- 1Physical Chemistry & Chemical Processes Laboratory, School of Environmental Engineering, Technical University of Crete (TUC), Chania, Greece
- 2Institute of Fundamental and Frontier Sciences, University of Electronic Science and Technology of China, Chengdu, China
This article presents and discusses several hot topics and current trends for Catalytic Remediation in processes of significant environmental and energy importance. These topics seem to have the potentiality to guide future endeavors and synergistic interactions of several scientific disciplines, not limited to the chemists' and chemical engineers' communities, toward a common goal of great importance that is “Toward a cleaner (green) and sustainable future.” Under this context, the following main topics and grand challenges are addressed here: (i) Further in-depth understanding and fine-tuning surface chemistry, catalytic activity, promotion, and stability, (ii) Current Advances and future perspectives in catalytic remediation of mobile and stationary sources' emissions (elimination of NOx, N2O, VOCs, CO, soot, etc.)–“Emissions Control Catalysis,” (iii) Clean energy production related catalytic reactions – H2 production and purification, (iv) Catalytic CO2 hydrogenation (CO2 utilization/recycling), (v) Photo-electro-Catalysis: Managing the effects of co-existence and mixtures of multiple pollutants in realistic conditions; understanding the interfacial reaction mechanism between reactants and photocatalysts; selectivity enhancement and intermediates/byproducts inhibition; development of catalysts with abundant and stable electrochemical active sites; precise identification of the time-resolved reaction information at the interface. Several sub-topics between them are also highlighted.
Introduction
Catalysis was essentially introduced in industrial technology growth in the 1960s, mostly focusing in refinery and petrochemical processes (Lanzafame et al., 2017). During the last two decades, catalysis recorded extraordinary growth rates, and was recognized as a multidisciplinary science, not limited in the chemists' and chemical engineers' scientific community but currently involving synergistic interactions between many other scientists, e.g., materials, electronic, and mechanical engineers, physicists, biologists, physicians, etc. (Derouane, 2001; Lanzafame et al., 2017). Catalysis has been (and continues to be) utilized in the vast majority of chemical transformations for manufacturing commodity chemicals and added-value products from raw materials and mineral resources, green chemistry, fine chemicals and pharmaceuticals production, hydrocarbons processing, wastewater treatment and water cleaning, gas pollutant emissions control, photo-electrochemistry and fuel cell technologies, and numerous other agriculture, food, energy, and environmental applications. Thus, catalysis has strongly contributed to human well-being as a result of the concomitant great advances achieved over the last years in industrial, agricultural, energy technologies, and health. Howbeit, rapid global industrialization together with population growth and the concomitant increase in energy consumption have caused a great threat to the environment due to a massive increase in the production and venting of poisonous and hazardous pollutants. Photochemical smog, stratospheric ozone depletion, acid rain, global warming, and finally climate change being the most well-known major issues resulting from the variety of pollutants emitted by human activities. Evidences reveal that we are already at a tipping point, which might lead to sudden environmental change on a global scale. In the face of these threatening environmental problems and concerns, the global community has raised awareness of the issue on several levels (individuals, local authority corporations, international organizations, and governments). Recently, the United Nation's Sustainable Development Goals and the EU's Environmental Action Plan included calls to action and priority objectives to protect life on land and water and tackling climate change, in an attempt to ensure that “we live well, with the planet's ecological limits” (European Commission, 2014; United Nations: Sustainable Development Goals, 2019). In this context, catalysis is once again at the forefront of grand challenges for the development of sustainable and clean energy generation technologies, green chemical synthesis methodologies, as well as tools and techniques for monitoring, protection, and improvement of the environment, ensuring that our achievements must not adversely affect our environment and at the same time we must mitigate any damage that has already occurred.
Since the discovery of photocatalysis technology by Fujishima and Honda (1972), the utilization of solar energy for the emerging environmental issues and energy crisis has attracted massive research interest. Various photocatalytic environmental and energy applications have been developed such as wastewater treatment, air purification, water splitting, and CO2 reduction. Within the rapid development of photocatalysis technology in the last half century, extensive studies have been carried out, which enriched the understanding of photocatalytic materials, light absorption capacity and carrier separation, and transfer properties. A structure-activity correlation has been widely reported in current literature however, except for the novel design and modification of photocatalysts, the study of mechanism-activity correlation remains a great challenge.
The so-called “Environmental Catalysis” (e.g., Centi et al., 2002; Ertl et al., 2008) provides, and continuously develops new, efficient and cost-effective “Catalytic Remediation” strategies and tools, and apparently gathers all the necessary expertise, powerfulness and flexibility in order to play a pivotal role as a leading technology toward a sustainable future and a clean environment, further improving the quality of life. In this context, the following highly challenging representative research topics could be highlighted:
• Further in-depth understanding of catalytic chemistry and promotion phenomena; development of cost-effective, ultra-active, selective, and durable catalysts; toward nano- or even single-atom catalysis.
• Further improvement in catalytic remediation of mobile and stationary sources emissions (e.g., abatement of NOx, N2O, VOCs, and gasoline-diesel-lean burn-natural gas engines emissions).
• Steps toward renewable and sustainable energy; H2 energy; fuel cells technology.
• Advanced eco-friendly management of natural gas, biogas, and CO2; CO2 capture, utilization, and storage; renewable fuels production;
• Photo-electro-catalytic materials and processes for wastewater and air pollution remediation.
• Managing the effects of co-existence and mixtures of multiple pollutants in realistic conditions.
• Understanding the interfacial reaction mechanism between reactants and photocatalysts.
• Selectivity enhancement and intermediates/byproducts inhibition in photocatalysis.
• Development of catalysts with abundant and stable electrochemical active sites.
• Precise identification of the time-resolved reaction information at the interface.
Tailoring Catalysts' Physicochemical Properties and Morphology at Nanoscale; In-Depth Understanding and Fine-Tuning Surface Chemistry, Catalytic Activity, and Stability
Thanks to the ongoing progress in nanotechnology—that provides efficient approaches and advanced methods for the rational design of nano-structured multi-component materials subjected to specific nano-morphologies (in shape, size, and composition)—great advances have been achieved in developing novel, ultra-active, selective, durable, and cost-effective catalyst formulations for environmental and energy applications. The simultaneous immense progress of state-of-the-art, in situ, high resolution, and fidelity characterization techniques has highly contributed toward these goals. Indeed, the employment of advanced operando characterization techniques, such as the in situ diffuse reflectance infrared Fourier transform spectroscopy (in situ DRIFTS) (Koukiou et al., 2007; Matsouka et al., 2008), the in situ X-ray Diffraction (in situ XRD) (Saraev et al., 2017), the in-situ transmission electron microscopy (in situ TEM) (Simonsen et al., 2010; deLaRiva et al., 2013), the in situ near-ambient pressure XPS (Mewafy et al., 2019; Zhong et al., 2019), among others, have tackled past difficulties for an in-depth understanding of complex surface chemistry and promotion phenomena involved, thus enabling catalysts performance optimization (Vayenas et al., 1994; Vayenas et al., 1992; Yentekakis et al., 2000; Koukiou et al., 2007; Matsouka et al., 2008; Simonsen et al., 2010; deLaRiva et al., 2013; Saraev et al., 2017; Kampouri and Stylianou, 2019; Mewafy et al., 2019; Zhong et al., 2019; Kyriakou et al., 2020).
Promotion of catalytic performance was and continues to be a grand topic in heterogeneous catalysis. Surface-induced and/or support-mediated catalysts' promotion methodologies for enhancing catalytic performance by using proper promoter species in catalysts' synthesis are routinely employed. Our knowledge of the mechanisms of promotion and of the mode of action of specific promoter species has been substantially improved—thus it is now possible to say that the appropriateness of a promoter species in a specific catalytic reaction and conditions could be in many cases qualitatively or even quantitatively predictable (Vayenas et al., 1992, 2001; Brosda and Vayenas, 2002; Brosda et al., 2006; Yentekakis et al., 2019a). Involved metal-metal and metal-support interactions—that play critical role in intrinsic catalytic performance—give advantages for tailoring physicochemical properties, thus fine-tuning local surface chemistry toward a successful and effective promotion (e.g., Papadakis et al., 1996; Pliangos et al., 1997; Nicole et al., 2001; Vayenas et al., 2003; Konsolakis et al., 2012; Vernoux et al., 2013; Yentekakis et al., 2018, 2019a,b).
Nanocatalysis, restricting the active phases to the nanoscale thus maximizing both (i) the number of active catalytic sites and (ii) the interaction area between the active phase and the supports (undercoordinated sites), is currently among the most important trends in heterogeneous catalysis (Turner et al., 2008; Flytzani-Stephanopoulos and Gates, 2012; Yang et al., 2013; Jones et al., 2016; Grillo et al., 2017, 2018; Datye and Wang, 2018; Akri et al., 2019; Amsler et al., 2020). The former, (i), provides efficient utilization of scarce and expensive noble metals (common active phases in catalyst formulations), besides the fact that many catalytic reaction systems are structure sensitive appearing non-linear dependence of catalytic activity on particle size; sharp size threshold in catalytic activity over particles with a small critical value of size (ca. 2 nm) above which were completely inactive, has also been reported (Turner et al., 2008). The latter, (ii), often leads to beneficial promotional effects on catalytic performance resulting from the increased metal-support interactions due to the higher number of undercoordinated sites. Several new or optimized traditional catalyst synthesis methodologies, enabling dispersion of nanoparticles with a very narrow particle size distribution, even to the level of atomic dispersion, give major advantages in nanocatalysis (e.g., Jones et al., 2016; Grillo et al., 2017, 2018; Datye and Wang, 2018; Akri et al., 2019; Goula et al., 2019; Yentekakis et al., 2019b; Amsler et al., 2020; Kyriakou et al., 2020).
However, long-term catalytic stability under typical operating conditions or during regeneration procedures is not less important in industrial catalysis than the intrinsic activity and particle dispersion issues (Moulijn et al., 2001; Argyle and Bartholomew, 2015). Thermal agglomeration, also called “sintering,” is a leading cause of the deterioration of catalytic performance of industrial catalysts operating at elevated temperatures, and still remains a key problem that receives much attention for reasons of fundamental significance and economical importance (Moulijn et al., 2001; Hansen et al., 2013; Argyle and Bartholomew, 2015; Goodman et al., 2017; Dai et al., 2018). Design of remarkably active thermal aging tolerant (the so-called “sinter-resistant”) catalysts is a hotspot issue in heterogeneous catalysis, involving in particular environmental and energy important catalytic processes (e.g., Papavasiliou et al., 2011; Yentekakis et al., 2015, 2016; Goodman et al., 2017; Goula et al., 2019). It is worth noting that besides the aforementioned advantages of nanocatalysts, they typically provide more stable and in situ regenerable formulations (Jones et al., 2016; Datye and Wang, 2018; Goula et al., 2019) due to intraparticle repulsive electrostatic forces originated from metal-support interactions (Goula et al., 2019) in combination with immobilization of catalyst atomic species on support “trapping” sites (Jones et al., 2016; Datye and Wang, 2018; Yentekakis et al., 2018; Goula et al., 2019).
The use of catalysts that restrict the active phase to the nanoscale (up to single-atom) and at the same time are durable during operation, is a long-term goal of large-scale industrial catalysis for obvious reasons (improved yield, cost-effective, environmentally friendly processes). The huge increase in knowledge and new findings in the past few years on this issue is expected to be rapidly adopted and implemented in industrial catalytic processes giving new prospects and value.
In this context, the following high challenging representative research topics could be highlighted:
• Implementation of advanced operando characterization techniques in heterogeneous catalysis.
• Implementation of new or optimized traditional catalysts synthesis methodologies for tailoring nanostructure morphology and physicochemical properties of catalysts.
• Developing of sinter-resistant catalysts; further understanding sintering mechanism.
• Implementation of new promotion methodologies; further understanding promotion in catalysis; metal-metal, metal-support interactions and structure—activity relationships.
• Steps toward nano-clusters and single-atom catalysis.
Advances in Catalytic Remediation of Mobile and Stationary Sources' Emissions (Catalytic Elimination of NOx, N2O, VOCs, CO, Soot etc.)- “Emissions Control Catalysis”
The technology of three-way catalysis (and its product three-way converter, TWC) for the simultaneous abatement of carbon monoxide (CO), unburned hydrocarbons (HCs) and nitrogen oxides (NOx = NO+NO2) emissions of stoichiometric gasoline engines, successfully records nearly 40 years of application. Commercial three-way converters (TWCs) typically use formulations of Pt/Rh, Pd/Rh, or even Pt/Pd/Rh dispersed on γ-Al2O3; the latter were usually stabilized against high temperature degradation by incorporation of low levels of alkaline earth and/or rare earth oxides, such as BaO, CeO2, La2O3. This constitutes the so-called “washcoat,” which is coated on metal foil or ceramic (cordierite) monolithic substrates in order to provide proper in size (~1–2 L) and rheology performance devices. Ce-based mixed oxides with particular emphasis to Ce-Zr-O into the γ-Al2O3 washcoat have been found to offer enhanced thermal stability and were already employed by the mid-1990s (“fourth generation” TWCs). Nowadays, TWCs containing Ce-Zr-O composites and related systems represent a well-established technology and in particular the most advanced technology for the control of cold-start emissions through the concept of close coupled catalysts (CCCs) (Yentekakis and Konsolakis, 2016). Besides thermal stabilization of the washcoat, pronounced effect of Ce-based oxides on noble metals dispersion and their intrinsic activity should be further accounted for.
Learning from this long period of TWCs application the following major and challenging aspects are still open (Yentekakis and Konsolakis, 2016): (i) Broadening the operating air/fuel ratio window (λ-window) for more tolerant systems to the lean-rich swings in engine exhaust gas composition during engine operation. (ii) Promoting intrinsic activity of the noble metals in an attempt to increase the efficiency of TWCs and simultaneously reduce the required noble metal loading (cost-effective TWCs) (Yentekakis et al., 2007). (iii) Minimizing (if possible, completely eliminating), the use of the rare and expensive Rh from the bi- or tri-metallic (Pt/Rh, Pd/Rh, Pt/Pd/Rh) formulations by promoting the De-NOx efficiency of Pt or Pd—a process for which Rh is the key TWC's component. Simple mono-metallic, cost-effective, and readily recycling TWC formulations can then be available (e.g., Papadakis et al., 1996; Yentekakis et al., 2000, 2007; Matsouka et al., 2008, 2011; Wang et al., 2010). (iv) Minimizing the formation of N2O undesirable by-products in TWCs (Yentekakis et al., 1999; Matsouka et al., 2011).
Regarding (iv), it is worth noting that improving selectivity toward N2 (instead of N2O) during NOx reduction still remains a major point in three-way catalytic chemistry, since commercial TWCs have been found to produce a small but notable amount of N2O as an undesired byproduct. However, N2O is a greenhouse gas with a global warming potential (GWP) ~300 times higher than that of CO2, and furthermore its harmful impact on the stratospheric ozone layer has been well-recognized (Ravishankara et al., 2009; Wuebbles, 2009). Therefore, its elimination from TWCs out-streams and several other industrial emissions (e.g., stationary combustion processes, use of fertilizers in land cultivation, production of adipic, and nitric acid) is an urgent need—an additional grand challenging aspect for Environmental Catalysis (Yentekakis et al., 1999, 2016, 2018, 2019a; Konsolakis et al., 2012; Yentekakis and Konsolakis, 2016).
Numerous high value and promising research findings in monometallic (Pt-only or Pd-only) TWC formulations, in which the noble metal phase is optimally promoted by cheap alkali additives (e.g., Matsouka et al., 2008; Papavasiliou et al., 2011; Yentekakis and Konsolakis, 2016), providing TWCs that meet all the above requirements, are already available in the open literature. The prospect of incorporating these innovative technological findings and knowledge into the next generation TWCs could be particularly lucrative in both environmental and economic terms.
Recent studies have also demonstrated that perovskite-type materials in combination with noble metals provide very promising catalytic formulations for TWCs. A novel, self-regenerating, noble metal-perovskite TWC has already been developed and applied in cars (Nishihata et al., 2002, 2005; Tanaka et al., 2006a,b). Further progress on the use of perovskite-based materials in TWCs and other environmental and energy processes is expected due to their adjustable solid-state properties, in particular their oxygen storage capacity (OSC) and O2− ion mobility characteristics that are often beneficial in redox reaction schemes.
The development of catalytic converters for the energy privileged diesel and lean-burn engines, offering adequate activity and selectivity, at the excess O2 conditions which they need to operate, capable to satisfy emission regulations is a long shoot goal in emissions control catalysis; the competitive role of NOx and O2 as oxidizing agents and its control is of fundamental importance here (Granger and Parvulescu, 2011). Although a remarkable progress has been achieved to this direction, further improvements are necessary. Another challenging topic in emissions control catalysis is the development of suitable catalytic converters specialized on the emissions of liquefied natural gas fueled vehicles (LNG-NGV) (Zheng and Farrauto, 2017, Farrauto et al., 2019). The topic is new and the research is mainly focusing to tackle the well-known difficult catalytic activation of methane (i.e., its low adsorption propensity (Yentekakis et al., 1998).
The vehicles industry is currently facing a growing desire for a quantum leap toward hydrogen fuel cell vehicles or battery electric vehicles (Farrauto et al., 2019). The transition to these non-fossil fuel zero emissions vehicles and their extended use may not be so far. In the case of H2-fueled vehicles, which are based on low temperature polymer electrolyte membrane fuel cells (PEM-FCs), the cost-effective procurement of H2 fuel is a critical aspect of this technology. The required clean hydrogen fuel will be produced either via water splitting electrochemical systems envisaging an ideal eco-friendly and cost-effective integrated process exploiting renewable energy resources (wind or solar energy), or via hydrocarbon (mostly methane from natural gas or biogas) reforming processes toward H2+CO (syngas). In both cases catalysis is called to play a key role (Farrauto et al., 2019). Due to the importance of the subject, further discussion is provided below.
Besides applications in automobiles, non-renewable fossil fuels (carbon, oil, natural gas) continue to remain the dominant source of energy in power plants and heat production units in industry and buildings to satisfy ever-growing energy demands. In addition, combustion processes are increasingly established in order to manage and combat the huge amount of solid wastes produced. All these activities are related with the emission of huge amounts of atmospheric pollutants, in particular NOx, owing to the high temperature combustion operation with excess air as oxidizing agent. Based on the fact that such stationary processes are not practically facing with space limitations several catalytic remediation technologies are available to reduce NOx emissions by using NH3, urea, H2 or hydrocarbons as reducing agents (Macleod et al., 2004; Konsolakis et al., 2006; Costa and Efstathiou, 2007; Kalamaras et al., 2017; Damma et al., 2019). Among them, the selective catalytic reduction of NOx with NH3 (NH3-SCR) has been widely applied due to its high NOx abatement efficiency (Damma et al., 2019). The research activity on the SCR of NOx process remains between the top subjects in heterogeneous catalysis, and the challenges are to reduce the operating temperature, to broaden the temperature window and to increase the selectivity of the process toward N2 by developing novel catalytic materials and/or using alternative reducing agents (Macleod et al., 2004; Konsolakis et al., 2006; Costa and Efstathiou, 2007; Kalamaras et al., 2017; Damma et al., 2019).
Regarding the above, the following high challenging representative research topics could be mentioned. Figure 1 illustrates the last 20 years research activity on some of these topics.
• Development of improved three-way catalytic converters (TWCs) for stoichiometric gasoline engine emissions; monometallic Pt- or Pd-only cost-effective and readily recyclable TWCs
• Development of efficient catalytic converters for lean-burn, diesel and LNG engine emissions.
• Development of efficient catalysts for low temperature CO oxidation and VOCs removal.
• Improving electrocatalytic activity, energy efficiency, and CO tolerance of low-temperature PEM fuel cells; extending the operating temperature widow of PEMs to higher temperatures.
• Development of effective catalytic materials for DeN2O and SCR DeNOx processes; lowering of the working temperature.
• Simultaneous abatement of N2O and NOx.
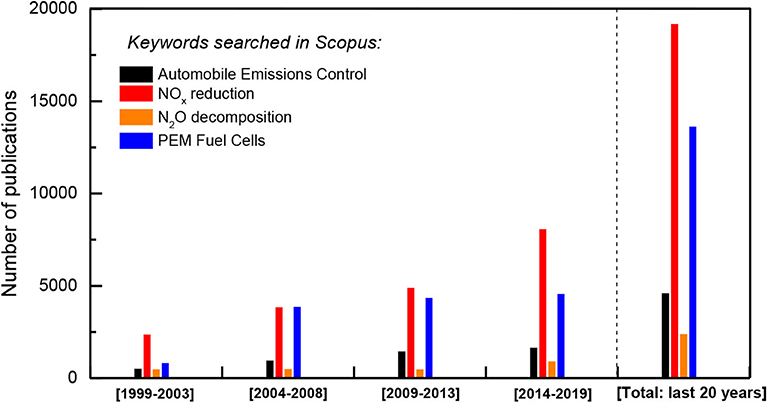
Figure 1. Last 20 years literature search on automobile and stationary sources emissions control related topics (Source: Scopus).
Clean Energy Production Related Catalytic Reactions—H2 Production and Purification
Much attention has been recently focused toward renewable energy, owing to the increasing demand for energy, the diminishing fossil fuel resources, as well as to the urgent need of actions to protect life on land and water and tackling climate change, under the general belief “we live well, with the planet's ecological limits.” Converting solar, wind, and geothermal renewable energy recourses into chemical fuels that can be stored and transported is a highly attractive vision. Under this view, the most proper fuel seems to be H2; among others, H2 is related to zero emissions during reacquisition of the “stored” chemical energy via its consumption. The hydrogen evolution reaction (HER), the half reaction of water splitting in electricity or sunlight driven water electrolysis cells, is crucial in the aforementioned energy cycle (Safizadeh et al., 2015; Gong et al., 2016; Mahammed-Ibrahim and Sun, 2019). The development of novel highly effective and stable electrocatalysts containing proper promoter species in order to expedite reaction kinetics in alkaline media via fine-tuning of their surface properties and chemistry is currently an important objective of numerous research groups worldwide (Safizadeh et al., 2015; Gong et al., 2016; Mahammed-Ibrahim and Sun, 2019).
Hydrocarbons, in particular methane, as the main component of natural gas and biogas, are also an abundant source of hydrogen. Methane reforming to syngas (H2+CO) achieved either by its reaction with steam (methane steam reforming, MSR), or with CO2 (dry reforming of methane, DRM) or even by its partial oxidation with O2 (oxy-reforming of methane, ORM) is the first step for H2 production via this resource (Verykios, 2003; Angeli et al., 2014; Lavoie, 2014; Pakhare and Spivey, 2014; Aramouni et al., 2018), which is then followed by purification processes of the reformate gas in order to obtain H2 fuel with very low CO-content suitable for transport applications. Regarding the reforming reaction, novel catalyst formulations with high reforming efficiency, low propensity for carbon formation/accumulation and long-term stability -owing to sinter-resistant catalytic particles at the typically high operating temperatures used (ca. 750–800°C) and beneficial metal-support interactions—are issues attracting significant research interest (e.g., Nikoo and Amin, 2011; Yentekakis et al., 2015, 2019b; Goula et al., 2017; Charisiou et al., 2018; Akri et al., 2019). Development of catalysts providing adequate reforming efficiency at a lower temperature operating window (ca. 550–750°C) is also a challenge for the subject (e.g., Angeli et al., 2014; Yentekakis et al., 2019b). It is also worth noting that between the aforementioned three alternative reactions for methane reforming, the case of its dry (CO2) reforming (DRM) is currently attracting resurgent interest (Verykios, 2003; Lavoie, 2014; Pakhare and Spivey, 2014; Aramouni et al., 2018; Akri et al., 2019; Yentekakis et al., 2019b). Among others, this reflects the fact that both reactants (CO2 and CH4) are important greenhouse gases. Moreover, compared to steam reforming (MSR) or partial oxidation (ORM), DRM reaction provides a route for direct utilization of biogas (consisting mainly of 50–70% CH4 and 25–50% CO2) produced via anaerobic microbial digestion or fermentation of biomass (Yentekakis and Goula, 2017). Furthermore, internal reforming of biogas by means of DRM enables its use in directly-fuelled solid oxide fuel cells, which are efficient and eco-friendly devices for the generation of electrical power (Yentekakis, 2006; Yentekakis et al., 2008; Papadam et al., 2012; Barelli and Ottaviano, 2014).
Highly dispersed, low Pt-group metal loading (ca. 0.1–1 wt%) catalysts supported on mixed oxide supports with high oxygen storage capacity and lability seems to satisfy the desirable criteria of high reforming activity and stability performance (Ashcroft et al., 1991; Pakhare and Spivey, 2014; Goodman et al., 2017; Yentekakis et al., 2019b). On the other hand, among non-noble metal catalysts, Ni-based catalysts are very active for methane reforming; however Ni shows high propensity for carbon formation and particles sintering. The use of active supports and/or dispersing Ni in atomic-scale particles was found to improve Ni performance characteristics against such problems (Goula et al., 2017; Charisiou et al., 2018; Akri et al., 2019).
A significant portion of the reformate gas (syngas: H2+CO) produced via methane reforming typically comprises feedstock for the Fischer-Tropsch industry (e.g., Jahangiri et al., 2014), where syngas is converted into hydrocarbons, or alternatively, into methanol or even ammonia. If H2 production is desired from the reformate gas CO elimination, it is demanded to be at the level of 20–50 ppm, in order to satisfy the CO-contamination limitations for its use in low temperature polymeric electrolyte membrane fuel cells (PEM-FCs) developed for transport utilities. To this line, the water gas shift reaction (WGS: CO + H2O → CO2 + H2) is of significant importance, able to reduce the undesirable CO content, simultaneously enriching the net H2 production rate (Panagiotopoulou and Kondarides, 2009; LeValley et al., 2014; Pal et al., 2018). However, further CO removal of the obtained gas is required in order to obtain the necessary very low CO-content (20–50 ppm) for PEM-FCs operation—CO is a strong poison for low temperature PEM -FCs. The preferential CO oxidation reaction (CO-PROX) is of great importance for this goal (Avgouropoulos and Ioannides, 2003). Alternatively, the selective methanation of CO can be used as the final purification step of re-formate gas for the generation of hydrogen-rich gas streams suitable for PEM fuel cell applications (Panagiotopoulou et al., 2009, 2011). Research on all these specific topics is currently hot in heterogeneous catalysis (Figures 2, 3). Besides them, other important subjects related to the application of H2-fueled vehicles technology are apparently the H2 transport safety and its storage and dispersed availability; advances on these issues will substantially contribute upon making zero emissions cars application a close reality.
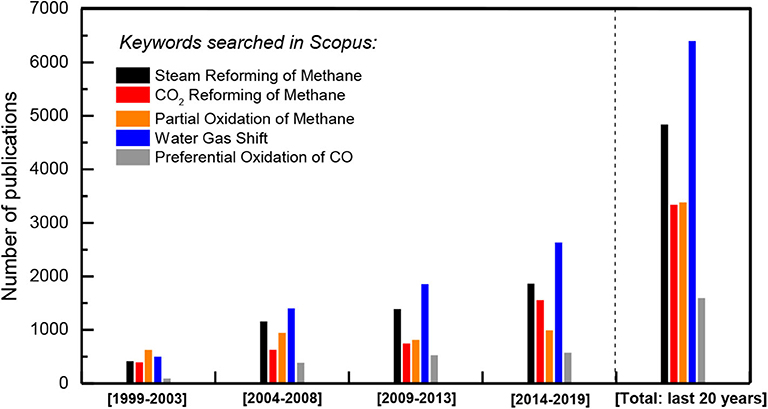
Figure 2. Literature search for the last 20 years papers production on methane reforming and reformate purification toward clean H2 fuel topics (Source: Scopus).
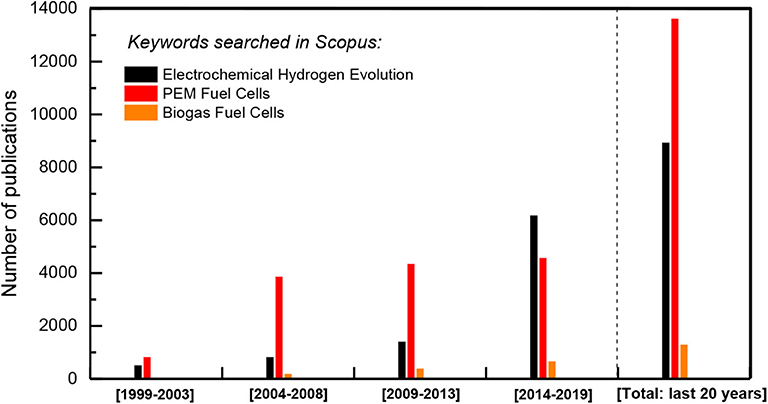
Figure 3. Literature search for the last 20 years papers production on electrochemical hydrogen and energy production issues (Source: Scopus).
The production of H2 via methane reforming is an industrially applied process. Currently, this large-scale process is mainly based on the steam reforming reaction and Ni-based catalysts, which are known to be prone to deactivation due to carbon deposition and Ni agglomeration. However, the great research interest on the dry (CO2) reforming process due to environmental issues and the current development of low loading (<0.5 wt%) and cost noble metal based catalysts, which are resistant to carbon deposition and particles agglomeration opens up new prospects for the H2 production industry, by turning its interest to the noble metal catalyzed dry reforming of methane process.
Regarding all the above, the following high challenging representative research topics could be mentioned.
• Steam reforming of methane; dry (CO2) reforming of methane; partial oxidation of methane; natural gas and biogas valorization; Syngas production; H2 production.
• Reformate gas purification; water gas shift reaction; preferential oxidation of CO (CO-PROX).
• Electrochemical water splitting-electrochemical hydrogen (or oxygen) evolution.
• Polymeric electrolyte membrane fuel cells (PEM-FCs); direct biogas fuel cells; direct hydrocarbon-fueled fuel cells.
Catalytic CO2 Hydrogenation
Despite the target and efforts for essential replacement of carbon-based fossil fuels (coal, oil, and natural gas) with eco-friendly renewable energy sources, owing to the high energy needs of modern life, fossil fuels utilization unfortunately continues to increase. This results in the emission of enormous quantities of CO2 in the atmosphere—the most contributing to global warming molecule. Scientific community currently searches new energy models with small environmental footprint that can also be combined with the so called cyclic economy and bio-refinery strategies. CO2 utilization/recycling through its catalytic conversion to added-value products and fuels (involving methane, methanol, higher hydrocarbons, alcohols, dimethyl ether, and formic acid) is nowadays among the approaches that receive intense research and technological interest (Wang et al., 2011; Yang et al., 2017; Yentekakis and Goula, 2017; Liu et al., 2019; Ye et al., 2019). Depending on the nature of the catalyst and conditions used, CO2 hydrogenation selectivity can be manipulated toward the desired product, mostly CH4 or methanol (Centi and Perathoner, 2009; Wang et al., 2011; Ganesh, 2014; Jadhav et al., 2014; Saeidi et al., 2014; Yang et al., 2017; Yentekakis and Goula, 2017; Liu et al., 2019; Ye et al., 2019). In general, elevated pressures and Cu, Cu-Zn, or Co-Ni based catalysts lead to the formation of methanol (Wang et al., 2011; Ganesh, 2014; Jadhav et al., 2014; Saeidi et al., 2014), while atmospheric pressure conditions and PGM, Ni, Fe, and Co based catalysts lead to the formation of methane, the so-called CO2 methanation or Sabatier reaction (Saeidi et al., 2014; Pal et al., 2018).
There are, however, both chemical and engineering problems to be solved in regard to CO2 hydrogenation processes toward methanol or methane. From a thermodynamic viewpoint both cases are exothermic and involve a reduction in volume; therefore are thermodynamically favored at low temperatures and elevated pressures. But low temperatures decrease reaction rates. The goal is, therefore, to develop catalysts that are sufficiently active and selective at low temperatures and, if possible, at atmospheric pressure and low, near to stoichiometry, H2/CO2 feed ratios thus saving expensive hydrogen. Given the possibility that H2 demand for CO2 hydrogenation toward methane can be provided by means of a carbon-neutral solar- or wind-powered water electrolysis plant, the overall process, called power-to-gas (P2G) process (Estermann et al., 2016; Mazza et al., 2018) can be considered as a highly convenient strategy for energy storage and distribution. On the other hand, the produced methane can follow an alternative and more attractive route, which is its utilization for ethylene (C2H4) production, via the catalytic oxidative coupling of methane (OCM) reaction (Keller and Bhasin, 1982; Ito and Lunsford, 1985). A highly effective, one-step OCM process, capable to lead up to 85% C2H4 yields has been already proposed, which gives significant advantages for industrial application (Jiang et al., 1994; Yentekakis et al., 1995, Yentekakis et al., 1996). Overall, catalytic CO2 hydrogenation is currently a priority research topic in environmental catalysis (Figure 4); surface- and/or support-induced promotion of the active phases and nano-structured catalyst architectures can be critical tailoring factors upon catalysts design for fine-tuning their activity and selectivity.
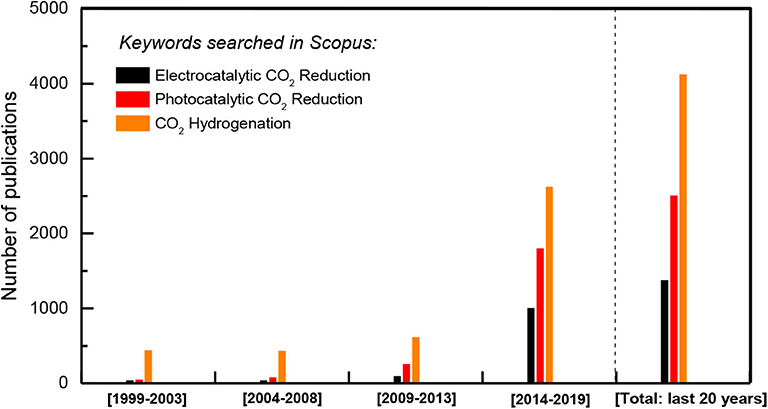
Figure 4. Literature search for the last 20 years papers production on CO2 conversion to added-value chemicals and fuels topics: heterogeneous catalytic hydrogenation, photo- and/or electro-catalytic reduction (Source: Scopus).
Besides the conventional heterogeneously catalyzed thermal CO2 hydrogenation, remarkable research efforts have been devoted for the photocatalytic and/or electrocatalytic reduction of CO2 into hydrocarbons or oxygenates as well (Kondratenko et al., 2013; de la Peña O'Shea et al., 2015; Qiao et al., 2016; Francke et al., 2018; Zhang et al., 2018; Ali et al., 2019; Albero et al., 2020; Zhu et al., 2020). This constitutes a promising approach toward the production of a variety of value-added products or fuels, such as methane, ethylene, methanol, formaldehyde, formic acid, carbon monoxide, using intermittent renewable energy sources (Francke et al., 2018; Wang et al., 2018; Zhang et al., 2018). An extremely large number of studies on this approach of CO2 utilization can be found in open literature (Figure 4), where several classes of promising materials as electrocatalysts are involved including metals and metal-alloys, inorganic metal compounds, metal-organic or metal-carbon molecular scaffold (e.g., g-C3N4) complexes with noble metal (Ru, Re, Pd, Au) centers -currently shifted toward earth-abundant metals (Ni, Co, Mn, Fe, Cu) (Riplinger and Carter, 2015; Jiao et al., 2017; Cao et al., 2018; Francke et al., 2018; Zhang et al., 2017, 2018; Hong et al., 2019; Yamazaki et al., 2019; Zhu et al., 2020). The use of organic ligands or carbon-based molecular scaffolds allowing for appropriate electronic modification of active metal centers acting cooperatively toward further tuning of the catalytic behavior and has recently become a well-established tool for catalyst performance optimization (e.g., Jiao et al., 2017; Francke et al., 2018; Zhang et al., 2018; Hong et al., 2019; Yamazaki et al., 2019; Zhu et al., 2020).
Nevertheless, aiming to the industrialization of the above approaches of CO2 utilization/recycling, substantial improvements of electrode performance and electrochemical cell designs are still required in the case direct electrocatalytic reduction in order this to become cost-attractive, while in the case of photo-electro-reduction approach a number of issues involving the low stability and/or efficiency of the photo-electro-catalysts, the limited energy efficiency, the unmanageable selectivity and a better understanding of the reaction mechanisms still remain challenging subjects and justify the intensive research interest and effort (Figure 4).
The intense interest in the CO2-hydrogenation reaction as a chemical and fuel production process is relatively recent. On the contrary, the CO-hydrogenation for this purpose through the use of synthesis gas (syngas: CO+H2) is a process that has been industrially applied for many years; the well-known Fischer-Tropsch industry. Due to the similarities on the reaction pathways and the catalytic materials used by these two reactions, it seems to be a valuable prospect of this industry to incorporate both feedstock (CO2 and syngas) and the current research finding obtained for the former reaction, thus gaining multiple merits.
Regarding to the above, the following high challenging representative research topics could be mentioned.
• CO2 hydrogenation toward methane, methanol, and other oxygenated products
• Electrocatalytic CO2 reduction into hydrocarbons and oxygenates.
• Photocatalytic and photo-electro-catalytic CO2 reduction.
Photo-Electro-Catalysis
Managing the Effects of Co-existence and Mixtures of Multiple Pollutants in Realistic Conditions
Photocatalysis has been widely developed in energy and environmental related fields (Figure 5). photocatalytic remediation technology is extensively applied for air purification and wastewater treatment, which are commonly on the basis of the activity evaluation against a single target component. However, a multitude of pollutants are present simultaneously in real-world conditions. The mechanisms of mutual effects between different pollutants are highly delicate to seek for their optimal degradation and mineralization. Taking volatile organic compounds (VOCs) as case studies, typical VOCs include hydrocarbons, aldehydes, alcohols, and aromatics, which are commonly distributed in petroleum refining, organic chemicals production, textile dyeing, and other industrial occasions (He et al., 2019). Although it has been proposed that photocatalysis is an effective approach to eliminate VOCs, which were normally investigated under the protocol of removing single component pollutants to testify the novelty of photocatalytic materials. The mutual effect between different VOCs plays a key role in achieving efficient and safe environmental remediation.
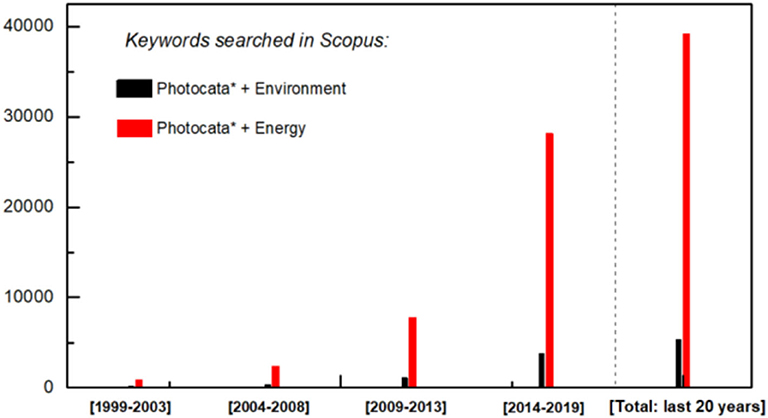
Figure 5. Literature search for the last 20 years papers production on photocatalytic environmental and energy application topic (Source: Scopus).
As described above, it is encouraged by the author that the following research topics should be considered for pollutants mixture degradation and in order to drive photocatalysis a step closer to the real application in environmental remediation:
• Competitive adsorption pattern of pollutants and reactants on catalyst surface.
• Potential mutual promotion effect and molecular interaction between multiple pollutants.
• Concentration and flow rate for pollutants mixture in a specific emission occasion.
Understanding the Interfacial Reaction Mechanism Between Reactants and Photocatalysts
Photocatalytic reactions can be divided into photophysical (light absorption and charge separation) and photochemical (surface/interfacial chemical reactions) process. Based on the advances in material science and nanotechnology, photophysical processes have been widely studied. Numerous catalyst systems and electronic structure construction have been developed to strengthen the redox ability of photocatalysts. Nevertheless, as a chemical reaction, the interfacial reaction mechanism in photocatalysis cannot be neglected. With rational regulation of the reaction pathways and reaction rates, a mechanism-activity correlation can be established, which could greatly elevate the photochemical kinetics and enhance the photocatalytic efficiency.
Based on this consideration, advanced research methods are required for reaction mechanism investigation. It is suggested that the in situ characterization technologies for reaction species detection should be primarily focused on revealing detailed reaction coordinates (Li et al., 2018a, 2020). Moreover, by conducting micro theoretical calculations, reaction rate, and activation energy can be clarified (Li et al., 2018b, 2019a). The development of combined in situ characterization technologies and theoretical calculations is a promising approach to surveying photochemical process and provide molecular insights to advance photocatalysis for environmental remediation and green energy.
Selectivity Enhancement and Intermediates/Byproducts Inhibition
For general environmental remediation technologies, the target pollutants are required to be completely mineralized into CO2 and H2O for their innocent treatment. However, the unexpected partial oxidation of pollutants is always observed, which leads to the generation of some intermediates and byproducts (Weon et al., 2019). For example in photocatalytic NO oxidation reaction, the potential concomitant production of intermediated NO2 and N2O4 is undesirable. These intermediates are more stable and toxic than NO itself and their uncontrolled release causes severe secondary pollution (Cui et al., 2017; Li et al., 2019b). To address this problem, intermediates and byproducts generated during photocatalytic remediation should be determined and quantified. Subsequent development of novel photocatalytic reactors and catalyst deposition technologies may then be helpful to prolong the retention time of pollutants and enhance the activation and transformation of intermediates, effectively impeding the accumulation of intermediates/byproducts and elevating mineralization rates.
As for the large scale application of photocatalysis, the stability and reusability of chosen photocatalysts should be principally considered. Continuous working, for months or even years of the photocatalytic system is required to guarantee the catalytic efficiency and reduce the cost of catalyst reactivation. Besides, other vital factors such as synthesis methods for mass production of photocatalysts, selectivity for specific reaction and rational design of photocatalytic reactor will contribute greatly to the real application of photocatalysis.
Development of Catalysts With Abundant and Stable Electrochemical Active Sites
Electrocatalysis is one promising alternative technology for environmental remediation (Figure 6), especially in treatment of the wastewater contaminated by persistent chemical pollutants (halogenated organics, antibiotics and ) or oxyanions (, , , and etc.) (Garcia-Segura et al., 2018; Chaplin, 2019).
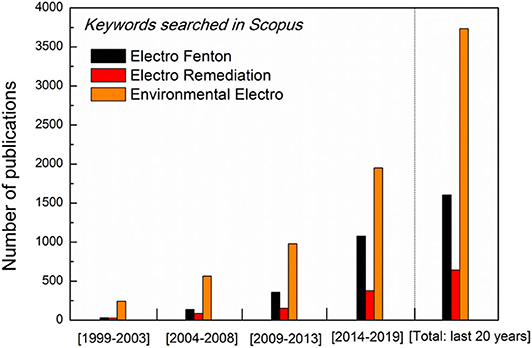
Figure 6. Literature search for the last 20 years papers production on electrocatalytic environmental remediation (Source: Scopus).
It thus shows its potential use in the safety guarantee of the urban drinking water quality, the pollution remediation in the natural surface/ground water, as well as the purification of the wastewater from agriculture, pharmaceuticals, and polymer industry.
In comparison to the well-established biological/chemical methods, the redox reactions in electrocatalysis are driven by the renewable electric energy, which thus require little maintenance (little chemicals addition and no sludge production) and can be potentially automated or remotely controlled, endowing this technology with high energy efficiency and environmental compatibility. In spite of these merits, there are several challenges that need to be addressed before scalable application of electrocatalysis in environmental remediation.
In the electrocatalytic system, the catalyst on the electrode is the vital part, which serves to adsorb, activate, and transfer the electrons from electrode to the pollutants (Fu et al., 2019). However, the development of the catalysts with abundant and stable electrochemical active sites remains a challenge. Nowadays, nanotechnology has significantly advanced electrocatalytic efficiency and reaction selectivity, but the durability of the nanocatalyst are usually questioned for their practical application (Li et al., 2019c; Peng et al., 2019; Su et al., 2019). In the current publications, the scenario of migration, aggregation, and corrosion of the nanoparticles (NPs) on electrode during the long-term reactions, and the interactions between the NPs and the surrounding chemicals (existing ions/molecules in water body or the intermediate products) are given less attention. As a result, it is difficult to evaluate the potential of the nanocatalysts in practical environmental remediation.
Precise Identification of the Time-Resolved Reaction Information at the Interface
Electrocatalysis proceeds via interfacial reactions. Thus, understanding the interactions of the pollutants, intermediate products and active sites at a catalyst surface should be one crucial gateway to the understanding of the reaction pathway and mechanism. However, due to the complexity of the reaction system, as well as the short lifetime of the intermediate products, the precise identification of the time-resolved reaction information at the interface is challenging. Recently, in situ analytical technologies, including the in situ Raman, FTIR and Mass spectrometry, have been rapidly developed and applied in some catalytic systems for mechanistic studies, which provided the opportunity (Duca et al., 2011; Ebbesen et al., 2011; Cui et al., 2017). The Catalytic Remediation section of Frontiers in Environmental Chemistry would like to stimulate such investigations with the tools of time-resolved surface analytical technology to reveal the detailed reaction information at the interface.
Faced with the complex and harsh wastewater conditions, electrocatalysis technology might sometimes become inefficient, especially when the pollutants are of a very low concentration or poor transportability. In this case, an extra technology is highly desired to improve or eliminate the weakness of the electrocatalysis. For instance, membrane technology has recently been integrated with electrolysis, and various electrocatalytic membrane reactors have been developed to promote the mass transfer of the low-concentrated pollutants around electrode, leading to the high energy efficiencies (Gayen et al., 2018; Zhu and Jassby, 2019). To improve the electrocatalysis efficiency, as well as its adaptability to the various/complex wastewater system, the authors were highly encouraged to combine the electrocatalysis with other wastewater treatment technologies.
Conclusions
This brief report on current and future trends and major challenges of catalysis in environmental and energy applications highlights the important role that environmental catalysis plays and will continue to play in the direction of a cleaner and more sustainable future for our planet.
The authors believe that by overcoming these great challenges it is possible to achieve a breakthrough for catalytic remediation and green energy. By publishing high quality articles containing not only materials design and fabrication, but also fundamental insights into the reaction nature of catalytic processes at molecular level, catalytic technology can be advanced into real utilization dealing with environmental issue and energy crisis. The Catalytic Remediation section of Frontiers in Environmental Chemistry will serve as a new platform to stimulate and discuss the scientific output in the highlighted here topics and related fields.
Author Contributions
This manuscript was co-authored by IY and FD as part of their Co-specialty Chief Editor roles. All authors contributed equally to the article and approved the submitted version.
Conflict of Interest
The authors declare that the research was conducted in the absence of any commercial or financial relationships that could be construed as a potential conflict of interest.
Acknowledgments
The Operational Program “Competitiveness, Entrepreneurship and Innovation,” under the call “RESEARCH-CREATE-INNOVATE” (project code: T1EΔ-00782) co-financed by the European Union and Greek National Funds, is gratefully acknowledged by IY.
References
Akri, M., Zhao, S., Li, X., Zang, K., Lee, A. F., Isaacs, M. A., et al. (2019). Atomically dispersed nickel as coke-resistant active sites for methane dry reforming. Nat. Commun. 10:5181. doi: 10.1038/s41467-019-12843-w
Albero, J., Peng, Y., and Garcia, H. (2020). Photocatalytic CO2 reduction to C2+ products. ACS Catal. 10, 5734–5749. doi: 10.1021/acscatal.0c00478
Ali, S., Flores, M. C., Razzaq, A., Sorcar, S., Hiragond, C. B., Kim, H. R., et al. (2019). Gas phase photocatalytic CO2 reduction, “A brief overview for benchmarking”. Catalysts 9:727. doi: 10.3390/catal9090727
Amsler, J., Sarma, B. B., Agostini, G., Prieto, G., Plessow, P. N., and Studt, F. (2020). Prospects of heterogeneous hydroformylation with supported single atom catalysts. J. Am. Chem. Soc. 142, 5087–5096. doi: 10.1021/jacs.9b12171
Angeli, S. D., Monteleone, G., Giaconia, A., and Lemonidou, A. A. (2014). State-of-the-art catalysts for CH4 steam reforming at low temperature. Int. J. Hydrog. Energy 39, 1979–1997. doi: 10.1016/j.ijhydene.2013.12.001
Aramouni, N. A. K., Touma, J. G., Tarboush, B. A., Zeaiter, J., and Ahmad, M. N. (2018). Catalyst design for dry reforming of methane: analysis review. Renew. Sust. Energy Rev. 82, 2570–2585. doi: 10.1016/j.rser.2017.09.076
Argyle, M. D., and Bartholomew, C. H. (2015). Heterogeneous catalyst deactivation and regeneration: a review. Catalysts 5, 145–269. doi: 10.3390/catal5010145
Ashcroft, A. T., Cheetham, A. K., Green, M. L. H., and Vernon, P. D. F. (1991). Partial oxidation of methane to synthesis gas using carbon dioxide. Nature 352, 225–226. doi: 10.1038/352225a0
Avgouropoulos, G., and Ioannides, T. (2003). Selective CO oxidation over CuO-CeO2 catalysts prepared via the urea-nitrate combustion method. Appl. Catal. A 244, 155–167. doi: 10.1016/S0926-860X(02)00558-6
Barelli, L., and Ottaviano, A. (2014). Solid oxide fuel cell technology coupled with methane dry reforming: a viable option for high efficiency plant with reduced CO2 emissions. Energy 71, 118–129. doi: 10.1016/j.energy.2014.04.070
Brosda, S., and Vayenas, C. G. (2002). Rules and mathematical modeling of electrochemical and classical promotion. J. Catal. 208, 38–53. doi: 10.1006/jcat.2002.3549
Brosda, S., Vayenas, C. G., and Wei, J. (2006). Rules of chemical promotion. Appl. Catal. B Environ. 68, 109–124. doi: 10.1016/j.apcatb.2006.07.021
Cao, Z., Zacate, S. B., Sun, X., Liu, J., Hale, E. M., Carson, W. P., et al. (2018). Tuning gold nanoparticles with chelating ligands for highly efficient electrocatalytic CO2 reduction. Angew. Chem. 130, 12857–12861. doi: 10.1002/ange.201805696
Centi, G., Ciambelli, P., Perathoner, S., and Russo, P. (2002). Environmental catalysis: trends and outlook. Catal. Today 75, 3–15. doi: 10.1016/S0920-5861(02)00037-8
Centi, G., and Perathoner, S. (2009). Opportunities and prospects in the chemical recycling of carbon dioxide to fuels. Catal. Today 148, 191–205. doi: 10.1016/j.cattod.2009.07.075
Chaplin, B. P. (2019). The prospect of electrochemical technologies advancing worldwide water treatment. Acc. Chem. Res. 52, 596–604. doi: 10.1021/acs.accounts.8b00611
Charisiou, N. D., Siakavelas, G., Tzounis, L., Sebastian, V., Monzon, A., Baker, M. A., et al. (2018). An in depth investigation of deactivation through carbon formation during the biogas dry reforming reaction for Ni supported on modified with CeO2 and La2O3 zirconia catalysts. Int. J. Hydrog. Energy 43, 18955–18976. doi: 10.1016/j.ijhydene.2018.08.074
Costa, C. N., and Efstathiou, A. M. (2007). Low-temperature H2-SCR of NO on a novel Pt/MgO-CeO2 catalyst. Appl. Catal. B Environ. 72, 240–252. doi: 10.1016/j.apcatb.2006.11.010
Cui, W., Li, J. Y., Dong, F., Sun, Y. J., Jiang, G. M., Cen, W. L., et al. (2017). Highly efficient performance and conversion pathway of photocatalytic NO oxidation on SrO-clusters@amorphous carbon nitride. Environ. Sci. Technol. 51, 10682–10690. doi: 10.1021/acs.est.7b00974
Dai, Y., Lu, P., Cao, Z., Campbell, C. T., and Xia, Y. (2018). The physical chemistry and materials science behind sinter-resistant catalysts. Chem. Soc. Rev. 47, 4314–4331. doi: 10.1039/C7CS00650K
Damma, D., Ettireddy, P. R., Reddy, B. M., and Smirniotis, P. G. (2019). A review of low temperature NH3-SCR for removal of NOx. Catalysts 9:349. doi: 10.3390/catal9040349
Datye, A., and Wang, Y. (2018). Atom trapping: a novel approach to generate thermally stable and regenerable single-atom catalysts. Natl. Sci. Rev. 5, 630–632. doi: 10.1093/nsr/nwy093
de la Peña O'Shea, V. A., Serrano, D. P., and Coronado, J. M. (2015). “Current challenges of CO2 photocatalytic reduction over semiconductors using sunlight,” in From Molecules to Materials. eds E. Rozhkova and K. Ariga (Cham: Springer), 171–191.
deLaRiva, A. T., Hansen, T. W., Challa, S. R., and Datye, A. K. (2013). In situ transmission electron microscopy of catalyst sintering. J. Catal. 308, 291–305. doi: 10.1016/j.jcat.2013.08.018
Derouane, E. G. (2001). Catalysis in the 21st century: lessons from the past, challenges for the future. CATTECH 5, 214–225. doi: 10.1023/A:1014036813345
Duca, M., Figueiredo, M. C., Climent, V., Rodriguez, P., Feliu, J. M., and Koper, M. T. M. (2011). Selective catalytic reduction at quasi-perfect Pt(100) domains: a universal low-temperature pathway from nitrite to N2. J. Am. Chem. Soc. 133, 10928–10939. doi: 10.1021/ja203234v
Ebbesen, S. D., Mojet, B. L., and Lefferts, L. (2011). Effect of pH on the nitrite hydrogenation mechanism over Pd/Al2O3 and Pt/Al2O3: details obtained with ATR-IR spectroscopy. J. Phys. Chem. C 115, 1186–1194. doi: 10.1021/jp106521t
Ertl, G., Knozinger, H., and Weitkamp, J., (eds) (2008). Handbook of Heterogeneous Catalysis. Vols. 4–5. Weinheim: Wiley-VCH GmbH & Co. KGaA. 1559–1695.
Estermann, T., Newborough, M., and Sterner, M. (2016). Power-to-gas systems for absorbing excess solar power in electricity distribution networks. Int. J. Hydrog. Energy 41, 13950–13959. doi: 10.1016/j.ijhydene.2016.05.278
European Commission 7th Environment Action Programme to 2020 (2014). Available online at: http://ec.europa.eu/environment/action-programme/ (accessed February 21, 2014).
Farrauto, R. J., Deeba, M., and Alerasool, S. (2019). Gasoline automobile catalysis and its historical journey to cleaner air. Nat. Catal. 2, 603–613. doi: 10.1038/s41929-019-0312-9
Flytzani-Stephanopoulos, M., and Gates, B. C. (2012). Atomically dispersed supported metal catalysts. Ann. Rev. Chem. Biomol. Eng. 3, 545–574. doi: 10.1146/annurev-chembioeng-062011-080939
Francke, R., Schille, B., and Roemelt, M. (2018). Homogeneously catalyzed electroreduction of carbon dioxide—methods, mechanisms, and catalysts. Chem. Rev. 118, 4631–4701. doi: 10.1021/acs.chemrev.7b00459
Fu, W., Shu, S., Li, J., Shi, X., Lv, X., Huang, Y. X., et al. (2019). Identifying the rate-determining step of the electrocatalytic hydrodechlorination reaction on palladium nanoparticles. Nanoscale 11, 15892–15899. doi: 10.1039/C9NR04634H
Fujishima, A., and Honda, K. (1972). Electrochemical photolysis of water at a semiconductor Electrode. Nature. 238, 37–38. doi: 10.1038/238037a0
Ganesh, I. (2014). Conversion of carbon dioxide into methanol – a potential liquid fuel: fundamental challenges and opportunities (a review). Renew. Sust. Energy Rev. 31, 221–257. doi: 10.1016/j.rser.2013.11.045
Garcia-Segura, S., Lanzarini-Lopes, M., Hristovski, K., and Westerhoff, P. (2018). Electrocatalytic reduction of nitrate: fundamentals to full-scale water treatment applications. Appl. Catal. B Environ. 236, 546–568. doi: 10.1016/j.apcatb.2018.05.041
Gayen, P., Spataro, J., Avasarala, S., Ali, A. M., Cerrato, J. M., and Chaplin, B. P. (2018). Electrocatalytic reduction of nitrate using magneli phase TiO2 reactive electrochemical membranes doped with Pd-based catalysts. Environ. Sci. Technol. 52, 9370–9379. doi: 10.1021/acs.est.8b03038
Gong, M., Wang, D.-Y., Chen, C.-C., Hwang, B.-J., and Dai, H. (2016). A mini review on nickel-based electrocatalysts for alkaline hydrogen evolution reaction. Nano Res. 9, 28–46. doi: 10.1007/s12274-015-0965-x
Goodman, E. D., Schwalbe, J. A., and Cargnello, M. (2017). Mechanistic understanding and rational design of sinter-resistant heterogeneous catalysts. ACS Catal. 7, 7156–7173. doi: 10.1021/acscatal.7b01975
Goula, G., Botzolaki, G., Osatiashtiani, A., Parlett, C. M. A., Kyriakou, G., Lambert, R. M., et al. (2019). Oxidative thermal sintering and redispersion of Rh nanoparticles on supports with high oxygen ion lability. Catalysts 9:541. doi: 10.3390/catal9060541
Goula, M. A., Charisiou, N. D., Siakavelas, G., Tzounis, L., Tsiaoussis, I., Panagiotopoulou, P., et al. (2017). Syngas production via the biogas dry reforming reaction over Ni supported on zirconia modified with CeO2 or La2O3 catalysts. Int. J. Hydrog. Energy 42, 13724–13740. doi: 10.1016/j.ijhydene.2016.11.196
Granger, P., and Parvulescu, V. I. (2011). Catalytic NOx abatement systems for mobile sources: from three-way to lean burn after-treatment technologies. Chem. Rev. 111, 3155–3207. doi: 10.1021/cr100168g
Grillo, F., Van Bui, H., La Zara, D., Aarnink, A. I., Kovalgin, A. Y., Kooyman, P., et al. (2018). From single atoms to nanoparticles: autocatalysis and metal aggregation in atomic layer deposition of Pt on TiO2 nanopowder. Small 14:1800765. doi: 10.1002/smll.201800765
Grillo, F., van Bui, H., Moulijn, J. A., Kreutzer, M. T., and van Ommen, R. (2017). Understanding and controlling the aggregative growth of platinum nanoparticles in atomic layer deposition: an avenue to size selection. J. Phys. Chem. Lett. 8, 975–983. doi: 10.1021/acs.jpclett.6b02978
Hansen, T. W., deLaRiva, A. T., Challa, S. R., and Datye, A. K. (2013). Sintering of catalytic nanoparticles: particle migration or ostwald ripening? Acc. Chem. Res. 46, 1720–1730. doi: 10.1021/ar3002427
He, C., Cheng, J., Zhang, X., Douthwaite, M., Pattisson, S., and Hao, Z. P. (2019). Recent advances in the catalytic oxidation of volatile organic compounds: a review based on pollutant sorts and sources. Chem. Rev. 119, 4471–4568. doi: 10.1021/acs.chemrev.8b00408
Hong, D., Kawanishi, T., Tsukakoshi, Y., Kotani, H., Ishizuka, T., and Kojima, T. (2019). Efficient photocatalytic CO2 reduction by a Ni(II) complex having pyridine pendants through capturing a Mg2+ ion as a lewis-acid cocatalyst. J. Am. Chem. Soc. 141, 20309–20317. doi: 10.1021/jacs.9b10597
Ito, T., and Lunsford, J. H. (1985). Synthesis of ethylene and ethane by partial oxidation of methane over lithium-doped magnesium oxide. Nature 314, 721–722. doi: 10.1038/314721b0
Jadhav, S. G., Vaidya, P. D., Bhanage, B. M., and Joshi, J. B. (2014). Catalytic carbon dioxide hydrogenation to methanol: a review of recent studies. Chem. Eng. Res. Des. 92, 2557–2567. doi: 10.1016/j.cherd.2014.03.005
Jahangiri, H., Bennett, J., Mahjoubi, P., Wilson, K., and Gu, S. (2014). A review of advanced catalyst development for Fischer–Tropsch synthesis of hydrocarbons from biomass derived syn-gas. Catal. Sci. Technol. 4, 2210–2229. doi: 10.1039/C4CY00327F
Jiang, Y., Yentekakis, I. V., and Vayenas, C. G. (1994). Methane to ethylene with 85 percent yield in a gas recycle electrocatalytic reactor-separator. Science 264, 1563–1566. doi: 10.1126/science.264.5165.1563
Jiao, Y., Zheng, Y., Chen, P., Jaroniec, M., and Qiao, S.-Z. (2017). Molecular scaffolding strategy with synergistic active centers to facilitate electrocatalytic CO2 reduction to hydrocarbon/alcohol. J. Am. Chem. Soc. 139, 18093–18100. doi: 10.1021/jacs.7b10817
Jones, J., Xiong, H., deLaRiva, A. T., Peterson, E. J., Pham, H., Challa, S. R., et al. (2016). Thermally stable single-atom platinum-on-ceria catalysts via atom trapping. Science 353, 150–154. doi: 10.1126/science.aaf8800
Kalamaras, C. M., Olympiou, G. G., Parvulescu, V. I., Cojocaru, B., and Efstathiou, A. M. (2017). Selective catalytic reduction of NO by H2/C3H6 over Pt/Ce1−xZrxO2−Δ: the synergy effect studied by transient techniques. Appl. Catal. B Environ. 206, 308–318. doi: 10.1016/j.apcatb.2017.01.052
Kampouri, S., and Stylianou, K. C. (2019). Dual-functional photocatalysis for simultaneous hydrogen production and oxidation of organic substances. ACS Catal. 9, 4247–4270; doi: 10.1021/acscatal.9b00332
Keller, G. E., and Bhasin, M. M. (1982). Synthesis of ethylene via oxidative coupling of methane. I. Determination of active catalysts. J. Catal. 73, 9–19. doi: 10.1016/0021-9517(82)90075-6
Kondratenko, E. V., Mul, G., Baltrusaitis, J., Larrazábal, G. O., and Pérez-Ramírez, J. (2013). Status and perspectives of CO2 conversion into fuels and chemicals by catalytic, photocatalytic and electrocatalytic processes. Energy Environ. Sci. 6, 3112–3135. doi: 10.1039/c3ee41272e
Konsolakis, M., Drosou, C., and Yentekakis, I. V. (2012). Support mediated promotional effects of rare earth oxides (CeO2 and La2O3) on N2O decomposition and N2O reduction by CO and C3H6 over Pt/Al2O3 structured catalysts. Appl. Catal. B Environ. 123–124, 405–413. doi: 10.1016/j.apcatb.2012.04.048
Konsolakis, M., Vrontaki, M., Avgouropoulos, G., Ioannides, T., and Yentekakis, I. V. (2006). Novel doubly-promoted catalysts for the lean NOx reduction by H2+CO:Pd(K)/Al2O3-(TiO2). Appl. Catal. B Environ. 68, 59–67. doi: 10.1016/j.apcatb.2006.07.011
Koukiou, S., Konsolakis, M., Lambert, R. M., and Yentekakis, I. V. (2007). Spectroscopic evidence for the mode of action of alkali promotersin Pt-catalyzed de-NOx chemistry. Appl. Catal. B Environ. 76, 101–106. doi: 10.1016/j.apcatb.2007.05.014
Kyriakou, V., Neagu, D., Zafeiropoulos, G., Sharma, R. K., Tang, C., Kousi, K., et al. (2020). Symmetrical exsolution of Rh nanoparticles in solid oxide cells for efficient syngas production from greenhouse gases. ACS Catal. 10, 1278–1288. doi: 10.1021/acscatal.9b04424
Lanzafame, P., Perathoner, S., Centi, G., Gross, S., and Hensen, E. J. M. (2017). Grand challenges for catalysis in the science and technology roadmap on catalysis for Europe: moving ahead for a sustainable future. Catal. Sci. Technol. 7, 5182–5194. doi: 10.1039/C7CY01067B
Lavoie, J.-M. (2014). Review on dry reforming of methane, a potentially more environmentally-friendly approach to the increasing natural gas exploitation. Front. Chem. 2:81. doi: 10.3389/fchem.2014.00081
LeValley, T. L., Richard, A. R., and Fan, M. (2014). The progress in water gas shift and steam reforming hydrogen production technologies – a review. Int. J. Hydrog. Energy 39, 16983–17000. doi: 10.1016/j.ijhydene.2014.08.041
Li, H., Guo, S. J., Shin, K., Wong, M. S., and Henkelman, G. (2019c). Design of a Pd–Au nitrite reduction catalyst by identifying and optimizing active ensembles. ACS Catal. 9, 7957–7966. doi: 10.1021/acscatal.9b02182
Li, H., Shang, H., Cao, X. M., Yang, Z. P., Ai, Z. H., and Zhang, L. Z. (2018a). Oxygen vacancies mediated complete visible light NO oxidation via side-on bridging superoxide radicals. Environ. Sci. Technol. 52, 8659–8665. doi: 10.1021/acs.est.8b01849
Li, J. Y., Cui, W., Chen, P., Dong, X. A., Chu, Y. H., Sheng, J. P., et al. (2020). Unraveling the mechanism of binary channel reactions in photocatalytic formaldehyde decomposition for promoted mineralization. Appl. Catal. B Environ. 260, 118130. doi: 10.1016/j.apcatb.2019.118130
Li, J. Y., Dong, X. A., Sun, Y. J., Jiang, G. M., Chu, Y. H., Lee, S. C., et al. (2018b). Tailoring the rate-determining step in photocatalysis via localized excess electrons for efficient and safe air cleaning. Appl. Catal. B Environ. 239, 187–195. doi: 10.1016/j.apcatb.2018.08.019
Li, J. Y., Dong, X. A., Zhang, G., Cui, W., Cen, W. L., Wu, Z. B., et al. (2019a). Probing ring-opening pathways for efficient photocatalytic toluene decomposition. J. Mater. Chem. A 7, 3366–3374. doi: 10.1039/C8TA11627J
Li, X. W., Zhang, W. D., Li, J. Y., Jiang, G. M., Zhou, Y., Lee, S. C., et al. (2019b). Transformation pathway and toxic intermediates inhibition of photocatalytic NO removal on designed Bi metal@defective Bi2O2SiO3, Appl. Catal. B Environ. 241, 187–195. doi: 10.1016/j.apcatb.2018.09.032
Liu, M., Yi, Y., Wang, L., Guo, H., and Bogaerts, A. (2019). Hydrogenation of carbon dioxide to value-added chemicals by heterogeneous catalysis and plasma catalysis. Catalysts 9:275. doi: 10.3390/catal9030275
Macleod, N., Cropley, R., Keel, J. M., and Lambert, R. M. (2004). Exploiting the synergy of titania and alumina in lean NOx reduction: in situ ammonia generation during the Pd/TiO2/Al2O3-catalysed H2/CO/NO/O2 reaction. J. Catal. 221, 20–31. doi: 10.1016/j.jcat.2003.07.005
Mahammed-Ibrahim, J., and Sun, X. (2019). Recent progress on earth abundant electrocatalysts for hydrogen evolution reaction (HER) in alkaline medium to achieve efficient water splitting – a review. J. Energy Chem. 34, 111–160. doi: 10.1016/j.jechem.2018.09.016
Matsouka, V., Konsolakis, M., Lambert, R. M., and Yentekakis, I. V. (2008). In situ DRIFTS study of the effect of structure (CeO2-La2O3) and surface (Na) modifiers on the catalytic and surface behaviour of Pt/γ-Al2O3 catalyst under simulated exhaust conditions. Appl. Catal. B Environ. 84, 715–722. doi: 10.1016/j.apcatb.2008.06.004
Matsouka, V., Konsolakis, M., Yentekakis, I. V., Papavasiliou, A., Tsetsekou, A., and Boukos, N. (2011). Thermal aging behaviour of Pt-only TWC converters under simulated exhaust conditions: effect of rare earths (CeO2, La2O3) and alkali (Na) modifiers. Top. Catal. 54, 1124–1134. doi: 10.1007/s11244-011-9734-6
Mazza, A., Bompard, E., and Chicco, G. (2018). Applications of power to gas technologies in emerging electrical systems. Renew. Sust. Energy Rev. 92, 794–806. doi: 10.1016/j.rser.2018.04.072
Mewafy, B., Paloukis, F., Papazisi, K. M., Balomenou, S. P., Luo, W., Teschner, D., et al. (2019). Influence of surface state on the electrochemical performance of nickel-based cermet electrodes during steam electrolysis. ACS Appl. Energy Mater. 2, 7045–7055. doi: 10.1021/acsaem.9b00779
Moulijn, J. A., van Diepen, A. E., and Kapteijn, F. (2001). Catalyst deactivation: is it predictable? What to do? Appl. Catal. A Gen. 212, 3–16. doi: 10.1016/S0926-860X(00)00842-5
Nicole, J., Tsiplakides, D., Pliangos, C., Verykios, X. E., Comninellis, C., and Vayenas, C. G. (2001). Electrochemical promotion and metal-support interactions. J. Catal. 204, 23–34. doi: 10.1006/jcat.2001.3360
Nikoo, M. K., and Amin, N. A. S. (2011). Thermodynamic analysis of carbon dioxide reforming of methane in view of solid carbon formation. Fuel Process. Technol. 92, 678–691. doi: 10.1016/j.fuproc.2010.11.027
Nishihata, Y., Mizuki, J., Akao, T., Tanaka, H., Uenishi, M., Kimura, M., et al. (2002). Self-regeneration of a Pd-perovskite catalyst for automotive emissions control. Nature 418, 164–167. doi: 10.1038/nature00893
Nishihata, Y., Mizuki, J., Tanaka, H., Uenishi, M., and Kimura, M. (2005). Self-regeneration of palladium-perovskite catalysts in modern automobiles. J. Phys. Chem. Solids 66, 274–282. doi: 10.1016/j.jpcs.2004.06.090
Pakhare, D., and Spivey, J. (2014). A review of dry (CO2) reforming of methane over noble metal catalysts. Chem. Soc. Rev. 43, 7813–7837. doi: 10.1039/C3CS60395D
Pal, D. B., Chand, R., Upadhyay, S. N., and Mishra, P. K. (2018). Performance of water gas shift reaction catalysts: a review. Renew. Sust. Energy Rev. 93, 549–565. doi: 10.1016/j.rser.2018.05.003
Panagiotopoulou, P., and Kondarides, D. I. (2009). Effects of alkali promotion of TiO2 on the chemisorptive properties and water–gas shift activity of supported noble metal catalysts. J. Catal. 267, 57–66. doi: 10.1016/j.jcat.2009.07.014
Panagiotopoulou, P., Kondarides, D. I., and Verykios, X. E. (2009). Selective methanation of CO over supported Ru catalysts. Appl. Catal. B Environ. 88, 470–478. doi: 10.1016/j.apcatb.2008.10.012
Panagiotopoulou, P., Kondarides, D. I., and Verykios, X. E. (2011). Mechanistic study of the selective methanation of CO over Ru/TiO2 catalyst: identification of active surface species and reaction pathways. J. Phys. Chem. C 115, 1220–1230. doi: 10.1021/jp106538z
Papadakis, V. G., Pliangos, C. A., Yentekakis, I. V., Verykios, X. E., and Vayenas, C. G. (1996). Development of high performance, Pd-based, three way catalysts. Catal. Today 29, 71–75. doi: 10.1016/0920-5861(95)00268-5
Papadam, T., Goula, G., and Yentekakis, I. V. (2012). Long-term operation stability tests of intermediate and high temperature Ni-based anodes' SOFCs directly fueled with simulated biogas mixtures. Int. J. Hydrog. Energy 37, 16680–16685. doi: 10.1016/j.ijhydene.2012.02.147
Papavasiliou, A., Tsetsekou, A., Matsouka, V., Konsolakis, M., Yentekakis, I. V., and Boukos, N. (2011). Synergistic structural and surface promotion of monometallic (Pt) TWCs: effectiveness and thermal aging tolerance. Appl. Catal. B Environ. 106, 228–241. doi: 10.1016/j.apcatb.2011.05.030
Peng, Y. Y., Cui, M. Y., Zhang, Z. Y., Shu, S., Shi, X. L., Brosnahan, J. T., et al. (2019). Bimetallic composition-promoted electrocatalytic hydrodechlorination reaction on silver–palladium alloy nanoparticles. ACS Catal. 9, 10803–10811. doi: 10.1021/acscatal.9b02282
Pliangos, C., Yentekakis, I. V., Papadakis, V. G., Vayenas, C. G., and Verykios, X. E. (1997). Support-induced promotional effects on the activity of automotive exhaust catalysts: 1. The case of oxidation of light hydrocarbons (C2H4). Appl. Catal. B Environ. 14, 161–173. doi: 10.1016/S0926-3373(97)00020-9
Qiao, J., Liu, Y, and Zhan, J. (2016). Electrochemical Reduction of Carbon Dioxide: Fundamentals and Technologies. Boca Raton, FL; London; New York, NY: CRC Press, Taylor & Francis Group.
Ravishankara, A. R., Daniel, J. S., and Portmann, R. W. (2009). Nitrous oxide (N2O): the dominant ozone-depleting substance emitted in the 21st Century. Science 326, 123–125. doi: 10.1126/science.1176985
Riplinger, C., and Carter, E. A. (2015). Influence of weak brønsted acids on electrocatalytic CO2 reduction by Manganese and Rhenium bipyridine catalysts. ACS Catal. 5, 900–908. doi: 10.1021/cs501687n
Saeidi, S., Amin, N. A. S., and Rahimpour, M. R. (2014). Hydrogenation of CO2 to value-added products—a review and potential future developments. J. CO2 Util. 5, 66–81. doi: 10.1016/j.jcou.2013.12.005
Safizadeh, F., Ghali, E., and Houlachi, G. (2015). Electrocatalysis developments for hydrogen evolution reaction in alkaline solutions - a review. Int. J. Hydrog. Energy 40, 256–274. doi: 10.1016/j.ijhydene.2014.10.109
Saraev, A. A., Vinokurov, Z. S., Kaichev, V. V., Shmakov, A. N., and Bukhtiyarov, V. I. (2017). The origin of self-sustained reaction-rate oscillations in the oxidation of methane over nickel: an operando XRD and mass spectrometry study. Catal. Sci. Technol. 7, 1646–1649. doi: 10.1039/C6CY02673G
Simonsen, S. B., Chorkendorff, I., Dahl, S., Skoglundh, M., Sehested, J., and Helveg, S. (2010). Direct observations of oxygen-induced platinum nanoparticle ripening studied by in situ TEM. J. Am. Chem. Soc. 132, 7968–7975. doi: 10.1021/ja910094r
Su, L., Han, D., Zhu, G., Xu, H., Luo, W., Wang, L., et al. (2019). Tailoring the assembly of iron nanoparticles in carbon microspheres toward high-performance electrocatalytic denitrification. Nano Lett. 19, 5423–5430. doi: 10.1021/acs.nanolett.9b01925
Tanaka, H., Taniguchi, M., Uenishi, M., Kajita, N., Tan, I., Nishihata, Y., et al. (2006a). Self-regenerating Rh- and Pt-based perovskite catalysts for automotive-emissions control. Angew. Chem. Int. Ed. 45, 5998–6002. doi: 10.1002/anie.200503938
Tanaka, H., Uenishi, M., Taniguchi, M., Tan, I., Narita, K., Kimura, M., et al. (2006b). The intelligent catalyst having the self-regenerative function of Pd, Rh and Pt for automotive emissions control. Catal. Today 117, 321–328. doi: 10.1016/j.cattod.2006.05.029
Turner, M., Golovko, V. B., Vaughan, O. P. H., Abadulkin, P. L., Berenguer-Murcia, A., Tikhov, M. S., et al. (2008). Selective oxidation with dioxygen by gold nanoparticle catalysts derived from 55-atom clusters. Nature 454, 981–983. doi: 10.1038/nature07194
United Nations: Sustainable Development Goals (2019). Available online at: https://www.un.org/sustainabledevelopment/sustainable-development-goals/
Vayenas, C. G., Bebelis, S., Yentekakis, I. V., and Lintz, H.-G. (1992). Non-faradaic electrochemical modification of catalytic activity: a status report. Catal. Today 11, 303–438. doi: 10.1016/0920-5861(92)80002-5
Vayenas, C. G., Brosda, S. C., and Pliangos, C. (2001). Rules and mathematical modeling of electrochemical and chemical promotion: 1. Reaction classification and promotional rules. J. Catal. 203, 329–350. doi: 10.1006/jcat.2001.3348
Vayenas, C. G., Brosda, S., and Pliangos, C. (2003). The double-layer approach to promotion, electrocatalysis, electrochemical promotion, and metal–support interactions. J. Catal. 216, 487–504. doi: 10.1016/S0021-9517(02)00127-6
Vayenas, C. G., Ladas, S., Bebelis, S., Yentekakis, I. V., Neophytides, S., Jiang, Y., et al. (1994). Electrochemical promotion in catalysis: non-faradaic electrochemical modification of catalytic activity, Electrochimica Acta 39, 1849–1855. doi: 10.1016/0013-4686(94)85174-3
Vernoux, P., Lizarraga, L., Tsampas, M. N., Sapountzi, F. M., de Lucas-Consuegra, A., Valverde, J.-L., et al. (2013). Ionically conducting ceramics as active catalyst supports. Chem. Rev. 113, 8192–8260. doi: 10.1021/cr4000336
Verykios, X. E. (2003). Catalytic dry reforming of natural gas for the production of chemicals and hydrogen. Int. J. Hydrog. Energy 28, 1045–1063. doi: 10.1016/S0360-3199(02)00215-X
Wang, Q. Y., Li, G. F., Zhao, B., Shen, M. Q., and Zhou, R. X. (2010). The effect of La doping on the structure of Ce0.2Zr0.8O2 and the catalytic performance of its supported Pd-only three-way catalyst, Appl. Catal. B Environ. 101, 150–159. doi: 10.1016/j.apcatb.2010.09.026
Wang, W., Wang, S. P., Ma, X. B., and Gong, J. L. (2011). Recent advances in catalytic hydrogenation of carbon dioxide. Chem. Soc. Rev. 40, 3703–3727. doi: 10.1039/c1cs15008a
Wang, Y., Chen, Z., Han, P., Du, Y., Gu, Z., Xu, X., et al. (2018). Single-atomic Cu with multiple oxygen vacancies on Ceria for electrocatalytic CO2 reduction to CH4. ACS Catal. 8, 7113–7119. doi: 10.1021/acscatal.8b01014
Weon, S., He, F., and Choi, W. Y. (2019). Status and challenges in photocatalytic nanotechnology for cleaning air polluted with volatile organic compounds: visible light utilization and catalyst deactivation. Environ. Sci. Nano 6, 3185–3214. doi: 10.1039/C9EN00891H
Wuebbles, D. J. (2009). Nitrous oxide: no laughing matter. Science 326, 56–57. doi: 10.1126/science.1179571
Yamazaki, Y., Onoda, T., Ishikawa, J., Furukawa, S., Tanaka, C., Utsugi, T., et al. (2019). Photocatalytic CO2 reduction using various heteroleptic diimine-diphosphine Cu(I) complexes as photosensitizers. Front. Chem. 7:288. doi: 10.3389/fchem.2019.00288
Yang, H., Zhang, C., Gao, P., Wang, H., Li, X., Zhong, L., et al. (2017). A review of the catalytic hydrogenation of carbon dioxide into value-added hydrocarbons. Catal. Sci. Technol. 7, 4580–4598. doi: 10.1039/C7CY01403A
Yang, X.-F., Wang, A., Qiao, B., Li, J., Liu, J., and Zhang, T. (2013). Single-atom catalysts: a new frontier in heterogeneous catalysis. Acc. Chem. Res. 46, 1740–1748. doi: 10.1021/ar300361m
Ye, R.-P., Ding, J., Gong, W., Argyle, M. D., Zhong, Q., Wang, Y., et al. (2019). CO2 hydrogenation to high-value products via heterogeneous catalysis. Nat. Commun. 10:5698. doi: 10.1038/s41467-019-13638-9
Yentekakis, I. V. (2006). Open- and closed-circuit study of an intermediate temperature SOFC directly fueled with simulated biogas mixtures. J. Power Sources 160, 422–425. doi: 10.1016/j.jpowsour.2005.12.069
Yentekakis, I. V., and Goula, G. (2017). Biogas management: advanced utilization for production of renewable energy and added-value chemicals. Front. Environ. Sci. 5:7. doi: 10.3389/fenvs.2017.00007
Yentekakis, I. V., Goula, G., Hatzisymeon, M., Betsi-Argyropoulou, I., Botzolaki, G., Kousi, K., et al. (2019b). Effect of support oxygen storage capacity on the catalytic performance of Rh nanoparticles for CO2 reforming of methane. Appl. Catal. B Environ. 243, 490–501. doi: 10.1016/j.apcatb.2018.10.048
Yentekakis, I. V., Goula, G., Kampouri, S., Betsi-Argyropoulou, I., Panagiotopoulou, P., Taylor, M. J., et al. (2018). Ir-catalyzed Nitrous oxide (N2O) decomposition: effect of the Ir particle size and metal-support interactions. Catal. Lett. 148, 341–347. doi: 10.1007/s10562-017-2233-z
Yentekakis, I. V., Goula, G., Panagiotopoulou, P., Kampouri, S., Taylor, M. J., Kyriakou, G., et al. (2016). Stabilization of catalyst particles against sintering on oxide supports with high oxygen ion lability exemplified by Ir-catalysed decomposition of N2O. Appl. Catal. B Environ. 192, 357–364. doi: 10.1016/j.apcatb.2016.04.011
Yentekakis, I. V., Goula, G., Panagiotopoulou, P., Katsoni, A., Diamadopoulos, E., Mantzavinos, D., et al. (2015). Dry reforming of methane: catalytic performance and stability of Ir catalysts supported on γ-Al2O3, Zr0.92Y0.08O2−δ (YSZ) or Ce0.9Gd0.1O2−δ (GDC) supports. Top. Catal. 58, 1228–1241. doi: 10.1007/s11244-015-0490-x
Yentekakis, I. V., Jiang, Y., Makri, M., and Vayenas, C. G. (1995). Ethylene production from methane in a gas recycle electrocatalytic reactor separator. Ionics 1, 286–291. doi: 10.1007/BF02390209
Yentekakis, I. V., and Konsolakis, M. (2016). “Three-way catalysis (Book Chapter),” in Perovskites and Related Mixed Oxides: Concepts and Applications, eds P. Granger, V. I. Parvulescu, S. Kaliaguine, and W. Prellier (Wiley-VCH Verlag GmbH & Co. KGaA), 559–586.
Yentekakis, I. V., Konsolakis, M., Lambert, R. M., Macleod, N., and Nalbantian, L. (1999). Extraordinarily effective promotion by sodium in emission control catalysis: NO reduction by propene over Na-promoted Pt/γ-Al2O3. Appl. Catal. B Environ. 22, 123–133. doi: 10.1016/S0926-3373(99)00042-9
Yentekakis, I. V., Konsolakis, M., Lambert, R. M., Palermo, A., and Tikhov, M. (2000). Successful application of electrochemical promotion to the design of effective conventional catalyst formulations. Solid State Ionics 136–137, 783–790. doi: 10.1016/S0167-2738(00)00547-6
Yentekakis, I. V., Konsolakis, M., Rapakousios, I. A., and Matsouka, V. (2007). Novel electropositively promoted monometallic (Pt-only) catalytic converters for automotive pollution control. Top. Catal. 42–43, 393–397. doi: 10.1007/s11244-007-0212-0
Yentekakis, I. V., Lambert, R. M., Konsolakis, M., and Kiousis, V. (1998). The effect of sodium on the Pd-catalyzed reduction of NO by methane. Appl. Catal. B Environ. 18, 293–305. doi: 10.1016/S0926-3373(98)00049-6
Yentekakis, I. V., Makri, M., Jiang, Y., and Vayenas, C. G. (1996). “A novel gas-recycle reactor-separator for the oxidative coupling of Methane,” in ACS Division of Petroleum Chemistry, Inc. Preprints 41, 119–124.
Yentekakis, I. V., Papadam, T., and Goula, G. (2008). Electricity production from wastewater treatment via a novel biogas-SOFC aided process. Solid State Ionics 179, 1521–1525. doi: 10.1016/j.ssi.2007.12.049
Yentekakis, I. V., Vernoux, P., Goula, G., and Caravaca, A. (2019a). Electropositive promotion by alkalies or alkaline earths of Pt-group metals in emission control catalysis: a status Report. Catalysts 9:157. doi: 10.3390/catal9020157
Zhang, L., Zhao, Z.-J., and Gong, J. (2017). Nanostructured materials for heterogeneous electrocatalytic CO2 reduction and their related reaction mechanisms. Angew. Chem. Int. Ed. 56, 11326–11353. doi: 10.1002/anie.201612214
Zhang, W., Hu, Y., Ma, L., Zhu, G., Wang, Y., Xue, X., et al. (2018). Progress and perspective of electrocatalytic CO2 reduction for renewable carbonaceous fuels and chemicals. Adv. Sci. 5:1700275. doi: 10.1002/advs.201700275
Zheng, Q., and Farrauto, R. (2017). In situ regeneration of Rhodium in three-way catalysts by aqueous ethanol injection for sustained methane emissions abatement. Catal. Commun. 95, 63–66. doi: 10.1016/j.catcom.2017.03.008
Zhong, L., Chen, D., and Zafeiratos, S. (2019). A mini review of in situ near-ambient presure XPS studies on non-noble, late transition metal catalysts. Catal. Sci. Technol. 9, 3851–3867. doi: 10.1039/C9CY00632J
Zhu, H.-J., Lu, M., Wang, Y.-R., Yao, S.-J., Zhang, M., Kan, Y.-H., et al. (2020). Efficient electron transmission in covalent organic framework nanosheets for highly active electrocatalytic carbon dioxide reduction. Nat. Comm. 11:497. doi: 10.1038/s41467-019-14237-4
Keywords: emissions control catalysis, nanocatalysis, catalysts promotion, steam or dry reforming of methane, fuel cells, CO2 hydrogenation, oxidative coupling of methane, photo-electro-catalysis in wastewater pollutants removal
Citation: Yentekakis IV and Dong F (2020) Grand Challenges for Catalytic Remediation in Environmental and Energy Applications Toward a Cleaner and Sustainable Future. Front. Environ. Chem. 1:5. doi: 10.3389/fenvc.2020.00005
Received: 21 April 2020; Accepted: 27 May 2020;
Published: 08 July 2020.
Edited by:
Mika Erik Tapio Sillanpää, Florida International University, United StatesCopyright © 2020 Yentekakis and Dong. This is an open-access article distributed under the terms of the Creative Commons Attribution License (CC BY). The use, distribution or reproduction in other forums is permitted, provided the original author(s) and the copyright owner(s) are credited and that the original publication in this journal is cited, in accordance with accepted academic practice. No use, distribution or reproduction is permitted which does not comply with these terms.
*Correspondence: Ioannis V. Yentekakis, eXllbnRlayYjeDAwMDQwO2lzYy50dWMuZ3I=; Fan Dong, ZG9uZ2ZhbiYjeDAwMDQwO3Vlc3RjLmVkdS5jbg==