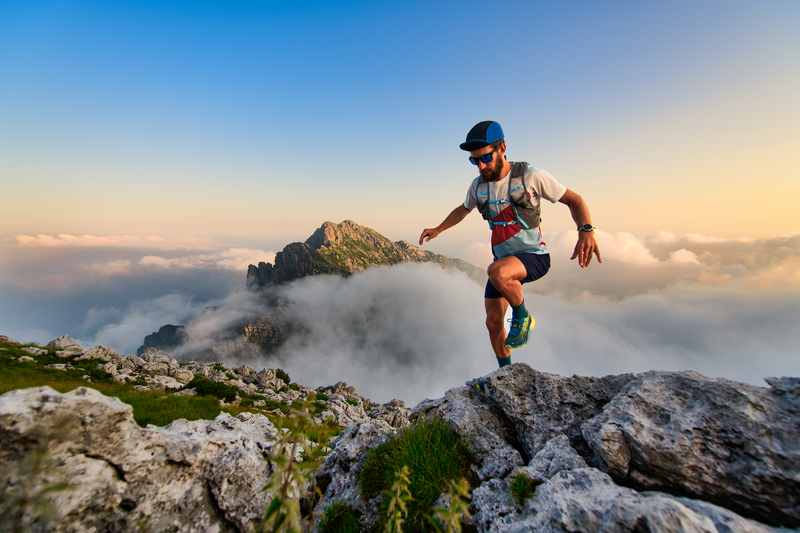
95% of researchers rate our articles as excellent or good
Learn more about the work of our research integrity team to safeguard the quality of each article we publish.
Find out more
METHODS article
Front. Energy Res. , 16 October 2024
Sec. Process and Energy Systems Engineering
Volume 12 - 2024 | https://doi.org/10.3389/fenrg.2024.1483914
This article is part of the Research Topic Advanced Water Splitting Technologies Development: Best Practices and Protocols Volume II View all 6 articles
The outdoor operation of electrochemical solar fuels devices must contend with challenges presented by the cycles of solar irradiance, temperature, and other meteorological factors. Herein, we discuss challenges associated with these fluctuations presented over three timescales, including the effects of diurnal cycling over the course of many days, a single diurnal cycle over the course of hours, and meteorological phenomena that cause fluctuations on the order of seconds to minutes. We also highlight both reaction-independent and reaction-specific effects of variable conditions for the hydrogen evolution reaction and CO2 reduction reaction. We identify key areas of research for advancing the outdoor operation of solar fuels technology and highlight the need for metrics and benchmarks to enable the comparison of diurnal studies across systems and geographical locations.
Solar-driven, electrified synthesis of chemical commodities, including H2, carbon-, and nitrogen-based chemicals from abundant feedstocks (e.g., H2O, CO2, N2), is an area of substantial research interest (Agyekum et al., 2022; Ardo et al., 2018; Basic Energy Sciences Roundtable, 2019; Hisatomi et al., 2014; Kibria et al., 2019; Pareek et al., 2020; Wang et al., 2019; Xu et al., 2024). Integrated electrochemical solar fuels reactors offer a pathway for decarbonized chemicals production and modular, off-grid deployment (Grimm et al., 2020; Mishra et al., 2007). These reactors are defined by the integration of photo- and electrocatalytically active components through careful coupling and/or co-design of active interfaces. However, there are significant research challenges associated with the translation of these solar-driven reactors from operation under “static” laboratory operating conditions to the variable conditions that these devices must tolerate once deployed outdoors (Nandjou and Haussener, 2017). While this transition has been successful for the photovoltaic (PV) industry, there remains a need to characterize the consequences of fluctuations inherent to outdoor, diurnal (i.e., the daily cycle of irradiation over a 24 h period) cycles to enable long-term, durable operation of integrated solar fuels systems.
The output of an electrochemical solar fuels reactor is a function of both photoactivity and electrocatalytic activity. While this concept is applicable to all types of electrochemical solar fuels reactors, it can be readily demonstrated in a photovoltaic-electrochemical (PV-EC) type reactor where the photovoltaic (PV) and electrochemical (EC) components can be characterized independently (Figure 1) (King et al., 2022; Wei et al., 2023; Yap et al., 2024b). The PV current-voltage curve is a function of multiple factors that dictate light-to-electricity conversion, including photon absorption, charge separation, and carrier transport (Moliton and Nunzi, 2006). The EC current-voltage curve captures the factors that dictate charge transfer across the electrode/electrolyte interface to produce fuels and chemicals, including the effects of catalytic activity, mass transport limitations, and ohmic losses (Gerhardt et al., 2021; Giordano et al., 2016). Under variable irradiation intensity and meteorological conditions (e.g., ambient temperature, wind, cloud coverage, irradiation spectra), both PV and EC polarization curves will vary (Figure 1A) (Faiman, 2008; Marion, 2002; van Dyk et al., 2005; Yap et al., 2024b). As a result, multiple operating points—up to and including a continuum of operating points—will arise for a solar fuels reactor throughout a diurnal cycle. Characterizing a representative subset of these operating points, especially those in which substantial changes to the structure and/or stability of photoactive or electrocatalytically active components occur, will be critical to the developing of solar fuels devices capable of matching the decades-long operating lifetimes already demonstrated for photovoltaics operating outdoors. The performance of other solar fuels devices, such as photoelectrochemical (PEC) devices that include direct semiconductor/electrolyte interfaces or photocatalytic (PC) systems in which the light absorber is nanoparticulate or molecular, will also be strongly affected by variable conditions. These present additional, important research challenges to disentangle the effects of diurnal cycling on photo- and electrocatalytic performance.
Figure 1. (A) Variable operating point of a solar-driven electrochemical device throughout the day via “load-line” type analysis. Two representative solar fuels reactors are illustrated, a PV-EC reactor and an integrated PV-EC reactor. The integrated PV-EC reactor schematic highlights the opportunity to measure potential differences across the PV component as well as current across the entire device, with red arrows indicating how connections can be made between the anode and cathode to measure the EC polarization curve and blue arrows indicating how the PV polarization curve can be measured. The current-voltage curve of the EC components also vary throughout the day due to changes in temperature, electrolyte composition, etc. — these variations are represented by the shaded region of the current-voltage curve. The changes underlying EC performance are expected to be small compared to the changes in PV behavior. (B) Representative schematic for outdoor and diurnal cycling effects over three timescales - days, hours, and minutes/seconds.
While the fluctuating conditions encountered in outdoor operation are complex and interrelated, we highlight three timescales on which to evaluate them: days, hours, and minutes/seconds. Over days, a solar fuels reactor must be able to maintain its performance (i.e., the activity, selectivity, and durability of the device over time) throughout many repetitions of cycling between operation during the day and “off” conditions at night (Datta et al., 2023). The “off” condition is defined as the state of operation for the solar-driven reactor where no, or nearly no, photo-driven response occurs. On the scale of hours, the operation of the solar fuels reactor will vary with incident solar irradiance as well as changes in meteorological conditions (e.g., changes in ambient temperature, humidity) — the hours of greatest solar irradiance will generally result in operation at points with greater power and total product output (Yap et al., 2024b). Finally, on the order of minutes/seconds, additional meteorological effects, such as dynamic cloud coverage and shading from surroundings, will cause fluctuations in the operation of the solar fuels reactor (Aly et al., 2019; Yap et al., 2024b; Ye et al., 2013). The fluctuations on these different timescales have distinct effects on solar fuels reactors and require the design of systems capable of maintaining selectivity, activity, and durability under variable conditions.
Efforts have been made to understand diurnal-driven effects for both the solar-driven electrochemical H2 evolution reaction (HER) and the CO2 reduction (CO2R) reaction. For example, GaInP2|GaAs|MoS2 photoelectrodes designed to drive HER were tested outdoors in Golden, Colorado over single daylight periods (i.e., <12 h) and exhibited gradual loss of H2 production throughout the day (Ben-Naim et al., 2022). These conditions, where the reactor is illuminated by the sun, are often referred to as “on-sun” conditions (in contrast to simulated conditions in a laboratory). SrTiO3:Al photocatalyst sheets were scaled to 100 m2 and operated outdoors for several months, achieving a maximum solar-to-hydrogen efficiency of 0.76% (Nandy et al., 2023; Nishiyama et al., 2021). Other efforts have assessed extended cycling stability (12 h/12 h) for HER systems for metal|oxide|semiconductor Si and tandem III-V photoelectrode architectures (Bae et al., 2019; Walczak et al., 2017). For CO2R systems, durability studies have been conducted operating at a single illumination intensity (e.g., 1000 W m−2) for over 10 hours with on/off cycling (Cheng et al., 2020; Gurudayal et al., 2019; Kamata et al., 2021; Kato et al., 2021a; Kato et al., 2021b; Kistler et al., 2021). Additionally, existing solar-driven CO2R studies probing diurnal performance have investigated operation under idealized conditions (e.g., assuming no loss of performance) via computational methods, with a focus on selectivity (King et al., 2022; Yap et al., 2023; Yap et al., 2024b). However, substantial advancements in durability as well as overall performance under outdoor operation (e.g., solar-to-fuel conversion efficiency > 10%) are still needed for electrochemical solar fuels to become a competitive technical solution (Haussener, 2022).
Herein, we consider the effects of fluctuating, diurnal conditions expected during outdoor operation of electrochemical solar fuels reactors across three timescales (days, hours, minutes/seconds) and with respect to both reaction-independent and reaction-specific effects. Reaction-independent effects refer to those which must be considered for any electrochemical solar fuels reaction, in contrast to reaction-specific effects associated with unique challenges which arise for individual reactions of interest (e.g., HER, CO2R, etc.). We also consider the role of reactor design engineering to optimize solar fuels performance with respect to diurnal conditions and highlight areas of research which can advance solar fuels technology. This work seeks to address the challenge of stable, selective electrochemical solar fuels systems under fluctuating conditions by providing a roadmap of potential focus areas for future solar fuels research.
Variations in light intensity/spectrum and temperature, which are a function of the local meteorological climate and geographical location, will exert influence on any solar-driven process.
During outdoor operation, variable irradiation drives changes in the operating point of the solar fuels reactor, causing variations in current flows and electrochemical potential differences across key interfaces (e.g., catalyst/electrolyte, electrolyte/membrane, catalyst/membrane, etc.) (Figure 1). For multiday, diurnal operation, cycling between illuminated (termed “on” for simulated illumination, or “on-sun” for outdoor illumination) and dark conditions at night (termed “off”) will affect the performance of all solar-driven devices. These on/off changes can substantially impact the stability of the electrochemical components. For example, in membrane electrode assembly (MEA) reactors, on/off cycling can affect ion transport properties across the membrane and separation efficiency of product species, resulting in loss of performance over the course of many cycles (Jao et al., 2011; Péron et al., 2008). At the (photo) electrode interface, periods under “off” conditions can result in corrosion of the electrocatalyst due to the formation and instability of surface oxide species — this is also relevant for the semiconductor in PEC/PC systems with semiconductor/electrolyte interfaces. The thermodynamics of such changes in stability should be evaluated and can often be understood in the context of an appropriately constructed Pourbaix diagram. Importantly, the nature of the device’s “off” condition should also be carefully considered. For example, a reactor at night may tend toward an “open-circuit” type condition, where no net current flows through the system, or a “reversed-current” condition depending on reactor design, electrolyte composition (e.g., concentration of dissolved redox-active species like H2 and O2) and electrode structure (Collins et al., 2024; Fu et al., 2020; Weiß et al., 2019). Studying the consequences of extended “off” periods on performance, such as at open-circuit, will be essential to designing solar-driven systems that are stable over the course of many diurnal cycles. To mirror the standard outdoor measurement protocols for PVs (Khenkin et al., 2020), experiments to isolate and evaluate device day/night durability over multiple cycles will be valuable and may involve cycling between different electrochemical potentials (e.g., expected peak current and open-circuit) over many cycles on timescales similar to those expected during diurnal operation (e.g., 12 h on/12 h off, 16 h on/8 h off). Shorter experiments, conducted using a potentiostat or solar simulator equipped with a light shutter, could be employed to evaluate stability during day/night cycling, but developing mechanistic connections between any accelerated diurnal cycling methodology and the full 24 h timescale of a true diurnal cycle will be critical.
Throughout a single diurnal cycle (i.e., 1 day), solar irradiation changes over the course of hours and is dependent on seasonal, locational, and other environmental factors. Since it is difficult to directly compare the “on-sun” performance of solar fuels devices at different locations, most solar fuels reactors have been evaluated under illumination at 1000 W m−2 (i.e., 1-sun) with a fixed AM 1.5G spectrum. This translates to operation of the solar fuels device at a single operating point (Cheng et al., 2020; Kuang et al., 2019; Pan et al., 2023; Yap et al., 2024a). However, estimating a diurnal output from constant illumination conditions can result in overestimations of performance and can fail to capture the consequences of diurnal variation in incident solar spectrum on effects such as carrier collection, solvent absorption, and PV heat absorption (Ishii et al., 2013; Kistler et al., 2020; Nofuentes et al., 2014). Ambient temperature, which can vary significantly over a day, as well as across locations and seasons, will also dictate changes in performance and durability. At low operating temperatures (e.g., at night or during the winter), ion transport rates decrease. This can decrease conductivity, slow ion transport, and cause deformation of membranes, resulting in expedited degradation of performance in the electrochemical device (Guo and Qi, 2006; Kim and Mench, 2007). Designs and studies that consider these temperature-driven phenomena, and the effects of cycling between freezing and thawing, will be key to building temperature-robust solar fuels devices (Mensharapov et al., 2022; Shengsheng, 2006).
Where enabled by device design, testing varying potential conditions that mimic those expected under the hourly diurnal irradiation profile can be used to experimentally probe the hours-scale stability of solar fuels devices. These potential profiles will be unique to each solar fuels setup and are dependent on the operating points described in Figure 1. To accurately simulate these profiles, it is valuable to measure both current across the device as well as voltage across the photoactive component (where possible) (Figure 1A) for the device in question from outdoor testing — this data is useful in assigning performance drops to specific components of the solar fuels reactor (Kistler and Agbo, 2020). It is useful to recognize that there may be multiple mechanisms of degradation in solar fuels devices, including some that may occur under illuminated conditions without requiring “on/off” cycling. Probing these degradation mechanisms over multiple cycles, without a simulated “off” state can elucidate the stability of the solar fuels device with respect to hourly changes, and the reversibility of any changes which may occur in the electrocatalyst (e.g., oxidation or electronic state changes, morphology changes) (Hochfilzer et al., 2023; Minguzzi et al., 2012). Recent work to investigate the durability of GaInP-based photocathodes for HER has emphasized the importance of operating conditions and photoelectrode design, highlighting the competition between charge transfer towards product formation versus degradation, and the need to control the movement of electrons and holes to dictate long-term durability, even when the photoelectrode is maintained under reducing and/or oxidizing conditions (Yu et al., 2021). Additionally, diurnal spectral variation and changes in temperature of the PV or EC components (due to ambient temperate and/or irradiation-driven heating) can be monitored and studied separately in a controlled lab environment — these efforts combined with varying applied potential could be used to simulate “on-sun” experiments.
The U.S. Department of Energy’s (DOE) protocol (Bloom et al., 2013; Yuan et al., 2011) for polymer-electrolyte membrane fuel cells (PEMFC) provides inspiration for durability testing of solar fuel devices under variable conditions, including steady-state durability tests, potential cycling durability tests, and start-up/shutdown cycling durability tests. Of particular applicability to solar fuels devices are load cycling tests with conditions spanning from minimum to maximum operating current/potential for the solar fuels device. When coupled with in-situ characterization techniques, such as online inductively-coupled plasma mass spectroscopy (ICP-MS) (Ji et al., 2020; Ledendecker et al., 2017), these tests can provide insight into the stability of the electrochemical components under conditions representative of those expected during on-sun operation.
Variations caused by meteorological and environmental fluctuations, such as cloud coverage, rain, shade, and wind, result in changes in the operating conditions of solar fuels devices on the second-to-minutes scale (Figure 1).30 Potential step methods with various step frequencies and applied potentials, or illuminated experiments with light chopping at various frequencies, can be employed to probe the consequences of meteorological fluctuations. Degradation studies in battery science provide insight into possible failure modes under these conditions — degradation mechanisms of Li-ion batteries under accelerated stress test conditions include electrode structural change and decomposition, particle fracture, solid-electrolyte interface growth and Li plating (Edge et al., 2021; Liu et al., 2022; Pender et al., 2020; Xiong et al., 2020; Zhu et al., 2020). Mirroring these battery degradation mechanisms, one significant challenge that may be associated with short-term fluctuation in conditions is catalyst degradation due to volume change or phase change, resulting in catalyst leaching (Bae et al., 2019). If the catalyst has multiple chemical states, or a small potential window in which it is stable, meteorological fluctuations may be a critical driver of degradation. Solar fuels systems will require the development of electrode structures, including the support, catalyst, and any protective overlayers, which are able to maintain their performance within a wide operating window.
Reaction-specific considerations pertaining to solar-driven HER and CO2R under variable conditions and diurnal cycles are also important to consider. These include selectivity variations for single product vs. multi-product electrochemical reactions as well as temperature changes throughout the diurnal cycle.
For reactions expected to produce a single product, such as HER, the product formation rate is expected to scale proportionally with the irradiance profile. In these cases, the challenge is to maximize product output per day (i.e., charge passed per day) and to maintain performance over the course of many days. For electrocatalysts that can be tested in isolation from photoactive components, these conditions could be simulated by well-designed potential profiles to sweep from “off” condition potentials to those that mimic the irradiation profile, followed by an extended hold at ‘open-circuit’ or under relevant “reversed-current” conditions to simulate the “off” period. Developing electrochemical systems able to maintain this performance over repeated on/off cycles would be a valuable step toward solar fuels systems capable of operating under outdoor and diurnal conditions for lifetimes similar to those of the PV component. Such advances would also benefit electrochemical systems, improving energy efficiency and tolerance to changes caused by fluctuations in local microenvironment conditions.
Multi-product electrochemical reactions, such as CO2R using a Cu-based catalyst, have product distributions (e.g., C2H4, EtOH, CH4, CO, H2, etc.) which are highly dependent on applied potential and features of the catalyst/electrolyte interface (Garza et al., 2018; Huang et al., 2019; Lv et al., 2018; Nitopi et al., 2019; Todorova et al., 2020). The CO2R product distribution is affected by the properties of the local microenvironment, including the structure of the electric double layer, pH, temperature, and catalyst morphology/active sites (Bui et al., 2022; Pan and Yang, 2020; Simon et al., 2021; Sun et al., 2020; Zhang et al., 2020). In solar fuels systems, the additional variability expected during outdoor operation introduces distinct performance considerations. The nature of the irradiation profile (Figures 2A, B) will result in the formation of variable electrochemical potential differences — in a PV-EC-type device this is readily represented by changes in operating point (i.e., full cell voltage and total current) throughout the day. For multi-product CO2R systems, this translates to varying product distributions and periods when different CO2R products will dominate (e.g., CO, C2H4) (Figures 2C, D). The extent to which product output ratios (e.g., CO:H2, C2H4:CH4) differ between low and high irradiation windows is dependent on the potential range in which the electrocatalyst can maintain similar product selectivity. For example, using a Cu electrocatalyst, modeling has suggested that CO and H2 will be favored at times of lower irradiation and products such as C2H4 will be favored during higher irradiation periods (Figure 2C) (Garza et al., 2018; Todorova et al., 2020; Yap et al., 2024b) Electrocatalysts capable of demonstrating the desired selectivity in wide potential windows are important for improving tolerance to fluctuating irradiation conditions and can minimize the extent to which downstream processes (e.g., separation or further reaction) will have to adapt to variations in the product stream. Fluctuating conditions are also important in CO2R systems designed to produce CO (e.g., those using Au, Ag-based cathodes), and recent work has highlighted the opportunity for stable Ag-based CO2R MEA systems over many cycles (Samu et al., 2022).
Figure 2. Schematic representation of (A) diurnal irradiance profile and (B) associated operating points for the electrochemical solar fuels device at four different times throughout the day. The EC curve is highlighted with a hypothetical major CO2R product produced at each respective I-V point. (C) Representative Faradaic efficiency vs. time plots which result from the irradiance profile in (A) and resultant operating points in (B). (D) Representative partial current density vs. time plots which correspond to the conditions from (A, B). Only gaseous CO2R products are illustrated in (C) for ease of illustration. The figure was adapted from results reported in Yap et al. (2024b) with permission from the Royal Society of Chemistry. Irradiance data sourced from the NREL National Solar Radiation Database (NSRDB, 2024).
The diurnal irradiation cycle is accompanied by a variable ambient temperature profile (Figure 3). Ambient temperature, combined with irradiation-derived heat adsorption, defines the operating temperature of both PV and EC components when left unregulated. While these temperature effects are well-studied for commercial-grade PVs (Faiman, 2008), the consequences of these phenomena on integrated solar-driven electrochemical systems remain an important question. For example, current, state-of-the-art water electrolyzers utilize proton exchange membranes (PEM) or anion exchange membranes (AEM) and operate at 80°C (Aili et al., 2023; Corti, 2022; Ion-Ebrasu et al., 2020; Ion-Ebrasu et al., 2020; Lee et al., 2023). If a solar fuels system uses a similar membrane-containing architecture, fluctuations in temperature are likely to have consequences on activation, ohmic, and diffusion overpotentials, and have potential consequences on the stability of even the most durable membranes commonly used in these electrolyzers (Chandesris et al., 2015; Guo and Qi, 2006; Kim and Mench, 2007; Okonkwo et al., 2021; Olivier et al., 2017; Yigit and Selamet, 2016).
Figure 3. Irradiance vs. time and ambient temperature vs. time profiles for Barstow, CA for 4 days in 2020. A representative day for each season was chosen. Data is sourced from NREL’s National Solar Radiation Database (NSRDB, 2024). (A) Spring equinox. (B) Summer solstice. (C) Fall equinox. (D) Winter solstice.
Temperature changes can influence selectivity, activity, and durability of the reactor. For conventional CO2R systems, temperature changes can affect the solubility of CO2 in the electrolyte, resulting in changes of the interfacial pH, selectivity, and activity (Vos et al., 2023; Vos and Koper, 2022). Additionally, in multi-product mechanisms, different products may have different temperature dependence, resulting in selectively profiles that are a complex function of temperature (Vos et al., 2023; Vos and Koper, 2022; Yap et al., 2024b). This temperature dependence can also vary as a function of the characteristics of the catalyst/electrolyte interface, adding to the complexity of temperature cycling in multi-product systems (Corpus et al., 2023; Weng et al., 2018). The temperature dependence of CO2R product output remains an important question and should be answered in controlled electrochemical environments to inform diurnal operation. Finally, in CO2R systems, AEM are commonly used to favor CO2R over the competing HER (Salvatore et al., 2021). The development of AEMs is an active field and the choice of AEM directly influences the selectivity of CO2R systems through changes in OH− conductivity, pH stability, and temperature tolerance (Luo et al., 2020; Salvatore et al., 2021; Yin et al., 2019). The chemical and mechanical stability of AEMs in CO2R-specific environments is a key concern, and a solar-fuels compatible AEM must exhibit a wide temperature stability range and be able to withstand short-term variations in ionic current, electric field, and pH under diurnal conditions (Krivina et al., 2022; Lindquist et al., 2021; Salvatore et al., 2021).
Reactor design will also be key toward optimizing outdoor, diurnal operation. Optimizing this design at the lab-scale will enable smoother transition to larger-scale systems and can provide resilience against changes in operating temperature, irradiation, or other environmental factors. Utilizing heat available from “on-sun” operation presents an opportunity to integrate heating/cooling in innovative and distinct ways to improve overall energy efficiency (Kemppainen et al., 2023; Kistler et al., 2022; Kölbach et al., 2022; Tembhurne et al., 2019; Tembhurne and Haussener, 2019). Additionally, a more complete utilization of the solar spectrum (e.g., low energy photons) can allow for thermal coupling of irradiation and electrocatalytic activity, improving the efficiency of the solar fuels reactor (Johnson and Haussener, 2024). Integrated solar fuels reactors also offer the unique opportunity to utilize generated heat for potentially beneficial hybrid technology, including thermally-driven conversion of H2 into higher value products, such as green ammonia (Tembhurne and Haussener, 2016). Designing and modeling heat integration systems under simulated diurnal conditions will improve energy efficiency when scaling electrochemical solar fuels reactors beyond the lab scale and identify ways to reduce capital (CapEx) and operating (OpEx) costs.
Another important consideration in scaling of solar fuels systems is the operating configuration. In a PV-EC architecture, for example, there is an opportunity to control product output and efficiency through plant design, as illustrated in Figure 4. In one configuration, an electrolyzer operates without any additional power regulation (Figure 4A). This results in a variable product generation rate (represented by current output) throughout the diurnal cycle that may add complexity in downstream separation processes. In the other, multiple electrolyzers are employed, where additional electrolyzers, connected in parallel, are turned on only after a particular power threshold (as defined by the current-voltage profile and associated selectivity) is reached (Figure 4B). This configuration will result in lower power efficiency and individual electrolyzer capacity factor, but may reduce the complexity and cost of the downstream separation processes. For a single product reaction, the first configuration may be advantageous to maximize total product output. Conversely, the second configuration may be more compatible with multi-product reactions where consistent product streams will lower separations costs. Designing the appropriate reactor for solar-driven fuel formation will require further technoeconomic studies to balance electrolyzer cost versus the cost of downstream separation.
Figure 4. Possible configurations for operation of electrochemical solar fuels reactors over one diurnal cycle. (A) One electrolyzer operates constantly throughout the diurnal cycle. (B) Multiple electrolyzers, connected in parallel, are powered up or powered down to drive operation in narrow current regimes.
Understanding the performance of electrochemical solar fuels reactors under fluctuating, outdoor, diurnal conditions will be important in advancing the field. To tackle the reaction-specific challenge of selectivity during diurnal cycling, catalysts for multi-product reactions with high selectivity for a desired product over a wide range of potentials are needed (Chen et al., 2021; Lim et al., 2023). Coupled with the careful design of photoactive components, this can mitigate variations in the composition of the outlet product stream during daytime operation and reduce the downstream costs of product separation. To promote long-term cycling stability, reports on durability of existing and novel electrocatalysts under outdoor-like and cycling conditions are encouraged. Potential methods for evaluating this stability include coupling in-situ characterization techniques, such as online ICP-MS, to longer electrochemical measurements which more accurately represent a full 24 h diurnal cycle (Pishgar et al., 2021; Popović et al., 2020). Identifying electrocatalytic changes which occur as potential is cycled is a crucial first step to designing durable catalysts and informing the design of appropriate “off” conditions for solar fuels devices. To enable comparison of various electrocatalysts under simulated outdoor conditions, there is a need to develop standardized durability testing methods representative of these conditions, focusing both on voltage changes and temperature changes driven by meteorological effects — these methods should address all three diurnal timescales (days, hours, and minutes/seconds). Similar experiments should also be conducted with temperature cycling to gain insight into the role of temperature on performance stability and to identify failure mechanisms under isolated conditions of potential and temperature cycling (Garbe et al., 2021; Tomić et al., 2023). Electrochemical systems designed for stability under these isolated potential and temperature conditions can then be advanced to outdoor performance tests.
Another critical aspect for the future outdoor, diurnal operation of solar fuels devices is the need to define standardized metrics and benchmarks to enable comparison across studies. Of particular importance are the parameters for evaluating electrocatalysts and membranes under the experimental regimes detailed above. For example, stability could be reported in relative values of loss (e.g., % activity loss over n cycles, % catalyst loss over n cycles) to remove differences derived from variable initial electrochemical systems [e.g., different catalyst loading, electrochemical active surface area (ECSA)] (Edgington and Seitz, 2023; Lopes, 2023). Another metric that must be considered for diurnal operation is diurnal solar-to-fuel conversion efficiency. Under outdoor, diurnal conditions, this calculation is complicated by changes in temperature, illumination, selectivity, and activity. Thus, there is a need to develop standardized methods for analyzing and comparing solar fuels performance across studies, as exists for PV systems (Kratochvil et al., 2004).
The design and performance of electrochemical solar fuels systems must necessarily reflect the outdoor, diurnal conditions in which they will operate. We have identified three timescales of relevance for to outdoor operation and diurnal cycling (days, hours, and minutes/seconds) and discussed the challenges which arise with each of these timescales from the perspective of reaction-independent and reaction-specific challenges. To design solar fuels systems capable of maintaining performance outdoors over diurnal cycles, it is crucial to understand the operation constraints imposed by this variability and to design electrochemical and photovoltaic components, as well as reactors, which are capable of withstanding fluctuating conditions. A well-engineered solar fuels system must be designed to manage its own circadian rhythm, much like a biological organism, to properly respond to changes in external conditions and to maintain performance dynamically over long timescales. The challenge of stable outdoor operation must be addressed for deployable solar fuels technologies to become reality, and the advances driven by this research should be mutually beneficial to a range of electrochemical systems.
The original contributions presented in the study are included in the article/supplementary material, further inquiries can be directed to the corresponding authors.
KY: Conceptualization, Formal Analysis, Investigation, Methodology, Validation, Visualization, Writing–original draft, Writing–review and editing. SL: Conceptualization, Formal Analysis, Investigation, Methodology, Validation, Visualization, Writing–original draft, Writing–review and editing. TK: Conceptualization, Validation, Writing–review and editing. DC: Conceptualization, Validation, Writing–review and editing. EW: Conceptualization, Funding acquisition, Supervision, Validation, Writing–review and editing. HA: Conceptualization, Funding acquisition, Supervision, Writing–review and editing. TJ: Conceptualization, Funding acquisition, Supervision, Writing–review and editing. CX: Conceptualization, Funding acquisition, Project administration, Resources, Supervision, Writing–review and editing. AN: Conceptualization, Funding acquisition, Project administration, Resources, Supervision, Visualization, Writing–original draft, Writing–review and editing, Methodology.
The author(s) declare that financial support was received for the research, authorship, and/or publication of this article. This work was funded by the Liquid Sunlight Alliance, which is supported by the U.S. Department of Energy, Office of Science, Office of Basic Energy Sciences, Fuels from Sunlight Hub under Award Number DE-SC0021266.
The authors declare that the research was conducted in the absence of any commercial or financial relationships that could be construed as a potential conflict of interest.
All claims expressed in this article are solely those of the authors and do not necessarily represent those of their affiliated organizations, or those of the publisher, the editors and the reviewers. Any product that may be evaluated in this article, or claim that may be made by its manufacturer, is not guaranteed or endorsed by the publisher.
Agyekum, E. B., Nutakor, C., Agwa, A. M., and Kamel, S. (2022). A critical review of renewable hydrogen production methods: factors affecting their scale-up and its role in future energy generation. Membranes 12, 173. doi:10.3390/membranes12020173
Aili, D., Kraglund, M. R., Rajappan, S. C., Serhiichuk, D., Xia, Y., Deimede, V., et al. (2023). Electrode separators for the next-generation alkaline water electrolyzers. ACS Energy Lett. 8, 1900–1910. doi:10.1021/acsenergylett.3c00185
Aly, S. P., Ahzi, S., and Barth, N. (2019). Effect of physical and environmental factors on the performance of a photovoltaic panel. Sol. Energy Mat. Sol. Cells 200, 109948. doi:10.1016/j.solmat.2019.109948
Ardo, S., Rivas, D. F., A. Modestino, M., Greiving, V. S., F. Abdi, F., Llado, E. A., et al. (2018). Pathways to electrochemical solar-hydrogen technologies. Energy Environ. Sci. 11, 2768–2783. doi:10.1039/C7EE03639F
Bae, D., Seger, B., Hansen, O., Vesborg, P. C. K., and Chorkendorff, I. (2019). Durability testing of photoelectrochemical hydrogen production under day/night light cycled conditions. ChemElectroChem 6, 106–109. doi:10.1002/celc.201800918
Basic Energy Sciences Roundtable (2019). Report of the basic energy sciences roundtable on Liquid solar fuels. doi:10.2172/1615599
Ben-Naim, M., Aldridge, C. W., Steiner, M. A., Nielander, A. C., Deustch, T. G., Young, J. L., et al. (2022). Demonstration of photoreactor platform for on-sun unassisted photoelectrochemical hydrogen generation with tandem III–V photoelectrodes. Chem. Catal. 2, 195–209. doi:10.1016/j.checat.2021.12.013
Bloom, I., Walker, L. K., Basco, J. K., Malkow, T., Saturnio, A., De Marco, G., et al. (2013). A comparison of fuel cell testing protocols – a case study: protocols used by the U.S. Department of energy, European union, international electrotechnical commission/fuel cell testing and standardization network, and fuel cell technical team. J. Power Sources 243, 451–457. doi:10.1016/j.jpowsour.2013.06.026
Bui, J. C., Kim, C., King, A. J., Romiluyi, O., Kusoglu, A., Weber, A. Z., et al. (2022). Engineering catalyst–electrolyte microenvironments to optimize the activity and selectivity for the electrochemical reduction of CO2 on Cu and Ag. Acc. Chem. Res. 55, 484–494. doi:10.1021/acs.accounts.1c00650
Chandesris, M., Médeau, V., Guillet, N., Chelghoum, S., Thoby, D., and Fouda-Onana, F. (2015). Membrane degradation in PEM water electrolyzer: numerical modeling and experimental evidence of the influence of temperature and current density. Int. J. Hydrog. Energy 40, 1353–1366. doi:10.1016/j.ijhydene.2014.11.111
Chen, Z., Huang, A., Yu, K., Cui, T., Zhuang, Z., Liu, S., et al. (2021). Fe 1 N 4 –O 1 site with axial Fe–O coordination for highly selective CO 2 reduction over a wide potential range. Energy Environ. Sci. 14, 3430–3437. doi:10.1039/D1EE00569C
Cheng, W.-H., Richter, M. H., Sullivan, I., Larson, D. M., Xiang, C., Brunschwig, B. S., et al. (2020). CO 2 reduction to CO with 19% efficiency in a solar-driven gas diffusion electrode flow cell under outdoor solar illumination. ACS Energy Lett. 5, 470–476. doi:10.1021/acsenergylett.9b02576
Collins, D. K., Schichtl, Z. G., Nesbitt, N. T., Greenaway, A. L., Mihailetchi, V. D., Tune, D., et al. (2024). Utilizing three-terminal, interdigitated back contact Si solar cells as a platform to study the durability of photoelectrodes for solar fuel production. Energy Environ. Sci. 17, 3329–3337. doi:10.1039/D4EE00349G
Corpus, K. R. M., Bui, J. C., Limaye, A. M., Pant, L. M., Manthiram, K., Weber, A. Z., et al. (2023). Coupling covariance matrix adaptation with continuum modeling for determination of kinetic parameters associated with electrochemical CO2 reduction. Joule 7, 1289–1307. doi:10.1016/j.joule.2023.05.007
Corti, H. R. (2022). Polymer electrolytes for low and high temperature PEM electrolyzers. Curr. Opin. Electrochem. 36, 101109. doi:10.1016/j.coelec.2022.101109
Datta, K., Branco, B., Zhao, Y., Zardetto, V., Phung, N., Bracesco, A., et al. (2023). Efficient continuous light-driven electrochemical water splitting enabled by monolithic perovskite-silicon tandem photovoltaics. Adv. Mat. Technol. 8, 2201131. doi:10.1002/admt.202201131
Edge, J. S., O’Kane, S., Prosser, R., Kirkaldy, N. D., Patel, A. N., Hales, A., et al. (2021). Lithium ion battery degradation: what you need to know. Phys. Chem. Chem. Phys. 23, 8200–8221. doi:10.1039/D1CP00359C
Edgington, J., and Seitz, L. C. (2023). Advancing the rigor and reproducibility of electrocatalyst stability benchmarking and intrinsic material degradation analysis for water oxidation. ACS Catal. 13, 3379–3394. doi:10.1021/acscatal.2c06282
Faiman, D. (2008). Assessing the outdoor operating temperature of photovoltaic modules. Prog. Photovolt. Res. Appl. 16, 307–315. doi:10.1002/pip.813
Fu, H. J., Moreno-Hernandez, I. A., Buabthong, P., Papadantonakis, K. M., Brunschwig, B. S., and Lewis, N. S. (2020). Enhanced stability of silicon for photoelectrochemical water oxidation through self-healing enabled by an alkaline protective electrolyte. Energy Environ. Sci. 13, 4132–4141. doi:10.1039/D0EE02250K
Garbe, S., Futter, J., Agarwal, A., Tarik, M., Mularczyk, A. A., Schmidt, T. J., et al. (2021). Understanding degradation effects of elevated temperature operating conditions in polymer electrolyte water electrolyzers. J. Electrochem. Soc. 168, 044515. doi:10.1149/1945-7111/abf4ae
Garza, A. J., Bell, A. T., and Head-Gordon, M. (2018). Mechanism of CO2 reduction at copper surfaces: pathways to C2 products. ACS Catal. 8, 1490–1499. doi:10.1021/acscatal.7b03477
Gerhardt, M. R., Pant, L. M., Bui, J. C., Crothers, A. R., Ehlinger, V. M., Fornaciari, J. C., et al. (2021). Method—practices and pitfalls in voltage breakdown analysis of electrochemical energy-conversion systems. J. Electrochem. Soc. 168, 074503. doi:10.1149/1945-7111/abf061
Giordano, L., Han, B., Risch, M., Hong, W. T., Rao, R. R., Stoerzinger, K. A., et al. (2016). pH dependence of OER activity of oxides: current and future perspectives. Catal. Today, Electrocatalysis 262, 2–10. doi:10.1016/j.cattod.2015.10.006
Grimm, A., de Jong, W. A., and Kramer, G. J. (2020). Renewable hydrogen production: a techno-economic comparison of photoelectrochemical cells and photovoltaic-electrolysis. Int. J. Hydrog. Energy 45, 22545–22555. doi:10.1016/j.ijhydene.2020.06.092
Guo, Q., and Qi, Z. (2006). Effect of freeze-thaw cycles on the properties and performance of membrane-electrode assemblies. J. Power Sources, Special issue Incl. Sel. Pap. Present. A. T. Int. Workshop Molten Carbonate Fuel Cells Relat. Sci. Technol. 2005 together Regul. Pap. 160, 1269–1274. doi:10.1016/j.jpowsour.2006.02.093
Gurudayal, G., Beeman, J. W., Bullock, J., Wang, H., Eichhorn, J., Towle, C., et al. (2019). Si photocathode with Ag-supported dendritic Cu catalyst for CO 2 reduction. Energy Environ. Sci. 12, 1068–1077. doi:10.1039/C8EE03547D
Haussener, S. (2022). Solar fuel processing: comparative mini-review on research, technology development, and scaling. Sol. Energy 246, 294–300. doi:10.1016/j.solener.2022.09.019
Hisatomi, T., Kubota, J., and Domen, K. (2014). Recent advances in semiconductors for photocatalytic and photoelectrochemical water splitting. Chem. Soc. Rev. 43, 7520–7535. doi:10.1039/C3CS60378D
Hochfilzer, D., Chorkendorff, I., and Kibsgaard, J. (2023). Catalyst stability considerations for electrochemical energy conversion with non-noble metals: do we measure on what we synthesized? ACS Energy Lett. 8, 1607–1612. doi:10.1021/acsenergylett.3c00021
Huang, J., Mensi, M., Oveisi, E., Mantella, V., and Buonsanti, R. (2019). Structural sensitivities in bimetallic catalysts for electrochemical CO2 reduction revealed by Ag–Cu nanodimers. J. Am. Chem. Soc. 141, 2490–2499. doi:10.1021/jacs.8b12381
Ion-Ebrasu, D., Pollet, B. G., Caprarescu, S., Chitu, A., Trusca, R., Niculescu, V., et al. (2020). Graphene inclusion effect on anion-exchange membranes properties for alkaline water electrolyzers. Int. J. Hydrog. Energy 45, 17057–17066. doi:10.1016/j.ijhydene.2020.04.195
Ishii, T., Otani, K., Takashima, T., and Xue, Y. (2013). Solar spectral influence on the performance of photovoltaic (PV) modules under fine weather and cloudy weather conditions. Prog. Photovolt. Res. Appl. 21, 481–489. doi:10.1002/pip.1210
Jao, T.-C., Jung, G.-B., Chi, P.-H., Ke, S.-T., and Chan, S.-H. (2011). Investigation of degradation behavior of membrane electrode assembly with polytetrafluoroethylene/Nafion composite membrane. J. Power Sources 196, 1818–1825. doi:10.1016/j.jpowsour.2010.09.068
Ji, S. G., Kim, H., Choi, H., Lee, S., and Choi, C. H. (2020). Overestimation of photoelectrochemical hydrogen evolution reactivity induced by noble metal impurities dissolved from counter/reference electrodes. ACS Catal. 10, 3381–3389. doi:10.1021/acscatal.9b04229
Johnson, E., and Haussener, S. (2024). Thermal-integration in photoelectrochemistry for fuel and heat co-generation. Sustain. Energy Fuels. 8, 4199–4212. doi:10.1039/D4SE00304G
Kamata, R., Kumagai, H., Yamazaki, Y., Higashi, M., Abe, R., and Ishitani, O. (2021). Durable photoelectrochemical CO 2 reduction with water oxidation using a visible-light driven molecular photocathode. J. Mat. Chem. A 9, 1517–1529. doi:10.1039/D0TA07351B
Kato, N., Mizuno, S., Shiozawa, M., Nojiri, N., Kawai, Y., Fukumoto, K., et al. (2021a). A large-sized cell for solar-driven CO2 conversion with a solar-to-formate conversion efficiency of 7.2%. Joule 5, 687–705. doi:10.1016/j.joule.2021.01.002
Kato, N., Takeda, Y., Kawai, Y., Nojiri, N., Shiozawa, M., Mizuno, S., et al. (2021b). Solar fuel production from CO 2 using a 1 m-Square-Sized reactor with a solar-to-formate conversion efficiency of 10.5. ACS Sustain. Chem. Eng. 9, 16031–16037. doi:10.1021/acssuschemeng.1c06390
Kemppainen, E., Bagacki, R., Schary, C., Bao, F., Dorbandt, I., Janke, S., et al. (2023). Dynamic operation of a heat exchanger in a thermally integrated photovoltaic electrolyzer. Energy Technol. 11, 2201081. doi:10.1002/ente.202201081
Khenkin, M. V., Katz, E. A., Abate, A., Bardizza, G., Berry, J. J., Brabec, C., et al. (2020). Consensus statement for stability assessment and reporting for perovskite photovoltaics based on ISOS procedures. Nat. Energy 5, 35–49. doi:10.1038/s41560-019-0529-5
Kibria, M. G., Edwards, J. P., Gabardo, C. M., Dinh, C.-T., Seifitokaldani, A., Sinton, D., et al. (2019). Electrochemical CO2 reduction into chemical feedstocks: from mechanistic electrocatalysis models to system design. Adv. Mat. 31, 1807166. doi:10.1002/adma.201807166
Kim, S., and Mench, M. M. (2007). Physical degradation of membrane electrode assemblies undergoing freeze/thaw cycling: micro-structure effects. J. Power Sources, Hybrid. Electr. Veh. 174, 206–220. doi:10.1016/j.jpowsour.2007.08.111
King, A. J., Bui, J. C., Bell, A. T., and Weber, A. Z. (2022). Establishing the role of operating potential and mass transfer in multicarbon product generation for photoelectrochemical CO2 reduction cells using a Cu catalyst. ACS Energy Lett. 7, 2694–2700. doi:10.1021/acsenergylett.2c01041
Kistler, T. A., and Agbo, P. (2020). Current loss analysis in photoelectrochemical devices. Apl. Mater 8, 031107. doi:10.1063/1.5142561
Kistler, T. A., Um, M. Y., and Agbo, P. (2020). Stable photoelectrochemical hydrogen evolution for 1000 h at 14% efficiency in a monolithic vapor-fed device. J. Electrochem. Soc. 167, 066502. doi:10.1149/1945-7111/ab7d93
Kistler, T. A., Um, M. Y., Cooper, J. K., Sharp, I. D., and Agbo, P. (2021). Monolithic photoelectrochemical CO2 reduction producing syngas at 10% efficiency. Adv. Energy Mat. 11, 2100070. doi:10.1002/aenm.202100070
Kistler, T. A., Um, M. Y., Cooper, J. K., Sharp, I. D., and Agbo, P. (2022). Exploiting heat transfer to achieve efficient photoelectrochemical CO2 reduction under light concentration. Energy Environ. Sci. 15, 2061–2070. doi:10.1039/D1EE03957A
Kölbach, M., Höhn, O., Rehfeld, K., Finkbeiner, M., Barry, J., and M. May, M. (2022). The annual-hydrogen-yield-climatic-response ratio: evaluating the real-life performance of integrated solar water splitting devices. Sustain. Energy Fuels 6, 4062–4074. doi:10.1039/D2SE00561A
Kratochvil, J., Boyson, W., and King, D. (2004). Photovoltaic array performance model. (No. SAND2004-3535, 919131). doi:10.2172/919131
Krivina, R. A., Lindquist, G. A., Yang, M. C., Cook, A. K., Hendon, C. H., Motz, A. R., et al. (2022). Three-electrode study of electrochemical ionomer degradation relevant to anion-exchange-membrane water electrolyzers. ACS Appl. Mat. Interfaces 14, 18261–18274. doi:10.1021/acsami.1c22472
Kuang, Y., Kenney, M. J., Meng, Y., Hung, W.-H., Liu, Y., Huang, J. E., et al. (2019). Solar-driven, highly sustained splitting of seawater into hydrogen and oxygen fuels. Proc. Natl. Acad. Sci. 116, 6624–6629. doi:10.1073/pnas.1900556116
Ledendecker, M., Mondschein, J. S., Kasian, O., Geiger, S., Göhl, D., Schalenbach, M., et al. (2017). Stability and activity of non-noble-metal-based catalysts toward the hydrogen evolution reaction. Angew. Chem. Int. Ed. 56, 9767–9771. doi:10.1002/anie.201704021
Lee, J. K., Schuler, T., Bender, G., Sabharwal, M., Peng, X., Weber, A. Z., et al. (2023). Interfacial engineering via laser ablation for high-performing PEM water electrolysis. Appl. Energy 336, 120853. doi:10.1016/j.apenergy.2023.120853
Lim, C. Y. J., Yilmaz, M., Arce-Ramos, J. M., Handoko, A. D., Teh, W. J., Zheng, Y., et al. (2023). Surface charge as activity descriptors for electrochemical CO2 reduction to multi-carbon products on organic-functionalised Cu. Nat. Commun. 14, 335. doi:10.1038/s41467-023-35912-7
Lindquist, G. A., Oener, S. Z., Krivina, R., Motz, A. R., Keane, A., Capuano, C., et al. (2021). Performance and durability of pure-water-fed anion exchange membrane electrolyzers using baseline materials and operation. ACS Appl. Mat. Interfaces 13, 51917–51924. doi:10.1021/acsami.1c06053
Liu, J., Yuan, H., Liu, H., Zhao, C.-Z., Lu, Y., Cheng, X.-B., et al. (2022). Unlocking the failure mechanism of solid state lithium metal batteries. Adv. Energy Mat. 12, 2100748. doi:10.1002/aenm.202100748
Lopes, P. P. (2023). A framework for the relationships between stability and functional properties of electrochemical energy materials. ACS Mat. Au 3, 8–17. doi:10.1021/acsmaterialsau.2c00044
Luo, X., Rojas-Carbonell, S., Yan, Y., and Kusoglu, A. (2020). Structure-transport relationships of poly(aryl piperidinium) anion-exchange membranes: eeffect of anions and hydration. J. Membr. Sci. 598, 117680. doi:10.1016/j.memsci.2019.117680
Lv, J.-J., Jouny, M., Luc, W., Zhu, W., Zhu, J.-J., and Jiao, F. (2018). A highly porous copper electrocatalyst for carbon dioxide reduction. Adv. Mat. 30, 1803111. doi:10.1002/adma.201803111
Marion, B. (2002). A method for modeling the current–voltage curve of a PV module for outdoor conditions. Prog. Photovolt. Res. Appl. 10, 205–214. doi:10.1002/pip.403
Mensharapov, R., Ivanova, N., Spasov, D., Grigoriev, S., and Fateev, V. (2022). SAXS investigation of the effect of freeze/thaw cycles on the nanostructure of Nafion® membranes. Polymers 14, 4395. doi:10.3390/polym14204395
Minguzzi, A., F. Fan, F.-R., Vertova, A., Rondinini, S., and J. Bard, A. (2012). Dynamic potential–pH diagrams application to electrocatalysts for water oxidation. Chem. Sci. 3, 217–229. doi:10.1039/C1SC00516B
Mishra, P. R., Shukla, P. K., and Srivastava, O. N. (2007). Study of modular PEC solar cells for photoelectrochemical splitting of water employing nanostructured TiO2TiO2 photoelectrodes. Int. J. Hydrog. Energy 32, 1680–1685. doi:10.1016/j.ijhydene.2006.10.002
Moliton, A., and Nunzi, J.-M. (2006). How to model the behaviour of organic photovoltaic cells. Polym. Int. 55, 583–600. doi:10.1002/pi.2038
Nandjou, F., and Haussener, S. (2017). Degradation in photoelectrochemical devices: review with an illustrative case study. J. Phys. Appl. Phys. 50, 124002. doi:10.1088/1361-6463/aa5b11
Nandy, S., Hisatomi, T., Takata, T., Setoyama, T., and Domen, K. (2023). Recent advances in photocatalyst sheet development and challenges for cost-effective solar hydrogen production. J. Mat. Chem. A 11, 20470–20479. doi:10.1039/D3TA04353C
Nishiyama, H., Yamada, T., Nakabayashi, M., Maehara, Y., Yamaguchi, M., Kuromiya, Y., et al. (2021). Photocatalytic solar hydrogen production from water on a 100-m2 scale. Nature 598, 304–307. doi:10.1038/s41586-021-03907-3
Nitopi, S., Bertheussen, E., Scott, S. B., Liu, X., Engstfeld, A. K., Horch, S., et al. (2019). Progress and perspectives of electrochemical CO2 reduction on copper in aqueous electrolyte. Chem. Rev. 119, 7610–7672. doi:10.1021/acs.chemrev.8b00705
Nofuentes, G., García-Domingo, B., Muñoz, J. V., and Chenlo, F. (2014). Analysis of the dependence of the spectral factor of some PV technologies on the solar spectrum distribution. Appl. Energy 113, 302–309. doi:10.1016/j.apenergy.2013.07.044
NSRDB (2024). Nsrdb. Available at: https://nsrdb.nrel.gov/ (Accessed March 23, 2024).
Okonkwo, P. C., Ben Belgacem, I., Emori, W., and Uzoma, P. C. (2021). Nafion degradation mechanisms in proton exchange membrane fuel cell (PEMFC) system: a review. Int. J. Hydrog. Energy 46, 27956–27973. doi:10.1016/j.ijhydene.2021.06.032
Olivier, P., Bourasseau, C., and Bouamama, Pr.B. (2017). Low-temperature electrolysis system modelling: a review. Renew. Sustain. Energy Rev. 78, 280–300. doi:10.1016/j.rser.2017.03.099
Pan, F., and Yang, Y. (2020). Designing CO 2 reduction electrode materials by morphology and interface engineering. Energy Environ. Sci. 13, 2275–2309. doi:10.1039/D0EE00900H
Pan, S., Li, R., Wang, J., Zhang, Q., Wang, M., Shi, B., et al. (2023). Floating seawater splitting device based on NiFeCrMo metal hydroxide electrocatalyst and perovskite/silicon tandem solar cells. ACS Nano 17, 4539–4550. doi:10.1021/acsnano.2c10477
Pareek, A., Dom, R., Gupta, J., Chandran, J., Adepu, V., and Borse, P. H. (2020). Insights into renewable hydrogen energy: recent advances and prospects. Mat. Sci. Energy Technol. 3, 319–327. doi:10.1016/j.mset.2019.12.002
Pender, J. P., Jha, G., Youn, D. H., Ziegler, J. M., Andoni, I., Choi, E. J., et al. (2020). Electrode degradation in lithium-ion batteries. ACS Nano 14, 1243–1295. doi:10.1021/acsnano.9b04365
Péron, J., Nedellec, Y., Jones, D. J., and Rozière, J. (2008). The effect of dissolution, migration and precipitation of platinum in Nafion®-based membrane electrode assemblies during fuel cell operation at high potential. J. Power Sources 185, 1209–1217. doi:10.1016/j.jpowsour.2008.06.098
Pishgar, S., Gulati, S., Strain, J. M., Liang, Y., Mulvehill, M. C., and Spurgeon, J. M. (2021). In situ analytical techniques for the investigation of material stability and interface dynamics in electrocatalytic and photoelectrochemical applications. Small Methods 5, 2100322. doi:10.1002/smtd.202100322
Popović, S., Smiljanić, M., Jovanovič, P., Vavra, J., Buonsanti, R., and Hodnik, N. (2020). Stability and degradation mechanisms of copper-based catalysts for electrochemical CO 2 reduction. Angew. Chem. 132, 14844–14854. doi:10.1002/ange.202000617
Salvatore, D. A., Gabardo, C. M., Reyes, A., O’Brien, C. P., Holdcroft, S., Pintauro, P., et al. (2021). Designing anion exchange membranes for CO2 electrolysers. Nat. Energy 6, 339–348. doi:10.1038/s41560-020-00761-x
Samu, A. A., Kormányos, A., Kecsenovity, E., Szilágyi, N., Endrődi, B., and Janáky, C. (2022). Intermittent operation of CO2 electrolyzers at industrially relevant current densities. ACS Energy Lett. 7, 1859–1861. doi:10.1021/acsenergylett.2c00923
Shengsheng, Z. (2006). Effects of freezemhaw cycles and gas purging method on polymer electrolyte membrane. Fuel Cells. doi:10.1016/S1004-9541(07)60015-9
Simon, G. H., Kley, C. S., and Roldan Cuenya, B. (2021). Potential-dependent morphology of copper catalysts during CO2 electroreduction revealed by in situ atomic force microscopy. Angew. Chem. Int. Ed. 60, 2561–2568. doi:10.1002/anie.202010449
Sun, D., Xu, X., Qin, Y., Jiang, S. P., and Shao, Z. (2020). Rational design of Ag-based catalysts for the electrochemical CO2 reduction to CO: a review. ChemSusChem 13, 39–58. doi:10.1002/cssc.201902061
Tembhurne, S., and Haussener, S. (2016). Integrated photo-electrochemical solar fuel generators under concentrated irradiation: I. 2-D non-isothermal multi-physics modeling. J. Electrochem. Soc. 163, H988–H998. doi:10.1149/2.0311610jes
Tembhurne, S., and Haussener, S. (2019). Controlling strategies to maximize reliability of integrated photo-electrochemical devices exposed to realistic disturbances. Sustain. Energy Fuels 3, 1297–1306. doi:10.1039/C8SE00441B
Tembhurne, S., Nandjou, F., and Haussener, S. (2019). A thermally synergistic photo-electrochemical hydrogen generator operating under concentrated solar irradiation. Nat. Energy 4, 399–407. doi:10.1038/s41560-019-0373-7
Todorova, T. K., Schreiber, M. W., and Fontecave, M. (2020). Mechanistic understanding of CO2 reduction reaction (CO2RR) toward multicarbon products by heterogeneous copper-based catalysts. ACS Catal. 10, 1754–1768. doi:10.1021/acscatal.9b04746
Tomić, A. Z., Pivac, I., and Barbir, F. (2023). A review of testing procedures for proton exchange membrane electrolyzer degradation. J. Power Sources 557, 232569. doi:10.1016/j.jpowsour.2022.232569
van Dyk, E. E., Gxasheka, A. R., and Meyer, E. L. (2005). Monitoring current–voltage characteristics and energy output of silicon photovoltaic modules. Renew. Energy 30, 399–411. doi:10.1016/j.renene.2004.04.016
Vos, R. E., Kolmeijer, K. E., Jacobs, T. S., van der Stam, W., Weckhuysen, B. M., and Koper, M. T. M. (2023). How temperature affects the selectivity of the electrochemical CO2 reduction on copper. ACS Catal. 13, 8080–8091. doi:10.1021/acscatal.3c00706
Vos, R. E., and Koper, M. T. M. (2022). The effect of temperature on the cation-promoted electrochemical CO2 reduction on gold. ChemElectroChem 9, e202200239. doi:10.1002/celc.202200239
Walczak, K. A., Segev, G., Larson, D. M., Beeman, J. W., Houle, F. A., and Sharp, I. D. (2017). Hybrid composite coatings for durable and efficient solar hydrogen generation under diverse operating conditions. Adv. Energy Mat. 7, 1602791. doi:10.1002/aenm.201602791
Wang, Z., Li, C., and Domen, K. (2019). Recent developments in heterogeneous photocatalysts for solar-driven overall water splitting. Chem. Soc. Rev. 48, 2109–2125. doi:10.1039/C8CS00542G
Wei, W. J., King, A. J., Bui, J. C., Weber, A. Z., and Bell, A. T. (2023). Co-design of multijunction photoelectrochemical devices for unassisted CO2 reduction to multicarbon products. J. Electrochem. Soc. 170, 126502. doi:10.1149/1945-7111/ad10e7
Weiß, A., Siebel, A., Bernt, M., Shen, T.-H., Tileli, V., and Gasteiger, H. A. (2019). Impact of intermittent operation on lifetime and performance of a PEM water electrolyzer. J. Electrochem. Soc. 166, F487–F497. doi:10.1149/2.0421908jes
Weng, L.-C., Bell, T., and Weber, A. (2018). Modeling gas-diffusion electrodes for CO2 reduction. Phys. Chem. Chem. Phys. 20, 16973–16984. doi:10.1039/C8CP01319E
Xiong, R., Pan, Y., Shen, W., Li, H., and Sun, F. (2020). Lithium-ion battery aging mechanisms and diagnosis method for automotive applications: recent advances and perspectives. Renew. Sustain. Energy Rev. 131, 110048. doi:10.1016/j.rser.2020.110048
Xu, X., Zhong, Y., Wajrak, M., Bhatelia, T., Jiang, S. P., and Shao, Z. (2024). Grain boundary engineering: an emerging pathway toward efficient electrocatalysis. InfoMat 6, e12608. doi:10.1002/inf2.12608
Yap, K. M. K., Aitbekova, A., Salazar, M., Kistler, T. A., Rodríguez Pabón, M., Su, M. P., et al. (2024a). CO2 conversion to butene via a tandem photovoltaic–electrochemical/photothermocatalytic process: a Co-design approach to coupled microenvironments. ACS Energy Lett. 9, 4369–4377. doi:10.1021/acsenergylett.4c01866
Yap, K. M. K., Lee, S.-W., Steiner, M. A., Acosta, J. E. A., Kang, D., Kim, D., et al. (2023). A framework for understanding efficient diurnal CO2 reduction using Si and GaAs photocathodes. Chem. Catal. 3, 100641. doi:10.1016/j.checat.2023.100641
Yap, K. M. K., Wei, W. J., Pabón, M. R., King, A. J., Bui, J. C., Wei, L., et al. (2024b). Modeling diurnal and annual ethylene generation from solar-driven electrochemical CO2 reduction devices. Energy Environ. Sci. 17, 2453–2467. doi:10.1039/D4EE00545G
Ye, J.-Y., Ding, K., Reindl, T., and Aberle, A. G. (2013). Outdoor PV module performance under fluctuating irradiance conditions in tropical climates. Energy Procedia 33, 238–247. PV Asia Pacific Conference 2012. doi:10.1016/j.egypro.2013.05.064
Yigit, T., and Selamet, O. F. (2016). Mathematical modeling and dynamic Simulink simulation of high-pressure PEM electrolyzer system. Int. J. Hydrog. Energy 41, 13901–13914. doi:10.1016/j.ijhydene.2016.06.022
Yin, Z., Peng, H., Wei, X., Zhou, H., Gong, J., Huai, M., et al. (2019). An alkaline polymer electrolyte CO2 electrolyzer operated with pure water. Energy Environ. Sci. 12, 2455–2462. doi:10.1039/C9EE01204D
Yu, W., Young, J. L., Deutsch, T. G., and Lewis, N. S. (2021). Understanding the stability of etched or platinized p-GaInP photocathodes for solar-driven H2 evolution. ACS Appl. Mat. Interfaces 13, 57350–57361. doi:10.1021/acsami.1c18243
Yuan, X.-Z., Li, H., Zhang, S., Martin, J., and Wang, H. (2011). A review of polymer electrolyte membrane fuel cell durability test protocols. J. Power Sources 196, 9107–9116. doi:10.1016/j.jpowsour.2011.07.082
Zhang, Z., Melo, L., Jansonius, R. P., Habibzadeh, F., Grant, E. R., and Berlinguette, C. P. (2020). pH matters when reducing CO2 in an electrochemical flow cell. ACS Energy Lett. 5, 3101–3107. doi:10.1021/acsenergylett.0c01606
Keywords: solar fuels and chemicals, electrochemistry, durability, photovoltaics, hydrogen, CO2 reduction, electrocatalysis
Citation: Yap KMK, Lee SA, Kistler TA, Collins DK, Warren EL, Atwater HA, Jaramillo TF, Xiang C and Nielander AC (2024) Addressing challenges for operating electrochemical solar fuels technologies under variable and diurnal conditions. Front. Energy Res. 12:1483914. doi: 10.3389/fenrg.2024.1483914
Received: 20 August 2024; Accepted: 27 September 2024;
Published: 16 October 2024.
Edited by:
Gianluigi De Falco, DICMAPI - University of Naples Federico II, ItalyCopyright © 2024 Yap, Lee, Kistler, Collins, Warren, Atwater, Jaramillo, Xiang and Nielander. This is an open-access article distributed under the terms of the Creative Commons Attribution License (CC BY). The use, distribution or reproduction in other forums is permitted, provided the original author(s) and the copyright owner(s) are credited and that the original publication in this journal is cited, in accordance with accepted academic practice. No use, distribution or reproduction is permitted which does not comply with these terms.
*Correspondence: Harry A. Atwater, aGFhQGNhbHRlY2guZWR1; Thomas F. Jaramillo, amFyYW1pbGxvQHN0YW5mb3JkLmVkdQ==; Chengxiang Xiang, Y3h4QGNhbHRlY2guZWR1; Adam C. Nielander, YW5pZWxhbmRAc2xhYy5zdGFuZm9yZC5lZHU=
†These authors have contributed equally to this work
Disclaimer: All claims expressed in this article are solely those of the authors and do not necessarily represent those of their affiliated organizations, or those of the publisher, the editors and the reviewers. Any product that may be evaluated in this article or claim that may be made by its manufacturer is not guaranteed or endorsed by the publisher.
Research integrity at Frontiers
Learn more about the work of our research integrity team to safeguard the quality of each article we publish.