- 1China Three Gorges Co., Ltd., Wuhan, China
- 2Faculty of Maritime and Transportation, Ningbo University, Ningbo, China
- 3Shipbuilding Information Center of China, Beijing, China
Offshore wind energy is characterized by its clean and renewable nature, leading to rapid growth in the industry. However, the incidence of ship collisions with offshore wind turbines has also risen with the increasing number of offshore wind farms, particularly in commercial shipping lanes. To gain a thorough understanding of dynamic response between wind turbines and vessels, this paper extensively reviews studies related to wind turbine–ship collisions over the past 2 decades to cover four key aspects: (i) the fundamental requirements and background of collision analysis study, (ii) the analysis of dynamic response and collision characteristics of the fixed-bottom and floating offshore wind turbines (OWTs) subjected to ship collision forces, (iii) the influence of key collision factors that include impact positions, initial ship kinetic energy, and soil–structure interaction on the structural response for the wind turbines, and (iv) a discussion of protection measures to mitigate the collision damage to the substructure. The limitations in the existing studies are discussed, and future research directions are suggested.
1 Introduction
As traditional resources like coal and carbon diminish, renewable energy resources such as wave, tidal, and wind energy have become more popular due to the abundant reserves and the advantage of being pollution-free. Among them, offshore wind energy has garnered widespread global attention due to its minimal land use, cleanliness, renewability, and the stable availability of wind resources since the 20th century. The wind industry has rapidly developed, achieving a mature industry scale and advanced technological foundation (Li et al., 2020). As depicted in Figure 1, the additional capacity installed in 2023 was approximately 117 GW, according to the “Global Wind Report 2024” presented by GWEC, representing 11% of the total installed capacity of wind turbines (Liu et al., 2024).
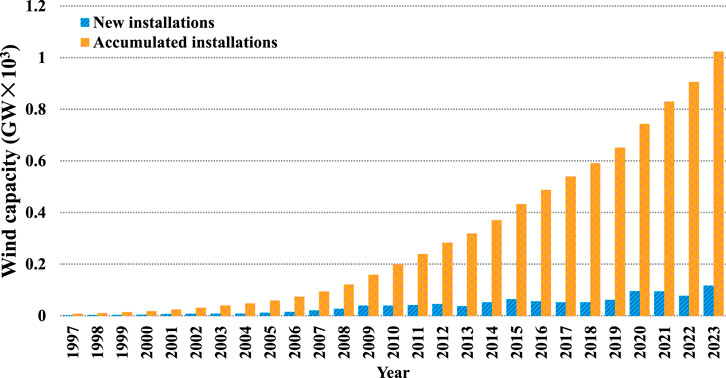
Figure 1. New and accumulated installations of global wind capacity from 1997 to 2023 reported by GWEC 2024.
Currently, the main types of fixed offshore wind power foundations are gravity base, monopile, high-pile cap, jacket, tripod, and suction bucket foundations (Guo Y. et al., 2022). The gravity base, monopile, tripod, and jacket foundation have been widely and extensively used in engineering applications, as shown in Figure 2. Each form has its benefits and drawbacks. Specifically, the monopile foundation has strong construction supporting capability, a simple fabrication process, and low costs. It is commonly found in shallow water. The gravity base has better stability and easy construction, with high geological command. The jacket foundation has stability in complicated wind and wave conditions; however, it has higher establishment and maintenance costs. The tripod has a large bearing capacity and is suitable in deep-sea areas but requires complicated and high-cost construction.
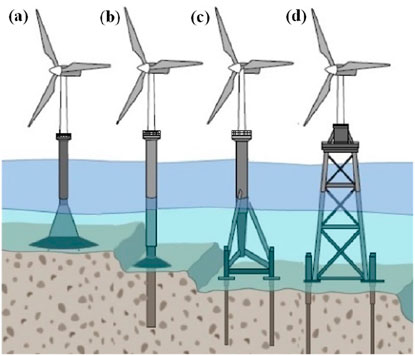
Figure 2. Most popular foundation types of the fixed-bottom OWTs: (A) gravity base, (B) monopile, (C) tripod, and (D) jacket.
However, because fixed-bottom OWTs are commonly used in nearshore areas, the trend for future wind farm development is to move “from shallow to deep” and “from nearshore to offshore.” This trend will lead to increasing installation and construction costs as water depth increases. Therefore, installing wind turbines on floating platforms is an effective and economical solution to enhance the economic benefits of wind turbines in deep-sea areas. At present, common floating wind platforms include spar, tension leg, semi-submersible, and barge-type foundations (Jahani et al., 2022), as shown in Figure 3. These wind turbine platforms have various advantages and disadvantages. Particularly, the design of a spar-type platform is simple and straightforward, facilitating easy manufacturing and maintenance; however, the installation and maintenance costs are high. Tension leg platforms (TLPs) exhibit excellent stability and can perform well under complex environmental conditions, but their construction costs are relatively high. Barges are characterized by a large square. Although they are more sensitive to wave responses, the construction process is relatively simple and low-cost. A semi-submersible platform offers satisfactory stability, but the fabrication and maintenance are difficult and expensive.
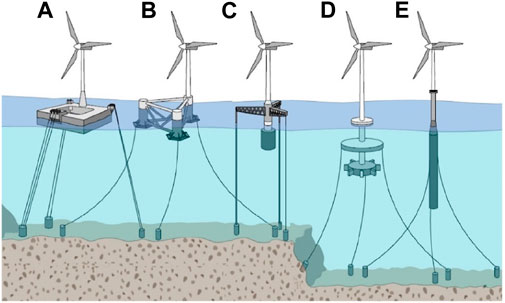
Figure 3. Most popular foundation types of floating offshore wind turbines (FOWTs): (A) barge, (B) semi-submersible, (C) TLP, and (D, E) spar.
Therefore, the number of floating offshore wind turbines (FOWTs) has significantly increased with the rapid development of the offshore wind energy industry. However, they have substantially elevated the risk of ship collisions with wind turbines, as most are employed in busy shipping lanes. Thevenet (2021) proposed that collisions may occur due to technical errors, navigation failure of the vessel, or heavy water. As the number of wind turbines has increased, the navigable space available for vessels has decreased with increasing traffic density. In addition, the number of maintenance workers required to complete operations on the wind turbine has increased. These workers require transportation on service vessels, which will increase safety risks (Yan et al., 2023). Moreover, a vessel–turbine collision can cause significant damage to both wind turbines and vessel structures, with a consequence of oil leaks, collapse of the wind turbine, and even sinking of the vessel (Yu et al., 2020).
OWTs are characterized by slender, tall, and concentrated mass structures at the top that make them highly susceptible to damage in the event of a collision. Severe collisions can cause significant structural damage or even collapse. Major components such as the blades, nacelle, and hub fall toward the vessel under the effects of gravity, inertia, and collision forces. Irreversible damage is likely to take place.
Collisions between FOWTs and vessels cause entanglement risks to mooring systems in floater drift areas. The mooring system is a critical component of the FOWTs for station-keeping under complicated environmental conditions in deep-sea areas. However, the length and mass of mooring lines have dramatically increased as the water depth increases. The larger radius of the mooring system will obstruct ship navigation, thereby increasing the likelihood of collisions between vessels, drift floaters, and wind turbines that may cause damage to mooring systems (Yang et al., 2022). Additionally, variations in wave fluctuations, water depth, and vessel draft can lead to different collision scenarios with wind turbines (Christensen et al., 2001).
Therefore, researching proactive vessel avoidance strategies for wind turbines can play a significant role in mitigating the risks associated with ship collisions and has substantial practical significance and societal value. It is also meaningful and necessary to study the dynamic behavior of vessel–turbine collisions, thus improving the characteristics of the crashworthy measures to better protect vessels and wind turbines.
The paper is divided into four aspects. First, a brief introduction to the meaning of collision analysis of OWTs subjected to vessel impacts will be presented. Second, an overview of studies in structural response and collision characteristics on the fixed-bottom– and floating OWT–ship collisions over the last few years will be discussed. Then, a discussion of representative impact factors that would play a significant role in collisions between OWTs and vessels will be summarized. Finally, the current anti-collision measures and some proposals to mitigate the negative effect of collisions found in the literature during the past 2 decades will be addressed.
2 Ship–fixed wind turbine collisions
Traditionally, ship collisions have been a major concern for bridge structures over navigable waterways, offshore oil and gas platforms, and even other ships. As the offshore wind energy industry expands, there is a growing focus on ensuring the safety of wind turbines against ship collisions. Research has predominantly focused on the ship impact forces and the associated structural damage. As shown in Figure 2, the main types of fixed-bottom OWTs used in engineering are monopile, gravity base, tripod, and jacket OWTs. Much attention has been paid to the dynamic response and damage impact of the OWTs by collision of vessels in the previous research related to ship–OWT collisions. Table 1 describes some representative collision scenarios between the fixed-bottom OWTs and some types of vessels using various simulation tools.
2.1 Monopile OWTs
Suzuki et al. (2013) estimated the collision risk in wind farms comprising bottom fixed-type wind turbines caused by 6788 GT drifting ships. Li et al. (2013) and Li et al. (2014) developed a numerical model of the monopile wind turbine and vessel through a proposed external dynamic link library (DLL) under various incident wave conditions. The shielding effects of the vessel were considered. They found that wave directions and length made a difference in impact behaviors for the wind turbine and the structural response of the vessel.
The transient response and damage of grouted connections in monopile-supported OWTs subjected to ship impacts were analyzed using LS-DYNA by Mo et al. (2018), as shown in Figure 4. A finite element model simulating a 2000-ton vessel colliding with a 5 MW monopile OWT was developed, considering the nonlinear behavior of structural materials. They found that significant damage could be caused even at a low impact velocity of 2 m/s, with the strain rate effect notably influencing the response and damage of the grouted connection.
Niklas and Bera (2022) examined the impact collision performance between a monopile wind turbine and an offshore supply vessel to investigate the effect of adopted strain-based failure criteria on damage. It was demonstrated that a six-fold underestimation of the ship damage was due to the strain failure standard. Ladeira et al. (2022) evaluated the low-energy impacts between monopile wind turbines and service ships using the proposed semi-analytical methodology. The validation of this method was achieved by comparison with nonlinear finite element method (FEM) code LS-DYNA.
2.2 Gravity base OWTs
Hamann et al. (2013) analyzed the collision behavior between a fully loaded single-hull tanker and a 6 MW gravity-base foundation wind turbine using the FEM tool. The calculated contact forces were compared with a simplified approach. The impacts of water depth, foundation diameter, and seabed embedment were also evaluated. The FEM tool effectively optimized the gravity base foundation designs for ship collisions in a cost-effective method. Osthoff and Grabe (2015) analyzed the collision characteristics between a double-hull tanker and a gravity base foundation wind turbine using the FEM code under various water levels. The effect of swell on the collision performance of the FOWT and damage to the tanker was also examined and discussed. Presencia and Shafiee (2018) developed a methodology to minimize the collision risks of multiple kinds of vessels used for maintenance with OWTs with a gravity base foundation. It was found that the collision risks were significantly subjected to the maintenance of those under a corrective replacement. Alerechi et al. (2018) analyzed the dynamic response of a reinforced concrete gravity platform exposed to crash loads from an offshore transporting vessel using ANSYS EXPLICIT DYNAMICS. Finite element analysis (FEA) was employed to evaluate the structural integrity of the offshore platform under collision scenarios with impacting velocities of 5 m/s, 10 m/s, 16 m/s, 50 m/s, and 100 m/s. The results revealed that increasing velocity was able to proportionally upgrade deformation.
2.3 Tripod OWTs
Biehl and Lehmann (2006) utilized numerical crash tests to compare multiple wind turbines colliding with vessels under various scenarios. They determined that the monopile foundation was the most reliable. Tripod and jacket OWTs must be examined in detail before being rated. The nacelle impacts and collision modes of the whole structures were examined. Lee (2013) investigated the dynamic response of a tripod wind turbine during boat collisions and compared the damage to that of a turbine protected by a rubber fender. Figure 5 shows the collision scenarios between the vessel and a tripod wind turbine. The results demonstrated that the rubber fender system using a high Mooney–Rivlin coefficient provided the most effective collision protection for the substructure. Additionally, a minimum fender thickness was found to reduce adverse effects on the wind turbine structure.
2.4 Jacket OWTs
Widjaja et al. (2013) assessed the impact energy and structural response of wind turbines based on the jacket platforms under extreme states in the Vietnamese water area. It was found that the maximum value of vessel impact energy could be decreased by adjusting structural design standards and offshore operations. Zhang et al. (2014) evaluated the collision behavior and dynamic response of a wind turbine with a jacket foundation using the automatic dynamic incremental nonlinear analysis (ADINA) method. In addition, the ship velocity, joint thickness, and impact points were calculated and compared. Hsieh (2015) proposed a simplified analytical method to accurately and efficiently estimate the impact load and structural response of the jacket foundation after a collision with a vessel based on the super-element concept. Travanca and Hao (2015) explored the dynamic response and energy dissipation of a wind turbine after collisions with ships based on the finite element (FE) framework. The effects of ship sizes, platform layout, and even ship–structure interactions were also extensively considered and examined. The data showed that plastic deformation mechanisms were more suitable for high-energy ship collision analysis on the jacket OWTs. Moulas et al. (2017) studied the damage to wind turbine foundations based on monopile and jacket structures by collisions with 4000-ton support vessels using a nonlinear finite element analysis (NLFEA) approach. They identified various collision scenarios, analyzed damage extent, evaluated reinforcement effects, and offered insights for designing more collision-resistant wind turbine foundations.
Hao and Liu (2017) evaluated the anti-impact performance of monopile, tripod, and jacket foundations for wind turbines using LS-DYNA. The FE model of the vessel and wind turbine is shown in Figure 6. Maximum collision forces, damage areas, bending moments, steel consumption, and nacelle accelerations were analyzed, revealing that jacket foundations exhibited the lowest collision forces and damage, along with moderate bending moments and steel use. It was concluded that jackets provided the best overall anti-impact performance under low-energy collisions.
Pire et al. (2018) evaluated the crashworthiness of the jacket base OWTs for each deformation mode based on the analytical formulations. A good accordance was shown between the data and the numerical results calculated by the nonlinear finite element tool. A simplified analytical method was introduced for estimating the anti-collision ability of an oblique cylinder for the jacket OWTs impacted by the stem of a striking ship by Buldgen et al. (2014) and Ladeira et al. (2023a). The collision angles were compared and examined. Closed-form solutions were derived for horizontal and vertical cylinders using the upper-bound method, and an interpolation formula was proposed for various angles. The numerical data were validated with those simulated by LS-DYNA. Although there was a high agreement, the model was limited to high-impact energy.
Although many studies have been carried out on the dynamic response of fixed-bottom offshore wind turbines to ship collisions, most calculations have used simplified models, which have a negative impact on the accuracy of the results. Additionally, soil–structure interaction effects have not been fully accounted for in these studies and need further consideration.
3 Ship–floating wind turbine collisions
Previous research has primarily investigated impacts between fixed-bottom OWTs and ships, with fewer studies examining collisions with FOWTs. This is because currently, fixed-bottom offshore wind farms are more widely and extensively operated than floating offshore wind farms. However, it is essential and valuable to study the collision impacts for all wind turbines subjected to ship impacts and develop related crashworthy measures, as the number of FOWTs is expected to increase to capture more clean energy. Some representative studies of FOWT–ship collision impacts over the last 20 years follow. The studies are summarized in Table 2.
3.1 Spar-type OWTs
Echeverry et al. (2019) analyzed the anti-collision performance between a spar-type platform and a 5000-ton vessel using LS-DYNA under various collision scenarios. The effect of properties such as nacelle mass, hydrodynamic forces, and mooring line tension of the FOWT were also investigated.
Ren et al. (2022) investigated the collision performance between a spar-type platform and a ship using a nonlinear FEM framework, as shown in Figure 7. The displacement, acceleration, collision forces, and incurred structural damage of a FOWT under various impact speeds were examined and discussed. It was found that the collision does more damage to the spar-type platform than the whole wind turbine.
A theoretical model was developed by Zhang et al. (2021), Zhang and Hu (2021), and Zhang and Hu (2022) to analyze the dynamic responses of an OC3-Hywind spar-type offshore floating wind turbine subjected to ship impacts, considering a collision duration of 0.5 s and an impact force of 2000 kN. The model integrated hydrodynamic effects using an added mass matrix and applied rigid body impact principles to derive expressions for energy dissipation and other kinetic parameters. A time-domain analytical program was developed to evaluate dynamic responses with parametric case studies revealing sensitivity to variables such as collision force and wave conditions. The surge and pitch motions of the wind turbine under ship collision with various impact velocities are shown in Figure 8. In addition, the effect of impact speed and tower flexibility of the FOWT, as well as the deformability of the ship, were studied under various wind-wave combined loading cases.
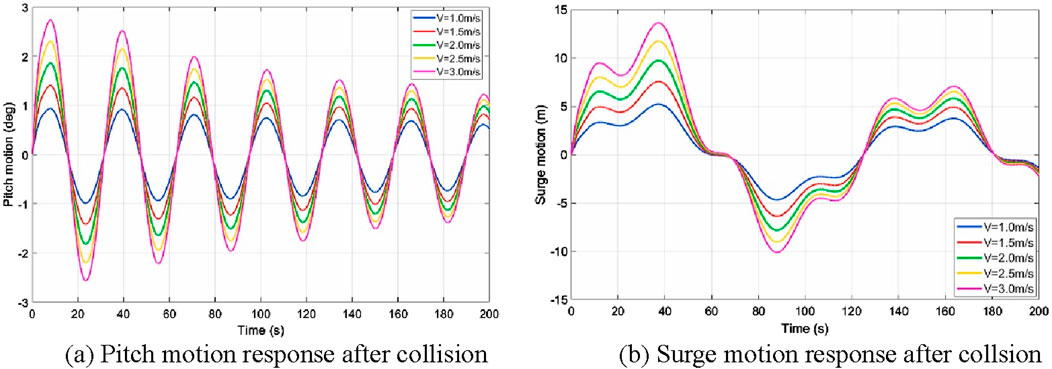
Figure 8. Comparison of motion between collision scenarios under various collision speeds: (A) pitch motion response after a collision and (B) surge motion response after a collision.
Ha and Kim (2022) investigated the dynamic response and impact characteristics of a 5 MW spar-type platform with ship collision using ABAQUS. The results were validated by comparing them to experimental data. It was found that the residual strength of the FOWT was reduced by 4.1% reduction after collision with a ship with a velocity of 3 m/s.
3.2 TLP OWTs
Yu and Bo (2021) studied the collision performance between a DTU Wind 10 MW wind turbine with an OO-STAR semi-submersible platform and an 8800-ton vessel under parked and operating conditions using LS-DYNA and OrcaFlex. The results simulated by LS-DYNA showed that a ship striking at 2.5 m/s could destroy 0.5 m concrete walls, while a ship moving at 10 m/s could damage 1 m concrete walls. In addition, the OrcaFlex calculations showed that nacelle accelerations generally exceeded safety limits, with tower stress and mooring forces also potentially surpassing thresholds, particularly under operating conditions. Guo J. et al. (2022) studied the collision between a vessel and tension leg platform wind turbines (TLPWTs) by LS-DYNA based on the fluid–structure interaction method and constant added mass. It was demonstrated that all tension legs were not slack during ship–TLPWT collisions due to prestress.
3.3 Semi-submersible OWTs
Yu and Amdahl (2021) and Yu et al. (2022) investigated the motion response and energy absorption of the OO-STAR semi-submersible wind turbine colliding with 7500-ton service and large passing vessels under parked and typical operating conditions using the nonlinear finite element code USFOS. With an initial kinetic energy of 420 MJ, the platform exhibited significant displacements and rotations. In addition, the floating wind turbines were more resistant to ship collision impact than the fixed wind turbines.
Zong et al. (2023) studied the dynamic response and collision performance of the OC4 wind turbine struck by a vessel under combined wind–wave–mooring loads based on Star-CCM+ and ABAQUS. The time-domain results, including during and after collisions, are shown in Figure 9. It was found that the collision on the side of the turbine caused a more significant effect on the structural response of the wind turbine.
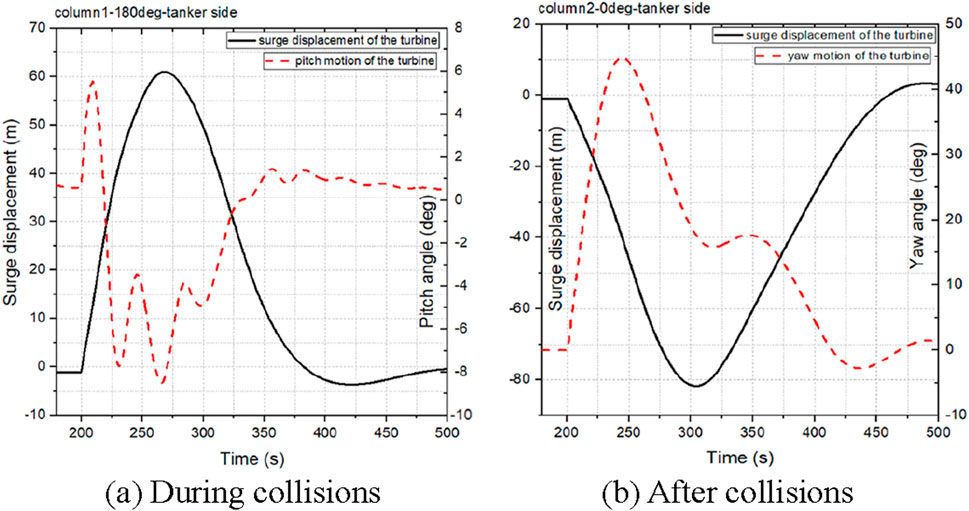
Figure 9. Motions of the OO-STAR floating wind turbine during and after tanker collisions: (A) during collisions and (B) after collisions.
Yu et al. (2024) studied the collision impact between the 15 MW UMaine VolturnUS-S wind turbines and ships, including service operation vessels, multi-purpose vessels, and anchor-handling tug ships, using ABAQUS. The influence of collision speed, displacement, angle, and ship shapes was examined and discussed based on the internal stiffener arrangement.
3.4 Barge-type OWTs
Márquez et al. (2022) proposed a mechanical model (MM) composed of an NREL 5 MW wind turbine, an ITI Energy barge-type platform, and a ship to evaluate the collision impact between the ship and the FOWT. In addition, the influence of wall thickness, collision location, and impact energy on the dynamic response of the FOWT were parametrically analyzed. The data showed that the developed model MM could precisely investigate the structural response and collision impact of both structures, as shown in Figure 10.
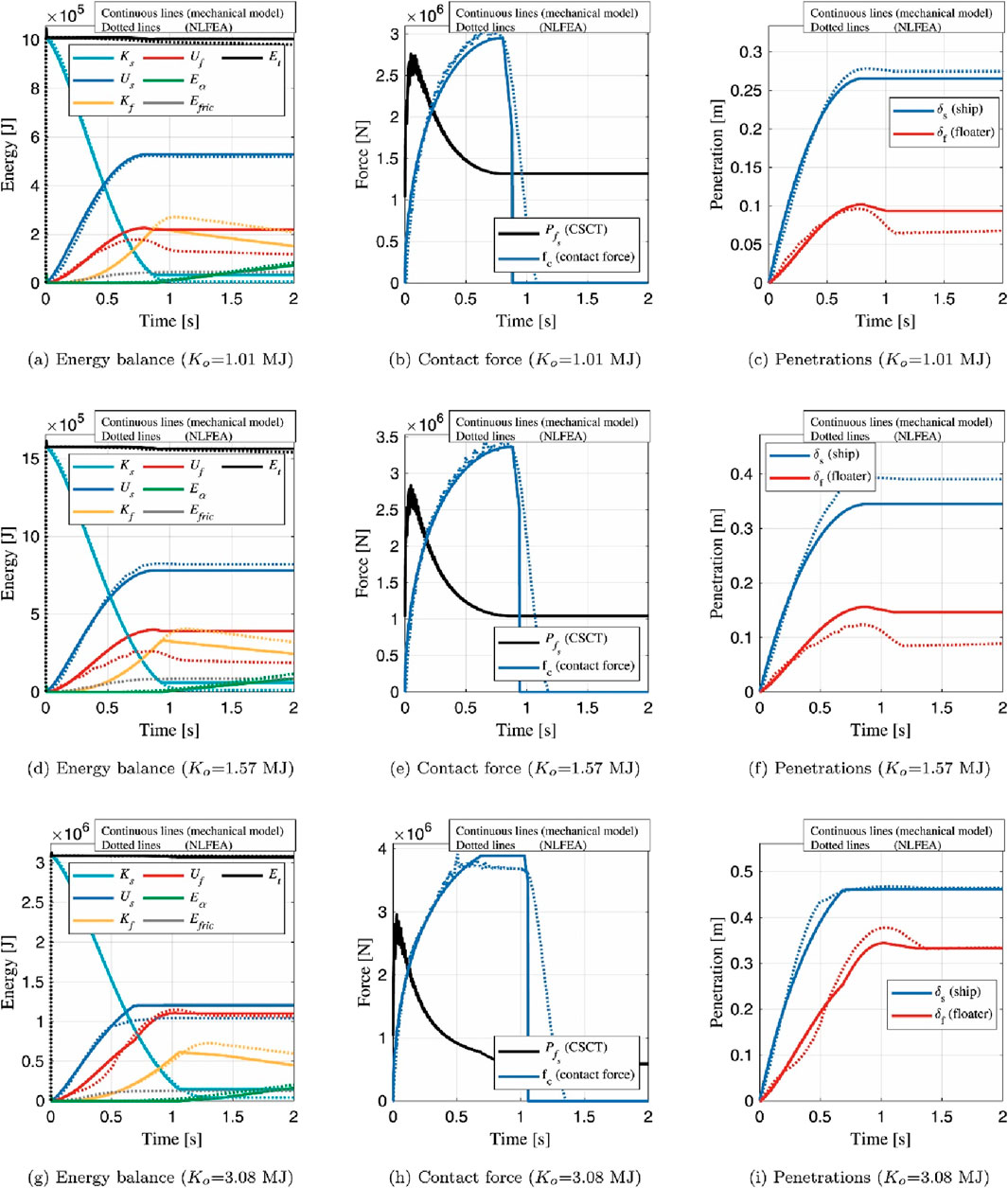
Figure 10. Energy balance, contact force, and penetration histories for different initial impact velocities.
Currently, research on collisions between floating wind turbines and ships remains limited and underdeveloped. In addition, research on collision behavior under extreme weather conditions is also inadequate. However, with the ongoing trend of installation in deep water, the number of floating wind turbines is expected to increase, increasing the risk of ship collisions. Therefore, future studies on ship collisions with floating wind turbines should receive more attention to enhance the operational safety of wind turbines in the presence of passing vessels.
4 Influence of collision factors
As offshore wind farms expand and their distance from the coast increases, the safe operation of vessels has become more crucial. However, the risk of collisions between vessels and OWTs is influenced by various factors, including wind loads, impact velocity, collision angles, and even soil properties. Therefore, it is important to study these key collision factors to thoroughly and extensively investigate the dynamic response and impact performance of OWT–ship collisions. Thus far, numerical studies have been conducted to analyze the effects of these critical collision factors, as shown in Table 3.
4.1 Collision velocity
Biehl (2004) investigated the collision resistance characteristics of monopile and tripod foundations for OWTs. The structural damage characteristics and energy dissipation curves of the monopile tripod foundation and the impact of vessel collision velocity on the results were elucidated and analyzed. Amdahl and Holmas (2011) analyzed the dynamic response of a jacket-supported wind turbine struck by a high-energy ship collision with 2 m/s impact speed. It was found that the water depth and jacket layout played an important role in the collapse mode. Ramberg (2011) analyzed the local buckling characteristics of the connection points between the jacket-supported wind turbines and US. The structural response of the wind turbine after collisions at various impact locations on the jacket was considered. Ding et al. (2014) studied the dynamic response of the FOWT subjected to the collision effect of a 5000-ton ship in the front point under various speeds based on the finite element code ABAQUS/Explicit. A significant inertial force was observed at the top of the tower, while the damage in the ship bow was relatively slight.
Travanca and Hao (2014) conducted a comprehensive numerical simulation of ship impacts on offshore jacket leg structures. The effects of impact velocity, bow stiffness, and pipe dimensions on ship collisions on the collision performance were also investigated. The impact velocity analysis with and without strain rate effects is shown in Figure 11. The vessel model comprised the range of 2000–5000-ton displacement.
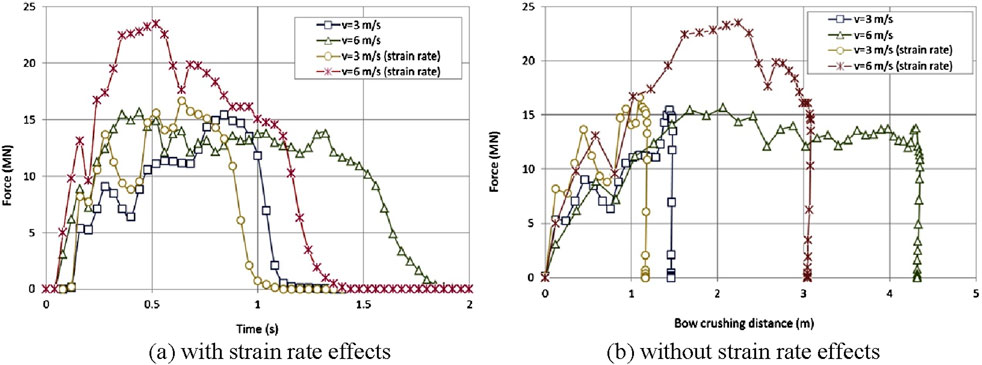
Figure 11. Impact velocity analysis with and without strain rate effects: (A) with strain rate effects and (B) without strain rate effects.
Moulas et al. (2017) analyzed the collision damage that occurred when wind turbine foundations were struck by a 4000-ton ship. The monopile and jacket structures in shallow and deep water were adopted in that study. It indicated that collision energy, vessel height, and collision position were significant factors influencing the impacts between monopile OWTs and vessels. Prabowoputra et al. (2020) showed that OWT–ship collisions were affected by velocity, types of vessels, impact direction, collision angle, and so on. It used the factorial design method to reveal that velocity and collision direction were the most significant factors.
4.2 Soil impact
Ramberg (2011) studied the impact of collisions between monopile wind turbines and vessels. The correct selection of soil parameters and the consideration of soil–structure interaction had a significant impact on the collision performance. Sourne et al. (2015) investigated the dynamic response of the wind turbine to a collision with a ship to optimize the design of the main aspects. Particularly, the impact of foundation soil characteristics, like site conditions, on the dynamic response of the wind turbine was studied. In addition, two vessel drift speeds, 2 m/s and 5 m/s, were adopted to distinguish the impact energy absorbed by the foundation. Bela et al. (2015) studied the nacelle dynamics and crushing behavior of the monopile wind turbine subjected to a ship collision. The effects of multiple properties like ship location, soil stiffness, and deformability of the striking ship were also presented. Han et al. (2019) and Han et al. (2020) investigated the anti-collision performance of a 4 MW tripod wind turbine that used fenders subjected to the collision impact of the 2500-ton ship using ANSYS/LS-DYNA. In addition, the collision impact of the wind turbine from a 5000-ton ship was discussed. They also considered various material properties and thickness of the fender as well as the influence of soil–structure interaction. It was found that more effective anti-collision performance would be achieved when the thickness of the fender surpassed 1.1 m.
4.3 Environmental load
Zhang et al. (2014) studied the dynamic response of a FOWT to ship collision scenarios under multiple environmental conditions. It was found that the surge and pitch motions of the platform were dramatically affected in still water, as was the mooring system, while the yaw response would be increased under combined wind-wave conditions. Song et al. (2021) evaluated the dynamic response of a 5 MW monopile wind turbine to a collision with a 4600-ton vessel under various collision scenarios. The influences of aerodynamic damping, mean wind velocity, and ship impact speed were examined and compared using numerical and analytical methods. It demonstrated that the difference between those methods was 5% and 7% under impact speeds of 1 m/s and 3 m/s, respectively.
4.4 Collision position
Kroondijk (2012) examined the collision performance of the jacket OWTs after a collision with a 160,000-ton oil tanker under loaded and unloaded conditions. Failures and overall structural damage to the jacket foundation were revealed. Dai et al. (2013) proposed a new framework to assess collision risks and key impact factors. The results indicated that low-speed collisions could potentially cause structural damage to wind turbines. In addition, seven collision scenarios, including head-on, maneuvering, and drifting collisions between the ship and wind turbine, were examined and compared. The results showed that the most significant damage would be caused if a ship directly struck the wind turbine. Hao and Liu (2017) investigated the foundation damage after a maximum collision force of a ship against a wind turbine. The impact factors, such as ship mass, velocity, and collision position, were compared.
A comprehensive evaluation of the anti-collision measures of various wind turbine platforms was conducted using the FEM tool LS-DYNA. The most optimum crashworthy performance was achieved by the jacket under low-energy collisions. Bela et al. (2017) conducted numerical simulations of vessel impacts on monopile foundation wind turbines. The effects of impact velocity, position, wind loads, and soil–structure interaction of dynamic responses of the tower structure for the wind turbine were studied. Gao and Zhang (2021) investigated the dynamic response of the jacket foundation for a 3 MW wind turbine after a collision with a 3000-ton cargo vessel. The impact of collision position and joint strength were distinguished and discussed. Liu et al. (2022) analyzed the collision performance of the wind turbine with a jacket foundation to identify the most vulnerable part and dangerous impaction point during a collision by vessels. The influence of impact points, collision directions, and angles were compared. The results showed that the eccentric impact would cause less severe local deformation than a centric collision impact, as depicted in Figure 12.
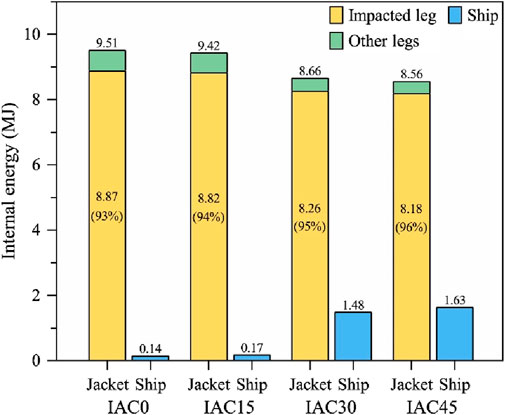
Figure 12. Comparison of energy distributions between jacket foundation and striking ship under four different centric impacts (IAC0-IAC45).
Mehreganian et al. (2024) studied two scenarios of blast phenomena and impact loads on wind turbines caused by collision with commercial ships. The impact of wind velocity, collision angle, and gravity loads have also been considered.
In conclusion, various studies have examined the significant impact factors on the dynamic response of the wind turbines subjected to a vessel collision. The parametrical analysis of the collision has a dramatic effect. However, the soil–structure interaction is so complicated that little research has addressed this component. In addition, the study of collision mechanics and structural response between wind turbines and ships is still insufficiently extensive.
5 Anti-collision measures
As the risks of collisions between ships and wind turbines increase, it is crucial and valuable to take measures to reduce the negative influence of such collisions. To mitigate the damage to wind turbines and ships caused by collisions, several protective measures have been proposed, including crashworthy devices and anti-collision prediction systems, as well as analyzing and predicting the OWT–ship collision risks. However, most of these measures are still in the study phase. Table 4 presents some crashworthy measures implemented during the last 2 decades.
5.1 Crashworthy devices
Cezary Graczykowski et al. Yue et al. (2021a) introduced a torus-shaped adaptive inflatable structure featuring multiple distinct air chambers with mechanisms for rapid inflation and pressure release and validated the effectiveness of the device through finite element simulations of ship–OWT collisions. Ren and Ou (2009), Graczykowski and Holnicki-Szulc (2009) investigated the dynamic response of the typical 3 MW wind turbine with a monopile foundation damaged by the collision of a simplified 2000-ton class ship model using LS-DYNA. A novel conceptual steel sphere ring aluminum foam pad for wind turbines has been proposed. Additionally, a spherical layout of the crashworthy device could deflect the ship from the main wind turbine substructure, thus minimizing the impact of the damage from the ship. The dynamic responses of a wind turbine subjected to vessel collisions under varying vessel velocities, rubber material, and fender thickness were considered and optimized using the FEM tool LS-DYNA by Lee and Park (2012) (Ren and Ou, 2009). In addition, they proposed a framework to mitigate the impact on tripod-type wind turbine substructures struck by ships. The materials, such as natural rubber, neoprene, and composite, were selected to optimize the anti-collision effect. The result has shown that a rubber fender with a relatively lower thickness played a significant role in mitigating the collision impact from a ship. The impact between the ship and the tripod wind turbine substructure is complicated. Liu et al. (2015), Lee and Park (2012) proposed a novel crashworthy device comprising an outer steel shell and a rubber blanket to decrease the damage of monopile wind turbines by ship collision. It was able to significantly decrease the maximum collision force and nacelle acceleration for vessels with smaller initial kinetic energy. Ren et al. (2022), Liu et al. (2015) carried out an experimental study on the dynamic response of a 4 MW monopile wind turbine with a collision by a vessel. The effect of the crashworthy device on the anti-collision performance of the wind turbine was investigated. The protected device was capable of effectively reducing the top nacelle acceleration and the ship impact force.
Han et al. (2019) investigated the anti-collision performance of various fenders for wind turbine tripods during collisions with 2500-ton ships through ANSYS/LS-DYNA. The installation position and shape of the fender are shown in Figure 13. Four fender types made from two materials and combined in different configurations were evaluated based on collision force, energy absorption, maximum bending moment, and plastic strain. The results indicated that aluminum foam fenders performed best in protection, and the coefficient of restitution (COR) provided further insights into fender longevity.
Yue et al. (2021b) developed a fender for a tripod wind turbine to mitigate collision impact by ship. The effects of external diameter and various orders on the anti-collision impact on the wind turbine were examined using LS-DYNA. It was found that the structure of the first-order fractal pores played a significant role in absorbing more collision energy and enhancing the anti-collision performance of the fender. A literature review of analytical, numerical, and experimental methods for assessing the structural response of OWTs during ship collisions was presented by Ladeira et al. (2023b). Various energy transfer mechanisms and common procedures for evaluating wind load effects, soil–structure interactions, and hydrodynamic coupling were examined. Internal mechanics and hydrodynamic coupling schemes have also been surveyed, and the limitations of current models are discussed with recommendations for future research. Sun and Fang (2023) proposed a floating composite honeycomb anti-collision structure to mitigate the damage from ship–OWT high-energy collisions under typical scenarios. The anti-collision performance and dynamic response of a wind turbine with crashworthy structures were examined and compared to that without protection. The results showed that the structure was capable of absorbing 80% of the initial kinetic energy, dramatically decreasing the negative dynamic response of the wind turbine. Niklas et al. (2023) studied the collision characteristics between a 15 MW monopile wind turbine and a 6500-ton displacement supply offshore vessel. It was found that utilizing S355 grade steel could dramatically mitigate collision impact by 50% during a head-on sliding collision. Nie et al. (2024) proposed a novel fender employed on the monopile foundation wind turbine to mitigate the collision impact by ship. A viscoelastic fender with high initial stiffness achieved the most significant reductions in collision metrics, with maximum collision force decreasing by approximately 46.5% and acceleration by 54.8%.
5.2 Anti-collision systems
Hirokawa et al. (2015) assessed the risk of mooring failure in FOWTs caused by collisions with drifting ships. A simulator was developed to replicate the collision process and drifting scenarios to establish a risk evaluation framework. The results indicated that the risk was primarily influenced by the potential for large ships to displace turbines, with additional risk depending on the arrangement of the wind farms. Copping et al. (2016) utilized the automatic identification system (AIS) system to mitigate the collision risk between ships and wind turbines, improving navigation safety. Mujeeb-Ahmed et al. (2018) developed a comprehensive and intelligible collision-risk method for OWTs exposed to ship collision impact through an AIS database. The statistical distribution of ship traffic and straightforward probabilistic methods were established to effectively predict the impact energy and collision risks for multiple types of vessels.
Kim et al. (2021) focused on the collision risks of wind turbine installation vessels (WTIVs) that are widely operated in offshore wind farms. A method for analyzing WTIV collision frequency was developed and compared with the existing methods. The study underscored the importance of considering the operational characteristics of WTIVs and discussed the implications for analyzing the collision frequency between wind turbines and vessels. The proposed method considered the design accidental load (DAL), while other methods only evaluated WTIVs as a fixed platform. Sen and Song (2021) examined the crashworthiness of the wind turbine against the collision by vessels based on the No.6 offshore wind farm project in Zhoushan Putuo, in eastern China. The data highlighted the demand for unified criteria so as to meet ocean challenges like channel distance, emergency management, and collision prevention. Xue and Qian (2023) proposed an anti-collision mechanism based on improved swarm intelligence. An adaptive brain storm optimization (ABSO) with variational inference-based expectation maximization was established to avoid collision impact. In addition, the algorithm precisely and effectively improved navigation safety near offshore wind farms by optimizing turning amplitudes and route distances in tests.
Although numerical methods have been investigated to mitigate the negative influence of ship collisions with wind turbines, such as installing fenders and adaptive devices on the wind turbines, there is still limited application to engineering practice. Additionally, methods to reduce collision-risk coefficients using computer algorithms remain underdeveloped due to the current measures only making differences after collision. Advanced technologies like automated warning systems and intelligent navigation prediction frameworks are still limited in anti-collision applications. Research on reducing collision risk factors between ships and wind turbines is becoming increasingly important with the rapid development of the wind industry.
6 Conclusion
This paper provides an in-depth overview of research on the dynamic analysis of OWTs subjected to ship impacts, including the associated impact factors. Additionally, it summarizes the crashworthy measures developed over the past 20 years to counteract the negative effects of collision impacts on wind turbines and vessels. The paper concludes with a review of the latest advancements in wind turbine collision analysis.
(1) With the rapid expansion of offshore wind farms, vessel–turbine collisions have become increasingly common. These incidents not only cause damage to the wind turbines but also increase the risks to navigation safety for the vessels. This collision risk will influence maritime navigation safety and significantly hamper the advancement of the offshore wind energy industry.
(2) Several studies have extensively investigated the dynamic response and damage impact of fixed OWTs subjected to vessel collision. However, most of these studies have only used simplified vessel models during simulation. Because the collisions are inherently complicated, the use of simplified vessel models may influence the accuracy of calculation results.
(3) Little attention has been paid to vessel–FOWT collisions. Even existing studies are limited to the spar-type platform, ignoring TLP, barge, and semi-submersible FOWTs. However, floating wind turbines are more suitable for deep-sea areas than the fixed OWTs. Additional analyses of FOWT–vessel collisions are needed due to the rapid development of floating wind turbines.
(4) Because OWT–vessel collisions are significantly complex, a variety of factors would affect the collision performance. Some research has analyzed the predominant impact factors, such as wind load, collision angle, impact velocity, and so on. However, the understanding of the effect of soil–structure interaction on the OWT–vessel collision still has limitations.
(5) Research on collisions between vessels and wind turbines primarily focuses on the dynamic response under various collision scenarios. Most studies emphasize physical protective measures, such as equipping fenders and adaptive devices, to reduce the damage sustained by wind turbines from vessel collisions.
Considering the current state of research and the practical demands in this field, analysis of turbine–vessel collision issues could be further studied in the following areas:
(1) Existing studies have focused on the dynamic behavior of ship collisions with wind turbines. However, most studies use simplified collision models, which do not accurately reflect the actual dynamic responses during collisions. Additionally, the complex and variable environmental loads of wind, waves, and currents are difficult to comprehensively consider, and extreme conditions especially lack attention.
(2) The current research has primarily focused on fixed wind turbines, neglecting the FOWTs. Given the significant growth of the wind energy industry in recent years and the increasing installed capacity of floating wind turbines, it is crucial to investigate the collision impact between the vessels and floating wind turbines.
(3) Various studies related to key impact factors of vessel–turbine collisions limit the influence of soil–structure interaction during a collision. However, soil–structure interaction significantly influences the dynamic response of fixed wind turbines subjected to vessel collision, particularly in terms of force transmission and energy dissipation. Additional research would help optimize structural design, enhance collision resistance, and reduce potential damage.
(4) Although there has been some research into proposed anti-collision measures to protect wind turbines, this area remains relatively unexplored. Current protective measures could be improved by optimizing size or material properties to limit risk mitigation using artificial intelligence databases. In addition, existing protective designs are ineffective in protecting wind turbines during high-energy impacts. Future research in this field is expected to become increasingly comprehensive and advanced.
Author contributions
LM: writing–original draft, resources, methodology, investigation, data curation, and conceptualization. JD: writing–review and editing and data curation. XaZ: writing–original draft, project administration, methodology, and funding acquisition. WW: writing–review and editing and project administration. XoZ: writing–review and editing and software. CS: writing–review and editing and funding acquisition. YY: writing–review and editing and funding acquisition.
Funding
The author(s) declare that financial support was received for the research, authorship, and/or publication of this article. The authors are grateful for the financial support from the China Three Gorges Group Co., Ltd. (Contract No: 202303059) and the Guangxi science and technology research and industrial application of offshore wind power supporting equipment in Beibu Gulf of Guangxi (Guike AA220681).
Conflict of interest
Authors LM, XaZ, WW, and XoZ were employed by China Three Gorges Co., Ltd.
The remaining authors declare that the research was conducted in the absence of any commercial or financial relationships that could be construed as a potential conflict of interest.
The authors declare that this study received funding from China Three Gorges Group Co., Ltd. The funder had the following involvement in the study: design, collection, analysis, interpretation of data, the writing of this article, and the decision to submit it for publication.
Publisher’s note
All claims expressed in this article are solely those of the authors and do not necessarily represent those of their affiliated organizations, or those of the publisher, the editors, and the reviewers. Any product that may be evaluated in this article, or claim that may be made by its manufacturer, is not guaranteed or endorsed by the publisher.
References
Alerechi, L. W., Oluwole, O. O., and Odunfa, K. M. (2018). Finite element analysis of concrete gravity based platform subjected to sudden crash (impact) load using ANSYS. COVENANT J. Eng. Technol. doi:10.1080/17445302.2020.1735835
Amdahl, J., and Holmas, T. (2011). High energy ship collisions with jacket supported offshore wind turbines.
Bela, A., Le Sourne, H., Buldgen, L., and Rigo, P. (2015). “Numerical crashworthiness analysis of an offshore wind turbine monopile impacted by a ship,” in MARSTRUCT 2015 5th international conference on marine structures (London, United Kingdom: Taylor & Francis Group).
Bela, A., Le Sourne, H., Buldgen, L., and Rigo, P. (2017). Ship collision analysis on offshore wind turbine monopile foundations. Mar. Struct. 51, 220–241. doi:10.1016/j.marstruc.2016.10.009
Biehl, F. (2004). “Ship collisions and offshore wind energy turbines: calculation and evaluation,” in Proceedings of the scientific forum of the federal ministry for the environment, nature conservation and nuclear safety (BMU) on offshore wind energy (Berlin, Germany: Utilisation), 15–18.
Biehl, F., and Lehmann, E. (2006). Collisions of ships and offshore wind turbines: calculation and risk evaluation. Int. Conf. Offshore Mech. Arct. Eng. 47462, 663–670. doi:10.1115/omae2006-92270
Buldgen, L., Le Sourne, H., and Pire, T. (2014). Extension of the super-elements method to the analysis of a jacket impacted by a ship. Mar. Struct. 38, 44–71. doi:10.1016/j.marstruc.2014.05.002
Christensen, C. F., Andersen, L. W., and Pedersen, P. H. (2001). Ship collision risk for an offshore wind farm. Struct. Saf. 1-7.
Copping, A., Breithaupt, S., Whiting, J., Grear, M., Tagestad, J., and Shelton, G. (2016). Likelihood of a marine vessel accident from wind energy development in the Atlantic. Wind Energy 19 (9), 1557–1566. doi:10.1002/we.1935
Dai, L., Ehlers, S., Rausand, M., and Utne, I. B. (2013). Risk of collision between service vessels and offshore wind turbines. Reliab. Eng. & Syst. Saf. 109, 18–31. doi:10.1016/j.ress.2012.07.008
Ding, H., Zhu, Q., and Zhang, P. (2014). Dynamic simulation on collision between ship and offshore wind turbine. Trans. Tianjin Univ. 20 (1), 1–6. doi:10.1007/s12209-014-2133-x
Echeverry, S., Márquez, L., Rigo, P., and Le Sourne, H. (2019). “Numerical crashworthiness analysis of a spar floating offshore wind turbine impacted by a ship,” in Developments in the collision and grounding of ships and offshore structures (Lisbon, Portugal: CRC Press), 85–95.
Gao, D. W., and Zhang, C. W. (2021). “Dynamic behavior of jacket foundation for offshore wind turbine subjected to ship impact,” in EASEC16: proceedings of the 16th east asian-pacific conference on structural engineering and construction, 2019 (Singapore: Springer), 719–729.
Graczykowski, C., and Holnicki-Szulc, J. (2009). Protecting offshore wind turbines against ship impacts by means of adaptive inflatable structures. Shock Vib. 16 (4), 335–353. doi:10.1155/2009/725969
Guo, J., Zhao, Y., Chen, W., and Zhou, G. (2022b). Simulation of a ship and tension leg platform wind turbine collision. J. Mar. Sci. Technol. 29 (6), 731–741. doi:10.51400/2709-6998.2553
Guo, Y., Wang, H., and Lian, J. (2022a). Review of integrated installation technologies for offshore wind turbines: current progress and future development trends. Energy Convers. Manag. 255, 115319. doi:10.1016/j.enconman.2022.115319
Ha, K., and Kim, J.-B. (2022). Collision analysis and residual longitudinal strength evaluation of a 5 MW spar floating offshore wind turbine impacted by a ship. Int. J. Precis. Eng. Manufacturing-Green Technol. 9, 841–858. doi:10.1007/s40684-021-00390-z
Hamann, T., Pichler, T., and Grabe, J. (2013). “Numerical simulation of ship collision with gravity base foundations of offshore wind turbines,” in International Conference on Offshore Mechanics and Arctic Engineering (American Society of Mechanical Engineers), V006T10A037. doi:10.1115/omae2013-1162755409
Han, Z., Li, C., Deng, Y., and Liu, J. (2019). The analysis of anti-collision performance of the fender with offshore wind turbine tripod impacted by ship and the coefficient of restitution. Ocean. Eng. 194, 106614. doi:10.1016/j.oceaneng.2019.106614
Han, Z., Zhao, X., Li, C., and Ding, Q. (2020). Effects on the various aluminum foam fenders of a tripod offshore wind turbine collision due to a ship. Mar. Technol. Soc. J. 54 (1), 79–96. doi:10.4031/mtsj.54.1.8
Hao, E., and Liu, C. (2017). Evaluation and comparison of anti-impact performance to offshore wind turbine foundations: monopile, tripod, and jacket. Ocean. Eng. 130, 218–227. doi:10.1016/j.oceaneng.2016.12.008
Hirokawa, E., Suzuki, H., Hirabayashi, S., and Muratake, M. (2015). “Estimation of risk of progressive drifts in a wind farm caused by collision of drift ship,” in International Conference on Offshore Mechanics and Arctic Engineering American Society of Mechanical Engineers, V006T05A011. doi:10.1115/omae2015-41473
Jahani, K., Langlois, R. G., and Afagh, F. F. (2022). Structural dynamics of offshore Wind Turbines: a review. Ocean. Eng. 251, 111136. doi:10.1016/j.oceaneng.2022.111136
Kim, S. J., Seo, J. K., Ma, K. Y., and Park, J. S. (2021). Methodology for collision-frequency analysis of wind-turbine installation vessels. Ships Offshore Struct. 16 (4), 423–439. doi:10.1080/17445302.2020.1735835
Kroondijk, R. (2012). High energy ship collisions with bottom supported offshore wind turbines (Master's thesis, Institutt for marin teknikk).
Ladeira, I., Jaramillo, S. E., and Le Sourne, H. (2023a). A simplified method to assess the elasto-plastic response of standalone tubular Offshore Wind Turbine supports subjected to ship impact. Ocean. Eng. 279, 114313. doi:10.1016/j.oceaneng.2023.114313
Ladeira, I., Le Sourne, H., Moran, J., and Salazar, P. (2022). “A semi-analytical methodology to assess the dynamic elastic response of a monopile offshore wind turbine subjected to low-energy ship impacts,” in International Conference on Offshore Mechanics and Arctic Engineering (American Society of Mechanical Engineers), V002T02A012. doi:10.1115/omae2022-7967285864
Ladeira, I., Márquez, L., Echeverry, S., Le Sourne, H., and Rigo, P. (2023b). Review of methods to assess the structural response of offshore wind turbines subjected to ship impacts. Ships Offshore Struct. 18 (6), 755–774. doi:10.1080/17445302.2022.2072583
Lee, K. (2013). Effects on the various rubber fenders of a tripod offshore wind turbine substructure collision strength due to boat. Ocean. Eng. 72, 188–194. doi:10.1016/j.oceaneng.2013.06.014
Lee, K. S., and Park, R. S. (2012). Effective arrangement of rubber fenders of wind-measuring met mast due to boat. Int. J. Offshore Polar Eng. 22 (01).
Li, J., Wang, G., Li, Z., Yang, S., Chong, W., and Xian, X. (2020). A review on development of offshore wind energy conversion system. Int. J. Energy Res. 44 (12), 9283–9297. doi:10.1002/er.5751
Li, L., Gao, Z., and Moan, T. (2013). “Numerical simulations for installation of offshore wind turbine monopiles using floating vessels,” in International Conference on Offshore Mechanics and Arctic Engineering (American Society of Mechanical Engineers), V008T09A076. doi:10.1115/omae2013-1120055423
Li, L., Gao, Z., Moan, T., and Ormberg, H. (2014). Analysis of lifting operation of a monopile for an offshore wind turbine considering vessel shielding effects. Mar. Struct. 39, 287–314. doi:10.1016/j.marstruc.2014.07.009
Liu, C., Hao, E., and Zhang, S. (2015). Optimization and application of a crashworthy device for the monopile offshore wind turbine against ship impact. Appl. Ocean Res. 51, 129–137. doi:10.1016/j.apor.2015.03.004
Liu, Q., Lei, X., Lai, Y., Qin, M., Zhang, X., Ma, L., et al. (2024). Analysis of dynamic response of offshore wind turbines subjected to earthquake loadings and the corresponding mitigation measures: a review. Ocean. Eng. 311, 118892. doi:10.1016/j.oceaneng.2024.118892
Liu, X., Jiang, D., Liufu, K., Fu, J., Liu, Q., and Li, Q. (2022). Numerical investigation into impact responses of an offshore wind turbine jacket foundation subjected to ship collision. Ocean. Eng. 248, 110825. doi:10.1016/j.oceaneng.2022.110825
Márquez, L., Le Sourne, H., and Rigo, P. (2022). Mechanical model for the analysis of ship collisions against reinforced concrete floaters of offshore wind turbines. Ocean. Eng. 261, 111987. doi:10.1016/j.oceaneng.2022.111987
Mehreganian, N., Safa, Y., and Boiger, G. K. (2024). “Impact analysis of wind turbines subjected to ship collision and blast loading,” in Multiphysics of wind turbines in extreme loading conditions (Academic Press), 101–138.
Mo, R., Li, M., and Kang, H. (2018). Transient behaviour of grouted connections of offshore wind turbines subject to ship impact. Appl. Ocean Res. 76, 159–173. doi:10.1016/j.apor.2018.05.006
Moulas, D., Shafiee, M., and Mehmanparast, A. J. O. E. (2017). Damage analysis of ship collisions with offshore wind turbine foundations. Ocean. Eng. 143, 149–162. doi:10.1016/j.oceaneng.2017.04.050
Mujeeb-Ahmed, M. P., Seo, J. K., and Paik, J. K. (2018). Probabilistic approach for collision risk analysis of powered vessel with offshore platforms. Ocean. Eng. 151, 206–221. doi:10.1016/j.oceaneng.2018.01.008
Nie, Y., Fang, H., and Meng, X. (2024). Ship collision mitigation for offshore wind turbine monopile foundations via high-general/low-collision-stiffness steel fenders. Ocean. Eng. 299, 117272. doi:10.1016/j.oceaneng.2024.117272
Niklas, K., and Bera, A. (2022). The influence of selected strain-based failure criteria on ship structure damage resulting from a collision with an offshore wind turbine monopile. Pol. Marit. Res. 28 (4), 42–52. doi:10.2478/pomr-2021-0048
Niklas, K., Bera, A., and Garbatov, Y. (2023). Impact of steel grade on a ship colliding with an offshore wind turbine monopile supporting structure. Ocean. Eng. 287, 115899. doi:10.1016/j.oceaneng.2023.115899
Osthoff, D., and Grabe, J. (2015). “Collision of double hull tanker with gravity base foundation of offshore wind turbine: case of horizontal drift and swell,” in Frontiers in Offshore Geotechnics III: Proceedings of the 3rd International Symposium on Frontiers in Offshore Geotechnics (ISFOG 2015) (Oslo, Norway: Taylor & Francis Books Ltd), 795–800. doi:10.1201/b18442-1111
Pire, T., Le Sourne, H., Echeverry, S., and Rigo, P. (2018). Analytical formulations to assess the energy dissipated at the base of an offshore wind turbine jacket impacted by a ship. Mar. Struct. 59, 192–218. doi:10.1016/j.marstruc.2018.02.002
Prabowoputra, D. M., Prabowo, A. R., Bahatmaka, A., and Hadi, S. (2020). Analytical review of material criteria as supporting factors in horizontal axis wind turbines: effect to structural responses. Procedia Struct. Integr. 27, 155–162. doi:10.1016/j.prostr.2020.07.021
Presencia, C. E., and Shafiee, M. (2018). Risk analysis of maintenance ship collisions with offshore wind turbines. Int. J. Sustain. Energy 37 (6), 576–596. doi:10.1080/14786451.2017.1327437
Ramberg, H. F. (2011). High energy ship collisions with bottom supported offshore wind turbines Master's thesis, Norges teknisk-naturvitenskapelige universitet, Fakultet for ingeniørvitenskap og teknologi, Institutt for marin teknikk).
Ren, N. X., and Ou, J. P. (2009). A crashworthy device against ship-OWT collision and its protection effects on the tower of offshore wind farms. China Ocean. Eng. 23 (4), 594–602.
Ren, Y., Meng, Q., Chen, C., Hua, X., Zhang, Z., and Chen, Z. (2022). Dynamic behavior and damage analysis of a spar-type floating offshore wind turbine under ship collision. Eng. Struct. 272, 114815. doi:10.1016/j.engstruct.2022.114815
Sen, Z., and Song, L. (2021). “Research on anti-ship collision measures for offshore wind farm in operation period-case study of zone 2 project of guodian zhoushan Putuo No. 6 offshore wind farm,” in Journal of Physics: Conference Series (Busan, Republic of Korea: IOP Publishing), 022016. doi:10.1088/1742-6596/1802/2/022016
Song, M., Jiang, Z., and Yuan, W. (2021). Numerical and analytical analysis of a monopile-supported offshore wind turbine under ship impacts. Renew. Energy 167, 457–472. doi:10.1016/j.renene.2020.11.102
Sourne, H. L., Barrera, A., and Maliakel, J. B. (2015). Numerical crashworthiness analysis of an offshore wind turbine jacket impacted by a ship. J. Mar. Sci. Technol. 23 (5), 13.
Sun, J., and Fang, H. (2023). The analysis of crashworthiness and dissipation mechanism of novel floating composite honeycomb structure against ship-OWT collision. Ocean. Eng. 287, 115819. doi:10.1016/j.oceaneng.2023.115819
Suzuki, H., Okayama, S., and Fukumoto, Y. (2013). “Collision of a drifting ship with wind turbines in a wind farm,” in International Conference on Offshore Mechanics and Arctic Engineering (American Society of Mechanical Engineers), V003T05A007. doi:10.1115/omae2013-1044055355
Travanca, J., and Hao, H. (2014). Numerical analysis of steel tubular member response to ship bow impacts. Int. J. Impact Eng. 64, 101–121. doi:10.1016/j.ijimpeng.2013.10.007
Travanca, J., and Hao, H. (2015). Energy dissipation in high-energy ship-offshore jacket platform collisions. Mar. Struct. 40, 1–37. doi:10.1016/j.marstruc.2014.10.008
Widjaja, J. H., Le Trung, T., and Quyen, T. T. (2013). Assessment of probability of failure of steel jacket structures subject to ship collision in offshore Viet Nam. Petrovietnam J. 6, 55–63.
Xue, H., and Qian, K. (2023). Ship collision avoidance based on brain storm optimization near offshore wind farm. Ocean. Eng. 268, 113433. doi:10.1016/j.oceaneng.2022.113433
Yan, K., Wang, Y., Wang, W., Qiao, C., Chen, B., and Jia, L. (2023). A system-theory and complex network-fused approach to analyze vessel–wind turbine allisions in offshore wind farm waters. J. Mar. Sci. Eng. 11 (7), 1306. doi:10.3390/jmse11071306
Yang, R., Zheng, X., Chen, J., and Wu, Y. (2022). Current status and future trends for mooring systems of floating offshore wind turbines. Sustain. Mar. Struct. 4 (2), 40–54. doi:10.36956/sms.v4i2.617
Yu, Q., Liu, K., Chang, C. H., and Yang, Z. (2020). Realising advanced risk assessment of vessel traffic flows near offshore wind farms. Reliab. Eng. & Syst. Saf. 203, 107086. doi:10.1016/j.ress.2020.107086
Yu, Y. J., Park, S. H., and Cho, S. R. (2024). Collision-damage analysis of a floating offshore wind turbine considering ship-collision risk. J. Ocean Eng. Technol. 38, 124–136. doi:10.26748/ksoe.2023.036
Yu, Z., Amdahl, J., Rypestøl, M., and Cheng, Z. (2022). Numerical modelling and dynamic response analysis of a 10 MW semi-submersible floating offshore wind turbine subjected to ship collision loads. Renew. Energy 184, 677–699. doi:10.1016/j.renene.2021.12.002
Yu, Z., and Bo, W. (2021). Analysis of a semi-submersible floating offshore wind turbine subjected to ship collisions. Master's thesis, uis.
Yu, Z. L., and Amdahl, J. (2021). “Ship collision against a 10 MW semi-submersible floating offshore wind turbine,” in Developments in the analysis and design of marine structures (Trondheim, Norway: CRC Press), 270–277.
Yue, X., Han, Z., Li, C., and Zhao, X. (2021a). The study on structure design of fender of offshore wind turbine based on fractal feature during collision with ship. Ocean. Eng. 236, 109100. doi:10.1016/j.oceaneng.2021.109100
Yue, X., Han, Z., Li, C., and Zhao, X. (2021b). The study on structure design of fender of offshore wind turbine based on fractal feature during collision with ship. Ocean. Eng. 236, 109100. doi:10.1016/j.oceaneng.2021.109100
Zhang, J., Gao, D., Sun, K., and Zhang, Z. (2014). “Ship impact behavior on jacket type offshore wind turbine foundation,” in International Conference on Offshore Mechanics and Arctic Engineering (American Society of Mechanical Engineers), V04BT02A040. doi:10.1115/omae2014-2457845431
Zhang, Y., and Hu, Z. (2021). “Investigation on the performance of an OC3-Hywind Spar-type floating wind turbine impact by an offshore service vessel,” in Maritime Technology and Engineering (Lisbon, Portugal: CRC Press), 537–544. doi:10.1201/9781003216582-60
Zhang, Y., and Hu, Z. (2022). An aero-hydro coupled method for investigating ship collision against a floating offshore wind turbine. Mar. Struct. 83, 103177. doi:10.1016/j.marstruc.2022.103177
Zhang, Y., Hu, Z., Ng, C., Jia, C., and Jiang, Z. (2021). Dynamic responses analysis of a 5 MW spar-type floating wind turbine under accidental ship-impact scenario. Mar. Struct. 75, 102885. doi:10.1016/j.marstruc.2020.102885
Keywords: offshore wind energy, offshore wind turbines, ship collision, dynamic response, protection measures
Citation: Ma L, Ding J, Zhang X, Wang W, Zhao X, Sun C and Yang Y (2024) Analysis of dynamic response of offshore wind turbines subjected to ship impacts and the corresponding protection measures: a review. Front. Energy Res. 12:1497210. doi: 10.3389/fenrg.2024.1497210
Received: 16 September 2024; Accepted: 16 October 2024;
Published: 14 November 2024.
Edited by:
Renwei Ji, Jiangsu University of Science and Technology, ChinaReviewed by:
Zhiyang Zhang, Jiangsu Ocean Universiity, ChinaRay-Yeng Yang, National Cheng Kung University, Taiwan
Xin Shen, Shanghai Jiao Tong University, China
Copyright © 2024 Ma, Ding, Zhang, Wang, Zhao, Sun and Yang. This is an open-access article distributed under the terms of the Creative Commons Attribution License (CC BY). The use, distribution or reproduction in other forums is permitted, provided the original author(s) and the copyright owner(s) are credited and that the original publication in this journal is cited, in accordance with accepted academic practice. No use, distribution or reproduction is permitted which does not comply with these terms.
*Correspondence: Yang Yang, eWFuZ3lhbmcxQG5idS5lZHUuY24=