allow the direct tracing of Hg fractionation between diet to consumer, without the influence by biochemical reactions. Every week, the right daily portion was weighed for each group and kept in small individual plastic bags (3 × 7) stored at −20°C.
The fish were housed in three tanks of approximately 600 L, with 45 fish per tank. The temperature was maintained around 20°C and salinity was kept around 32. The water aeration was set to maintain 100% oxygen saturation. The water was continuously filtered through mechanical, charcoal, and extensive biological filters before being recycled. The water quality was checked daily for nitrates and ammonia levels. When ammonia levels became too high, 2/3 of the tank was replaced by new water. Despite these precautions, unexpected mortality occurred in the Control group after 7 weeks (after 4 weeks of the feeding trial, t4; Figure 1). Therefore, it was decided to refrain from further sampling in this group. Sampling from the low and moderate exposure groups continued until the 9th week.
Fifteen individuals were randomly selected to determine the average initial body length (20 ± 2 cm) and body mass (98 ± 17 g). Fish were divided between three different diet treatments (Control, Low, Mod) and fed with commercial food pellets displaying different THg concentrations (Figure 1). The Control group was fed plain commercial fish pellets (measured THg: 49 ± 5 ng g−1). The lowly contaminated group (Low) was fed pellet slightly enriched with MeHg (measured THg: 204 ± 19 ng g−1). The moderately contaminated group (Mod) was fed pellets more enriched in MeHg (measured THg: 506 ± 71 ng g−1). Concentrations of Hg species, δ202Hg and Δ199Hg values were determined in the three food pellet categories (Table 1).
Across all treatments, fish were fed daily with approximately 1% of their body mass in food pellets, for a total period of 9 weeks (except for the Control group, 4 weeks). In terms of doses, it comes to 50, 200, and 500 ng per day for the Control, Low, and Mod treatments, respectively. Uneaten pellets were removed from the water after 1 h. Feces were collected from each group separately, at the same time of fish sampling.
The first 3 weeks were an acclimatization period and all groups were fed the same Control diet. At the end of acclimatization, considered to be t0, five fish were sampled from each group (Figure 1). Contamination phase began eventually and two fish groups were switched to their respective diets for 6 weeks (Low and Mod). Five fish were harvested every 2 weeks in each group (Control, Low, and Mod) and euthanized using MS-222 (0.8 g L−1). Fish were immediately measured and weighed. The fish were dissected to sample 10 g of muscle and whole liver. All samples were stored at −20°C. Muscle and liver were later freeze-dried and ground to powder.
All fish achieved similar body length and mass after the experiment (average length = 22 ± 1.9 cm, average body mass = 115 ± 30 g, n = 12), regardless of the MeHg levels in the food pellets (Supplementary Table S1).
Detailed description of analytical procedures has been published elsewhere (Cransveld et al., 2017). Briefly, THg concentrations (expressed in ng g−1 dry weight, dw) were measured via atomic absorbance spectrometry (AAS) with a DMA-80 Milestone. MeHg and iHg concentrations were determined by isotope dilution GC-ICP-MS after a microwave-assisted extraction and derivatization. Reference materials were BCR CRM-464 (tuna fish muscle, from Adriatic Sea, certified for MeHg and THg concentration), and NIST SRM-1947 (Lake Michigan fish muscle) (Supplementary Table S2). The sum of iHg and MeHg was then used to estimate THg concentration as previously validated (Clémens et al., 2011). The T-Hg concentrations measured with the DMA-80 and those resulting from the sum of iHg and MeHg from the GC-ICP-MS analysis were compared for cross-validation of the analytical methods. The THg concentrations were validated when less than 20% of difference was found between the two analyses. Any discrepancy between the methods was assumed to derive from sample heterogeneity (Clémens et al., 2011). Mercury isotopic composition analysis was performed using cold vapor generation with MC-ICP-MS. To optimize extraction and reduce sample manipulations, samples were weighed after which nitric acid HNO3 6 N 50% was added plus 1 ml of hydrogen peroxide (H2O2) and the samples were systematically put to rest for predigestion during at least 6 h. Then, the quartz vials were sealed and put in High pressure Asher (HPA, Anton Parr) for mineralization. Afterward, the quartz vials were passed for 1–2 h in an ultrasound bath (unsealed), before their content was transferred to clean glass vials and stored at 7°C before use. Reference material NIST RM-8160 (previously UM-Almaden) and BCR CRM-464 and NIST 1947 were used as secondary standards.
MeHg recovery percentage was 90% for BCR CRM-464 and 103% for NIST 1947 (Supplementary Table S1). The long-term precision (2SD) for Hg isotopic values was satisfactory for the most analyzed reference samples: UM-Almaden (0.22 ‰ and 0.19‰, n = 25) and BCR CRM-464 (0.12 ‰ and 0.15‰, n = 7) for δ202Hg and ∆199Hg values, respectively (Supplementary Table S2).
Samples of pellets (at t0) and feces (from t2 to t6) were collected. Hg speciation and isotopic composition was conducted following the same protocol as for fish tissues (Section 2.3). However, collection of feces was not possible in a reproducible way because they were very liquid and directly recovered by the filtering system of each fish tank. Both speciation and isotopic analysis of the few collected samples resulted inconclusive, due to the extremely low Hg concentrations, the small quantity of sample (max. mg), and high sample heterogeneity (Supplementary Table S3).
Hg mass-dependent fractionation (Hg MDF) processes will be represented by δ202Hg values, which were calculated using the following equation:
Hg mass-independent fractionation (MIF) processes will be represented only as Δ199Hg values. Even MIF did not show any variation and is not shown in the manuscript.
We applied an exponential fit model that has been used to describe δ15N and δ13C turnover in a wide range of species of fish, mammals, and birds (Tieszen et al., 1983; MacAvoy et al., 2006; Madigan et al., 2012), to better understand the internal variation of Hg isotopes in the captive juvenile seabass. The equation for the exponential fit model describing isotopic turnover (Tieszen et al., 1983) is
where XXXHgt represents either δ202Hg or ∆199Hg values at time t, a represents the difference between the initial and the final steady-state isotope ratio, c represents the final steady-state isotope ratio, and λ represents the first-order rate constant (days−1). Using the exponential fit model, we calculated the half-lives of δ202Hg and Δ199Hg in muscle and liver of juvenile seabass, using the equation
where t0.5 represents the isotopic half-life or “isotopic turnover rate,” and λ represents the first-order rate constant (days−1). The isotopic half-life is defined as time required to reach 50% equilibration with the diet. This method was successfully applied in the previous literature to assess Hg stable isotopes turnover in tissues of marine fish (Kwon et al., 2016), as well as to describe δ15N and δ13C turnover in a wide range of species of fish, mammals, and birds (Tieszen et al., 1983; Madigan et al., 2012; Vander Zanden et al., 2015).
Descriptive statistics were performed on GraphPad Software (GraphPad Prism version 5.00 for Windows), and p < 0.05 was considered significant (with α = 0.05). To assess differences in Hg concentrations, speciation, and isotopic values between sites, Kruskal–Wallis (K–W) tests were used, and Dunn’s Multiple Comparison test (Dunn) was used to compare each pair of sites. The Mann-Whitney test was used for pairwise comparisons between tissues. Correlations between two variables were evaluated with Spearman’s rank correlation coefficient (Spearman).
Standard length and body mass of seabass did not differ between the three groups at the start of the experiment, t0. Standard length and body mass did not vary significantly throughout the 6 weeks of experiment (K-W; p > 0.05) (Supplementary Table S4).
At t0, the THg concentrations of the three groups were similar (p = 0.429, K-W = 1.82), resulting in a mean THg of 308 ± 56 ng g−1 and 58 ± 20 ng g−1 in muscle and liver, respectively. The same was found for MeHg concentrations, which represented 95% and 70% of THg in muscle and liver respectively, as well as iHg, which represented 4% and 28% of THg in muscle and liver respectively (Supplementary Table S5). Afterward, MeHg and iHg trends varied between tissues and treatments (Figure 2). In fish from the Control and Low diet groups, MeHg concentrations in muscle and iHg concentrations in liver were higher than those measured in the pellet throughout the entire experiment. In fish from the Mod diet group, MeHg concentrations in muscle and iHg concentrations in liver were lower than pellet between t0 and t2, and increased afterward (Figure 2).
FIGURE 2. Temporal variation of MeHg and iHg concentrations (in ng g−1 dw) in muscle (circle) and liver (triangle) of seabass Dicentrarchus labrax exposed to three distinct diets (Control in blue, Low in green, and Mod in orange) containing increasing concentration in MeHg. Data are represented as mean ± standard error. Time is expressed as t, indicating the number of weeks from the beginning of the experiment, after the acclimation period. The gray asterisk represents the MeHg and iHg concentrations in the Control diet (pellet). The gray dotted line represents the MeHg and iHg concentrations at t0.
Fish from the control group displayed similar THg and MeHg throughout the experiment (Supplementary Table S5). Both hepatic and muscle iHg did not vary throughout the experiment (p = 0.182, K-W = 3.46 and p = 0.358, K-W = 2.18, respectively). MeHg was higher in muscle compared to liver at all times (M-W; p < .001, U = 0). iHg was significantly higher than muscle iHg (M-W; p = .001, U = 28.5). Although the early fish mortality was observed in this group at t4, the constant trends of MeHg and iHg concentrations and stable isotope ratios supported the use of these data.
MeHg increased over time in muscle of fish from the Low diet group, ranging from a mean of 290 ng g−1 at t0 to 493 ng g−1 at t4 (p = 0.04, K-W = 5.78). MeHg remained similar in liver during the all experiment. In the same way, muscle iHg significantly increased over time, ranging from a mean of 10 ng g−1 at t0 to 37 ng g−1 at t4 (p = .003, K-W = 8.27). Liver iHg remained constant throughout the experiment (p = 0.177, K=W = 4.94, respectively).
Muscle MeHg increased steadily from t0 to t6, ranging between 275 and 955 ng g−1 (p < 0.001, K-W = 16.8). Muscle iHg increased steadily from t0 to t6 (p = 0.003, K-W = 13.9), ranging from 10 (lower than pellet) to 124 ng g−1. Liver MeHg increased significantly from t0 to t2, but remained constant afterward, ranging from 28 ng g−1 at t0 to 522 ng g−1 at t6 (p = 0.022, K-W = 9.61). Liver iHg increased over time from concentrations lower than pellet at t0 (mean liver iHg: 14 ng g−1) to similar ones at t6 (mean liver iHg: 51 ng g−1), showing a significant increase over time (p = .014, K-W = 10.5).
At t0, mean δ202Hg was 0.69 and .27‰ in muscle and liver, respectively (Supplementary Table S5). These values were similar to those measured in pellet (0.51 ± 0.06‰, Table 1). δ202Hg values remained stable over time (Figure 3). During the entire experiment muscle δ202Hg was significantly higher than liver δ202Hg of .43 ± 0.20‰ (M-W; p < .0001, U = 0). At t0, mean Δ199Hg was 1.43 ± 0.02‰ and 1.25 ± .07‰ in muscle and liver, respectively. Δ199Hg values remained stable over time, with a nonsignificant inter-tissue difference of 0.27 ± .48‰. Δ199Hg of fish muscle and liver were significantly lower than those measured in pellet (1.83 ± .05‰; p = 0.0002, K-W = 9.69) during the entire experiment.
FIGURE 3. Temporal variation of δ202Hg and Δ199Hg in muscle and liver of seabass Dicentrarchus labrax exposed to three distinct diets (Control in blue, Low in green, and Mod in orange) containing increasing concentration in MeHg. Data are represented as mean ± standard error. Time is expressed as t, indicating the number of weeks from the beginning of the experiment, after the acclimation period. The gray asterisk and gray dotted line represent the MeHg and iHg concentrations in the Control diet (pellet) at t0.
At t0, mean δ202Hg was 0.72 ± 0.08‰ and 0.28 ± 0.06‰ in muscle and liver, respectively (Supplementary Table S5). These values were significantly higher than those measured in pellet (−.48 ± 0.09‰, Table 1). At t0, mean Δ199Hg was 1.53 ± .09‰ and 1.30 ± 0.07‰ in muscle and liver, respectively. These values were similar to those measured in pellet (.32 ± 0.05‰). δ202Hg and Δ199Hg values in liver decreased significantly toward the isotopic composition of the pellets over time between t0 and t6 (Figure 3; p = 0.042, K-W = 7.05 and p = .0003 and K-W = 9.97). A significant decrease between t0 and t6 was also observed for both δ202Hg and Δ199Hg values in muscle (p = 0.0003, K-W = 9.97 and p < .0001, K-W = 10.4, respectively). Muscle δ202Hg and Δ199Hg remained however always higher than pellet’s values. Both δ202Hg and Δ199Hg muscle values were significantly higher than those measured in liver (p = 0.001, U = 20). δ202Hg difference between muscle and liver (.49 ± .17‰) remained similar throughout the experiment (p = .161, K-W = 5.18). Δ199Hg difference between muscle and liver (.49 ± .31‰) remained similar throughout the experiment (p = .52, K-W = 2.50).
At t0, mean δ202Hg was 0.84 ± 0.09‰ and 0.30 ± 0.19‰ in muscle and liver, respectively. At t0, mean Δ199Hg was 1.46 ± 0.06‰ and 1.24 ± 0.18‰ in muscle and liver, respectively. Both muscle and liver δ202Hg were significantly lower at t2 compared t0 (p < 0.001, K-W = 10 and p = .042, K-W = 7.05, respectively). The same was observed for Δ199Hg values (p = 0.0001, K-W = 10.5 and p < 0.0001 K-W = 6.44 for muscle and liver, respectively). At t2, liver δ202Hg and Δ199Hg values reached equilibrium with the food pellet from the Mod treatment, before reaching a steady state (t2–t6). At t2, muscle δ202Hg and Δ199Hg values reached a steady state (t2–t6), but remained higher than the food pellet. At all time, no difference was found between muscle and liver for both δ202Hg and Δ199Hg muscle (p = .057, U = 0 and p = 0.04, U = 3, respectively).
We applied the exponential fit model to describe turnover of Hg isotopes in seabass (Table 2). For the Low treatment, in muscle, the curve fit modeling (Eq. 1) provided isotopic half-lives (or turnover rates) of 33 and 14 days for δ202Hg and Δ199Hg respectively. In liver, isotopic half-lives were 3.5 and 6.7 days for δ202Hg and Δ199Hg, respectively. For the Moderate treatment, in muscle, the curve fit modeling (Eq. 1) provided isotopic half-lives of 5.5 and 8.7 days of δ202Hg and Δ199Hg respectively. In liver, isotopic half-lives were 3.4 and 2.4 days respectively (Table 1). The large 95% confidential interval found for Hg half-life in both muscle and liver of seabass from the Low diet group and muscle of seabass from the Mod diet group suggested that isotopic turnover and curve-fit estimates could be partly biased by the lack of sufficient time-points. Additionally, while an exponential trend could be satisfying for long-term observations and in the absence of MDF, when an internal MDF occurs (because of in vivo demethylation for instance), δ202Hg dynamics in the beginning of the isotopic shift should not correspond to an exponential trend. As such we will not discuss in detail these data.
TABLE 2. Parameters of the fit curve estimating the Hg isotopic turnover (δ202Hg and Δ199Hg) in each tissue and for each treatment. Y0 is the isotopic value at t0. Plateau is the isotopic value reached at the steady state. λ is the rate constant. Half-life is the time required for isotopic values to have shifted to half the span. Span is the interval between the isotopic value at t0 and at the steady state. Values are means (95% confidence interval).
THg concentrations in muscle and liver of the seabass exposed to MeHg (Low and Mod) increased during the 6 weeks of the feeding experiment (t0–t6, Supplementary Table S5). This is consistent with other studies that have documented a positive relationship between Hg concentrations in commercial food pellets and Hg concentrations in the muscle and liver tissues of other fish species (Kwon et al., 2012; Kwon et al., 2013; Wang et al., 2013). THg concentrations in liver of seabass were similar to the diet in all cases (Supplementary Table S5). As such, Hg measurements in liver of seabass are valuable integrators of short-term exposition, consistent with a previous study on wild seabass (Mieiro et al., 2011).
Unlike previous studies (Mieiro et al., 2011; Pinzone et al., 2021), THg in liver never surpassed THg in muscle in Control and exposed seabass. This discrepancy may be related to the abundance of the different Hg species in the diet (77–96% of MeHg and 6–22% of iHg, Table 1) used to contaminate the fish. We exposed seabass nearly exclusively to dietary MeHg in our treatments, while wild seabass can be exposed to higher iHg concentrations directly from the environment (Feng et al., 2015).
Δ199Hg values were much higher (by ∼.5‰) in the pellets compared to liver and muscle at t0 and in Control group (Figure 2). This shows the influence of the diet imparted before the beginning of the experiment. Juvenile fish in captivity are sometimes switched to new diets as they grow to better meet their physiological requirements (Royes and Chapman, 2003) and this might have been the case shortly before the fish farm provided the seabass for this experiment. The slight increase in Δ199Hg values with time in the Control group, mostly observed in liver, suggests that Δ199Hg values in Control fish were also slowly shifting toward the diet. The extremely slow rate of isotopic shift in the Control diet group is probably related to the so called isotopic reservoir effect: because of the low THg levels in the Control diet, the influence of recently acquired Hg into the isotopic composition of seabass is reduced (Renedo et al., 2021). As a result, seabass from the Control group will take more time to show a shift from the Δ199Hg values incorporated prior to the feeding experiment (Renedo et al., 2021).
At t0, MeHg concentrations in muscle were higher than liver in all diet groups, whereas iHg concentrations were similar (Figure 2). In the Control group, muscle MeHg concentrations remained higher than liver MeHg throughout the entire experiment. The primary target of MeHg in the body are sulfhydryl (thiol; -SH) groups of low- and high-weight biological ligands (Ajsuvakova et al., 2020). MeHg complexation with these molecules appears to regulate the accumulation and elimination in the different organs (Wagemann et al., 1998; Bridges and Zalups, 2010). This suggests that muscle is the endpoint storage compartment for dietary MeHg either directly via the blood stream, or after a transition in liver (Kwon et al., 2012; Kwon et al., 2013; Wang et al., 2013).
In the Low and Mod diet groups, iHg concentrations increased in liver of seabass from t0 to t2 and reached a plateau thereafter (Figure 2). In predators such as seabirds and whales, liver is the organ where dietary MeHg is demethylated in Hg2+ and made inert, through the co-precipitation of iHg with Se, to form HgSe granules or tiemanite (Wagemann et al., 1998; Wang et al., 2013; Kwon et al., 2014; Gentès et al., 2015; Cransveld et al., 2017; Wang and Wang, 2017). The lower MeHg in liver compared to muscle at all times, as well as the iHg plateau shown after t2 in the low and Mod diets, point to in-situ MeHg breakdown processes such as demethylation, which could serve as a buffering role for MeHg exposure in our captive seabass.
In the Low and Mod diet groups a significant increase in muscle iHg occurred between t2 and t4/t6 (Figure 2). Several hypotheses can be drawn. First, at low to moderate levels of environmental Hg pollution (represented by the Low and Mod diet groups) dietary iHg could be directly stored in muscle of seabass, like MeHg (Gentès et al., 2015). Direct storage of dietary iHg in muscle or other internal tissues (e.g., gills) has been observed in fish exposed to high inorganic Hg pollution (Rodríguez Martín-Doimeadios et al., 2014). While food pellets of the Mod diet group presented higher iHg concentrations (Mean: 30 ng g−1) with respect to the other groups, iHg in the pellets of the Control and Low treatments was similar (8 ng g−1 for both diets; Figure 1). Thus, while direct muscle bioaccumulation could be linked to the increase in iHg observed in liver of seabass from the Mod diet, it does not explain the trend in the Low diet. MeHg detoxification processes have been proposed to occur in other tissues than liver in some fish species such as tilapia (Wang et al., 2013). We could hypothesize that at higher levels of MeHg exposure, MeHg demethylation could occur also in muscle of juvenile seabass. However, the sole assessment of Hg species cannot confirm such hypothesis. Previous studies showed that Hg stable isotope ratios successfully trace the occurrence of Hg detoxification processes in marine organisms (Renedo et al., 2021). As such, we analyzed Hg MDF and MIF dynamic of our captive seabass juveniles to assess the potential co-occurrence of MeHg demethylation in muscle and liver.
Hg mass independent fractionation (Hg MIF, represented by Δ199Hg values) is caused mostly by photochemical reactions in the environment and is not modified throughout the food web, providing a unique fingerprint of Hg sources in the marine environment (Obrist et al., 2018). As such, it can be used to assess the influence of fish diet composition on the assimilation processes of Hg species assimilated by seabass.
During our experiment, a small but persistent Hg MIF difference was observed between muscle and liver of seabass from the Control and Low diet group. This Hg MIF was however too small to potentially link to metabolic Hg MIF occurring in fish organism. Previous studies showed a strong correlation between the percentage of MeHg in the diet and Δ199Hg in fish organs, attributing it to a difference of Δ199Hg between MeHg and iHg in the diet (Kwon et al., 2013). In our case Δ199Hg values did not correlate with MeHg or iHg concentrations. In liver of seabass from the Low diet, Δ199Hg values shifted slowly toward the values of pellet (from 1.4 to .5‰ in 6 weeks), whereas in the Mod Diet Δ199Hg values significantly decreased from t0 to t2 (from 1.3 to .2‰ in 2 weeks), reaching equilibrium with diet Hg MIF, that was maintained from t2 to the end of the experiment (t6). These results confirm that Δ199Hg values in captive seabass are strongly influenced by the diet and not from internal metabolic processing.
The fact that Δ199Hg values seabass values never reached equilibrium with the pellet in the Low diet group could be linked to the isotopic reservoir effect observed also in the Control group (Section 4.1). It is then possible that a longer captive experiment would have resulted in a Δ199Hg equilibrium with the diet also for the Control and Low group.
Hg MDF (represented by δ202Hg values) occurs during a variety of biological reactions and has been used to detail the processes controlling Hg transport, transformation, and bioaccumulation in wildlife (Perrot et al., 2010). As such, differences in δ202Hg values between the tissues of seabass can trace Hg isotopes fractionation during metabolic processes (Renedo et al., 2021). A positive MDF between muscle and liver was observed in the Control and Low diet groups, throughout the entire experiment (Figure 3). Despite the biases in our calculation of Hg isotopic turnover, the comparison of half-lives between tissues can help understanding such MDF shift. δ202Hg turnover rates differed a lot between tissues, ranging for example between 3 days in liver to 33 days in muscle of seabass from the Low diet group (Table 2). If there was no internal fractionation process δ202Hg should evolve at the same rate in both tissues, namely the rate at which the “new” Hg is integrated to tissues. Thus, the difference of δ202Hg turnover rates between muscle and liver indicates that an internal Hg MDF process might occur in seabass (Li et al., 2020).
Hg MDF related to MeHg demethylation is broadly accepted to occur in marine mammals, whereas its occurrence in fish is still under debate due to contrasting findings (Man et al., 2019; Wang and Tan, 2019). This underlines the importance of species-specific studies in fish species (like seabass), for which MeHg demethylation was never observed before. MeHg demethylation involves preferentially lighter isotopes via mass-dependent kinetic fractionation and generates newly formed iHg with lower δ202Hg values (Perrot et al., 2015). This kinetic MDF imparts lower or negative δ202Hg values to seabass liver. The remaining non-demethylated MeHg is then enriched in heavier Hg isotopes with higher δ202Hg values (Perrot et al., 2015). Such isotopically heavier MeHg can be transferred to muscle, which thus exhibits higher δ202Hg values compared to liver (positive MDF) (Feng et al., 2015). Our isotopic and turnover findings add up to the few experimental evidences previously published and our results of Hg species dynamic (Section 4.2), indicating that seabass may demethylate MeHg in their liver (Kwon et al., 2013; Feng et al., 2015; Gentès et al., 2015).
If diet isotopic composition and hepatic MeHg demethylation were the only factors influencing Hg isotopic dynamic in captive seabass, we would expect a steady increase in muscle δ202Hg values over time, as a result of the continuous transfer of non-demethylated MeHg from the liver, as well as similar δ202Hg dynamics in all diet groups. Although in this study captive seabass were all fed to the same type of pellets, fish from the Mod diet did not show any Hg MDF shift between muscle and liver, in contrast to the Control and Low diets. In addition, δ202Hg values in muscle and liver of seabass from the Mod diet showed a significant decrease over time (Figure 3). Muscle δ202Hg values decreased from .84‰ at t0 to -.35‰ at t2, whereas liver δ202Hg values decreased from .30‰ at t0 to -.68‰ at t2. This was concomitant to a significant increase in iHg concentrations in both tissues (Figure 2; Section 4.2). A first hypothesis could be that the δ202Hg values in muscle are influenced by the increased percentage of iHg in the pellets of the Mod diet, which was 3.75 times higher than the Control and Low diets. However, when compared to the levels of MeHg from t0 to t6 in all seabass groups, iHg always remained a small fraction of the THg accumulated in muscle (Supplementary Table S5). Even if the isotopic composition of iHg surely modifies seabass δ202Hg values, we do not expect that it would result in the large negative MDF observed in seabass from the Mod diet. We suggest that other in-vivo metabolic processes, mostly interesting the MeHg fraction, might be influencing Hg MDF rates in muscle of seabass from the Mod diet.
Fish muscle stores both dietary MeHg and non-demethylated MeHg deriving from liver. Here, MeHg can bound with thiol-ligands (-SH) or Se-containing molecules. HgSe-mediated detoxification in muscle was proposed to occur in striped dolphins and pilot whales (Li et al., 2020; Nakazawa et al., 2011). In long-finned pilot whale, muscle HgSe complexes were found depleted in lighter isotopes with respect to MeHg, because of sequential demethylation and co-precipitation of lighter Hg isotopes particles (Bolea-Fernandez et al., 2019). We could then assume that the increasing proportion of HgSe particles (represented by the increasing iHg concentrations, Figure 2) vs. MeHg in muscle of seabass could lead to the observed decrease in δ202Hg values. Although the biomineralization of HgSe nanoparticles is understood to be an efficient MeHg detoxification mechanism, the occurrence of negative MDF resulting from this process is still under debate. Indeed, Queipo-Abad et al. (2022) recently showed that in seabirds’ muscle the largest isotopic fractionation was mostly linked to demethylation rather than HgSe formation.
As for HgSe, the formation of MeHg thiol-complexes preferentially interest lighter Hg isotopes. Wiederhold et al. (2010) showed how thiol-ligands have lower δ202Hg values compared to unbound Hg in solution. As such, the negative MDF observed in muscle of captive seabass could result from the increase in thiol-bound MeHg over time. This was suggested also by Kwon et al. (2016) to explain the decrease in δ202Hg values over time in the muscle of captive tunas. The authors hypothesized that while MeHg bound to thiol-ligands with low δ202Hg values remains in muscle during internal turnover, 202Hg-enriched MeHg deriving from liver or the diet is excreted via urines and feces (Kwon et al., 2016). In our study, Hg analysis in the feces of seabass was for the most part inconclusive (Section 2.5; Supplementary Material). The few available results in feces did not show any differences in iHg concentrations over time and between diet treatments (Supplementary Table S3), suggesting no shift in the elimination rates of iHg. An increase in MeHg concentrations seemed to occur, supporting the idea of a potential increase in MeHg excretion over time. However, our data do not allow to confirm it statistically.
Previous captive studies showed that juvenile fish with high internal metabolism like amberjacks (Seriola dumerili) displayed rapid equilibration of the muscle tissue to the dietary δ202Hg within ∼30 days of the feeding experiment (Kwon et al., 2013). Our estimation of muscle δ202Hg isotopic turnover in the Mod group was even shorter (5.5 days, Table 1). This means that we can expect to observe the effects of MeHg transformation processes on muscle Hg isotopic composition of our seabass, even if our experiment lasted only 6 weeks.
Our findings suggest that at higher Hg exposure (500 ng g−1 dw, Mod diet group), muscle could become an important metabolizing tissue of dietary Hg. This could counteract the increase in muscle δ202Hg values, expected from the transfer of non-demethylated MeHg from liver, and cause a negative MDF due to MeHg in situ breakdown pathways. At this stage, our results do not allow us to confirm if the observed negative MDF is more related to the co-precipitation of dietary iHg directly stored in muscle, demethylation and co-precipitation of MeHg coming from liver or the diet, leaving this as an open question for future studies. This underlines the potential great scientific advance that compound-specific Hg stable isotope could bring in the future (Perrot et al., 2015; Queipo-Abad et al., 2022).
Trophic MDF has been observed in nature, but not universally. Significant δ202Hg trophic enrichment (≈1.5‰) has been measured in top predators such as marine mammals, but not in intermediate consumers such as fish (Tsui et al., 2020). In our study, fish exposed to the lowest dietary THg concentration (200 ng g−1) were characterized by a significant shift in δ202Hg values between muscle and liver, which is not the case for the higher exposure (500 ng g−1) (Figure 3). Moreover, muscle turnover rates were higher in seabass from the Low diet group, compared to the Mod diet (Table 2). These observations, combined with our previous studies on wild juvenile seabass (Cransveld et al., 2017; Pinzone et al., 2021), strongly suggest that the dose of dietary Hg will impact Hg fractionation and bioaccumulation rates in muscle and liver of exposed fish.
Another important factor that seemed to influence Hg stable isotopes incorporation in captive fish is the Hg species composition of the diet. If we compare Hg turnover rates estimated for juvenile seabass in this work (Table 2) to rates reported previously for tunas (calculated over 400 days for δ202Hg in muscle), we observe that δ202Hg and Δ199Hg turnovers in muscle and liver of seabass were much lower than those calculated for tuna (Kwon et al., 2016). The fraction of the different Hg species in diet and THg in fish muscle at the beginning of experiment seem to both influence Hg turnover rates (Kwon et al., 2013). As MeHg has a strong affinity with thiol- (and probably Se-) containing proteins and peptides in muscle tissues, when MeHg contamination through the diet is quite low (as for the tuna experiment), MeHg turnover in muscle tissue probably occurs simultaneously to muscle tissue turnover. Higher MeHg concentrations in the diet compared to seabass muscle, could instead lead to muscle MeHg concentrations (as for our seabass experiment), could lead to a faster metabolization of MeHg and therefore lower Hg isotopic turnover rate, as observed in our study. This finding has important implication for Hg isotopic data interpretation when comparing liver and muscle in moderately Hg-contaminated areas, underling that the type of Hg pollution (organic vs. inorganic) is another factor influencing Hg isotopic incorporation in fish.
Our experiment assesses temporal dynamics and internal variations of Hg isotope ratios in a juvenile predatory fish (European seabass, Dicentrarchus labrax) exposed to distinct environmentally relevant Hg levels. Our first important finding points to the existence of MeHg hepatic demethylation in juvenile seabass fish. Second, we suggest the occurrence of Hg detoxification processes in the muscle tissue, when fish are exposed to moderate levels of MeHg. Third, we confirm the hypothesis stated in our previous study on wild seabass about the influence of the levels and species profile of dietary Hg on the rates of Hg MDF in juvenile fish. Although a much longer feeding experiment is needed, these baseline data bring new implications in the interpretation of Hg isotope ratios in fish sampled from the uncontaminated and contaminated areas.
The original contributions presented in the study are included in the article/Supplementary Material, further inquiries can be directed to the corresponding author.
The animal study was reviewed and approved by the Local Ethics Committee, University of Antwerp (Permit Number: 2014-23).
KD, JoS, GB, and DA contributed to conception and design of the study. AC performed the sampling strategy and sample conditioning. JoS, AC, and MP performed the statistical analysis. AC, MP, ET, JyS, and SB have performed the sample preparation, sample analysis, and data treatment. AC and MP, interpreted the data and wrote the first draft, which was also edited and commented on by the other authors. All authors contributed to manuscript revision, read, and approved the submitted version.
MP and AC were supported by a FRIA doctoral fellowship (Fund for Research Training in Industry and in Agriculture). KD is a Senior FRS-FNRS Research Associate.
The authors declare that the research was conducted in the absence of any commercial or financial relationships that could be construed as a potential conflict of interest.
All claims expressed in this article are solely those of the authors and do not necessarily represent those of their affiliated organizations, or those of the publisher, the editors and the reviewers. Any product that may be evaluated in this article, or claim that may be made by its manufacturer, is not guaranteed or endorsed by the publisher.
The authors are grateful to Nemo Maes, Amit Kumr Sinha and Nitin Pipralia for great logistical assistance. Our thanks also go to Joana Cavalheiro Gomez and Julien Barre from the UPPA. Thanks to F. Remy for his help in statistical analyses.
The Supplementary Material for this article can be found online at: https://www.frontiersin.org/articles/10.3389/fenvc.2022.767202/full#supplementary-material
Ajsuvakova, O. P., Tinkov, A. A., Aschner, M., Rocha, J. B. T., Michalke, B., Skalnaya, M. G., et al. (2020). Sulfhydryl Groups as Targets of Mercury Toxicity. Coord. Chem. Rev. 417, 213343. doi:10.1016/j.ccr.2020.213343
Bergquist, B. A., and Blum, J. D. (2007). Mass-Dependent and -Independent Fractionation of Hg Isotopes by Photoreduction in Aquatic Systems. Science 318, 417–420. doi:10.1126/science.1148050
Bergquist, B. A., and Blum, J. D. (2009). The Odds and Evens of Mercury Isotopes: Applications of Mass-dependent and Mass-independent Isotope Fractionation. Elements 5, 353–357. doi:10.2113/gselements.5.6.353
Bolea-Fernandez, E., Rua-Ibarz, A., Krupp, E. M., Feldmann, J., and Vanhaecke, F. (2019). High-precision Isotopic Analysis Sheds New Light on Mercury Metabolism in Long-Finned Pilot Whales (Globicephala melas). Sci. Rep. 9, 7262. doi:10.1038/s41598-019-43825-z
Bridges, C. C., and Zalups, R. K. (2010). Transport of Inorganic Mercury and Methylmercury in Target Tissues and Organs. J. Toxicol. Environ. Health B 13, 385–410. doi:10.1080/10937401003673750
Clémens, S., Monperrus, M., Donard, O. F. X., Amouroux, D., and Guérin, T. (2011). Mercury Speciation Analysis in Seafood by Species-specific Isotope Dilution: Method Validation and Occurrence Data. Anal. Bioanal. Chem. 401, 2699–2711. doi:10.1007/s00216-011-5040-1
Cransveld, A., Amouroux, D., Tessier, E., Koutrakis, E., Ozturk, A. A., Bettoso, N., et al. (2017). Mercury Stable Isotopes Discriminate Different Populations of European Seabass and Trace Potential Hg Sources Around Europe. Environ. Sci. Technol. 51, 12219–12228. doi:10.1021/acs.est.7b01307
Du, B., Yin, R., Fu, X., Li, P., Feng, X., and Maurice, L. (2021). Use of Mercury Isotopes to Quantify Sources of Human Inorganic Mercury Exposure and Metabolic Processes in the Human Body. Environ. Int. 147, 106336. doi:10.1016/j.envint.2020.106336
Feng, C., Pedrero, Z., Gentès, S., Barre, J., Renedo, M., Tessier, E., et al. (2015). Specific Pathways of Dietary Methylmercury and Inorganic Mercury Determined by Mercury Speciation and Isotopic Composition in Zebrafish (Danio rerio). Environ. Sci. Technol. 49, 12984–12993. doi:10.1021/acs.est.5b03587
Gantner, N., Hintelmann, H., Zheng, W., and Muir, D. C. (2009). Variations in Stable Isotope Fractionation of Hg in Food Webs of Arctic Lakes. Environ. Sci. Technol. 43, 9148–9154. doi:10.1021/es901771r
Gentès, S., Maury-Brachet, R., Feng, C., Pedrero, Z., Tessier, E., Legeay, A., et al. (2015). Specific Effects of Dietary Methylmercury and Inorganic Mercury in Zebrafish (Danio rerio) Determined by Genetic, Histological, and Metallothionein Responses. Environ. Sci. Technol. 49, 14560–14569. doi:10.1021/acs.est.5b03586
Gonzalez, P., Dominique, Y., Massabuau, J. C., Boudou, A., and Bourdineaud, J. P. (2005). Comparative Effects of Dietary Methylmercury on Gene Expression in Liver, Skeletal Muscle, and Brain of the Zebrafish (Danio rerio). Environ. Sci. Technol. 39, 3972–3980. doi:10.1021/es0483490
Kwon, S. Y., Blum, J. D., Carvan, M. J., Basu, N., Head, J. A., Madenjian, C. P., et al. (2012). Absence of Fractionation of Mercury Isotopes during Trophic Transfer of Methylmercury to Freshwater Fish in Captivity. Environ. Sci. Technol. 46, 7527–7534. doi:10.1021/es300794q
Kwon, S. Y., Blum, J. D., Chen, C. Y., Meattey, D. E., and Mason, R. P. (2014). Mercury Isotope Study of Sources and Exposure Pathways of Methylmercury in Estuarine Food Webs in the Northeastern U.S. Environ. Sci. Technol. 48, 10089–10097. doi:10.1021/es5020554
Kwon, S. Y., Blum, J. D., Chirby, M. A., and Chesney, E. J. (2013). Application of Mercury Isotopes for Tracing Trophic Transfer and Internal Distribution of Mercury in marine Fish Feeding Experiments. Environ. Toxicol. Chem. 32, 2322–2330. doi:10.1002/etc.2313
Kwon, S. Y., Blum, J. D., Madigan, D. J., Block, B. A., and Popp, B. N. (2016). Quantifying Mercury Isotope Dynamics in Captive Pacific Bluefin Tuna (Thunnus Orientalis). Elem. Sci. Anthr. 4, 000088. doi:10.12952/journal.elementa.000088
Li, M., Juang, C. A., Ewald, J. D., Yin, R., Mikkelsen, B., Krabbenhoft, D. P., et al. (2020). Selenium and Stable Mercury Isotopes Provide New Insights into Mercury Toxicokinetics in Pilot Whales. Sci. Total Environ. 710, 136325. doi:10.1016/j.scitotenv.2019.136325
MacAvoy, S. E., Arneson, L. S., and Bassett, E. (2006). Correlation of Metabolism with Tissue Carbon and Nitrogen Turnover Rate in Small Mammals. Oecologia 150, 190–201. doi:10.1007/s00442-006-0522-0
Madigan, D. J., Litvin, S. Y., Popp, B. N., Carlisle, A. B., Farwell, C. J., and Block, B. A. (2012). Tissue Turnover Rates and Isotopic Trophic Discrimination Factors in the Endothermic Teleost, Pacific Bluefin Tuna (Thunnus Orientalis). PLoS One 7, e49220. doi:10.1371/journal.pone.0049220
Man, Y., Yin, R., Cai, K., Qin, C., Wang, J., Yan, H., et al. (2019). Primary Amino Acids Affect the Distribution of Methylmercury rather Than Inorganic Mercury Among Tissues of Two Farmed-Raised Fish Species. Chemosphere 225, 320–328. doi:10.1016/j.chemosphere.2019.03.058
Mieiro, C. L., Pacheco, M., Pereira, M. E., and Duarte, A. C. (2011). Mercury Organotropism in Feral European Sea Bass (Dicentrarchus labrax). Arch. Environ. Contam. Toxicol. 61, 135–143. doi:10.1007/s00244-010-9591-5
Nakazawa, E., Ikemoto, T., Hokura, A., Terada, Y., Kunito, T., Tanabe, S., et al. (2011). The Presence of Mercury Selenide in Various Tissues of the Striped Dolphin: Evidence from μ-XRF-XRD and XAFS Analyses. Metallomics 3, 719–725. doi:10.1039/c0mt00106f
Obrist, D., Kirk, J. L., Zhang, L., Sunderland, E. M., Jiskra, M., and Selin, N. E. (2018). A Review of Global Environmental Mercury Processes in Response to Human and Natural Perturbations: Changes of Emissions, Climate, and Land Use. Ambio 47, 116–140. doi:10.1007/s13280-017-1004-9
Pentreath, R. J. (1976). The Accumulation of Mercury from Food by the Plaice, Pleuronectes Platessa L. J. Exp. Mar. Biol. Ecol. 25, 51–65. doi:10.1016/0022-0981(76)90075-7
Perrot, V., Masbou, J., Pastukhov, M. V., Epov, V. N., Point, D., Bérail, S., et al. (2015). Natural Hg Isotopic Composition of Different Hg Compounds in Mammal Tissues as a Proxy for In Vivo Breakdown of Toxic Methylmercury. Metallomics 8, 170–178. doi:10.1039/c5mt00286a
Perrot, V., Epov, V. N., Pastukhov, M. V., Grebenshchikova, V. I., Zouiten, C., Sonke, J. E., et al. (2010). Tracing Sources and Bioaccumulation of Mercury in Fish of Lake Baikal− Angara River Using Hg Isotopic Composition. Environ. Sci. Technol. 44, 8030–8037. doi:10.1021/es101898e
Perrot, V., Pastukhov, M. V., Epov, V. N., Husted, S., Donard, O. F. X., and Amouroux, D. (2012). Higher Mass-independent Isotope Fractionation of Methylmercury in the Pelagic Food Web of Lake Baikal (Russia). Environ. Sci. Technol. 46, 5902–5911. doi:10.1021/es204572g
Pinzone, M., Cransveld, A., Tessier, E., Bérail, S., Schnitzler, J., Das, K., et al. (2021). Contamination Levels and Habitat Use Influence Hg Stable Isotopes and Accumulation in the European Seabass Dicentrarchus labrax. Environ. Pollut. 281, 117008. doi:10.1016/j.envpol.2021.117008
Queipo-Abad, S., Pedrero, Z., Marchán-Moreno, C., El Hanafi, K., Bérail, S., Corns, W. T., et al. (2022). New Insights into the Biomineralization of Mercury Selenide Nanoparticles through Stable Isotope Analysis in Giant Petrel Tissues. J. Hazard. Mater. 425, 127922. doi:10.1016/j.jhazmat.2021.127922
Renedo, M., Pedrero, Z., Amouroux, D., Cherel, Y., and Bustamante, P. (2021). Mercury Isotopes of Key Tissues Document Mercury Metabolic Processes in Seabirds. Chemosphere 263, 127777. doi:10.1016/j.chemosphere.2020.127777
Rodríguez Martín-Doimeadios, R. C., Berzas Nevado, J. J., Guzmán Bernardo, F. J., Jiménez Moreno, M., Arrifano, G. P., Herculano, A. M., et al. (2014). Comparative Study of Mercury Speciation in Commercial Fishes of the Brazilian Amazon. Environ. Sci. Pollut. Res. Int. 21, 7466–7479. doi:10.1007/s11356-014-2680-7
Royes, J.-A., and Chapman, F. (2003). Preparing Your Own Fish Feeds. EDIS 2003, 9. doi:10.32473/edis-fa097-2003
Selin, N. E. (2009). Global Biogeochemical Cycling of Mercury: A Review. Annu. Rev. Environ. Resour. 34, 43–63. doi:10.1146/annurev.environ.051308.084314
Senn, D. B., Chesney, E. J., Blum, J. D., Bank, M. S., Maage, A., and Shine, J. P. (2010). Stable Isotope (N, C, Hg) Study of Methylmercury Sources and Trophic Transfer in the Northern Gulf of Mexico. Environ. Sci. Technol. 44, 1630–1637. doi:10.1021/es902361j
Sherman, L. S., Blum, J. D., Franzblau, A., and Basu, N. (2013). New Insight into Biomarkers of Human Mercury Exposure Using Naturally Occurring Mercury Stable Isotopes. Environ. Sci. Technol. 47, 3403–3409. doi:10.1021/es305250z
Tieszen, L. L., Boutton, T. W., Tesdahl, K. G., and Slade, N. A. (1983). Fractionation and Turnover of Stable Carbon Isotopes in Animal Tissues: Implications for ?13C Analysis of Diet. Oecologia 57, 32–37. doi:10.1007/bf00379558
Tsui, M. T.-K., Blum, J. D., and Kwon, S. Y. (2020). Review of Stable Mercury Isotopes in Ecology and Biogeochemistry. Sci. Total Environ. 716, 135386. doi:10.1016/j.scitotenv.2019.135386
Tsui, M. T. K., Blum, J. D., Kwon, S. Y., Finlay, J. C., Balogh, S. J., and Nollet, Y. H. (2012). Sources and Transfers of Methylmercury in Adjacent River and forest Food Webs. Environ. Sci. Technol. 46, 10957–10964. doi:10.1021/es3019836
UNEP (2002). Global Mercury Assessment. Geneva: United Nation Environment Programme Chemical Branch.
UNEP (2013). Global Mercury Assessment 2013: Sources, Emissions, Releases and Environmental Transport. Geneva: United Nation Environment Programme Chemical Branch.
Vander Zanden, M. J., Clayton, M. K., Moody, E. K., Solomon, C. T., and Weidel, B. C. (2015). Stable Isotope Turnover and Half-Life in Animal Tissues: A Literature Synthesis. PLoS One 10, e0116182–16. doi:10.1371/journal.pone.0116182
Wagemann, R., Trebacz, E., Boila, G., and Lockhart, W. (1998). Methylmercury and Total Mercury in Tissues of Arctic marine Mammals. Sci. Total Environ. 218, 19–31. doi:10.1016/s0048-9697(98)00192-2
Wang, R., Feng, X.-B., and Wang, W.-X. (2013). In Vivo Mercury Methylation and Demethylation in Freshwater Tilapia Quantified by Mercury Stable Isotopes. Environ. Sci. Technol. 47, 7949–7957. doi:10.1021/es3043774
Wang, W.-X., and Tan, Q.-G. (2019). Applications of Dynamic Models in Predicting the Bioaccumulation, Transport and Toxicity of Trace Metals in Aquatic Organisms. Environ. Pollut. 252, 1561–1573. doi:10.1016/j.envpol.2019.06.043
Wang, X., and Wang, W.-X. (2017). Selenium Induces the Demethylation of Mercury in marine Fish. Environ. Pollut. 231, 1543–1551. doi:10.1016/j.envpol.2017.09.014
Wang, X., Wu, F., and Wang, W.-X. (2017). In Vivo Mercury Demethylation in a Marine Fish (Acanthopagrus Schlegeli). Environ. Sci. Technol. 51, 6441–6451. doi:10.1021/acs.est.7b00923
Keywords: Dicentrarchus labrax, mercury, methylmercury, mercury isotopy, demethylation
Citation: Pinzone M, Cransveld A, De Boeck G, Shrivastava J, Tessier E, Bérail S, Schnitzler JG, Amouroux D and Das K (2022) Dynamics of Dietary Mercury Determined by Mercury Speciation and Isotopic Composition in Dicentrarchus labrax. Front. Environ. Chem. 3:767202. doi: 10.3389/fenvc.2022.767202
Received: 30 August 2021; Accepted: 14 January 2022;
Published: 14 February 2022.
Edited by:
Marc Amyot, Université de Montréal, CanadaReviewed by:
Martin Tsui, The Chinese University of Hong Kong, ChinaCopyright © 2022 Pinzone, Cransveld, De Boeck, Shrivastava, Tessier, Bérail, Schnitzler, Amouroux and Das. This is an open-access article distributed under the terms of the Creative Commons Attribution License (CC BY). The use, distribution or reproduction in other forums is permitted, provided the original author(s) and the copyright owner(s) are credited and that the original publication in this journal is cited, in accordance with accepted academic practice. No use, distribution or reproduction is permitted which does not comply with these terms.
*Correspondence: Krishna Das, S3Jpc2huYS5kYXNAdWxpZWdlLmJl
†These authors have contributed equally to this work and share first authorship
Disclaimer: All claims expressed in this article are solely those of the authors and do not necessarily represent those of their affiliated organizations, or those of the publisher, the editors and the reviewers. Any product that may be evaluated in this article or claim that may be made by its manufacturer is not guaranteed or endorsed by the publisher.
Research integrity at Frontiers
Learn more about the work of our research integrity team to safeguard the quality of each article we publish.