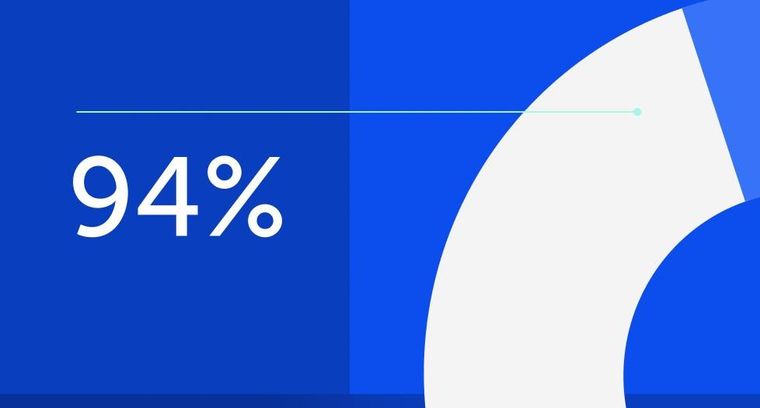
94% of researchers rate our articles as excellent or good
Learn more about the work of our research integrity team to safeguard the quality of each article we publish.
Find out more
ORIGINAL RESEARCH article
Front. Energy Res., 09 April 2025
Sec. Bioenergy and Biofuels
Volume 13 - 2025 | https://doi.org/10.3389/fenrg.2025.1547215
Introduction: Developing sustainable hydrogen production is critical for advancing renewable energy and reducing reliance on fossil fuels. Cyanobacteria, which harness solar energy through photosynthesis, provide a promising biological platform for hydrogen generation. However, improving hydrogen yields requires strategic metabolic and genetic modifications to optimize energy flow and overcome photosynthetic limitations.
Methods: Four cyanobacterial species were evaluated for their hydrogen production capacities under varying experimental conditions. Photosynthesis was partially inhibited using distinct chemical inhibitors, including 3-(3,4-dichlorophenyl)-1,1-dimethylurea (DCMU). Exogenous glycerol was introduced as a supplementary carbon source. Hydrogen production was monitored over time, and rates were normalized to chlorophyll a content. Genomic analysis of transporter proteins was conducted to identify potential genetic loci for further enhancement of hydrogen output.
Results: Nitrogen-fixing Dolichospermum sp. exhibited significantly higher hydrogen production compared to the other tested species. Supplementation with glycerol notably increased both the rate and duration of hydrogen evolution, far exceeding previously established benchmarks. The maximum hydrogen production rate for Dolichospermum sp. reached 132.3 μmol H₂/mg Chl a/h—representing a 30-fold enhancement over the rates observed with DCMU. Genomic screening revealed key transporter proteins with putative roles in carbon uptake and hydrogen metabolism.
Discussion: These findings underscore the potential of cyanobacteria, particularly Dolichospermum sp., as robust platforms for sustainable hydrogen production. The substantial improvements in hydrogen yield highlight the importance of targeted metabolic engineering and carbon supplementation strategies. Future work focused on optimizing identified transporter proteins and refining genetic interventions could further enhance biohydrogen efficiency. By leveraging the inherent photosynthetic machinery of cyanobacteria, this platform offers a renewable hydrogen source with significant promise for global energy sustainability.
• The influence of organic carbon on H2 production rate by cyanobacteria is shown
• The H2 production rate by heterocystous cyanobacterium was increased by 30-fold
• The process of hydrogen release has been extended to 46 days
• Up to 67% H2 in the gas phase was obtained for the Dolichospermum sp. IPPAS B-1213
With their remarkable metabolic versatility, Cyanobacteria hold significant promise for producing biofuels and other valuable compounds, such as molecular hydrogen, biodiesel, and bioethanol (Bolatkhan et al., 2019; Parmar et al., 2011; Salleh et al., 2016). As global fossil fuel reserves face depletion and concerns about climate change intensify, the urgent need for alternative, sustainable energy sources has become a major international priority (A.A. and T, 2007). The widespread adoption of cleaner alternatives is essential for meeting the rising energy demands of a growing global population while mitigating the negative environmental impacts of fossil fuel combustion. Hydrogen stands out as a promising candidate in this context, with its high specific heat of combustion and ability to produce only water as a byproduct when burned in pure oxygen, making it an environmentally friendly and carbon-neutral energy source (Adam et al., 2020).
Hydrogen derived from biological sources, such as cyanobacteria, among renewable energy options, offers distinct advantages. Molecular hydrogen (H2) produced by cyanobacteria relies solely on naturally abundant resources—air, sunlight, water, and trace elements—positioning it as an ideal candidate for future sustainable energy solutions (Elam et al., 2003; Sakurai et al., 2013; A.A. et al., 2019). This process circumvents the release of harmful pollutants and aligns with global carbon reduction goals, contributing to a cleaner and more sustainable energy landscape (Yeager et al., 2011). As a result, phototrophic microorganisms like cyanobacteria, capable of efficiently producing biohydrogen via photosynthesis, are under increasing scrutiny worldwide as potential contributors to renewable energy portfolios (Yeager et al., 2011; Allahverdiyeva et al., 2010; Kossalbayeu et al., 2020; Pansook et al., 2019; Touloupakis et al., 2016). To unlock the full potential of cyanobacteria in biohydrogen production, researchers are actively exploring a range of technological, genetic, and metabolic strategies to improve yield, efficiency, and scalability (Bandyopadhyay et al., 2010; Cournac et al., 2004; Kamshybayeva et al., 2022; Min and Sherman, 2010; Ananyev et al., 2008).
Cyanobacteria are among the most ancient organisms, with metabolic adaptability enabling survival in diverse environments. Their success stems from their ability to perform oxygenic photosynthesis and respiration within the same cellular compartment, supporting growth in nutrient-poor conditions (Figure 1) (Fj., 2001). Many species also fix atmospheric nitrogen, further enhancing their resilience. Cyanobacterial biohydrogen production relies on complex electron transport pathways that drive respiration and photosynthesis, facilitated by specialized protein complexes within membrane structures. These pathways converge in the thylakoid membranes, where photosynthetic and respiratory electron transport chains interact to optimize energy conversion. In most cyanobacteria, photosynthetic electron transport occurs exclusively within the thylakoids, while respiratory transport is distributed between the thylakoid and cytoplasmic membranes (Fj., 2001). This integrated system underscores cyanobacteria’s efficiency and potential as robust biohydrogen producers for sustainable energy applications.
Figure 1. Pathways of electron flow involved in cyanobacterial hydrogen metabolism. This schematic illustrates the key components and processes in cyanobacterial electron transport, highlighting interactions across photosystem I (PS I) and II (PS II), the plastoquinone pool (PQ), plastocyanin (PC), and cytochrome b6f (Cyt b6f). Additional electron carriers include ferredoxin (Fd), ferredoxin: NADP + oxidoreductase (FNR), quinol oxidase (Cyd), succinate dehydrogenase (SDH), and NADPH dehydrogenase (NDH-1 and NDH-2). Cytochrome c oxidase (Cyt ox) and cytochrome c553 (Cyt c553) are also illustrated, along with hydrogenase enzymes (Hox for bidirectional hydrogenase, Hup for uptake hydrogenase) and nitrogenase (N2ase). The Calvin–Benson–Bassham (CBB) cycle, mediated by carbonic anhydrase (CA) and ribulose-1,5-bisphosphate carboxylase oxygenase (Rubisco), plays a role in carbon fixation. Supporting pathways, such as the oxidative pentose phosphate (OPP) and tricarboxylic acid (TCA) cycle, and cofactors FADH2, NAD, and NADP are also shown (Fj., 2001; Allahverdiyeva and Kosourov, 2014; Khetkorn et al., 2013; G. and K, 2013).
The Paris Agreement aims for net-zero emissions by 2,100, necessitating drastic carbon reductions and advanced removal technologies. Algae- and cyanobacteria-based carbon capture (CCU) presents a scalable, cost-effective solution due to high CO2 uptake—up to 50 times more efficient than traditional methods—self-regeneration, and the ability to generate biofuels, bioplastics, and pharmaceuticals. However, industrial adoption faces challenges such as CO2 dissolution inefficiencies, mass transfer limitations, and high costs. Enhancing efficiency through exogenous carbon sources like glycerol or bicarbonate, alongside genetic engineering for improved growth, can help overcome these barriers. Integrating algal and cyanobacterial CCU with renewable energy and bioeconomy strategies could significantly reduce fossil fuel reliance. Additionally, biohydrogen production from cyanobacteria offers a promising route to diversifying energy resources and mitigating environmental impact. Optimizing cyanobacterial metabolic pathways for hydrogen production advances renewable energy solutions while addressing climate challenges. The research presented here provides insights into enhancing biohydrogen yields, contributing to scalable, sustainable hydrogen production to meet future global energy demands (Allahverdiyeva and Kosourov, 2014).
Cyanobacteria rely on intricate electron flow pathways to facilitate hydrogen metabolism, as shown in Figure 1 (Khetkorn et al., 2013). At the core of these pathways is reduced ferredoxin, an essential electron carrier that donates electrons for various cellular processes. Ferredoxin is particularly essential for enzymes such as ferredoxin/NADP oxidoreductase (FNR), which catalyzes the transfer of electrons from reduced ferredoxin to nicotinamide adenine dinucleotide phosphate (NADP+), forming NADPH. NADPH, a pivotal reducing agent in cyanobacterial cells, subsequently fuels hydrogen production through two main enzymatic routes: the hydrogenase and nitrogenase pathways. Importantly, electrons from oxygenic photosynthesis can be directed not only towards H2 production but also to competing pathways, including the respiratory electron transport chain and carbon fixation via the Calvin cycle, thereby influencing the net yield of hydrogen (Khetkorn et al., 2013). Cyanobacteria utilize two principal pathways for H2 production:
The Hox hydrogenase enzyme complex, part of the bidirectional NiFe hydrogenase family, catalyzes hydrogen evolution by accepting electrons from reduced NADPH. NADPH is generated either from through light-driven electron transfer from PSII through PSI to FNR; or from the catabolism of polysaccharides or lipids accumulated within cyanobacterial cells under nutrient-limited conditions, providing an endogenous substrate reservoir for H2 evolution (Mathews and Wang, 2009).
In nitrogen-fixing cyanobacteria, hydrogen production is facilitated by nitrogenase, a metalloenzyme complex with a FeMo cofactor that catalyzes N2 reduction and H2 evolution. This pathway operates under anaerobic or microaerobic conditions within specialized cells known as heterocysts, which create a low-oxygen environment favorable for nitrogenase activity. Nitrogenase-dependent H2 production involves electrons sourced from the light-driven oxidation of water at PSII and the degradation of stored carbohydrates, such as glycogen, which provides an additional electron reservoir for hydrogen evolution during dark or nitrogen-depleted conditions (G. and K, 2013).
Current biohydrogen research focuses on maximizing H2 yields by identifying robust cyanobacterial strains with enhanced hydrogenase or nitrogenase activity and optimizing growth conditions to direct electron flow toward H2 production. This involves manipulating environmental factors such as light intensity, nutrient availability, and oxygen levels to favor hydrogen metabolism over competing pathways. In this study, we optimize hydrogen production using nitrogen-fixing, heterocyst-forming cyanobacteria, which sustain hydrogen generation under low-oxygen conditions, and compare their efficiencies with non-heterocystous strains. We also explore glycerol, a biodiesel industry by-product, as an exogenous carbon source to enhance hydrogen output. Glycerol is produced in large quantities during the transesterification of triglycerides, and its surplus from biodiesel production has motivated research into its use as an alternative energy source (Yang et al., 2012). Specifically, we examine Dolichospermum sp. IPPAS B-1213, Sodalinema gerasimenkoaeIPPAS B-353, and Cyanobacterium sp. IPPAS B-1200 from the IPPAS Collection of Microalgae and Cyanobacteria at the Institute of Plant Physiology RAS, assessing their unique metabolic and physiological traits. By characterizing and comparing their hydrogen-producing efficiencies, this work aims to uncover key metabolic adaptations that can be leveraged for scalable, sustainable biohydrogen production, positioning cyanobacteria as a vital component of future renewable energy strategies.
We investigated the genome of Dolichospermum sp. IPPAS B-1213 and Anabaena for a GlpF homolog. The glycerol facilitator (GlpF) is a protein that helps glycerol and other small molecules pass through the cell membrane of many bacteria. GlpF is a member of the Major Facilitator Superfamily (MFS) family of channels, which has compared to aquaporin evolved key amino acid changes enabling selective glycerol transport. Unlike most cyanobacteria, Dolichospermum sp. IPPAS B-1213 and Anabaena possess a putative glycerol transporter. This may enhance metabolic flexibility and allow exogenous glycerol to serve as a carbon substrate that boosts hydrogen production by stimulating electron flow through photosynthetic and fermentative pathways while regenerating reducing equivalents essential for hydrogenase activity. The ability to efficiently uptake and metabolize glycerol suggests a key adaptation, positioning their GlpF homologs as critical for optimizing biohydrogen production.
This study utilized cyanobacterial strains obtained from the IPPAS Collection of Microalgae and Cyanobacteria at the Institute of Plant Physiology RAS: Dolichospermum sp. IPPAS B-1213, Sodalinema gerasimenkoae IPPAS B-353, Cyanobacterium sp. IPPAS B-1200, and Synechocystis sp. PCC 6803 GT-L (used as a positive control).
• Cyanobacterium sp. IPPAS B-1200: A unicellular cyanobacterium isolated from the saline region of Lake Balkhash in Kazakhstan. This strain rapidly grows across a 24°C–39°C temperature range (Userbaeva et al., 2017). The optimal cultivation medium is the Zarrouk medium, with a pH of 9 (Sarsekeyeva et al., 2015).
• Dolichospermum sp. IPPAS B-1213: A filamentous, nitrogen-fixing cyanobacterium with heterocysts shows intensive biomass accumulation when cultured between 32°C and 39°C (Bozieva et al., 2023). The optimal medium for this strain is BG110 medium, with a pH of 7.5 (Stanier et al., 1971).
• Sodalinema gerasimenkoae IPPAS B-353: A filamentous, non-heterocyst cyanobacterium isolated from Lake Khilganta in Transbaikalia. This strain grows best in S medium, with a pH of 9.5 (Samylina et al., 2021).
• Synechocystis sp. PCC 6803 GT-L: A well-characterized unicellular cyanobacterium was selected as a positive control due to its known ability to produce hydrogen (Chongsuksantikul et al., 2014). The optimal medium for this strain is BG11, with a pH of 7.5 (Stanier et al., 1971).
Each strain was cultured in 180 mL glass vessels containing the appropriate growth medium, maintained at the optimal temperature and pH conditions. Cultures were exposed to continuous illumination at 100–110 µmol photons/m2/s, provided by a LI-COR Biosciences light source (Lincoln, United States), and aerated with air enriched to 1.5% CO2.
The growth of cyanobacterial cultures was monitored by measuring optical density at 750 nm (OD750) using a Thermo Scientific Genesys 40 Vis UV-Vis spectrophotometer (Thermo Fisher Scientific, Waltham, MA, United States) (Sinetova et al., 2020). This wavelength was selected to minimize interference from chlorophyll and other pigments, providing an accurate cell density assessment.
Chlorophyll a (Chl a) content was quantified to evaluate the photosynthetic capacity of the cultures. Pigments were extracted by adding 5 mL of 100% methanol to 1 mL of concentrated cell suspension, followed by incubation in the dark at 4°C for 24 h to ensure complete extraction. After centrifugation at 8,500 × g for 5 min to remove cell debris, the absorbance of the supernatant was measured at 665 nm using the above spectrophotometer. Chl a concentration was calculated using the extinction coefficient and equations provided in Porra et al. (Zavřel and Červený, 2015).
To prepare the cyanobacterial cells for hydrogen (H2) production assays, cultures were grown under optimal conditions as described in Section 2.1 until they reached the desired cell density, typically after 2–3 days. Cultures were then harvested by centrifugation at 8,500 × g and 20°C for 5 min. The resulting cell pellets were washed twice with fresh nutrient medium to remove residual extracellular metabolites and then resuspended in 30 mL of the same medium. The cell suspensions were transferred into 50 mL glass bottles with rubber stoppers and aluminum caps.
To establish anaerobic conditions necessary for H2 production, the headspace of each bottle was purged with high-purity argon gas (99.999%) for 30 min. This was achieved by inserting a 19-gauge (90 mm) Quincke needle connected to the argon source into the bottle, with a second 19-gauge (40 mm) needle serving as an outlet to allow gas exchange (see Supplementary Materials S2).
Post-purging, the cultures were incubated at 32°C under two different conditions:
• Light Incubation: Bottles were exposed to continuous illumination at 100–110 µmol photons/m2/s to promote photosynthetic activity.
• Dark Incubation: Bottles were wrapped in aluminum foil to ensure complete darkness, inhibiting photosynthesis and allowing assessment of H2 production under non-photosynthetic conditions.
Cultures were maintained on a shaking platform at 100 rpm throughout incubation to ensure uniform suspension and gas exchange.
This standardized preparation protocol ensured consistent conditions for evaluating the hydrogen production capabilities of the cyanobacterial strains under study.
To enhance hydrogen production, we employed 2,5-dibromo-3-methyl-6-isopropyl-p-benzoquinone (DBMIB), a known inhibitor of plastoquinol oxidation that impedes electron flow from photosystem II (PSII) to photosystem I (PSI) (Dawson et al., 1986). DBMIB was dissolved in dimethyl sulfoxide (DMSO) to create a stock solution. Before argon purging, the DBMIB solution was added to the cyanobacterial cultures to achieve final concentrations of 5, 10, and 20 µM. The cultures were gently mixed for 5–10 min to ensure uniform distribution of the inhibitor.
Glycerol, with a purity of 99.3%, was utilized as an exogenous carbon source to potentially boost hydrogen production. Glycerol was added to the cultures at 225–1,500 mM concentrations (as indicated in the Figures) before argon purging. The mixtures were gently stirred for 5–10 min to ensure thorough integration. Glycerol is an additional carbon source, potentially increasing intracellular glycogen levels, and provides reducing equivalents and ATP necessary for nitrogenase activity (Bandyopadhyay et al., 2010).
Gas samples were collected from the headspace of each culture vessel every 24 h using gas-tight syringes. Hydrogen concentrations were analyzed using a Tsvet-800 gas chromatograph (Russia) equipped with a thermal conductivity detector and a packed column filled with molecular sieve 5A (0.2–0.3 mesh). The column temperature was maintained at 30°C during measurements. High-purity argon (99.999%) was used as the carrier gas at a 30 mL/min flow rate. Hydrogen production rates were normalized to the chlorophyll content of the cultures and expressed as micromoles of H2 per milligram of chlorophyll per hour (µmol H2/mg Chl a/h).
The rate of oxygen evolution was determined using a Clark-type oxygen electrode integrated into the Oxytherm System (Hansatech Instruments, UK). This system facilitates precise measurement of dissolved oxygen in liquid-phase samples, making it suitable for assessing photosynthetic activity in cyanobacteria. Measurements were conducted at a constant temperature of 32°C, maintained by the system’s temperature control unit. Before each measurement, the electrode was calibrated using air-saturated water to establish 100% oxygen saturation and sodium dithionite-treated water to define the zero-oxygen baseline. Approximately 2 mL of cyanobacterial culture was introduced into the electrode chamber, and oxygen evolution was recorded under continuous illumination at 100 µmol photons/m2/s. Data acquisition and analysis were performed using OriginPro 9.1 software (OriginLab Corporation, United States), which offers advanced data analysis and graphing tools.
All experiments were conducted in triplicate to ensure data reliability and reproducibility. Results are presented as mean values accompanied by their standard deviations. Statistical analyses were performed using Microsoft Excel 2019 (Microsoft Corporation, United States). Comparative analyses between different experimental groups were conducted using a one-way analysis of variance (ANOVA), followed by Tukey’s post hoc test to identify significant differences. A p-value of less than 0.05 was considered statistically significant. By employing these methodologies, we obtained accurate and reproducible measurements of oxygen evolution and hydrogen production, providing a robust foundation for evaluating the biohydrogen production potential of the selected cyanobacterial strains.
All the protein sequences were obtained from the NCBI Protein database. Multiple sequence alignments were obtained using the NCBI COBALT MSA tool. UCSF Chimera was used for visualizing the protein sequences and rendering protein cartoon structures. For 16S rRNA analysis, full-length sequences were obtained from the GreenGenes database. These sequences were aligned and curated using BGME. The obtained sequences were then used to generate a distance matrix using FastME and visualized using iTOL.
2,5-Dibromo-3-methyl-6-isopropyl-p-benzoquinone (DBMIB) is a well-characterized photosynthesis inhibitor (Trebst, 2007) that impedes electron transfer between cytochrome b6/f complex and plastoquinone, effectively blocking electron flow to Photosystem I (PSI) (Cournac et al., 2004; Metz et al., 1986; Skizim et al., 2012). This inhibition is crucial for maintaining anaerobic conditions necessary for activating hydrogen-metabolizing enzymes, which are highly sensitive to oxygen (Hallenbeck, 2012). In contrast, 3-(3,4-dichlorophenyl)-1,1-dimethylurea (DCMU) inhibits electron transfer between the primary and secondary quinone acceptors (Qa and Qβ) on the acceptor side of Photosystem II (PSII) (Pansook et al., 2019; Metz et al., 1986).
Previous studies have demonstrated the impact of DCMU on hydrogen production in cyanobacterial strains, including Sodalinema gerasimenkoae IPPAS B-353, Dolichospermum sp. IPPAS B-1213, Cyanobacterium sp. IPPAS B-1200, and Synechocystis sp. PCC 6803 GT 926) (Bozieva et al., 2023). In this study, we investigated the effect of DBMIB on hydrogen production rates under artificial lighting conditions.
Figure 2A illustrates the influence of two concentrations of DBMIB (30 μM and 110 µM) on the hydrogen production rates of the studied strains. Under these conditions, detectable hydrogen production was observed in Dolichospermum sp. and Synechocystis sp. In the control strain Synechocystis sp., the addition of DBMIB resulted in a modest increase in hydrogen production, from 0.14 µmol H2/mg Chl a/h to 1.34 µmol H2/mg Chl a/h and 1.72 µmol H2/mg Chl a/h with 30 μM and 110 µM DBMIB, respectively. However, these values remained lower than the hydrogen production rate of Dolichospermum sp. (5.88 µmol H2/mg Chl a/h) in the absence of the inhibitor. Notably, Sodalinema gerasimenkoaeand Cyanobacterium sp. did not produce hydrogen under light conditions, regardless of DBMIB presence.
Figure 2. (A) The effect of DBMIB on light-induced hydrogen release in studied cyanobacterial strains. This figure presents the influence of DBMIB, an inhibitor of the plastoquinone pool, on hydrogen release rates under light conditions, highlighting its impact on the photosynthetic electron transport chain across different strains. (B) The effect of glycerol on hydrogen release rates in cyanobacteria. This figure demonstrates how varying glycerol concentrations as an exogenous carbon source influence the hydrogen production rate in cyanobacterial cultures.
In this study, we employed a modified methodology compared to previous work (Bozieva et al., 2023). Specifically, cultures were purged with pure argon for 30 min, effectively replacing the gas phase components with argon and creating an anaerobic atmosphere. Consequently, chromatographic analyses detected no peaks for oxygen or nitrogen, confirming the establishment of anaerobic conditions essential for accurate assessment of hydrogen production.
Cyanobacteria are a morphologically diverse group that includes both unicellular and filamentous forms, with some species containing specialized cells known as heterocysts (Singh and Montgomery, 2011). Heterocysts are differentiated cells that enable nitrogen fixation, allowing cyanobacteria to adapt to low-nitrogen environments and contribute to their overall survival (D.W. and P. S. L. a. W, 2014).
Heterocyst-containing cyanobacteria can produce hydrogen through two primary mechanisms: (1) the nitrogenase-catalyzed, ATP-dependent reduction of protons and (2) hydrogen production by Hox hydrogenase. Under aerobic conditions, hydrogen produced can be recycled by uptake hydrogenase enzymes. These enzymes facilitate the transfer of electrons from hydrogen to oxygen through the respiratory system in a reaction known as the oxyhydrogen or “Knallgas” reaction. Uptake hydrogenase comprises two subunits: the hupL-encoded protein, responsible for hydrogen consumption, and the hupS-encoded protein, which transfers electrons to an electron acceptor via iron-sulfur (FeS) clusters. Generally, hydrogen is re-oxidized in heterocysts, meaning that strains utilizing this mechanism do not release pure H2 under optimal conditions (Dutta et al., 2005; Tsygankov et al., 1998).
However, when nitrogen is limited in the medium and oxygen is absent from the gas phase, H2 production increases as a byproduct of nitrogen fixation. Under these anaerobic conditions, the hup-hydrogenase does not consume the produced hydrogen, leading to a net release of H2 (Tsygankov et al., 1998; Bothe et al., 1977; Tsygankov, 2007; Zhang et al., 1984).
Nitrogen fixation physiology varies across cyanobacterial types, particularly in managing oxygen produced during photosynthesis (Hallenbeck, 2012; Gallon, 1992). Unicellular cyanobacteria and filamentous cyanobacteria without heterocysts achieve this separation by conducting photosynthesis during daylight and maximizing nitrogenase activity at night. In contrast, heterocyst-forming species can simultaneously carry out oxygenic photosynthesis and nitrogen fixation (Equations 1, 2; Figure 3). This capability arises from their two distinct cell types: vegetative cells, which perform photosynthesis, release oxygen, and fix carbon, and heterocysts, which lack photosystem II and provide an oxygen-free environment for nitrogenase activity (Hallenbeck, 2012).
Figure 3. Spatial separation of oxygenic photosynthesis and nitrogen fixation in heterocystous cyanobacteria. This figure illustrates the distinct roles of vegetative cells and heterocysts, with vegetative cells performing oxygenic photosynthesis and heterocysts specialized in nitrogen fixation and hydrogen production. The metabolic interactions between these cell types are shown in the context of biophotolysis (Skizim et al., 2012).
Additionally, heterocysts possess multiple barriers to prevent oxygen penetration, thereby maintaining an oxygen-free environment essential for nitrogen fixation (Gallon, 1992). Under conditions where nitrogen levels in the medium are limited and oxygen is absent from the gas phase, nitrogen fixation proceeds with hydrogen released as a byproduct according to the following equation:
Without molecular nitrogen (N2) in the gas phase, nitrogenase acts as an ATP-dependent hydrogenase, reducing protons to produce H2 instead of ammonia (Skizim et al., 2012; Tsygankov, 2007; Bothe et al., 2010; McKinlay and Harwood, 2010; Seefeldt et al., 2012). This process is described by:
During experiments conducted on Dolichospermum sp., this form of hydrogen production was observed. The results of these experiments and previous findings indicate that using photosynthesis inhibitors may temporarily enhance hydrogen production efficiency. However, this effect is short-lived and does not support sustained H2 production.
Exploring exogenous carbon sources, such as glycerol, has shown promise in promoting cyanobacterial hydrogen production, as glycerol addition can stimulate light-dependent hydrogen release (Min and Sherman, 2010; Sadvakasova et al., 2020). This study added 225 ± 5 mM glycerol (Figure 2B) to cyanobacterial cultures prepared as described, followed by incubation under continuous illumination after a 30-min argon purge to create anaerobic conditions.
The addition of glycerol led to a significant increase in hydrogen production rates for both Dolichospermum sp. and Synechocystis sp. strains, achieving maximum rates of 19.94 and 2.24 µmol H2/mg Chl a/h, respectively. Remarkably, glycerol also triggered hydrogen generation in S. gerasimenkoae IPPAS B-353—a response not observed in previous light-only experiments. However, under dark conditions, no hydrogen production was detected in any strain, even in the presence of glycerol (data not shown), confirming the light dependency of this enhancement.
These findings encouraged a deeper investigation into the effect of heightened glycerol concentrations on hydrogen production rates. To further optimize, we tested 350 mM glycerol on the nitrogen-fixing strains S. gerasimenkoae and Dolichospermum sp. (Figure 4A).
Figure 4. (A) Hydrogen production performance of Dolichospermum sp. IPPAS B-1213. This figure presents data on the hydrogen production rates achieved by Dolichospermum sp. IPPAS B-1213 under specific cultivation conditions. (B) The impact of different glycerol concentrations on hydrogen release by Dolichospermum sp. IPPAS B-1213. This figure explores how varying glycerol concentrations affect the hydrogen production rate in Dolichospermum sp., highlighting the optimal concentration range for enhanced biohydrogen output.
While S. gerasimenkoae showed minimal change in hydrogen production rates, Dolichospermum sp. exhibited a strong positive correlation between glycerol concentration and hydrogen output up to 350 mM. After 9 days (216 h) of incubation, Dolichospermum sp. reached a maximum production rate of 132.3 µmol H2/mg Chl a/h—a 30-fold increase over the highest rate previously achieved with 20 µM DCMU (4.24 µmol H2/mg Chl a/h) (Bozieva et al., 2023). Furthermore, on the 11th day of incubation under light and anaerobic conditions with 350 mM glycerol, the H2 concentration in the gas phase reached an impressive 67% (Table 1).
However, increasing glycerol concentration beyond 350 mM (up to 1,500 mM) provided no additional benefits and ultimately proved inhibitory, as shown by a loss of pigmentation associated with healthy cyanobacterial activity (Figure 4B). This suggests that while glycerol is beneficial within an optimal range, excessive concentrations may induce metabolic stress or osmotic imbalance in the cells.
The optimal glycerol concentration range for promoting hydrogen production in Dolichospermum sp. was determined to be between 200 and 400 mM, with this range yielding sustained hydrogen release. Remarkably, under these conditions, Dolichospermum sp. sustained hydrogen production for up to 46 days, while S. gerasimenkoae produced hydrogen continuously for up to 24 days (Table 1).
These results underscore glycerol’s potential as a metabolic enhancer for long-term hydrogen production in cyanobacteria, particularly in Dolichospermum sp. This sustained hydrogen release highlights glycerol’s utility as a cost-effective, stable carbon source, which could be further optimized for renewable biohydrogen production applications.
These findings highlight a notable feature of certain cyanobacteria: their capacity to utilize organic molecules such as glycerol as sources of energy, carbon, and electrons, which provides critical metabolic flexibility (Stebegg et al., 2023). During hydrogen production, the addition of glycerol introduces a supplementary pool of reducing equivalents, creating a more reductive redox environment within the cells. This shift promotes an increase in nitrogenase activity, likely to fulfill the heightened nitrogen demands of the cyanobacteria, which is essential for sustaining growth and hydrogen production (Bandyopadhyay et al., 2010; Berman-Frank et al., 2001).
In this study, two lighting protocols—continuous light (LL) and continuous dark (DD)—were employed, though the potential influence of a light-dark (LD) cycle remains unexplored. Glycerol, a carbon and electron source, enables cyanobacteria to shift into a photoheterotrophic mode of metabolism, effectively combining photosynthesis with organic carbon utilization. Under continuous illumination and optimized glycerol concentrations (200–400 mM), Dolichospermum sp. achieved peak hydrogen production rates, underscoring the potential of glycerol to enhance biohydrogen yields under controlled conditions.
From a sustainability perspective, glycerol represents an economically viable and renewable carbon source, especially given its widespread availability as a byproduct of the biodiesel industry (Zhang et al., 2021). While purified glycerol was used in this study, crude glycerol—an often-low-cost byproduct—also holds promise as a feedstock for hydrogen production. Using crude glycerol aligns with circular economy principles, as it repurposes waste from biodiesel production and reduces reliance on synthetic carbon sources. Previous studies with other phototrophic microorganisms have successfully utilized crude glycerol, demonstrating its feasibility as a raw material (Sabourin-Provost and Hallenbeck, 2009).
Integrating waste-derived glycerol into cyanobacterial hydrogen production supports renewable energy generation and offers a scalable, cost-effective approach for biohydrogen production. This sustainable method leverages a byproduct of existing biofuel processes, contributing to a closed-loop system where biodiesel waste is converted into valuable hydrogen fuel. Optimizing such systems could further establish cyanobacteria as efficient platforms for sustainable biohydrogen production, positioning them as key players in the transition toward low-carbon energy solutions.
This study also investigated whether the continuous release of hydrogen impacts the normal physiological functions of Dolichospermum sp. IPPAS B-1213. Specifically, we examined the culture’s ability to return to typical metabolic activity after hydrogen production. For this, Dolichospermum sp., actively releasing hydrogen for 18 days, was transferred into fresh BG-110 medium, a nitrogen-free medium commonly used to induce nitrogen fixation. After 3 days of cultivation in this fresh medium, the culture’s rate of oxygen release was measured as an indicator of its photosynthetic health and metabolic recovery.
The results revealed that the culture continued to grow and photosynthesize effectively, even as it transitioned back to non-hydrogen-producing conditions. This suggests that the hydrogen production process does not induce irreversible alterations in cellular metabolism and has only a limited, mild effect on the physiological state of the culture. The measured oxygen release rate for the hydrogen-producing culture was 141.2 μmol O2/mg Chl a/h, which, while somewhat lower than the control culture’s rate of 235.7 μmol O2/mg Chl a/h, still indicates substantial metabolic activity.
These findings highlight the resilience of Dolichospermum sp., as it can endure periods of active hydrogen production without significant compromise to its ability to perform photosynthesis. The temporary reduction in oxygen evolution suggests a mild but reversible metabolic adjustment rather than permanent damage. Thus, hydrogen production in Dolichospermum sp. can be managed without severely impacting the culture’s health, making this strain a viable candidate for sustainable biohydrogen production applications.
In conclusion, Dolichospermum sp. demonstrates robust metabolic adaptability, sustaining essential physiological functions throughout and beyond active hydrogen production. This resilience supports the potential for integrating hydrogen production into cyanobacterial cultivation systems on a broader scale. By enabling hydrogen generation without long-term harm to the culture, this approach can contribute to biohydrogen production strategies that maintain culture viability over extended periods, enhancing the feasibility of cyanobacteria-based renewable energy solutions.
Glycerol has been shown to be a possible source of carbon energy in several organisms (Duskova et al., 2015; Fu et al., 2000; Yilmaz et al., 2020; Zardoya et al., 2002). It has also been implicated in cell redox balance, osmoprotection, and antifreeze metabolite due to its colligative properties. Similarly, cyanobacteria have also acquired/evolved these glycerol transport mechanisms as being exclusively phototrophic will make survival in complete darkness difficult. Even those that primarily engage in heterotrophic metabolism often require low-intensity light, likely to activate photoreceptor-mediated development or shifts in gene expression. However, the adaptive evolution of these facilitators is not completely understood. Expanding the heterotrophic capabilities of cyanobacteria to include substrates like glycerol offers promising potential for both basic research and industrial applications. From a renewable energy perspective, glycerol is an attractive carbon source due to its abundance as a byproduct of biodiesel production (Goyal et al., 2021) and other industrial processes (Husna et al., 2024; Pacheco et al., 2022). Enabling cyanobacteria to metabolize glycerol could enhance their utility in biofuel production, bioremediation, and other biotechnological applications. However, the mechanisms and regulation of glycerol metabolism in cyanobacteria remain underexplored.
While many microorganisms, such as bacteria (Poladyan et al., 2019; Strittmatter et al., 2022; Gonzalez et al., 2023), single-celled fungi (Kuttiraja et al., 2015; Gao et al., 2024), and eukaryotic algae (Bolatkhan et al., 2019; Parmar et al., 2011; Salleh et al., 2016; A.A. and T, 2007; Adam et al., 2020; Elam et al., 2003; Sakurai et al., 2013; A.A. et al., 2019; Yeager et al., 2011), naturally metabolize or can be engineered to metabolize glycerol, there has been limited research on the capability of cyanobacteria to utilize smaller carbon substrates like glycerol and acetate (Ingram et al., 1973; Van Baalen et al., 1971; Feng et al., 2010; Das et al., 2021). Glycerol, a three-carbon molecule, feeds directly into central metabolic pathways, including glycolysis and the citric acid cycle, making it a valuable substrate for microbial growth.
Some cyanobacterial strains have shown potential for glycerol metabolism under specific conditions. For instance, Leptolyngbya subtilis can grow on 5% glycerol and produce nearly five times more lipids than under photoautotrophic conditions (Das et al., 2021). Additionally, Cyanothece species, one of the few capable of heterotrophic growth on glycerol in darkness (Min and Sherman, 2010; Feng et al., 2010), has demonstrated high hydrogen production under aerobic conditions when using glycerol as a substrate (Bandyopadhyay et al., 2010). In Cyanothece sp. ATCC 51142, hydrogen production occurs primarily under diazotrophic conditions, with nitrogen addition to the growth medium sharply reducing nitrogen fixation and hydrogen production. This suggests that hydrogen production is mediated by the nitrogenase enzyme system rather than the bidirectional hydrogenase (Hox) system. By comparison, Anabaena 29413 and Synechocystis 6803 have shown limited hydrogen production—approximately 40 and 1 mmol per mg of chlorophyll per hour, respectively—only under anaerobic conditions, with no production observed under aerobic conditions. Before further exploring the distribution of enzymes involved in glycerol metabolism, we constructed a 16S rRNA sequence phylogeny to identify the close relatives of the Dolichospermum type species (holotype), Dolichospermum flos-aquae (Figure 5A). As reported, Dolichospermum is a recently classified genus, and several Nostocaceae members have been proposed to be reclassified into this genus (Li et al., 2016).
Figure 5. Glycerol metabolism in Dolichospermum sp. and the diversity in the metabolic pathways. (A) 16S rRNA phylogenetic analysis of Dolichospermum holotype, Nostocaceae neighbors, and other cyanobacterial species. (B) Sequence alignment showing conserved motifs lining the pore in photosynthetic and non-photosynthetic aquaporins, glyceroporins, and related facilitator proteins. (C) Cytoplasmic face of AqpZ, aquaglyceroporin with the pore highlighted by a red dotted circle(top) and a protein slice showing the motifs lining the pore (bottom). Motif color coding follows from panel (B). (D) The broad range of organisms harboring GlpF proteins and the diverse metabolic pathways constituted by a variety of proteins. The filled and open squares indicate the presence and absence of the genes. “*” means the gene is present in closely related species. (E) Schematic of vegetative and heterocyst cells with uptake of Glycerol via the GlpF, phosphorylation with GK, production of GG in veg. cells and transport into heterocysts via the plasmodesmata-like connections, and used to drive H2 production via the HOX hydrogenase or via the Nitrogenase (not shown), and diffusion into the media as a non-polar gas.
Cyanobacteria are primarily known for their ability to perform oxygenic photosynthesis. However, certain strains can also grow heterotrophically by utilizing external organic carbon sources—for example, Synechocystis sp. PCC 6803 can grow in the dark with glucose, known as light-activated heterotrophic growth. This process requires minimal light exposure to regulate gene expression necessary for glucose uptake and metabolism. Advancements in genomic sequencing have identified various sugar transporters in cyanobacteria, including those for hexoses and pentoses. This knowledge allows genetic engineering to introduce or enhance glycerol uptake and utilization pathways. The availability and compilation of this information will inform the potential advancements in the expression of heterologous genes encoding glycerol transporters and key metabolic enzymes, it may also be possible to increase the cyanobacterial glycerol metabolism efficiency. To this end, we performed an extensive search to identify glycerol facilitators, GlpF, in various cyanobacteria (Figure 5B). GlpF is a member of the major intrinsic protein (MIP) family and facilitates the passive diffusion of glycerol (Fu et al., 2000). These genomic insights provide a glimpse at the possibility of transformation and gene-editing techniques to allow for the expression of novel transporters, potentially enabling cyanobacteria to uptake and utilize additional carbon sources. Such modifications could improve growth rates and biomass production under heterotrophic conditions.
Cyanobacteria possess specialized systems for the cellular uptake of glycerol. This uptake is mediated by the transport proteins embedded in the plasma membrane. The key proteins responsible for glycerol uptake include major intrinsic protein (MIP). These membrane-embedded proteins facilitate diffusion across the concentration gradient through GlpF channels. It has also been shown that aquaglyceroporins (AqpZ), a subclass of aquaporins, facilitate the transport of water, glycerol, and other uncharged molecules across the membrane. These channel proteins have specific motifs that line the pore, and the residues in these motifs dictate the molecule that passes through the pore (Figure 5C). Once inside the cell, glycerol undergoes a cascade of metabolic reactions that feed into cell cycles. Glycerol kinase, GlpK (Figure 5E), phosphorylates glycerol to form glycerol-3-phosphate (G3P); this reaction is essential for integrating glycerol into the glycolytic pathway. The presence of GlpK has been shown in several cyanobacterial cell types that can utilize glycerol as an energy source, including cyanobacteria. Once inducted into the glycolytic pathway, several enzymes come into play to process or transfer G3P to heterocyst. The presence of these enzymes is widely distributed (Figure 5D), and they are involved in the G3P downstream processing.
Some of the proteins involved in analogous molecule catabolism are glucosylglycerol phosphate synthase (GGPS) and glucosylglycerol phosphate phosphatase (GGPP). Both GGPS and GGPP play a role in GG biosynthesis. GGPS catalyzes the reaction, converting ADP-Glucose and G3P to GG-3-P phosphate and ADP. Then, GGPP, a phosphatase, hydrolyzes GG-3-P to generate GG. In several organisms, these enzymes are present separately and encoded by two different genes, but there have been reports of fusion proteins called GGPPS. GGPPS is a bifunctional enzyme, where GGPP is present at the N-terminal and GGPS is at the C-terminal, suggesting a novel regulatory pathway (Cheng et al., 2024; Hagemann et al., 2008). Glucosylgycerol hydrolase (GGHA) is involved in the hydrolysis of GG to generate glucose and glycerol. We show that GGPP and GGHA are present in the organisms and possibly point towards a synergistic regulation of GG anabolism and catabolism. However, the homologs of GGHA are absent in the organisms synthesizing GGPPS fusion protein. Recently, it was shown that another protein, glycoside hydrolase (GGP), is present in a few GGPPS-expressing organisms to mediate GG catabolism in the absence of GGHA. Two more proteins, glucosyl-3-phosphoglycerate synthase (GPGS) and GGA phosphorylase (GGAP) (not shown in Figure 5E), are involved in GGA synthesis and degradation in non-phototrophic bacteria (Cheng et al., 2024).
Organic compounds, such as glycerol or other carbon intermediates, can be processed in heterocysts to generate both fixed nitrogen via nitrogenase and molecular hydrogen via hydrogenase. Heterocysts are specialized, terminally differentiated cells that create a microoxic environment, which is essential for the activity of oxygen-sensitive enzymes such as nitrogenase and hydrogenase. These structures serve as metabolic hubs, where the division of labor between vegetative cells and heterocysts ensures efficient resource utilization and metabolic specialization.
In this system (Figure 5E), organic compounds transported from vegetative cells through plasmodesmata-like structures act as key substrates for heterocyst metabolism. For nitrogen fixation, the nitrogenase enzyme reduces atmospheric dinitrogen (N2) to ammonia (NH3), a process that requires significant energy input in the form of ATP and reducing power (electrons). These electrons can be derived from organic carbon metabolism, where glycerol and other compounds are donors for producing NAD(P)H. Electrons can be supplied through cyclic electron flow involving Photosystem I (PSI) and ferredoxin. In this pathway, ferredoxin acts as an electron carrier, transferring electrons back to PSI, where they are recycled to generate additional reducing power without producing oxygen. This cyclic electron flow is significant in heterocysts, where oxygenic photosynthesis is absent to maintain the microoxic environment required for nitrogenase and hydrogenase activity.
Simultaneously, hydrogenase in heterocysts catalyzes the evolution of molecular hydrogen (H2) as a byproduct or alternative outlet for the reducing equivalents generated during nitrogen fixation. This dual activity allows the heterocysts to manage excess reducing power, which could otherwise lead to cellular stress or inhibit nitrogenase function. Hydrogenase can also recycle the hydrogen produced, further enhancing the energy efficiency of the system.
The coordinated transport and metabolism of organic carbon compounds, combined with cyclic electron flow through PSI and ferredoxin, reflect a highly integrated strategy in cyanobacteria like Dolichospermum sp. and Anabaena. This process not only supports nitrogen fixation under nitrogen-limiting conditions but also provides an efficient means of hydrogen production, a process of significant interest for sustainable bioenergy applications. By sequestering both nitrogenase and hydrogenase within the oxygen-protected environment of heterocysts, cyanobacteria circumvent oxygen inhibition, thereby maximizing the efficiency of both nitrogen and hydrogen production.
This remarkable metabolic flexibility highlights the evolutionary adaptations of heterocystous cyanobacteria, enabling them to thrive in diverse environments while simultaneously contributing to carbon, nitrogen, and energy cycling. This coordination between glycerol metabolism, intercellular transport, and hydrogenase function underscores the metabolic integration in Dolichospermum sp. and related species. It highlights how plasmodesmata-like structures facilitate the division of labor between vegetative and heterocyst cells, enabling efficient resource allocation for biohydrogen production under specific environmental conditions. This structural and metabolic organization provides a robust model for exploring the interplay of carbon metabolism and hydrogen production in cyanobacteria.
Harnessing glycerol as a carbon source for cyanobacterial growth presents transformative opportunities for both industrial biotechnology and environmental sustainability. The biodiesel industry generates vast quantities of glycerol as a byproduct, often leading to costly disposal challenges and environmental concerns. Repurposing this surplus glycerol as a feedstock for cyanobacterial cultivation not only reduces waste but also provides a low-cost, renewable substrate for producing high-value bioproducts, including biofuels, bioplastics, and specialty chemicals.
Beyond industrial applications, engineered cyanobacteria capable of metabolizing glycerol offer promising avenues for bioremediation, enabling the degradation of glycerol-contaminated waste streams from various sectors. Advancing these technologies requires an interdisciplinary approach integrating systems biology, metabolic engineering, and synthetic biology to optimize glycerol utilization pathways, enhance metabolic flux, and improve overall efficiency. Unlocking these capabilities could significantly accelerate the development of cyanobacteria as a scalable, sustainable platform for hydrogen production and other bio-based innovations, bridging the gap between waste management and renewable energy solutions.
This study is the first to demonstrate long-term hydrogen production by the heterocystous cyanobacterial strain Dolichospermum sp. IPPAS B-1213 uses glycerol as an exogenous carbon source. The hydrogen production rate achieved with glycerol supplementation reached 132.3 µmol H2/mg Chl a/h—a 30-fold increase over rates achieved with conventional inhibitors. An optimal glycerol concentration range (200–400 mM) was identified for maximizing hydrogen output, providing a basis for fine-tuning biohydrogen production in cyanobacterial systems.
Several inherent traits of Dolichospermum sp. likely contribute to its robust hydrogen production capabilities. The strain’s metabolic flexibility allows it to utilize various organic substrates, such as glycerol, sustaining energy production and cellular growth during extended periods of hydrogen release. Its nitrogen fixation capability through specialized heterocysts further supports survival and metabolic activity in nitrogen-depleted environments, enabling continuous hydrogen production even under nutrient-limited conditions. Additionally, Dolichospermum sp. benefits from efficient energy transfer systems, including uptake hydrogenases that recycle hydrogen, enhancing energy use and supporting stable hydrogen output. The strain’s resilience to environmental stressors, such as variations in nutrient availability and light intensity, underscores its adaptability and suitability for diverse cultivation setups.
This research demonstrates that glycerol, a renewable and cost-effective byproduct of the biodiesel industry, can effectively stimulate sustained hydrogen production in cyanobacteria, highlighting its potential as a scalable substrate for biohydrogen production. Future studies could explore the comparative effects of various exogenous carbon sources across different nitrogen-fixing cyanobacterial strains to identify optimal substrates and environmental conditions for maximizing hydrogen yields.
The robust performance of Dolichospermum sp. in photosynthetic hydrogen production opens the door to further innovations in renewable energy. By harnessing the metabolic versatility of cyanobacteria, there are opportunities to develop low-cost, high-efficiency systems for biohydrogen production. Such systems could operate with minimal input in nutrient-depleted environments, making them suitable for various applications, from waste treatment facilities to off-grid renewable energy setups. Additionally, exploring other resilient strains with similar physiological traits could yield cyanobacterial systems capable of operating under industrially relevant conditions where consistent hydrogen production is required over extended periods. Optimizing these systems to use waste-derived substrates like crude glycerol would further enhance sustainability, contributing to a circular economy model and reducing production costs.
Continued research in this area could develop bioreactor systems designed explicitly for cyanobacteria, integrating efficient light capture, nutrient recycling, and controlled gas exchange. Such advancements could significantly boost the feasibility and scalability of cyanobacteria-based hydrogen production, positioning these systems as a valuable component of future renewable energy portfolios.
ll of the sequences analyzed in Figure 5 are derived from open sources such as NCBI protein and the GreenGenes2 rRNA database (http://ftp.microbio.me/greengenes_release/.) and (https://rnacentral.org/search?q=Greengenes).
AB: Methodology, Writing – original draft, Formal Analysis, Investigation. MK: Investigation, Methodology, Writing – original draft, Formal Analysis. MR: Investigation, Methodology, Writing – original draft, Data curation, Visualization, Writing – review and editing. MS: Formal Analysis, Investigation, Methodology, Writing – original draft. RV: Formal Analysis, Investigation, Methodology, Writing – original draft, Visualization. DD: Formal Analysis, Investigation, Methodology, Writing – original draft. AT: Formal Analysis, Investigation, Methodology, Writing – original draft. YL: Formal Analysis, Investigation, Methodology, Writing – original draft. J-SC: Investigation, Methodology, Writing – original draft, Visualization. SA: Writing – original draft, Conceptualization, Funding acquisition, Project administration, Supervision, Writing – review and editing. BB: Conceptualization, Funding acquisition, Project administration, Supervision, Writing – original draft, Writing – review and editing, Methodology, Visualization.
The author(s) declare that financial support was received for the research and/or publication of this article. This work was supported by the joint RSF-MOST grant (22–44-08001, awarded to JS and SA). Specifically, Figure 3 was funded by RSF grant No. 22-19-00516. Figure 4 was made possible through the state contract with the Ministry of Science and Higher Education of the Russian Federation (Project No. 122050400128-1). AT and MK received support from the Ministry of Science and Higher Education of the Russian Federation (Project No. 122041200039-0) and the Shared Core Facilities of the Pushchino Scientific Center for Biological Research. The authors also acknowledge financial support from Taiwan’s National Science and Technology Council under grant numbers 113-2321-B-029-002, 113-2221-E-029-032-MY3, 113-2811-E-006-015-MY3, 112-2221-E-006-194-MY3, and 112-2811-E-029-004-MY3. Additional support was provided through the Charles P. Postelle Distinguished Professorship of Biotechnology and the NSF Grant IOS-2233695 awarded to BB.
The authors declare that the research was conducted in the absence of any commercial or financial relationships that could be construed as a potential conflict of interest.
The author(s) declare that no Generative AI was used in the creation of this manuscript.
All claims expressed in this article are solely those of the authors and do not necessarily represent those of their affiliated organizations, or those of the publisher, the editors and the reviewers. Any product that may be evaluated in this article, or claim that may be made by its manufacturer, is not guaranteed or endorsed by the publisher.
The Supplementary Material for this article can be found online at: https://www.frontiersin.org/articles/10.3389/fenrg.2025.1547215/full#supplementary-material
A.A., S. H. a. T (2019). Second and third generation of feedstocks. Editors A Basile, and F. Dalena (Elsevier Inc.)
A.A., T. Biological generation of hydrogen. Russ. J. Gen. Chem. 77, 685–693. (2007). doi:10.1134/S1070363207040317
Adam, P., H., F., Bussche, Ch., Engelshove, S., and Thiemann, T. (2020). “Hydrogen infrastructure – the pillar of energy transition,” in The practical conversion of long-distance gas networks to hydrogen operation, 32. Whitepaper.
Allahverdiyeva, Y., and Kosourov, S. N. (2014). Bioenergy research: advances and applications. Editors Tuohy, M.G., Gupta, V.K., Kubicek, C.P, and Saddler, J.
Allahverdiyeva, Y., Leino, H., Saari, L., Fewer, D. P., Shunmugam, S., Sivonen, K., et al. (2010). Screening for biohydrogen production by cyanobacteria isolated from the Baltic Sea and Finnish lakes. Int. J. Hydrogen Energ 35, 1117–1127. doi:10.1016/j.ijhydene.2009.12.030
Ananyev, G., Carrieri, D., and Dismukes, G. C. (2008). Optimization of metabolic capacity and flux through environmental cues to maximize hydrogen production by the cyanobacterium Arthrospira (Spirulina) maxima. Appl. Environ. Microbiol. 74, 6102–6113. doi:10.1128/AEM.01078-08
Bandyopadhyay, A., Stockel, J., Min, H., Sherman, L. A., and Pakrasi, H. B. (2010). High rates of photobiological H2 production by a cyanobacterium under aerobic conditions. Nat. Commun. 1, 139. doi:10.1038/ncomms1139
Berman-Frank, I., Lundgren, P., Chen, Y. B., Küpper, H., Kolber, Z., Bergman, B., et al. (2001). Segregation of nitrogen fixation and oxygenic photosynthesis in the marine cyanobacterium Trichodesmium. Science 294, 1534–1537. doi:10.1126/science.1064082
Bolatkhan, K., Kossalbayev, B. D., Zayadan, B. K., Tomo, T., Veziroglu, T. N., and Allakhverdiev, S. I. (2019). Hydrogen production from phototrophic microorganisms: Reality and perspectives. Int. J. Hydrogen Energ 44, 5799–5811. doi:10.1016/j.ijhydene.2019.01.092
Bothe, H., Schmitz, O., Yates, M. G., and Newton, W. E. (2010). Nitrogen fixation and hydrogen metabolism in cyanobacteria. Microbiol. Mol. Biol. Rev. 74, 529–551. doi:10.1128/MMBR.00033-10
Bothe, H., Tennigkeit, J., and Eisbrenner, G. (1977). The utilization of molecular hydrogen by the blue-green alga Anabaena cylindrica. Arch. Microbiol. 114, 43–49. doi:10.1007/BF00429628
Bozieva, A. M., Khasimov, M. K., Voloshin, R. A., Sinetova, M. A., Kupriyanova, E. V., Zharmukhamedov, S. K., et al. (2023). New cyanobacterial strains for biohydrogen production. Int. J. Hydrogen Energ 48, 7569–7581. doi:10.1016/j.ijhydene.2022.11.198
Cheng, L., Zhang, Z., Zhu, D., Luo, Q., and Lu, X. (2024). Glucosylglycerol phosphorylase, a potential novel pathway of microbial glucosylglycerol catabolism. Appl. Microbiol. Biotechnol. 108, 214. doi:10.1007/s00253-024-13035-3
Chongsuksantikul, A. A. K., Yoshikawa, S., and Ohtaguchi, K. (2014). Hydrogen production by anaerobic dark metabolism in Synechocystis sp. strain PCC 6803-GT: effect of monosaccharide in nitrate free solution. J. Biochem. Tech.
Cournac, L., Guedeney, G., Peltier, G., and Vignais, P. M. (2004). Sustained photoevolution of molecular hydrogen in a mutant of Synechocystis sp. strain PCC 6803 deficient in the type I NADPH-dehydrogenase complex. J. Bacteriol. 186, 1737–1746. doi:10.1128/JB.186.6.1737-1746.2003
Das, S., Nath, K., and Chowdhury, R. (2021). Comparative studies on biomass productivity and lipid content of a novel blue-green algae during autotrophic and heterotrophic growth. Environ. Sci. Pollut. Res. Int. 28, 12107–12118. doi:10.1007/s11356-020-09577-4
Dawson, R. M. C., E, D. C., Elliott, W. H., and Jones, K. M. (1986). Data for biochemical research. 3 edn. Clarendon Press.
Duskova, M., Borovikova, D., Herynkova, P., Rapoport, A., and Sychrova, H. (2015). The role of glycerol transporters in yeast cells in various physiological and stress conditions. Fems Microbiol. Lett. 362, 1–8. doi:10.1093/femsle/fnu041
Dutta, D., De, D., Chaudhuri, S., and Bhattacharya, S. K. (2005). Hydrogen production by cyanobacteria. Microb. Cell Fact. 4, 36. doi:10.1186/1475-2859-4-36
D.W., P. S. L. a. W (2014). Microbiology of Waterborne Diseases. Editors Yates, M.V., Percival, S.L., Williams, D.W., Chalmers, R.M, and Gray, N.F
Elam, C. C., et al. (2003). Realizing the hydrogen future:: the International Energy Agency's efforts to advance hydrogen energy technologies. Int. J. Hydrogen Energ 28, 601–607. doi:10.1016/S0360-3199(02)00147-7
Feng, X., Bandyopadhyay, A., Berla, B., Page, L., Wu, B., Pakrasi, H. B., et al. (2010). Mixotrophic and photoheterotrophic metabolism in Cyanothece sp. ATCC 51142 under continuous light. Microbiol. Read. 156, 2566–2574. doi:10.1099/mic.0.038232-0
Fu, D., Libson, A., Miercke, L. J. W., Weitzman, C., Nollert, P., Krucinski, J., et al. (2000). Structure of a glycerol-conducting channel and the basis for its selectivity. Science 290, 481–486. doi:10.1126/science.290.5491.481
Gallon, J. R. (1992). Reconciling the Incompatible - N-2 fixation and O-2. New Phytol. 122, 571–609. doi:10.1111/j.1469-8137.1992.tb00087.x
Gao, Y. L., Cournoyer, J., De, B. C., Wallace, C. L., Ulanov, A. V., La Frano, M. R., et al. (2024). Introducing carbon assimilation in yeasts using photosynthetic directed endosymbiosis. Nat. Commun. 15, 5947. doi:10.1038/s41467-024-49585-3
G., K (2013). Advances in utilizing cyanobacteria for hydrogen production. Adv. Microbiol. doi:10.4236/aim.2013.36A008
Gonzalez, E., Zuleta, C., Zamora, G., Maturana, N., Ponce, B., Rivero, M. V., et al. (2023). Production of poly (3-hydroxybutyrate) and extracellular polymeric substances from glycerol by the acidophile Acidiphilium cryptum. Extremophiles 27, 30. doi:10.1007/s00792-023-01313-3
Goyal, S., Hernández, N. B., and Cochran, E. W. (2021). An update on the future prospects of glycerol polymers. Polym. Int. 70, 911–917. doi:10.1002/pi.6209
Hagemann, M., Ribbeck-Busch, K., Klähn, S., Hasse, D., Steinbruch, R., and Berg, G. (2008). The plant-associated bacterium Stenotrophomonas rhizophila expresses a new enzyme for the synthesis of the compatible solute glucosylglycerol. J. Bacteriol. 190, 5898–5906. doi:10.1128/JB.00643-08
Hallenbeck, P. C. (2012). “Hydrogen production by cyanobacteria,” in Microbial technologies in advanced biofuels production, 15–28. doi:10.1007/978-1-4614-1208-3_2
Husna, M., Tabak, Y., and Yildiz, M. (2024). Glycerol as a feedstock for chemical synthesis. Chembioeng Rev. 11. doi:10.1002/cben.202400010
Ingram, L. O., Calder, J. A., Van Baalen, C., Plucker, F. E., and Parker, P. L. (1973). Role of reduced exogenous organic compounds in the physiology of the blue-green bacteria (algae): photoheterotrophic growth of a “heterotrophic” blue-green bacterium. J. Bacteriol. 114, 695–700. doi:10.1128/jb.114.2.695-700.1973
Kamshybayeva, G. K., Kossalbayev, B. D., Sadvakasova, A. K., Zayadan, B. K., Bozieva, A. M., Dunikov, D., et al. (2022). Strategies and economic feasibilities in cyanobacterial hydrogen production. Int. J. Hydrogen Energ 47, 29661–29684. doi:10.1016/j.ijhydene.2022.06.277
Khetkorn, W. K. N., Incharoensakdi, A., and Lindblad, P. (2013). Metabolic and genetic engineering of cyanobacteria for enhanced hydrogen production. Biofuels 4, 535–561. doi:10.4155/bfs.13.41
Kossalbayeu, B. D., Tomo, T., Zayadan, B. K., Sadvakasova, A. K., Bolatkhan, K., Alwasel, S., et al. (2020). Determination of the potential of cyanobacterial strains for hydrogen production. Int. J. Hydrogen Energ 45, 2627–2639. doi:10.1016/j.ijhydene.2019.11.164
Kuttiraja, M., Krishna, S., Dhouha, A., and Tyagi, R. D. (2015). A substrate-based approach for the selection of oil-bearing heterotrophs from nitrogen-deficient soil for lipid production. Appl. Biochem. Biotechnol. 175, 1926–1937. doi:10.1007/s12010-014-1378-0
Li, X., Dreher, T. W., and Li, R. (2016). An overview of diversity, occurrence, genetics and toxin production of bloom-forming Dolichospermum (Anabaena) species. Harmful Algae 54, 54–68. doi:10.1016/j.hal.2015.10.015
Mathews, J., and Wang, G. Y. (2009). Metabolic pathway engineering for enhanced biohydrogen production. Int. J. Hydrogen Energ 34, 7404–7416. doi:10.1016/j.ijhydene.2009.05.078
McKinlay, J. B., and Harwood, C. S. (2010). Photobiological production of hydrogen gas as a biofuel. Curr. Opin. Biotechnol. 21, 244–251. doi:10.1016/j.copbio.2010.02.012
Metz, J. G., Pakrasi, H. B., Seibert, M., and Arntzen, C. J. (1986). Evidence for a dual function of the Herbicide-Binding D1-protein in photosystem-Ii. Febs Lett. 205, 269–274. doi:10.1016/0014-5793(86)80911-5
Min, H., and Sherman, L. A. (2010). Hydrogen production by the unicellular, diazotrophic cyanobacterium Cyanothece sp. strain ATCC 51142 under conditions of continuous light. Appl. Environ. Microbiol. 76, 4293–4301. doi:10.1128/AEM.00146-10
Pacheco, J. R., Villardi, H. G. D., Cavalcante, R. M., and Young, A. F. (2022). Biodiesel production through non-conventional supercritical routes: process simulation and technical evaluation. Energ Convers. Manage 251, 114998. doi:10.1016/j.enconman.2021.114998
Pansook, S., Incharoensakdi, A., and Phunpruch, S. (2019). Effects of the photosystem II inhibitors CCCP and DCMU on hydrogen production by the unicellular Halotolerant cyanobacterium Aphanothece halophytica. ScientificWorldJournal 2019, 1–10. doi:10.1155/2019/1030236
Parmar, A., Singh, N. K., Pandey, A., Gnansounou, E., and Madamwar, D. (2011). RETRACTED: cyanobacteria and microalgae: a positive prospect for biofuels. Bioresour. Technol. 102, 10163–10172. doi:10.1016/j.biortech.2011.08.030
Poladyan, A., Blbulyan, S., Sahakyan, M., Lenz, O., and Trchounian, A. (2019). Growth of the facultative chemolithoautotroph Ralstonia eutropha on organic waste materials: growth characteristics, redox regulation and hydrogenase activity. Microb. Cell Fact. 18, 201. doi:10.1186/s12934-019-1251-5
Sabourin-Provost, G., and Hallenbeck, P. C. (2009). High yield conversion of a crude glycerol fraction from biodiesel production to hydrogen by photofermentation. Bioresour. Technol. 100, 3513–3517. doi:10.1016/j.biortech.2009.03.027
Sadvakasova, A. K., Kossalbayev, B. D., Zayadan, B. K., Bolatkhan, K., Alwasel, S., Najafpour, M. M., et al. (2020). Bioprocesses of hydrogen production by cyanobacteria cells and possible ways to increase their productivity. Renew. Sust. Energ Rev. 133, 110054. doi:10.1016/j.rser.2020.110054
Sakurai, H., Masukawa, H., Kitashima, M., and Inoue, K. (2013). Photobiological hydrogen production: Bioenergetics and challenges for its practical application. J. Photoch Photobio C 17, 1–25. doi:10.1016/j.jphotochemrev.2013.05.001
Salleh, S. F., Kamaruddin, A., Uzir, M. H., Karim, K. A., and Mohamed, A. R. (2016). Investigation of the links between heterocyst and biohydrogen production by diazotrophic cyanobacterium A. variabilis ATCC 29413. Arch. Microbiol. 198, 101–113. doi:10.1007/s00203-015-1164-6
Samylina, O. S., Sinetova, M. A., Kupriyanova, E. V., Starikov, A. Y., Sukhacheva, M. V., Dziuba, M. V., et al. (2021). Ecology and biogeography of the 'marine Geitlerinema' cluster and a description of Sodalinema orleanskyi sp. nov., Sodalinema gerasimenkoae sp. nov., Sodalinema stali sp. nov. and Baaleninema simplex gen. et sp. nov. (Oscillatoriales, Cyanobacteria). FEMS Microbiol. Ecol. 97, fiab104. doi:10.1093/femsec/fiab104
Sarsekeyeva, F., Zayadan, B. K., Usserbaeva, A., Bedbenov, V. S., Sinetova, M. A., and Los, D. A. (2015). Cyanofuels: biofuels from cyanobacteria. Reality and perspectives. Photosynth Res. 125, 329–340. doi:10.1007/s11120-015-0103-3
Seefeldt, L. C., Hoffman, B. M., and Dean, D. R. (2012). Electron transfer in nitrogenase catalysis. Curr. Opin. Chem. Biol. 16, 19–25. doi:10.1016/j.cbpa.2012.02.012
Sinetova, M. A., Sidorov, R. A., Starikov, A. Y., Voronkov, A. S., Medvedeva, A. S., Krivova, Z. V., et al. (2020). Assessment of the biotechnological potential of cyanobacterial and Microalgal strains from IPPAS culture collection. Appl. Biochem. Micro+ 56, 794–808. doi:10.1134/S0003683820070030
Singh, S. P., and Montgomery, B. L. (2011). Determining cell shape: adaptive regulation of cyanobacterial cellular differentiation and morphology. Trends Microbiol. 19, 278–285. doi:10.1016/j.tim.2011.03.001
Skizim, N. J., Ananyev, G. M., Krishnan, A., and Dismukes, G. C. (2012). Metabolic pathways for photobiological hydrogen production by nitrogenase- and hydrogenase-containing unicellular cyanobacteria Cyanothece. J. Biol. Chem. 287, 2777–2786. doi:10.1074/jbc.M111.302125
Stanier, R. Y., Kunisawa, R., Mandel, M., and Cohen-Bazire, G. (1971). Purification and properties of unicellular blue-green algae (order Chroococcales). Bacteriol. Rev. 35, 171–205. doi:10.1128/br.35.2.171-205.1971
Stebegg, R., Schmetterer, G., and Rompel, A. (2023). Heterotrophy among cyanobacteria. ACS Omega 8, 33098–33114. doi:10.1021/acsomega.3c02205
Strittmatter, C. S., Eggers, J., Biesgen, V., Pauels, I., Becker, F., and Steinbüchel, A. (2022). The reliance of glycerol utilization by Cupriavidus necator on CO(2) fixation and improved glycerol catabolism. Appl. Microbiol. Biotechnol. 106, 2541–2555. doi:10.1007/s00253-022-11842-0
Touloupakis, E., Rontogiannis, G., Silva Benavides, A. M., Cicchi, B., Ghanotakis, D. F., and Torzillo, G. (2016). Hydrogen production by immobilized Synechocystis sp. PCC 6803 sp PCC 6803. Int. J. Hydrogen Energ 41, 15181–15186. doi:10.1016/j.ijhydene.2016.07.075
Trebst, A. (2007). Inhibitors in the functional dissection of the photosynthetic electron transport system. Photosynth Res. 92, 217–224. doi:10.1007/s11120-007-9213-x
Tsygankov, A. A. (2007). Nitrogen-fixing cyanobacteria: producents of hydrogen. Prikl. Biokhim Mikrobiol. 43, 279–288.
Tsygankov, A. A., Serebryakova, L. T., Rao, K. K., and Hall, D. O. (1998). Acetylene reduction and hydrogen photoproduction by wild-type and mutant strains ofAnabaenaat different CO2and O2concentrations. Fems Microbiol. Lett. 167, 13–17. doi:10.1111/j.1574-6968.1998.tb13201.x
Userbaeva, A. Z. B., Sadvakasova, A., Sarsekeeva, F., and Talpakova, A. (2017). Comparative analysis of lipid extraction methods for biomass of the Cyanobacterium sp. IPPAS B-1200 strain – po-tential producer of biodiesel. Vestn. Seriya Biol.
Van Baalen, C., Hoare, D. S., and Brandt, E. (1971). Heterotrophic growth of blue-green algae in Dim light. J. Bacteriol. 105, 685–689. doi:10.1128/jb.105.3.685-689.1971
Yang, F., Hanna, M. A., and Sun, R. (2012). Value-added uses for crude glycerol--a byproduct of biodiesel production. Biotechnol. Biofuels 5, 13. doi:10.1186/1754-6834-5-13
Yeager, C. M., Milliken, C. E., Bagwell, C. E., Staples, L., Berseth, P. A., and Sessions, H. T. (2011). Evaluation of experimental conditions that influence hydrogen production among heterocystous Cyanobacteria. Int. J. Hydrogen Energ 36, 7487–7499. doi:10.1016/j.ijhydene.2011.03.078
Yilmaz, O., Chauvigné, F., Ferré, A., Nilsen, F., Fjelldal, P. G., Cerdà, J., et al. (2020). Unravelling the complex Duplication history of Deuterostome glycerol transporters. Cells 9, 1663. doi:10.3390/cells9071663
Zardoya, R., Ding, X., Kitagawa, Y., and Chrispeels, M. J. (2002). Origin of plant glycerol transporters by horizontal gene transfer and functional recruitment. Proc. Natl. Acad. Sci. U. S. A. 99, 14893–14896. doi:10.1073/pnas.192573799
Zavřel, T. S. M. A., and Červený, J. (2015). Measurement of сhlorophyll a and сarotenoids сoncentration in сyanobacteria. Bio-protocol. doi:10.21769/BioProtoc.1467
Zhang, B. Z. S.-H., Yao, R., Wu, Y.-H., and Qiu, J.-Sh. (2021). Progress and prospects of hydrogen production: opportunities and challenges. J. Electron Sci. Technol. 19, 100080. doi:10.1016/j.jnlest.2021.100080
Keywords: biohydrogen, hydrogenase, renewable energy, glycerol carbon source, sustainable hydrogen, Dolichospermum sp, GlpF, glycerol facilitator
Citation: Bozieva AM, Khasimov MK, Rao MS, Sinetova MA, Voloshin RA, Dunikov DO, Tsygankov AA, Leong YK, Chang J-S, Allakhverdiev SI and Bruce BD (2025) Optimizing cyanobacterial hydrogen production: metabolic and genetic strategies with glycerol supplementation. Front. Energy Res. 13:1547215. doi: 10.3389/fenrg.2025.1547215
Received: 17 December 2024; Accepted: 21 March 2025;
Published: 09 April 2025.
Edited by:
Ranjeet Kumar Mishra, Manipal Institute of Technology, IndiaReviewed by:
Harvey J. M. Hou, Alabama State University, United StatesCopyright © 2025 Bozieva, Khasimov, Rao, Sinetova, Voloshin, Dunikov, Tsygankov, Leong, Chang, Allakhverdiev and Bruce. This is an open-access article distributed under the terms of the Creative Commons Attribution License (CC BY). The use, distribution or reproduction in other forums is permitted, provided the original author(s) and the copyright owner(s) are credited and that the original publication in this journal is cited, in accordance with accepted academic practice. No use, distribution or reproduction is permitted which does not comply with these terms.
*Correspondence: Suleyman I. Allakhverdiev, c3VsZXltYW4uYWxsYWtodmVyZGlldkBnbWFpbC5jb20=; Barry D. Bruce, YmJydWNlQHV0ay5lZHU=
Disclaimer: All claims expressed in this article are solely those of the authors and do not necessarily represent those of their affiliated organizations, or those of the publisher, the editors and the reviewers. Any product that may be evaluated in this article or claim that may be made by its manufacturer is not guaranteed or endorsed by the publisher.
Research integrity at Frontiers
Learn more about the work of our research integrity team to safeguard the quality of each article we publish.