- Biotechnology Research Institute, Universiti Malaysia Sabah, Kota Kinabalu, Sabah, Malaysia
Microalgae are considered valuable bioresources due to their ability to produce high lipid content and grow under a variety of environmental conditions, making them strong candidates for sustainable biofuel production. However, the economic feasibility of microalgae-based biofuels depends on optimizing growth conditions in large-scale cultivation systems. This study investigates the effects of varying inoculum cell concentrations on the growth, lipid yield, and fatty acid composition of the locally isolated microalga Chlorella sp. SW5 in 2 L and 5 L cultivation systems. The results indicate that higher inoculum concentrations generally enhance biomass accumulation, with the 2 L system achieving the highest growth rate of 0.42 ± 0.01 day⁻1 at an inoculum concentration of 10⁶ cells/mL. Interestingly, while higher inoculum concentrations reduced lipid production in the 2 L system, the 5 L system showed the highest lipid yield (51.23% ± 4.71% dry weight) at the highest inoculum concentration (10⁷ cells/mL). Despite its moderate growth rate, the 5 L culture with a starting inoculum concentration of 10⁷ cells/mL was selected for fatty acid profiling due to its superior lipid yield and productivity. Gas chromatography-mass spectrometry (GC-MS) analysis revealed that the culture produced a total of 93.18% C14-C18 fatty acids, with a profile dominated by saturated (56.33%) and monounsaturated (16.85%) fatty acids, which are essential for biodiesel quality. These findings provide valuable insights into the potential for scaling up microalgal systems for commercial biofuel production, highlighting strategies to optimize productivity.
1 Introduction
The increasing scarcity of fossil fuel resources and rising levels of greenhouse gas emissions from fossil fuel combustion have driven research toward alternative, biomass-derived energy sources. Biomass, including agricultural crops such as corn, soybeans, sugarcane, switchgrass, and woody plants, as well as algae and waste materials like agricultural residues, has become a focal point in the quest for sustainable biofuel production (Antar et al., 2021). According to the International Energy Agency (IEA), global biodiesel production has experienced remarkable growth, expanding from 806 million liters in 2000 to 21,400 million liters in 2011, and reaching approximately 80,000 million liters in 2020 (Hossain et al., 2020). The yield of biodiesel varies significantly depending on the feedstock used, with microalgae standing out for their exceptional productivity. Microalgae have the potential to produce between 5,000 and 15,000 gallons of biodiesel per acre annually, far surpassing the biodiesel output of other feedstocks (Griffiths et al., 2021).
Microalgae present numerous advantages as a biofuel feedstock, including high lipid content, rapid growth, and efficient carbon dioxide capture. Their cultivation can be carried out on non-arable land, particularly in rural areas where costs are lower, making them a viable and sustainable solution (Chin et al., 2023). As photosynthetic organisms, microalgae thrive in both marine and freshwater environments, and their photosynthetic process is more efficient than that of traditional biofuel feedstocks due to their simple cellular structure, high surface-to-volume ratio, and aquatic habitat. This combination enables microalgae to convert greater quantities of water, carbon dioxide, and nutrients into biomass (Chhandama et al., 2021). Microalgae can double their biomass every two to 5 days due to their rapid growth, and their lipid content ranges from 5% to 68% of their dry mass, depending on the species. As a third-generation biofuel feedstock, microalgae offer a significant advantage over other sources since they do not contain lignin, eliminating the need for the costly delignification processes commonly required in biodiesel production from other biomass feedstocks (Khoo et al., 2023).
Several species of microalgae, including Botryococcus braunii (Thurakit et al., 2022), Nannochloropsis sp (Palanisamy et al., 2023), Dunaliella salina (de Souza Celente et al., 2022), Chlorella vulgaris (Carino and Vital, 2022), and Scenedesmus obliquus (Trivedi et al., 2022), are known for their high production of hydrocarbons and lipids, making them excellent candidates for biodiesel production. Among them, members of the genus Chlorella, particularly C. vulgaris, are highly valued for biodiesel production and biomass recovery due to their rapid growth rate, high photosynthetic efficiency, and significant oil content (Abreu et al., 2023). One of the distinctive features of C. vulgaris is its ability to thrive in both freshwater and seawater environments. The use of marine Chlorella strains is especially appealing given the growing demand for fresh water, which is projected to increase by approximately 30% by 2050 (Gu et al., 2023). Marine Chlorella strains offer a unique advantage as they can be cultivated on non-arable land using saline water supplies. A study by Rautenberger et al. (2024) demonstrated that C. vulgaris cultivated in a medium with 50% seawater and 50% freshwater under mixotrophic conditions achieved optimal growth and lipid content, with lipids comprising 49% of its dry weight. The lipids were predominantly composed of palmitoleic and oleic acids, highlighting the potential for more sustainable biomass and lipid production. These attributes make marine Chlorella sp. an ideal candidate for large-scale biofuel production, further enhancing its commercial potential across various industries.
In previous studies, the primary focus of optimizing microalgal cultures has been to enhance both the quantity and quality of lipid production (Chin et al., 2023; Justine et al., 2023; Andrew et al., 2022; Ibrahim et al., 2022; Sani et al., 2021; Andrew et al., 2013). In this study, the optimization of growth conditions aims to maximize the overall productivity and efficiency of microalgae cultivation in larger laboratory-scale cultivation systems, with inoculum concentration being a key factor. Inoculum concentration plays a critical role in the cultivation of microalgae, influencing various aspects of growth and productivity (Bohutskyi et al., 2016). The concentration of inoculum directly affects the duration of the lag phase, growth rate, final biomass yield, and overall metabolite production. Lower inoculum concentrations can lead to extended lag phases and reduced growth rates due to the limited number of cells available for reproduction and nutrient metabolism. Conversely, higher inoculum concentrations typically shorten the lag phase and accelerate growth by providing a larger initial population of cells for reproduction and nutrient uptake (Zhang et al., 2017). However, excessively high inoculum concentrations can result in competition for light and nutrients, ultimately limiting cell growth and productivity (Bohutskyi et al., 2016). Therefore, optimizing inoculum concentration is essential for achieving maximum biomass yield and efficient resource utilization during microalgae cultivation.
Expanding microalgae cultivation from laboratory settings to commercial scale presents significant challenges, particularly in adapting operational conditions to larger systems (Mutanda et al., 2020). A gradual transition from small-scale to larger volumes is crucial to mitigate these challenges. Typically, scaling up is carried out in increments of 10, progressing from 10 mL to 100 mL, then to 1 L, 10 L, and beyond (Borowitzka and Vonshak, 2017). Producing the inoculum for large-scale commercial cultures is a critical and complex step that directly impacts the success of the cultivation process. This study aims to determine the optimal inoculum concentration for maximizing biomass growth and lipid production in 2 L and 5 L cultures of the marine microalga Chlorella sp., a locally isolated strain with significant potential for biofuel applications. The findings from this study are expected to provide valuable insights for enhancing microalgal biofuel production on an industrial scale.
2 Materials and methods
2.1 Microalga isolation and culture conditions
The marine microalga, Chlorella sp. SW5, was isolated from a seawater sample collected at the Borneo Marine Research Institute Fish Hatchery, Universiti Malaysia Sabah (6.03916, 116.11284). The microalga was successfully established in Walne’s medium (Walne, 1970) and identified as Chlorella sp. based on the 18S ribosomal RNA gene using the primer pair, 18SCOMF1 (5′-GCTTGTCTCAAA GATTAAGCCATGC-3′) and 18SCOMR1 (5′- CACCTACGG AAACCTTGTTACGAC-3′) (Zhang et al., 2005). The DNA sequence was subsequently deposited in the NCBI GenBank (Acc. no.: PQ579654). The microscopic image of the microalga Chlorella sp. is shown in Figure 1. The Chlorella sp. cultures were maintained at a temperature range of 23°C–25°C, a 16:8 light/dark photoperiod, and a light intensity of 135 μmol/m2/s (Chin et al., 2023) provided by 40W cool-white fluorescent light (AQUA-GLO, Japan). All experiments were conducted in triplicate (n = 3) in 2 L and 5 L culture volumes.
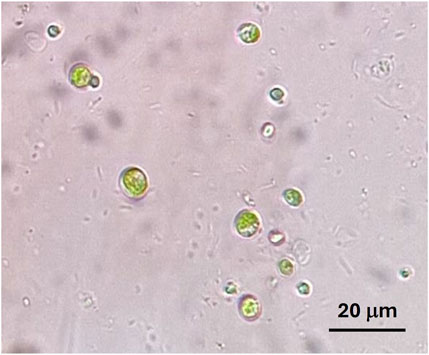
Figure 1. Microscopic image of the microalga Chlorella sp, showing its characteristic round shape and green pigmentation.
2.2 Inoculum concentrations in 2-liter and 5-liter culture medium
The initial inoculum concentrations were set at five levels (103, 104, 105, 106, and 107 cells/mL) for the 2 L and 5 L laboratory-scale culture systems. Inoculum concentrations were determined by cell counting using a hemocytometer. The microalgal cell inoculum were added into the media in order to achieve the desired five initial cell concentrations (103, 104, 105, 106, and 107 cells/mL). The culture conditions for this experiment were similar with the maintenance conditions, with each culture flask was aerated using an air pump to promote cell growth. In addition, the nitrate concentration in the Walne’s medium was maintained at 5 g/L for this experiment (Chin et al., 2023).
2.3 Growth profile determination
The growth profile of the isolated Chlorella sp. was monitored by cell counting every 3 days until cell death was observed, using a hemocytometer (Sambrook and Russell, 2001). For cell suspension preparation, 100 µL of microalgal culture were diluted with 400 µL of Trypan Blue. Approximately 10 µL of the diluted culture were carefully dispensed onto both chambers of the hemocytometer under the coverslip. After a 5-minute incubation, cells were counted on both sides of the hemocytometer under a light microscope. The cell density (cells/mL) was calculated using Equation 1 and used to generate a growth curve. Additionally, the specific growth rate (µ) was determined using Equation 2.
Where N1 and N2 represent the biomass at time t1 (start point of cultivation) and t2 (end point of cultivation), respectively.
2.4 Lipid content and lipid productivity determination
Lipid content was determined using a method described by Bligh and Dyer (1959). Microalgal cultures were harvested weekly for lipid analysis. A total of 50 mL of microalgal culture was collected into a pre-weighed tube and centrifuged. The resulting pellet was dried in an oven until a constant weight was achieved, then ground into a fine powder. A mixture of chloroform, methanol, and distilled water (1:1:1 ratio) was added to the tube, with each solvent measuring 1 mL. The mixture was vortexed and left to stand at room temperature for 1 h. The sample was then centrifuged at 5,500 ×g for 5 min, resulting in the formation of two layers. The top layer, containing methanol and water, was discarded, while the bottom layer, containing chloroform and lipids, was transferred to a pre-weighed microcentrifuge tube. Methanol was completely removed using a vacuum rotary evaporator (V-AQ mode) for 1.5 h. The extracted lipid was then weighed, and the lipid yield and lipid productivity were calculated using Equation 3 (Cordeiro et al., 2017) and Equation 4 (Nascimento et al., 2013), respectively.
2.5 Fatty acid methyl esters (FAMEs) composition determination
Only Chlorella sp. culture with the inoculum concentration that produced the highest lipid yield was selected for FAMEs composition analysis. Triplicate samples were sent to the UNIPEQ Holdings Sdn. Bhd., National University of Malaysia, for detailed FAME analysis. Total fat extraction was performed using a Soxhlet apparatus according to the AOAC 20th Edition (method 991.36). Fatty acid analysis was carried out using Gas Chromatography with Flame Ionization Detection (GC-FID) based on the AOAC 20th Edition: method 996.06.
2.6 Data analysis
All the experiments were conducted in triplicate, and the results were presented as means with standard deviations. Statistical analyses were conducted using SPSS software (version 21.0). Analysis of variance (ANOVA) was employed to assess the experiments’ data, and correlation analyses were used to determine the relationships between specific growth rate, lipid content, and lipid productivity with the different inoculum concentrations in the 2-liter and 5-liter cultivation systems. A p-value of <0.05 was considered statistically significant for all tests.
3 Results and discussion
Scaling up microalgae cultivation from small-scale to mass production presents significant challenges, as microalgae naturally thrive in low-density environments (Qin et al., 2023). Several factors, including the species of microalgae, culture conditions, types of cultivation, metabolic pathways, and the type of culture vessels, play critical roles in successful large-scale cultivation (Novoveská et al., 2023). In this study, the culture volumes of Chlorella sp. were increased from 250 mL to 2,000 and 5,000 mL, utilizing optimized culture conditions from previous research (Chin et al., 2023; Andrew et al., 2022). The microalgae’s responses in terms of growth rate, lipid content, lipid productivity, and fatty acid profile were comprehensively analyzed to assess the effects of this volume expansion.
The growth curves and growth rates of Chlorella sp. in 2 L and 5 L culture media with different inoculum concentrations are depicted in Figure 2A, B and summarized in Table 1, Table 2, respectively. The effect of inoculum concentrations on specific growth rate was statistically significant in both 2 L (F = 155.90, p < 0.05) and 5 L (F = 17.01, p < 0.05) culture volumes. The results indicate that higher inoculum concentrations generally enhanced biomass accumulation in both culture volumes. Notably, the growth rates were higher in the 2 L culture system, ranging from 0.23 to 0.42 day-1 (Table 1), compared to the 5 L system, which exhibited growth rates between 0.11 and 0.21 day-1 (Table 2). The highest growth rate (0.42 day-1) was observed in the 2 L culture system with an inoculum concentration of 106 cells/mL, while the lowest growth rate (0.11 day-1) occurred in the 5 L system with a 104 cells/mL inoculum concentration. According to Figures 2A,B, all cultures transitioned almost immediately into the exponential growth phase, experiencing a very short lag phase of only one to 2 days, due to the high starting inoculum. The exponential phase lasted approximately 40–45 days for all cultures before entering the stationary phase for about 10 days. However, the 2 L culture with a 103 cells/mL inoculum concentration continued its exponential growth phase until day 60. Subsequently, all cultures transitioned into death phase.
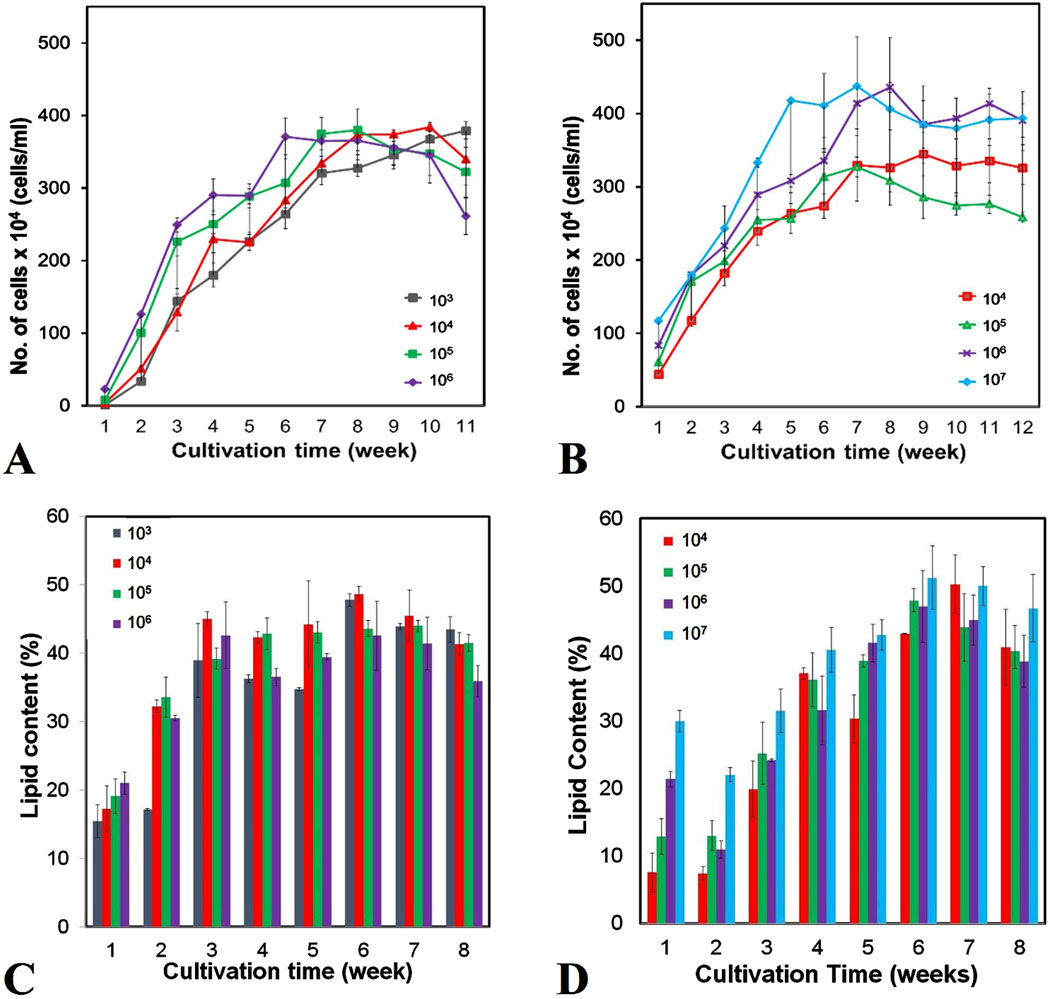
Figure 2. Growth curves (A, B) and weekly lipid content (C, D) of Chlorella sp cultured at different initial inoculum concentrations in 2-liter (A, C) and 5-liter (B, D) systems. Error bars represent the standard deviation of the mean (n = 3).
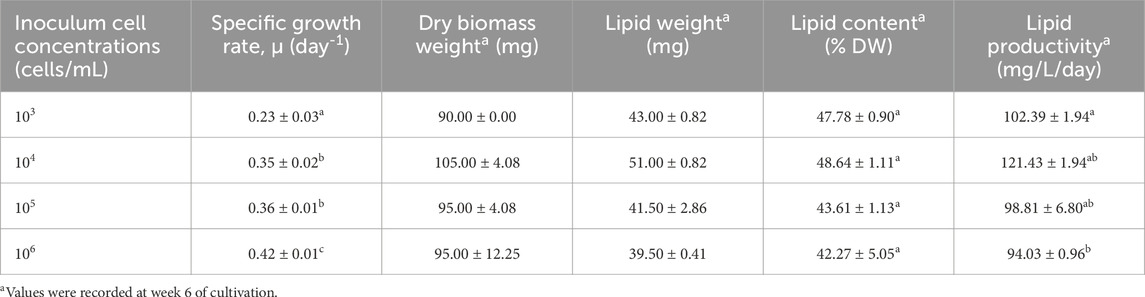
Table 1. Specific growth rate, lipid content, and lipid productivity of Chlorella sp. cultures at different inoculum concentrations in 2-liter cultivation system. Means (±SD) with different superscripts in the same column are significantly different.
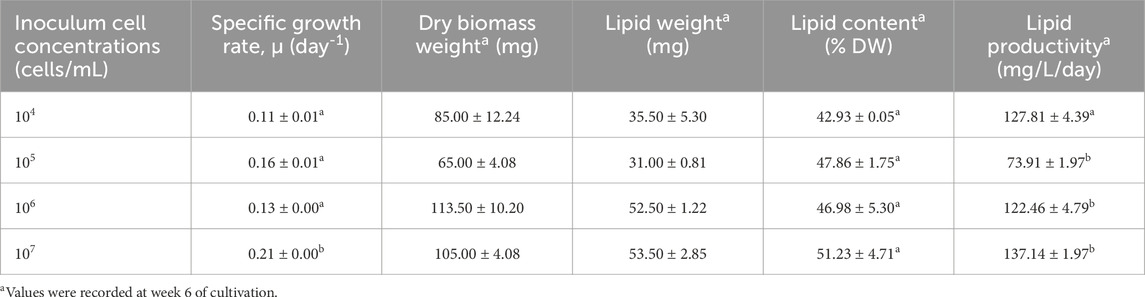
Table 2. Specific growth rate, lipid content, and lipid productivity of Chlorella sp. cultures at different inoculum concentrations in 5-liter cultivation system. Means (±SD) with different superscripts in the same column are significantly different.
Generally, inoculum size directly influences the number of microalgal cells available for replication, thereby affecting overall biomass production. A well-chosen inoculum size enables microalgae to rapidly establish a competitive advantage and adapt to their environment (Bohutskyi et al., 2016). However, excessively large inoculum sizes can introduce growth-limiting stresses due to nutrient depletion and light limitations. Cheng et al. (2018) observed that excessive inoculum sizes may lead to rapid cell growth, increased culture viscosity, and reduced dissolved oxygen levels, which can negatively impact metabolite synthesis. Therefore, selecting an optimal inoculum concentration range is crucial for minimizing retention time, enhancing nutrient recovery, and improving daily biomass productivity (Zhang et al., 2017).
The weekly lipid content and productivity of Chlorella sp. cultivated in 2 L and 5 L culture media with varying inoculum concentrations are presented in Figures 2C,D and summarized in Table 1, Table 2. Statistical analysis revealed that the effect of inoculum concentrations on lipid productivity was significant in both the 2 L (F = 7.03, p < 0.05) and 5 L (F = 22.67, p < 0.05) cultivation systems. However, there were no significant differences in lipid content across inoculum sizes in either the 2 L (F = 0.835, p > 0.05) or 5 L (F = 0.581, p > 0.05) systems. The results indicate that the Chlorella sp. isolate accumulated lipids in concentrations ranging from 42.27% to 51.23% dry weight (DW) and achieved lipid productivity between 73.91 and 137.81 mg/L/day at week 6 of cultivation (Table 1, 2). The highest lipid content (51.23% DW) and lipid productivity (137.81 mg/L/day) were observed in the 5 L culture system with an inoculum concentration of 10⁷ cells/mL. In contrast, the lowest lipid content (42.27% DW) was recorded in the 2 L system with inoculum concentration of 106 cells/mL, while the lowest lipid productivity (73.91 mg/L/day) occurred in the 5 L system with an inoculum concentration of 10⁵ cells/mL. As shown in Figures 2C,D, lipid accumulation peaked between weeks 6 and 7 (42.27–51.23 %DW) in both cultivation systems. These findings suggest that the optimal harvest period is between weeks 6 and 7, coinciding with the late exponential growth phase, where biomass reaches its maximum.
The lipid content of Chlorella sp. strains generally vary between 5% and 58%, depending on the specific strain and environmental conditions (Ru et al., 2020). In this study, Chlorella sp. exhibited lipid contents ranging from 42.27% to 51.23% DW in both the 2 L (Table 1) and 5 L (Table 2) cultivation systems. The elevated lipid content observed in this study can be attributed to a combination of nutrient stress, specifically limited nitrogen availability, and higher light intensity (135 μmol/m2/s). The optimized culture conditions of nitrogen-limited media and high light intensity were adopted from a previous study by Chin et al. (2023), where increased lipid yield was observed in four microalgae species cultured in 5 L media. These conditions, including reduced nitrogen and high light intensity, have been shown to promote lipid accumulation by inducing metabolic shifts in the microalgae.
The nitrogen content in the media was reduced by preparing a nutrient stock with 5 g/L of NaNO₃, as described by Chin et al. (2023), in contrast to the original NaNO₃ concentration of 100 g/L. Under nitrogen-deficient conditions, algae undergo metabolic shifts, which promote the accumulation of lipids and starch in their biomass (Yaakob et al., 2021). Similarly, a study by Ratomski and Hawrot-Paw (2021) observed a two-fold increase in lipid content in C. vulgaris when cultured in nitrogen-deficient aquaculture waste. Griffiths et al. (2014) also highlighted the strong correlation between nitrogen limitation and increased lipid accumulation in C. vulgaris. Under nitrogen-replete conditions, the lipid content remained stable at 10%-12% throughout the growth period. However, when nitrogen availability was limited, C. vulgaris cultures exhibited a substantial rise in lipid content, reaching 50%–65% dry weight, depending on the initial nitrate concentration and the severity of nitrogen depletion (Griffiths et al., 2014).
In this study, Chlorella sp. was cultivated under a higher light intensity of 135 μmol/m2/s (Chin et al., 2023), compared to the typical 68 μmol/m2/s used in previous studies (Justine et al., 2023; Andrew et al., 2022; Ibrahim et al., 2022). Light intensity plays a crucial role in photosynthesis and lipid production, with higher intensities favoring the accumulation of neutral storage lipids, particularly triacylglycerols, while lower intensities promote the formation of polar lipids associated with chloroplast membranes (Wacker et al., 2016). Studies on microalgae such as Scenedesmus sp. have shown that increasing light intensity not only enhances lipid content but also boosts the production of key fatty acids, particularly C16 and C18 (He et al., 2015). Furthermore, a recent study by Liao et al. (2018) demonstrated that optimizing light and nutrient conditions during different growth phases of C. vulgaris can significantly increase lipid productivity, achieving a maximum of 163.42 mg/L/day in the stationary phase under a light intensity of 180 μmol/m2/s and nutrient-starvation conditions.
The results in Table 1, Table 2 reveal that the cultivation systems with the highest growth rates did not correspond to the highest lipid yields or productivities. Notably, the 5 L system with an initial inoculum of 107 cells/mL achieved the highest lipid content (51.23% DW) and productivity (137.81 mg/L/day), despite exhibiting only a moderate growth rate (0.21 day-1). Previous studies have shown an inverse relationship between biomass and lipid yield, highlighting the impact of specific culture conditions and nutrient availability on this relationship. This inverse correlation has been observed in species such as Chlorophyta sorokiniana (Griffiths and Harrison, 2009) and Chlorella sp. (Cho et al., 2020). A high specific growth rate in microalgae does not necessarily correlate with enhanced lipid production, as lipid accumulation primarily occurs during the stationary phase when biosynthesis shifts towards lipid storage. Therefore, lipid productivity and qualitative lipid composition are more critical parameters than growth rate for selecting species suitable for biodiesel production (Nascimento et al., 2013). The cultivation system with the highest lipid productivity in this study was the 5 L system with the starting inoculum of 107 cells/mL (137.81 mg/L/day), coinciding also producing the highest lipid content (51.23% DW). Given its superior lipid productivity and content, the 5 L system with 107 cells/mL inoculum was selected for further fatty acid methyl esters (FAMEs) analysis.
Table 3 provides a detailed analysis of the fatty acid compositions of Chlorella sp. cultivated in a 5-liter system with an initial inoculum concentration of 107 cells/mL. A total of 37 fatty acids (FAs) were analyzed, comprising saturated fatty acids (butyric, caproic, caprylic, capric, undecanoic, lauric, tridecanoic, myristic, pentadecanoic, palmitic, heptadecanoic, stearic, arachidic, henicosanoic, behenic, tricosanoic, and lignoceric acids), monounsaturated fatty acids (myristoleic, cis-10-pentadecenoic, palmitoleic, cis-10-heptadecanoic, elaidic, oleic, cis-11-eicosenoic, erucic, and nervonic acids), and polyunsaturated fatty acids (linolelaidic, linoleic, γ-linolenic, α-linolenic, cis-11,14-eicosadienoic, cis-8,11,14-eicosatrienoic, cis-11,14,17-eicosatrienoic, arachidonic, cis-5,8,11,14,17-eicosapentaenoic, cis-13,16-docosadienoic, and cis-4,7,10,13,16,19-docosahexaenoic acids). The FAs that significantly influence biofuel quality include C16 and C18 FAs, particularly palmitic acid (C16:0), stearic acid (C18:0), oleic acid (C18:1), linoleic acid (C18:2), and linolenic acid (C18:3) (Bharti et al., 2024). As detailed in Table 3, Chlorella sp. predominantly produced these candidate fatty acids, with palmitic acid (C16:0) comprising 39.46%, linoleic acid (C18:2) at 23.24%, oleic acid (C18:1) at 14.01%, stearic acid (C18:0) at 9.68%, and α-linolenic acid (C18:3) at 3.26%. These FAs are particularly preferred for biofuel production due to their favorable properties, such as oxidative stability and energy content (Khethiwe et al., 2020).
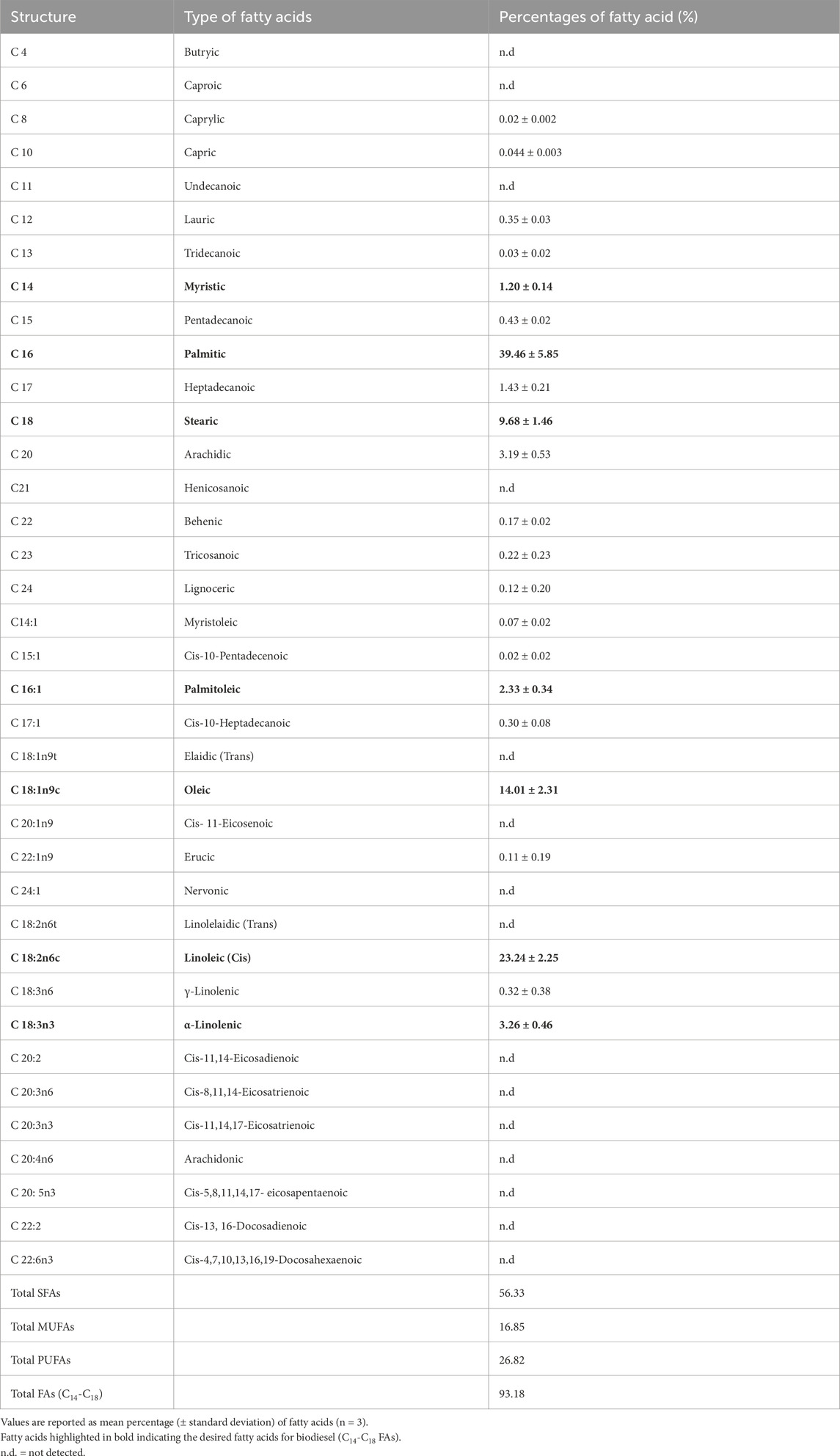
Table 3. Fatty acid profile of Chlorella sp. in 5-liter cultivation system, with starting inoculum concentration of 107 cells/mL.
Another fatty acid worth noting for biofuel feedstocks is oleic acid (C18:1). This omega-9 monounsaturated fatty acid is a crucial component due to its multiple applications across various industries, including food, health, and chemicals. High oleic acid content is particularly beneficial for biofuel production, as it enhances the cold-flow properties of biodiesel and is advantageous for hydro-conversion processes in bio-jet fuel production (Choi et al., 2023). The presence of oleic acid in microbial oils contributes significantly to improving the quality and efficiency of biofuels. In this study, Chlorella sp. produced a moderate level of oleic acid at 14.01%. A similar finding was reported by Kreft et al. (2020), where C. vulgaris cultured in Bold’s Basal Medium (BBM) produced 18% oleic acid under both autotrophic and heterotrophic conditions. There is ongoing research focused on enhancing oleic acid production in microalgae, particularly with the goal of achieving more than 50% oleic acid content. This is especially relevant in the development of algae-based edible oils, where higher oleic acid levels can significantly improve the quality and commercial viability of the oils (Kona et al., 2022).
Additionally, Chlorella sp. demonstrated a fatty acid profile highly favorable for biodiesel production, with 93.18% of its fatty acids falling within the C14-C18 range, which is considered ideal for biofuel. The fatty acid composition included a relatively low percentage of polyunsaturated fatty acids (PUFAs) at 26.82%, balanced by an optimal concentration of saturated fatty acids (SFAs) at 56.33% and monounsaturated fatty acids (MUFAs) at 16.85%. This balance is crucial, as SFAs contribute to good oxidation resistance for biofuel (Longanesi et al., 2022), while the reduced PUFA content and moderate MUFA levels provide the necessary fuel properties, such as oxidative stability and cold flow characteristics, for efficient biodiesel performance (Kumbhar et al., 2022). When compared to previous studies on locally isolated microalgae Chaetoceros muelleri and Isochrysis galbana cultured under similar conditions (Chin et al., 2023), Chlorella sp. exhibited higher levels of SFAs and PUFAs, although MUFA levels were lower. Despite this, the overall FA composition remains desirable for biofuel production, ensuring the robustness of the biodiesel derived from Chlorella sp.
4 Conclusion
This study successfully evaluated the growth, lipid content, and lipid productivity of Chlorella sp. in 2-liter and 5-liter cultures, using a locally isolated strain previously identified for its biodiesel potential. The study identified optimal inoculum concentrations, which resulted in satisfactory lipid yields, with growth rates ranging from 0.11 to 0.42 day-1, lipid content between 42.27% and 51.23%, and lipid productivity from 73.91 to 137.81 mg/L/day. The 5-liter cultivation system, with an initial inoculum concentration of 10⁷ cells/mL, emerged as particularly promising for biodiesel production, exhibiting the ideal fatty acid profile and balance of SFAs, MUFAs, and PUFAs for biofuel feedstock.
Data availability statement
This manuscript does not involve any research on animals, and therefore, no ethical approval was required for the study.
Ethics statement
The manuscript presents research on animals that do not require ethical approval for their study.
Author contributions
EA-S: Formal Analysis, Investigation, Methodology, Writing–original draft, Writing–review and editing. GC: Conceptualization, Formal Analysis, Investigation, Supervision, Writing–original draft, Writing–review and editing. WY: Conceptualization, Data curation, Funding acquisition, Project administration, Supervision, Validation, Writing–review and editing. MM: Conceptualization, Data curation, Supervision, Validation, Writing–review and editing.
Funding
The author(s) declare that financial support was received for the research, authorship, and/or publication of this article. This research was funded by Universiti Malaysia Sabah through the Priority Field Research Grant Scheme (SBK0385-2018).
Acknowledgments
The authors gratefully acknowledge the administrative assistance and financial support from Universiti Malaysia Sabah through the Priority Field Research Grant Scheme (SBK0385-2018).
Conflict of interest
The authors declare that the research was conducted in the absence of any commercial or financial relationships that could be construed as a potential conflict of interest.
Publisher’s note
All claims expressed in this article are solely those of the authors and do not necessarily represent those of their affiliated organizations, or those of the publisher, the editors and the reviewers. Any product that may be evaluated in this article, or claim that may be made by its manufacturer, is not guaranteed or endorsed by the publisher.
References
Abreu, A. P., Martins, R., and Nunes, J. (2023). Emerging applications of Chlorella sp. and spirulina (arthrospira) sp. Bioeng. 10 (8), 955. doi:10.3390/bioengineering10080955
Andrew, A. R., Anton, A., and Chin, G. J. W. L. (2013). Local bioprospecting of lipid producing microalgal strains for biofuel production. Malays. J. Sci. 32 (SCS Sp Issue), 301–308.
Andrew, A. R., Yong, W. T. L., Misson, M., Anton, A., and Chin, G. J. W. L. (2022). Selection of tropical microalgae species for mass production based on lipid and fatty acid profiles. Front. Energy Res. 10, 912904. doi:10.3389/fenrg.2022.912904
Antar, M., Lyu, D., Nazari, M., Shah, A., Zhou, X., and Smith, D. L. (2021). Biomass for a sustainable bioeconomy: an overview of world biomass production and utilization. Renew. Sustain. Energy Rev. 139, 110691. doi:10.1016/j.rser.2020.110691
Bharti, R. K., Kaushal, C., Singh, A., Dhar, D. W., Babu, R., and Kaushik, A. (2024). Evaluation of fuel properties for possible biodiesel output based on the fatty acid composition of oleaginous plants and microalgae. Sci.Total Environ. 918, 170448. doi:10.1016/j.scitotenv.2024.170448
Bligh, E. G., and Dyer, W. J. (1959). A rapid method of total lipid extraction and purification. Can. J. Biochem. Physiol. 37, 911–917. doi:10.1139/o59-099
Bohutskyi, P., Kligerman, D. C., Byers, N., Nasr, L. K., Cua, C., Chow, S., et al. (2016). Effects of inoculum size, light intensity, and dose of anaerobic digestion centrate on growth and productivity of Chlorella and Scenedesmus microalgae and their poly-culture in primary and secondary wastewater. Algal Res. 19, 278–290. doi:10.1016/j.algal.2016.09.010
Borowitzka, M. A., and Vonshak, A. (2017). Scaling up microalgal cultures to commercial scale. Eur. J. Phycol. 52, 407–418. doi:10.1080/09670262.2017.1365177
Carino, J. D., and Vital, P. G. (2022). Characterization of isolated UV-C-irradiated mutants of microalga Chlorella vulgaris for future biofuel application. Environ. Dev. Sustain. 25, 1258–1275. doi:10.1007/s10668-021-02091-8
Cheng, P., Wang, Y., Osei-Wusu, D., Liu, T., and Liu, D. (2018). Effects of seed age, inoculum density, and culture conditions on growth and hydrocarbon accumulation of Botryococcus braunii SAG807-1 with attached culture. Bioresour. Bioprocess. 5, 15. doi:10.1186/s40643-018-0198-4
Chhandama, M. V. L., Satyan, K. B., Changmai, B., Vanlalveni, C., and Rokhum, S. L. (2021). Microalgae as a feedstock for the production of biodiesel: a review. Bioresour. Technol. Rep. 15, 100771. doi:10.1016/j.biteb.2021.100771
Chin, G. J. W. L., Andrew, A. R., Abdul-Sani, E. R., Yong, W. T. L., Misson, M., and Anton, A. (2023). The effects of light intensity and nitrogen concentration to enhance lipid production in four tropical microalgae. Biocatal. Agric. Biotechnol. 48, 102660. doi:10.1016/j.bcab.2023.102660
Cho, J. M., Oh, Y. K., Park, W. K., and Chang, Y. K. (2020). Effects of nitrogen supplementation status on CO2 biofixation and biofuel production of the promising microalga Chlorella sp. ABC-001. J. Microbiol. Biotechnol. 30 (8), 1235–1243. doi:10.4014/jmb.2005.05039
Choi, Y., Son, H. F., Hwang, S., Kim, J., Ko, J. K., Gong, G., et al. (2023). Hexanoic acid improves the production of lipid and oleic acid in Yarrowia lipolytica: the benefit of integrating biorefinery with organic waste management. Environ. Technol. Inno. 31, 103168. doi:10.1016/j.eti.2023.103168
Cordeiro, R. S., Vaz, I. C., Magalhães, S. M., and Barbosa, F. A. (2017). Effects of nutritional conditions on lipid production by cyanobacteria. Acad. Bras. Cienc. 89 (3), 2021–2031. doi:10.1590/0001-3765201720150707
de Souza Celente, G., Rizzetti, T. M., Sui, Y., and de Souza Schneider, R. D. C. (2022). Potential use of microalga Dunaliella salina for bioproducts with industrial relevance. Biomass Bioenergy 167, 106647. doi:10.1016/j.biombioe.2022.106647
Griffiths, G., Hossain, A. K., Sharma, V., and Duraisamy, G. (2021). Key targets for improving algal biofuel production. Clean. Technol. 3 (4), 711–742. doi:10.3390/cleantechnol3040043
Griffiths, M. J., and Harrison, S. T. (2009). Lipid productivity as a key characteristic for choosing algal species for biodiesel production. J. Appl. Phycol. 21, 493–507. doi:10.1007/s10811-008-9392-7
Griffiths, M. J., Van Hille, R. P., and Harrison, S. T. (2014). The effect of nitrogen limitation on lipid productivity and cell composition in Chlorella vulgaris. Appl. Microbiol. Biotechnol. 98, 2345–2356. doi:10.1007/s00253-013-5442-4
Gu, X., Deng, Y., Wang, A., Gan, Q., Xin, Y., Paithoonrangsarid, K., et al. (2023). Engineering a marine microalga Chlorella sp. as the cell factory. Biotechnol. Biofuels Bioprod. 16, 133. doi:10.1186/s13068-023-02384-2
He, Q., Yang, H., Wu, L., and Hu, C. (2015). Effect of light intensity on physiological changes, carbon allocation and neutral lipid accumulation in oleaginous microalgae. Bioresour. Technol. 191, 219–228. doi:10.1016/j.biortech.2015.05.021
Hossain, N., Hasan, M. H., Mahlia, T. M. I., Shamsuddin, A. H., and Silitonga, A. S. (2020). Feasibility of microalgae as feedstock for alternative fuel in Malaysia: a review. Energy Strategy Rev. 32, 100536. doi:10.1016/j.esr.2020.100536
Ibrahim, S., Chin, G. J. W. L., and Misson, M. (2022). Enhanced microbial biomass and lipid production through co-cultivation of yeast Rhodotorula toruloides and microalga Chaetoceros muelleri. Malays. J. Microbiol. 18 (5), 463–472. doi:10.21161/mjm.211333
Justine, I., Chin, G. J. W. L., Yong, W. T. L., and Misson, M. (2023). Characterization and optimization of Rhodotorula toruloides and Ankistrodesmus falcatus co-culture in palm oil mill effluent for efficient COD removal and lipid production. Biocatal. Agric. Biotechnol. 51, 102782. doi:10.1016/j.bcab.2023.102782
Khethiwe, E., Clever, K., and Jerekias, G. (2020). Effects of fatty acids composition on fuel properties of Jatropha curcas biodiesel. Smart Grid Renew. Energy 11, 165–180. doi:10.4236/sgre.2020.1110010
Khoo, K. S., Ahmad, I., Chew, K. W., Iwamoto, K., Bhatnagar, A., and Show, P. L. (2023). Enhanced microalgal lipid production for biofuel using different strategies including genetic modification of microalgae: a review. Prog. Energy Combust. Sci. 96, 101071. doi:10.1016/j.pecs.2023.101071
Kona, R., Katakojwala, R., Pallerla, P., Sripadi, P., and Mohan, S. V. (2022). High oleic acid biosynthesis and its absolute quantification by GC/MS in oleaginous Scenedesmus sp. SVMIICT1 cultivated in dual stress phase. Algal Res. 67, 102865. doi:10.1016/j.algal.2022.102865
Kreft, J., Moe, E., Garcia, N., Ross, A., and Seames, W. (2020). Comparative scoping study report for the extraction of microalgae oils from two subspecies of Chlorella vulgaris. Clean. Energy 4 (3), 233–246. doi:10.1093/ce/zkaa009
Kumbhar, V., Pandey, A., Sonawane, C. R., El-Shafay, A. S., Panchal, H., and Chamkha, A. J. (2022). Statistical analysis on prediction of biodiesel properties from its fatty acid composition. Case Stud. Therm. Eng. 30, 101775. doi:10.1016/j.csite.2022.101775
Liao, Q., Chang, H. X., Fu, Q., Huang, Y., Xia, A., Zhu, X., et al. (2018). Physiological-phased kinetic characteristics of microalgae Chlorella vulgaris growth and lipid synthesis considering synergistic effects of light, carbon and nutrients. Bioresour. Technol. 250, 583–590. doi:10.1016/j.biortech.2017.11.086
Longanesi, L., Pereira, A. P., Johnston, N., and Chuck, C. J. (2022). Oxidative stability of biodiesel: recent insights. Biofuels Bioprod. Biorefin. 16 (1), 265–289. doi:10.1002/bbb.2306
Mutanda, T., Naidoo, D., Bwapwa, J. K., and Anandraj, A. (2020). Biotechnological applications of microalgal oleaginous compounds: current trends on microalgal bioprocessing of products. Front. Energy Res. 8, 598803. doi:10.3389/fenrg.2020.598803
Nascimento, I. A., Marques, S. S. I., Cabanelas, I. T. D., Pereira, S. A., Druzian, J. I., de Souza, C. O., et al. (2013). Screening microalgae strains for biodiesel production: lipid productivity and estimation of fuel quality based on fatty acids profiles as selective criteria. Bioenergy Res. 6, 1–13. doi:10.1007/s12155-012-9222-2
Novoveská, L., Nielsen, S. L., Eroldoğan, O. T., Haznedaroglu, B. Z., Rinkevich, B., Fazi, S., et al. (2023). Overview and challenges of large-scale cultivation of photosynthetic microalgae and cyanobacteria. Mar. Drugs 21 (8), 445. doi:10.3390/md21080445
Palanisamy, K. M., Bhuyar, P., Rahim, M. A., Vadiveloo, A., Al-Dhabi, N. A., Govindan, N., et al. (2023). Lipid enhancement in oleaginous Nannochloropsis sp. under nitrate limitation for future bioenergy production. Int. J. Energy Res. 2023 (1), 1–8. doi:10.1155/2023/5412660
Qin, S., Wang, K., Gao, F., Ge, B., Cui, H., and Li, W. (2023). Biotechnologies for bulk production of microalgal biomass: from mass cultivation to dried biomass acquisition. Biotechnol. Biofuels Bioprod. 16, 131. doi:10.1186/s13068-023-02382-4
Ratomski, P., and Hawrot-Paw, M. (2021). Influence of nutrient-stress conditions on Chlorella vulgaris biomass production and lipid content. Catalysts 11 (5), 573. doi:10.3390/catal11050573
Rautenberger, R., Détain, A., Skjånes, K., Schulze, P. S., Kiron, V., and Morales-Sánchez, D. (2024). Growth strategies of Chlorella vulgaris in seawater for a high production of biomass and lipids suitable for biodiesel. Algal Res. 77, 103360. doi:10.1016/j.algal.2023.103360
Ru, I. T. K., Sung, Y. Y., Jusoh, M., Wahid, M. E. A., and Nagappan, T. (2020). Chlorella vulgaris: a perspective on its potential for combining high biomass with high value bioproducts. Appl. Phycol. 1 (1), 2–11. doi:10.1080/26388081.2020.1715256
Sambrook, J., and Russell, D. W. (2001). Molecular cloning: a laboratory manual. New York: Cold Spring Harbour Laboratory Press.
Sani, E. R. A., Yong, W. T. L., Chin, G. J. W. L., and Misson, M. (2021). Growth and lipid production of Isochrysis galbana in an upscale cultivation system. Trans. Sci. Technol. 8, 203–209.
Thurakit, T., Pathom-Aree, W., Pumas, C., Brocklehurst, T. W., Pekkoh, J., and Srinuanpan, S. (2022). High-efficiency production of biomass and biofuel under two-stage cultivation of a stable microalga Botryococcus braunii mutant generated by ethyl methanesulfonate-induced mutation. Renew. Energy 198, 176–188. doi:10.1016/j.renene.2022.08.029
Trivedi, J., Agrawal, D., Atray, N., and Ray, A. (2022). Enhanced lipid production in Scenedesmus obliquus via nitrogen starvation in a two-stage cultivation process and evaluation for biodiesel production. Fuel 316, 123418. doi:10.1016/j.fuel.2022.123418
Wacker, A., Piepho, M., Harwood, J. L., Guschina, I. A., and Arts, M. T. (2016). Light-induced changes in fatty acid profiles of specific lipid classes in several freshwater phytoplankton species. Front. Plant Sci. 7, 264. doi:10.3389/fpls.2016.00264
Walne, P. R. (1970). Studies on the food value of nineteen genera of algae to juvenile bivalves of the Genera Ostrea, Crassostrea, Mercenaria and Mytilus, in Fishery Investigations, Series 2. London: H. M. Stationery Office.
Yaakob, M. A., Mohamed, R. M. S. R., Al-Gheethi, A., Aswathnarayana Gokare, R., and Ambati, R. R. (2021). Influence of nitrogen and phosphorus on microalgal growth, biomass, lipid, and fatty acid production: an overview. Cells 10 (2), 393. doi:10.3390/cells10020393
Zhang, H., Bhattacharya, D., and Lin, S. (2005). Phylogeny of dinoflagellates based on mitochondrial cytochrome b and nuclear small subunit rDNA sequence comparisons. J. Phycol. 41 (2), 411–420. doi:10.1111/j.1529-8817.2005.04168.x
Keywords: biofuel scalability, fatty acid profile, inoculum concentration, lipid production, microalgae
Citation: Abdul-Sani ER, Chin GJWL, Yong WTL and Misson M (2024) Optimization of inoculum cell concentration for enhanced lipid production in laboratory-scale cultivation of the marine microalga Chlorella sp. for biofuel applications. Front. Energy Res. 12:1490421. doi: 10.3389/fenrg.2024.1490421
Received: 03 September 2024; Accepted: 14 November 2024;
Published: 27 November 2024.
Edited by:
Shunni Zhu, Chinese Academy of Sciences (CAS), ChinaReviewed by:
Sourav Kumar Bagchi, Durban University of Technology, South AfricaChanghua Shang, Guangxi Normal University, China
Copyright © 2024 Abdul-Sani, Chin, Yong and Misson. This is an open-access article distributed under the terms of the Creative Commons Attribution License (CC BY). The use, distribution or reproduction in other forums is permitted, provided the original author(s) and the copyright owner(s) are credited and that the original publication in this journal is cited, in accordance with accepted academic practice. No use, distribution or reproduction is permitted which does not comply with these terms.
*Correspondence: Grace Joy Wei Lie Chin, Z3JhY2Vqb3lAdW1zLmVkdS5teQ==