- 1Mineral Processing Division, Mintek, Randburg, South Africa
- 2Department of Chemical Engineering, Faculty of Engineering and the Built Environment, Durban University of Technology, Durban, South Africa
- 3Scientific Services, Rand Water, Vereeniging, South Africa
- 4Institute for Catalysis and Energy Solutions, University of South Africa (UNISA), Johannesburg, South Africa
Carbon Capture and Storage (CCS) is recognized as a potent strategy for managing the accumulation of human-generated CO2 in the atmosphere, helping to alleviate climate change’s effects. The CO2 gas is captured from the point source through methods such as pre-treating fossil fuels, oxy-fuel combustion, or post-combustion capture; thereafter; it is transported to a storage location and injected into geological formations. This article provides an overview of carbon dioxide capture and sequestration, focusing on its key principles, technologies, associated risks, and challenges. Direct Air Capture (DAC) and Scalable Modelling, Artificial intelligence (Al), Rapid Theoretical calculations SMART technologies are detailed as emerging and promising approaches to CO2 capture. Numerous pilot and commercial projects commissioned to manage carbon dioxide emissions are presented. Additionally, the paper explores approaches combining geological, geophysical, geochemical, and environmental monitoring techniques to ensure the secure and sustainable storage of CO2 underground. These are essential to address uncertainties, minimize risks, and build public confidence in CCS as a viable climate mitigation strategy. The successful deployment of these technologies on a global scale will require continued innovation, particularly in the areas of monitoring, risk management, and public engagement. Emerging technologies such as AI and SMART systems could play a crucial role in enhancing the efficiency and safety of CCS operations. However, the integration of these advancements with existing infrastructure and regulatory frameworks remains a challenge. Ultimately, a multi-disciplinary approach, combining technological, economic, and regulatory perspectives, will be vital to realizing the full potential of CCS in combating climate change.
Highlights
• Overview of the available and emerging technologies for carbon capture including the computational approach
• Carbon dioxide sequestration in various geological landforms and Carbon Cycling in Terrestrial Ecosystems
• Carbon capture and sequestration pilot and commercial projects in operation around the world.
• Techniques for monitoring leakages of carbon dioxide stored in storage locations
1 Introduction
Increasing levels of atmospheric greenhouse gases are affecting the environment and quality of life, leading to increased global temperatures. The reported carbon dioxide (CO2) concentration increase in 2016 was 3.3 parts per million (ppm) (Olivier et al., 2017). As of December 2023, Mauna Loa Observatory, Hawaii, reported that atmospheric CO2 was at 421.86 ppm and was expected to increase to 422.85 ppm by January 2024 (EarthSky, 2024). In accordance with the Paris Agreement of 2015, countries committed to reducing carbon dioxide emission to a level that keeps the atmosphere temperature increment under 2° relative to the pre-industrial amount so as to arrest adverse consequences of climate change. Most of the existing commercial and laboratory CO2 capture methods are expensive, with nearly 80% of the total cost emanating from the capturing stage (Blomen et al., 2009). Recent investigations are focused on reducing operating costs, reducing the energy penalty, and developing a technology adaptable to any industrial system (Leung et al., 2014; Su and Yang, 2022; Zhang T. et al., 2022). Different methods have been utilized to capture and sequester CO2 emissions from industrial processes. However, there is currently no universally adopted sustainable solution on a large scale. Essential aspects of the Carbon Capture and Storage (CCS) methods are composed mainly of the falling steps, capture, transportation, injection, the safety of the operations to prevent leakages of CO2 after injection and monitoring of the geological landforms (Ramos et al., 2023). Ongoing research endeavours are investigating alternative or enhanced technologies for the capture of CO2. The most known method of CO2 storage is pumping the gas into geological landforms resulting in the formation of solid minerals such as carbonate or permanent seals for CO2 (Martin et al., 2010; Kelemen et al., 2019). This approach has been thoroughly studied and proven through a number of pilot programs and global commercial operations (Aminu et al., 2017).
In the 75th session of the United Nations General Assembly in 2021, China brought forward an initiative to bring about carbon neutrality by 2060. To achieve this, they devised a Digital Elevation Model (DEM) that enables automatic site selection for hydro-pumping energy storage based on geographic information system (GIS) hydrological assessment to eliminate and select potential sites for hydro energy storage (Song et al., 2024). This technique can be modified for the selection of CO2 storage sites eliminating the time consuming and costly field survey based method which is commonly applied. Hence, the use of digital systems and computational techniques is critical in the selection of CO2 gas sequestration sites. However, careful consideration is necessary when pumping CO2 into the soil/underground as it dissolves in aquifer waters forming carbonic acid (H2CO3), resulting in an acidic solution which is unsafe for consumption. Numerous factors affect the chemical reactions taking place in the interaction of acidic water and underground rocks. The main factor being the partial pressures of CO2 and the mixing of different solutions, a phenomenon known as the Bogli effect (Waele, 2017). It is imperative for the injected CO2 in deep geological landforms that it does not leak as it will affect the environment and the economics of the process and put human beings in the proximity of the sites at risk (Milad et al., 2024). Therefore, it is imperative to have sub-surface control to monitor CO2 seeps; hence, the surface topography and geology have to be characterized to have an effective monitoring strategy (Lichtschlag et al., 2021). The main parameters affecting CO2 sequestration in geological landforms are salt caverns, deep geothermal reservoirs, shale formations, basalt formations, unmineable coal seams and deep saline aquifers) are the temperature, CO2 flux, and landform type. It has been noted that leakage of CO2 from the geological storage site is highly influenced by the topography of the landform and the flow properties of the out-cropping rocks. In landforms with low permeability, there are several dry seeps that exhibit substantial fluxes (Masaoka et al., 2016). Different seep types have been observed such as diffuse seeps, bubbling water seeps, and CO2 vents (Roberts et al., 2015).
Several comprehensive reviews have been conducted on CO2 capture and storage technologies. For instance, Kelemen et al. (2019) discussed the challenges associated with CO2 storage in various minerals and geological formations, focusing on the mineralization process, kinetic limitations, costs, and storage potential. Similarly, Ajayi et al. (2019) examined CO2 storage technologies, the physical processes, modelling techniques, capacity estimation, monitoring and verification methods, and the risks and challenges of field- and pilot-scale projects. In another review, Ali et al. (2022) extensively analyzed CO2 emissions and geological storage systems. They highlighted recent advancements in direct quantitative experimental procedures within anoxic rock/CO2/brine systems, the impact of organic contaminants on experimental methodologies, and the role of organics and nanomaterials in rock/CO2/brine and rock/oil/brine systems. Massarweh and Abushaikha (2024) recently focused on the mechanisms involved in geological CO2 trapping. They also discussed the techniques used to assess CO2 sequestration potential before injection and the methods employed for monitoring sequestration progress post-injection. While these studies have significantly advanced our understanding of CO2 capture, storage, and monitoring technologies, a critical gap persists. There is a noticeable lack of comprehensive integration and large-scale optimization of these technologies, particularly regarding economic feasibility and regulatory challenges across different geological settings. Existing research and pilot projects have primarily concentrated on specific CO2 capture and storage aspects, such as mineral carbonation and enhanced rock weathering. However, there is a deficiency in unified frameworks that effectively combine computational models with practical applications, especially for monitoring CO2 leakage and addressing the risks associated with CO2 seepage in diverse geological environments.
This paper seeks to address this gap by thoroughly reviewing current CO2 capture and storage technologies, emphasizing integrating computational models with field data to optimize site selection, storage efficiency, and leakage monitoring. Additionally, the paper will explore the economic, regulatory, and public perception challenges that impede the widespread adoption of these technologies, proposing potential solutions to bridge these gaps and enhance the sustainability and safety of carbon capture and storage initiatives. A more holistic approach integrating technological, economic, and regulatory perspectives is needed to advance the carbon capture and storage field.
2 Carbon dioxide capture technologies
Massive research has been carried out on the removal of carbon dioxide from coal power plants, exploring methods such as pre-treating fossil fuels, oxyfuel combustion, and post-combustion capture, as illustrated in Figure 1 (Sabil and Partoon, 2018; Adu et al., 2019; Dikhanbaev et al., 2024). Oxyfuel combustion entails the substitution of air with oxygen, thereby decreasing thermal nitrogen oxides (NOX) (Kuznetsov et al., 2023). In pre-combustion, the fossil fuel undergoes treatment before combustion, typically in a gasifier with reduced oxygen, resulting in syngas containing CO and H2. Post-combustion processes employ adsorption and absorption for CO2 removal from the combustion gases after combustion. Adsorption employs solid absorbents like calcium oxides, activated carbon, hydrotalcite, lithium zirconate, molecular sieves, and zeolites to bind CO2 to their surfaces (Wall, 2007). Nandi and Uyama (2014) conducted a comprehensive literature review on materials exhibiting high adsorption capacities for carbon dioxide under varying conditions, temperatures, and pressures. The materials were classified into five categories: porous carbon, porous organic frameworks, zeolite, metal-organic, and mesoporous silica. The study revealed that the gas adsorption characteristics are notably influenced by surface area, pore volume, and the presence of moieties containing O-, S-, and N-. The adsorption of the gases is facilitated by the interaction between framework and CO2 molecules involving H-bonding, dipole-quadrupole interactions, and the presence of a microporous structure. Most industries employ the absorption method for capturing CO2 (Atkins and De Paula, 2010; Leung et al., 2014; Petrovic et al., 2022). Various chemicals, including methyl diethanolamine (MDEA), diethanolamine (DEA), monoethanolamine (MEA), potassium carbonate, dimethyl ether of polyethylene glycol (DEPG), cetyltrimethyl ammonium bromide (CTAB), 1-butyl-3-methylimidazolium tetrafluoroborate, dialkyl imidazolium cations, propylene carbonate, and sodium hydroxide have been investigated to enhance the absorption volume of CO2 (Cowdhury et al., 2013; Bui et al., 2018b; Einloft and Bernard, 2020). However, these technologies tend to be energy-intensive. Consequently, extensive global research, innovation, and development efforts are underway to create more efficient and cost-effective post-combustion systems (Bertz et al., 2005). With the relaxation in electricity prices, there is the possibility for benefits of the diffusion of CCS technology. Therefore, adopting renewable energy technologies will assist in lowering the price of electricity price, hence cutting down on the potentially high prices for the expansion of the CCS technology (Lackner, 2009; Tan et al., 2023).
The CO2 capture methods such as mixed matrix membrane, gas hydrate separation methods, chemical-looping combustion, enzyme-based separation, and integrated gasification combined cycle (IGCC), are promising in CO2 capture from combustion gases; these are described in Table 1 (Mondal et al., 2012; Li et al., 2013; Khalik, and Behzad, 2018; Al-Mamoori et al., 2018; Wang and Song., 2020; Zhang et al., 2020; Ndlovu et al., 2024a; Leonzio, and Shah, 2024).
A new technology is emerging in the market where carbon dioxide is extracted from the atmosphere using direct air capture technology (DAC). This commercial technology has been applied by companies such as Clime Works and Carbon Engineering (Lackner, 2003; Haszeldinez, 2009). Contrary to the capture of carbon dioxide from generation point sources that handle large amounts of CO2 in high concentrations, the capture of CO2 from the atmosphere is cumbersome due to lower CO2 concentrations. Membrane technology is considered a promising approach in direct air capture (DAC) technologies due to its minimal environmental impact and reduced operational and energy costs in comparison to current sorbent-based systems (Ozkan, 2021; Zhu et al., 2022; Ignatusha et al., 2024).
The current design of DAC utilizes fans to suck in air containing CO2, which flows past a contactor or a filter with a solid or liquid sorbent material that selectively binds CO2 molecules. The CO2 molecules can be adsorbed on the solid sorbent (amine-functionalised porous material) or absorbed in a liquid sorbent (aqueous solutions of amines or alkali salts). When the sorbent is saturated with CO2, it is regenerated to release the captured CO2, usually by heat, pressure, or chemical reactions (Ammendola et al., 2017; Siegelman et al., 2021; Fatima et al., 2021; Sodiq et al., 2023). The advantage of the DAC technology is the ease of mobility as it is independent of the emission source. Hence, the machine can be located in proximity to the storage or utilization site (Ozkan et al., 2022; Abdullatif et al., 2023). The environmental impact of the DAC can be detrimental if the energy source is fossil-based. Moreover, due to the complexity of the technology and the requirement for extensive infrastructure, DAC system installation, operation, and maintenance can be costly. This could impede deployment and broad adoption (Zhao et al., 2023).
2.1 Computational approach to carbon capture
Computational tools and machine learning (ML) have been widely used in carbon capture and storage (CCS) for sequestration purposes, but their application specifically to carbon capture has been less extensive. ML has been used most for post-combustion CO2 capture (PCC) predictions and optimizations. Recently, Hosseinpour et al. (2023) carried out a literature review on the application of ML methods for PCC adsorption-based techniques and provided a roadmap for future direction in applying these technologies. Rahimi et al. (2021) employed ML to predict the thermodynamic properties of the absorption process, the most cost-effective process scheme, and the design of the process configuration. Wu et al. (2020) developed an intelligent predictive controller (IPC) for a large-scale solvent-based PCC with artificial neural network and particle swarm optimization programs. The IPC was configured to manipulate the operation conditions, thus improving the CO2 captured during the process. Li et al. (2015) modeled post-combustion CO2 capture using bootstrap aggregated neural networks, which could predict CO2 capture capacity and rate based on the model input variables such as pressure and temperature of the flue gas, the inlet flow rate of CO2, the concentration of the inlet flue gas, lean solvent flow rate and concentration. In similar, Li et al. (2018) examined the post-combustion capture of CO2 with deep learning modeling machine-learning tool with an unsupervised pre-training phase [with deep belief network (DBN)] and a supervised back-propagation phase. The DBN model predicted CO2 production and capture levels using model operation variables presented by Li et al. (2015).
Molecular simulations and process modeling to identify the best adsorbent for carbon capture applications have been carried out through multiscale material screening strategies. Challenges such as the interfacial interaction of molecules were reported (Farmahini et al., 2018). Machine learning models [Matern Gaussian Process Regression (GPR), fine tree, rational quadratic GPR, squared exponential GPR models] were used to predict the outcomes of the PCC unit. The models resulted in a 98% accuracy in predicting the model outcomes, with optimum conditions determined through the utilization of a Sequential Quadratic Programming algorithm (SQP) and Genetic Algorithm (GA) (Shalaby et al., 2021). The utilization of monoethanolamine (MEA) for post-combustion capture of CO2 in the flue gas from an absorption column was analysed using a Support Vector Machine (SVM) and Artificial Neural Network (ANN). The derivative-based sensitivity analysis showed that absorbent-based input variables such as lean solvent flow rate and temperature were the most significant input variables on CO2 capture (Ashraf and Dua, 2023). Jablonka et al. (2023) utilized ML models to predict the emission of a power plant operating with 2-amino-2-methyl-1-propanol and piperazine (CESAR1). It was observed that there were adverse effects on the solvent as the amine emissions from a solvent-based carbon capture plant are complex. A new interdisciplinary method called Scalable Modelling, Artificial Intelligence, Rapid Theoretical (SMART) has been introduced. This approach integrates data-driven modeling with expertise in environmental science, chemical materials, data science, computer science, and engineering principles. This enables SMART to have advanced capabilities in optimizing and simulating carbon capture operations (Lei et al., 2023). This approach integrates data-centric modeling, high-throughput calculations, and expertise from multiple disciplines, in contrast to previous reviews that only focused on materials or computational techniques (Golze et al., 2022; Nassef, 2023). This holistic approach provides an in-depth understanding of the carbon capture landscape. Therefore, the SMART technology approach is set to aid industries’ energy transition and decarbonisation.
3 Carbon dioxide storage in geological landforms
The advancement of CCS technologies allows for meeting electricity demand with a minimal carbon footprint. Numerous initiatives and projects have been launched, with a significant number located in the United States. The geological storage of captured carbon dioxide in deep aquifers or depleted oil and gas reservoirs as a method for sequestering CO2 is a well-established technology (Bui et al., 2018b; Budinis, et al., 2018; Kelley et al., 2018; Shell Canada, 2019; Gentzis, 2000; Arlota and Costa., 2021; Harati, et al., 2023). From the syngas before combustion or from the flue gas after combustion, which can then be stored or used to enhance oil recovery (Kumar and Kumar, 2018). The IGCC process that has been described earlier captures CO2 emissions by separating them. Moreover, the injection and storage of CO2 in porous and permeable underground landform formations in the North Sea at Sleipner West gas field have been proven secure, safe, and socially satisfactory, as confirmed by public acceptance (DW Documentary, 2021; Holloway, 2005).
Geological features like aquifers, coal seams, depleted oil, basalt formations, gas reservoirs, salt caverns, and organic shales exhibit significant potential for CO2 storage. This potential is realized through diverse trapping mechanisms such as hydrodynamic processes, dissolution, mineralization, and primary chemical adsorption (Al Hameli et al., 2002; Wu et al., 2023; Shi et al., 2005; Godec et al., 2014; Liu et al., 2019; Bachu and Adams., 2014; Michael et al., 2017; Iglauer, 2017; Vishal and Singh, 2016; Metz et al., 2005). Organic shells (corals and molluscs) have a low capacity for CO2 sequestration but sequestrate CO2 through the formation of carbonate sediments by the organic shell which accumulates on the ocean floor leading to the formation of carbonate sediments (Filgueira et al., 2019). The reservoirs that have been utilized for CO2 storage are made from sandstone or carbonates (e.g., limestone or dolomite), iron, or other minerals that promote CO2 binding to its structure, and CO2 can be injected to recover unrecovered oil or recovery of CH4 from coal beds (Haq et al., 2023). The fluid in the pores of saline aquifers contains various compositions of ionic solutions with elements such as magnesium, calcium, potassium, iron, chlorine, sulphate, and other minerals. The presence of these elements and ions provides a vast number of reactions that can enhance mineral trapping with diverse reaction kinetics (Riaz, and Cinar, 2014; De Silva et al., 2015). Moreover, it has been observed that basaltic geological formations exhibit reactivity with H2O-rich supercritical carbon dioxide and the formation of H2O with dissolved CO2. This reactivity leads to the generation of carbonate minerals, ensuring long-term and secure permanent sequestration (McGrail et al., 2009). Carbon mineralization is the most common reaction when CO2 is injected into the geological landform. This is a process of a long-lasting solution for carbon dioxide storage in the solid form as it has minimal environmental health and environmental implications. Moreover, as carbonated solids with base metal form in underground rock, there are minimal risks of leakages (Isahak et al., 2015; Zhang and DePaolo, 2017; Kelemen et al., 2019).
3.1 Geological trappings
Schematic illustrations of various methods and technologies utilised in carbon sequestration are shown in Figure 2. The CO2 gas remains trapped inside a reservoir by four main mechanisms: mineral trapping, residual trapping, structural trapping, and solubility trapping. Mineral trapping occurs when mineral ions (Mg2+, Ca2+, Fe2+) in the reservoir react with dissolved CO2 to form a solid carbonate. In residual trapping, CO2 gas is confined within the spaces in the rock grains, while structural trapping is the physical trapping of the gas within rocks (Ali et al., 2022). The CO2 trapping mechanisms are shown in Figure 3. Mineral trapping takes longer than the other forms of CO2 trapping and takes place over a long period, resulting in increased CO2 storage security with time from the injection. Dissolution trapping is another mechanism for enhancing the storage security of supercritical CO2 in saline geological formations. This occurs when the supercritical CO2 dissolves in the formation brine, producing a denser solution than the formation brine, resulting in gravity-enhanced convective mixing, enhancing CO2 dissolution and trapping. In heterogeneous geological formations, density-driven flow does not cause significant mixing, especially in layered systems, whereas in homogenous formations, density-driven flow enhances both the storage and the contact between the dissolved CO2 and the host rock (Agartan et al., 2015).
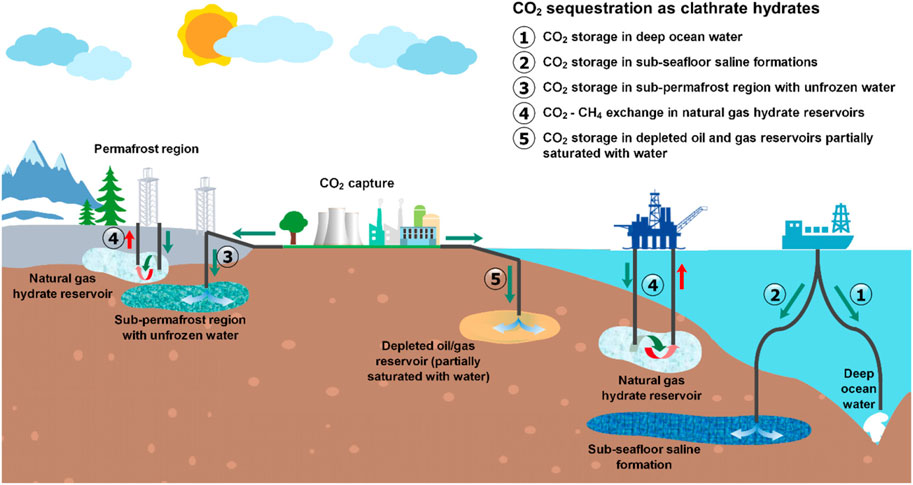
Figure 2. The various storage options available for CO2 storage (Zheng et al., 2020).
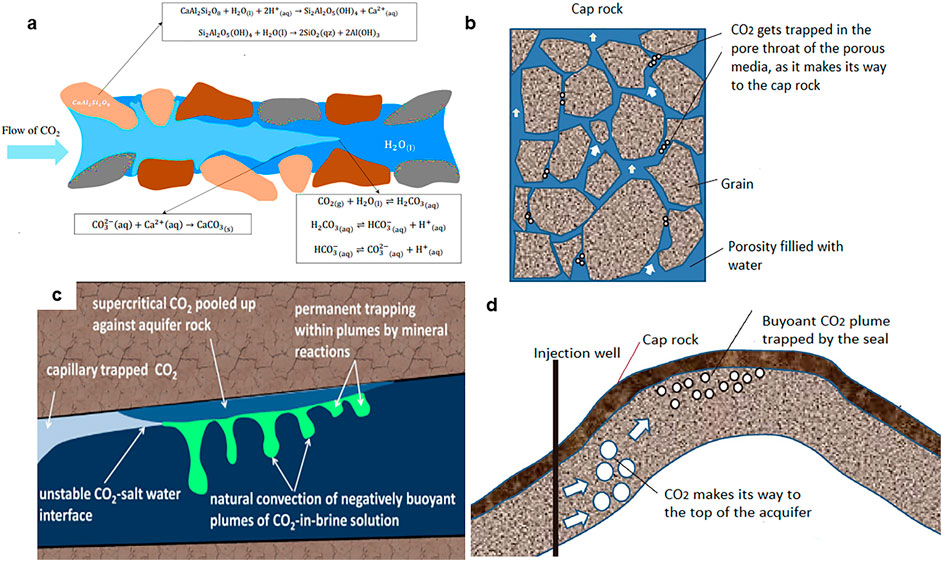
Figure 3. Carbon dioxide trapping mechanisms in geological locations; a) mineral trapping b) residual trapping c) solubility trapping with residual trapping d) physical trapping (Riaz and Cinar, 2014; Nomeli, 2014; Ajayi et al., 2019).
Huang et al. (2019) carried out a study on hydrocarbon (oil or gas molecule) release from shale formations through the injection of supercritical CO2 into shale formations at high pressures, with CO2 being adsorbed on the nanopores. As shown in Figure 4, as supercritical CO2 is injected into shale, a rich organic rock composed mainly of nanoporous organic matter, it creates fractures (black trees) in the shale formations (brown). The supercritical CO2 then flows in the channels formed in the rocks, displacing the gas and the oil (shown in orange and blue). Then, it finally adsorbs and diffuses into the shale matrix and pores (grey and black), the driving force being its affinity for the shale. When the injection period is over, CO2 is trapped underground, resulting in the reduction of greenhouse gases in the atmosphere. Cole et al., 2010 reported that nanoporous environments with shale or clay-rich mudstones have seal rocks and cap seals that seal CO2 underground and prevent leakage. The nature of the confined geometrics, such as hydrophobicity and hydrophilicity, the chemical structure of the fluids, and their physical properties affect the reaction of the fluid with the environment. The study of adsorption in nanoporous materials such as muscovite and silica aerogel using the Adsorbed Phase Model (APM) showed fluid depletion for conditions above the critical density. The CO2 can be sequestrated in significant amounts at very low pressures, and the density of physisorbed CO2 could be three times higher than that of the bulk fluid. With molecular dynamics, simulation studies provide insights into the supercritical CO2 adsorbed in the muscovite layers of sequestration reservoirs. The space between the cleaved muscovite surfaces with a separation of 5 Å provides a pathway that cannot permanently store CO2 but allows free gas diffusion. Moreover, for permanent storage, the molecular muscovite must be in a single layer in the crystalline structure or in thicker layers in the carbonate form.
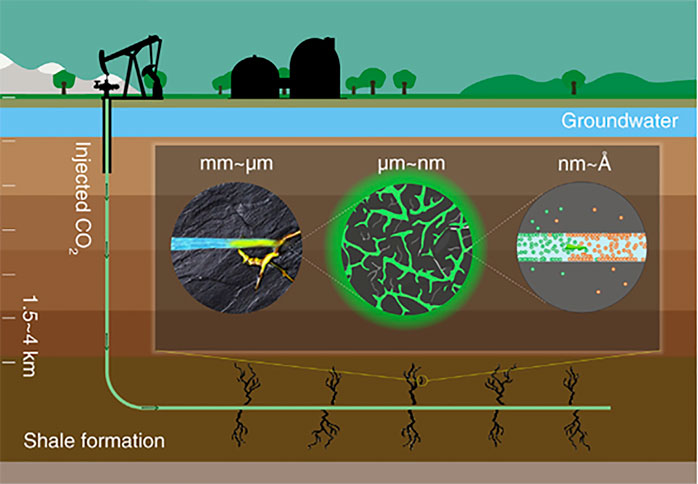
Figure 4. Pictorial depiction of multiscale interaction of supercritical CO2 (green) with nano porous shale (Huang et al., 2019).
The injection of supercritical CO2 has numerous advantages in geothermal explorations when compared to heat transmission fluids such as water. In its supercritical state, CO2 has high heat capacity and low viscosity, which can increase its injectivity. Moreover, CO2 has higher expansiveness and compressibility than water, increasing the buoyancy force in the wellbore (Pruess, 2008; Cui et al., 2016). Through experimental studies, it was observed that supercritical CO2 in sandstone leads to the dissolution of ankerite and clay minerals and the precipitation of plagioclase, increasing Ca2+ and Mg2+ in the formation of water. In carbonate reservoirs, CO2 causes dolomite dissolution and precipitation of ankerite and calcite. In addition, 3D reactive transport simulation models were created to assess how geochemical reactions affect heat-mining rates during geothermal exploitation with supercritical CO2 and evaluate CO2 storage potential. Simulation results showed that geochemical reactions influence the flow behaviour and heat mining rates differently depending on reservoir type and mineral composition. In sandstone, mineral precipitation reduces porosity and heat-mining rates, while in carbonate reservoirs, the dissolution of dolomite and clay minerals can enhance the heat-mining rate despite the precipitation of calcite and silicate minerals (Cui et al., 2017).
The affinity of CO2 to replace methane molecules within hydrates has presented a promising avenue for addressing the issue of CO2 emissions into the atmosphere. This can be achieved by extracting fuel stored in natural hydrates while storing CO2 (Seo et al., 2013). Gas hydrates are non-stoichiometric compounds produced through the physical interaction of guest molecules (CH4, H2, and CO2) and water molecules under high-pressure and low-temperature conditions (Sloan, 2003). Solidified natural gas in the form of gas hydrate has the capacity to store large volumes of gas in solid form (180 m3 per 1 m3 of hydrate) (Khokhar et al., 1998). The high storage capacity of the solid gas hydrates is based on their lattice structure which has the capacity to store large amounts of gas in a relatively small compact volume. The stored potential energy in natural gas hydrates represents a closed-cycle energy form where the CH4 molecule is released and burned to produce CO2, which is then captured and used as a substitute for the CH4 in natural gas hydrates, providing a solution to address the issue of CO2 emissions. Injection of CO2 into the CH4 gas hydrates is a promising technique being researched which can simultaneously store CO2 while releasing CH4 gas (Hildenbrand et al., 2002; Ota et al., 2005; Zhou et al., 2015; Zhang et al., 2017; Ouyang et al., 2020; Ndlovu, 2022; Fan et al., 2022). The storage of CO2 in the sea bed and deep water has proven to be an ideal place for CO2 hydrate formation as it is also a solid CO2 storage option; there is slower dissolution due to the surrounding waters, and the hydrate cements the porous sediments. The challenges faced by this option include a lack of knowledge of the hydrodynamics and permeability and the kinetics/thermodynamics of CO2 hydrate formation in natural seabeds. In addition, there is missing data on the long-term stability of the CO2 gas hydrates and the lack of effective toll for the geographical assessments of ideal geolocation for CO2 pumping to form gas hydrates (Castellani, 2023; Nordbotten et al., 2024 conducted a double-blinded forecasting lab-scale experimental study to assess the uncertainty of geological carbon storage. The study forecasted the physical properties of an active field, and the opinions of seasoned researchers were used to guide the research direction.
3.2 CO2 storage through mineral carbonation
The fundamental concept of mineral carbonation is that all the atmosphere, vegetation, and ocean emanate from the rock, and it ultimately ends up in the rock where it began, which is portrayed in the carbon cycle, where the movement of carbon is detailed. Hence, the mineral storage of carbon is the most permanent solution for carbon storage (Snaebjörnsdóttir et al., 2018). Basalt and ultramafic rocks are the most effective rocks for natural mineral carbonation as they are highly reactive and abundant. Basaltic rocks account for 70% of the earth’s surface, most on the ocean floor. The weathering of the basaltic rock is responsible for the natural reduction of CO2 from the atmosphere through silicate weathering on the continents and volcanic islands exhibiting excellent potential for mineral carbonation (Dessert et al., 2003). Rogers et al. (2006) reported on extensive carbonate mineralisation of petroleum substances migration, which contains CO2-bearing fluids altering basalt rocks as they flow, initiating carbon mineralisation.
Mineral carbonation occurs through the interaction of a mafic or ultramafic rock with water containing dissolved CO2. This solution is acidic with a pH of about 3–5 and promotes the dissolution of cations in silicate minerals to form stable carbonate minerals (Saldi et al., 2009). Other factors affecting carbon mineralization are permeability or porosity of the host rock formation, which allows the migrating fluid to percolate the body of the rock and be able to precipitate the carbonates available. Temperature is another factor that affects the mineralization process; for example, dissolved Mg precipitate as dolomite (CaMg(CO3)2 and carbonate magnesite (MgCO3) at temperatures exceeding 80°C (Saldi et al., 2009). This reaction is kinetically inhibited at lower temperatures by stable hydrous Mg-carbonate minerals such as hydromagnesite, nesquhinite, and dypinite (Turvey et al., 2018).
The utilization of natural minerals or waste from mineral processes and industrial furnaces through mineral carbonation has been shown to be the key to attaining sustainability in reducing the carbon footprint and waste management in industrial processes. The wastes that can be managed through this process are coal fly, red mud, blast furnace slags, steel slags, and waste gypsum, which can be managed by indirect and direct aqueous carbonation routes. The schematic representation of the carbonation processes is shown in Figure 5. This figure presents the sources of mineral waste carbonation and the source of CO2 gas.
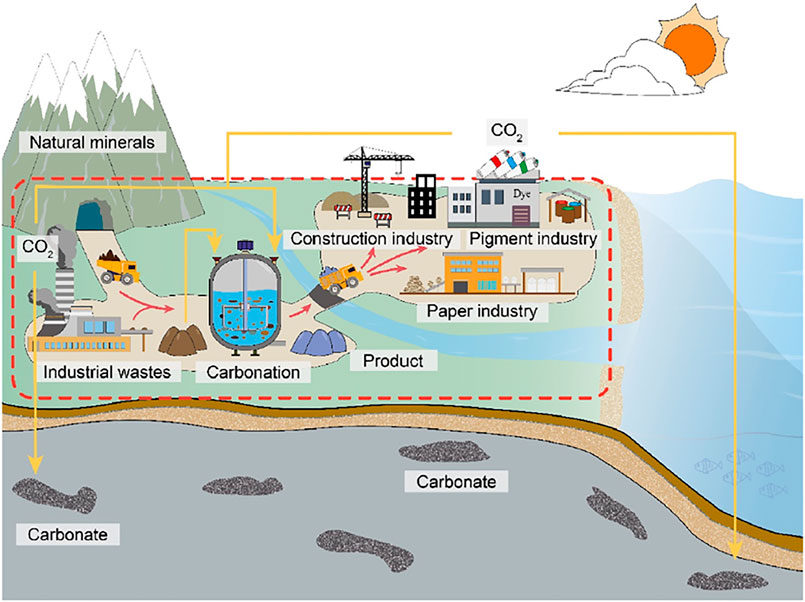
Figure 5. Schematic representation of mineral carbonation for industrial and mineral waste (Lin et al., 2024).
Carbonation of industrial solid waste (mainly from mining, sludge from wastewater treatment and construction industry) occurs through both direct and indirect routes. Due to the high composition of MgO and CaO in industrial solid waste, the carbonation process is effective, making the method ideal for CO2 storage. When CO2 reacts directly with industrial solid waste, the process is known as direct carbonation and may occur through gas-solid carbonation or direct aqueous carbonation. In indirect carbonation, the process occurs through two stages, which are the utilization of an acidic solution to extract Mg and Ca from minerals and then the reaction of Ca and Mg solution CO2 in an alkaline solution to precipitate a carbonate (Bobicki et al., 2012).
Steel slag has been studied in depth in comparison to other waste materials for the CO2 carbonation process, with a steel slag capacity of 536 g/kg (mass of CO2 sequestrated to a mass of steel), while blast furnace had a capacity of 361 g/kg (Lin et al., 2024). Natural minerals required for the carbonation process are serpentine (Mg3Si2O5(OH)4), wollastonite (CaSiO3), and olive (Mg2SiO4). The peridotite rock is mined and prepared by crushing for the sole purpose of CO2 capture and storage. This method has been reported to be able to store about 0.2 GtCO2/yr. However, due to the cost associated with the mining process, this method might be expensive and unsustainable in the long run compared to industrial waste (Monkman et al., 2018; Sun et al., 2021; Pan et al., 2020). In a separate investigation, Molahid et al. (2023) utilized a mineral waste sample containing Fe-oxide (haematite and magnetite) and Ca-Mg-silicates (anorthite, wollastonite, diopside). The compound Fe2O3 was between 39.52%–62.94%, while CaO was in the range of 7.19%–15.24%, and 0.10%–9.58% was MgO. The Ca and Fe carbonation efficiency was found to be 2.38%–6.31% and 4.04%–6.45%, respectively. This resulted in 63.99–156.04 g CaCO3/kg and 60.94–155.44 g FeCO3/kg, which are permanently stored in the mine waste. Overall, about 12.27–44.92 g of CO2 per kg of a mining sample can be sequestrated.
Cement and concrete are another promising area for CO2 sequestration through mineral carbonation. The cement industry is known to be one of the highest CO2-releasing processes from kilns through the decarbonation of limestone and fuel combustion (Habert et al., 2020). The use of supplementary cementitious materials from industrial processes as a cement substitute has been proposed to mitigate CO2 emissions; however, these have not achieved the desired outcomes (Juenger et al., 2019). Through natural carbonation, Portland cement contains calcium hydroxide, which can make it possible for concrete to continuously absorb CO2 from its surroundings, thereby maintaining a high pH and preventing the corrosion of steel reinforcements. However, natural carbonation is slow and can lead to the carbonation front reaching and corroding the steel reinforcement. A more attractive route is accelerated carbonation of concrete; CO2 is injected into fresh concrete in a process known as carbonation mixing. A nanocrystalline amorphous CaCO3 enhances the reaction and compressive strength; though the amount of CO2 stored through this method is limited, it does reduce the amount of CO2 released (Winnefeld et al., 2022; Zajac et al., 2022). Carbonation curing in concrete applies to the gain strength and pore structure densification. Carbonation curing of concrete can be applied to fresh and hardened concrete, which leads to less cement, which decreases its production (Thonemann et al., 2022).
3.3 Enhanced rock weathering (ERW)
Enhanced Rock Weathering (ERW) involves applying finely ground silicate minerals and rocks, such as basalt and glauconite, to soil surfaces. This speeds up the natural weathering process, which typically unfolds over millions of years, while also enhancing the soil’s capacity to capture CO2 (Schuiling and Krijgsman, 2006). This method is increasingly gaining ground as both a carbon dioxide removal technique, soil fertility enhancement, and a reduction technique in ocean acidification, making it favourable as a geoengineering tool that addresses climate change (Swift, 2001). The key difference between enhanced rock weathering and mineral carbonation is that the former is dependent on the natural weathering process, which is enhanced by mineral application, while the latter relies on the direct chemical reaction between minerals and CO2. Moreover, ERW improves soil fertility and sequestrates CO2; on the other hand, mineral carbonation primarily focuses on CO2 storage (Paulo et al., 2021). An integrated modeling approach was utilized to make the techno-economic assessment for the year 2050, focussing on carbon dioxide removal (CDR), cost and deduced that China, United States, and Brazil have the potential to achieve CDR of 0.5–2 gigatonnes per year with an estimated cost of US$80–180 per tonne of CO2 (Beerling et al., 2020).
The majority of enhanced rock weathering (ERW) studies are primarily theoretical, as they involve long-term projections. A simulation study projected that ERW on croplands can achieve a 2 Gt CO2 yr−1, doubling the probability of meeting the 1.5°C temperature increase set at Paris by 2,100. The high breed of ERW and CCS has the potential to reverse the effects of atmospheric CO2 in a range of 23%–67%. Moreover, ERW will increase coral reefs by 16%–39% by 2,100 (Vakilifard et al., 2021). In another simulation study, it was shown that ERW working with a CO2 fixation rate of 10 tons of basalt dust per hectare on the global cropland, over 200 gigatons of CO2 could be sequestrated over a period of 75 years (Baek et al., 2023).
3.4 Computational studies on carbon sequestration
The research on CO2 sequestration using computational techniques is more extensive and detailed than carbon capture studies (Lackner, 2003; Gerstenberger et al., 2009; Neal et al., 2014; Nomeli, 2014; Hung et al., 2019). In computational studies on carbon sequestration, techniques for absorbing and storing CO2 are analysed and optimized using sophisticated models and simulations. The key focus area for these studies includes; modeling and simulating the injection and storage in geological landforms such as coal seams, deep saline aquifers, and depleted gas and oil fields (Celia et al., 2015), as well as behaviour prediction of how CO2 interacts with the rocks and surrounding fluids in the storage sites overtime (Iglauer, 2017). Moreover, it focuses on examining the effectiveness and capability of various materials and sequestration methods for absorbing and retaining CO2 (Cao et al., 2020).
Davoodi et al. (2023) carried out a literature review on the utilization of ML in CO2 sequestration, the most common logarithms such as extreme learning machine (ELM), artificial neural network (ANN), long short-term memory (LSTM), random forest (RF), support vector machine (SVM) and extreme gradient boosting (XGB). Kalam et al. (2021) reviewed the modeling, experimental, and field studies on storing CO2 underground. It was reported that adsorption, chemical reactions, wettability, and hysteresis influenced CO2 sequestration in geological. A model developed by Zhou et al. (2008) estimated the capacity of the CO2 storage capacity in closed and open reservoir systems. In addition, Zhang et al. (2011) developed a model to estimate the amount of CO2 gas that can be stored in oil reservoirs. Bemani et al. (2020) developed hybrid models to analyse the physical properties of CO2, which are crucial in ensuring the security and safety of carbon storage. Wu and Li (2020) modelled CO2-capture processes and forecasted reservoir capacity using different distributions of influencing variables. You et al. (2021) carried out a study on forecasting the performance of enhancing oil recovery using CO2, while Wu et al. (2021) examined the mechanical stability of caprock and reservoirs. Yao et al. (2023) predicted the physical properties of CO2-rich captured gases, reservoir pressure, leakage, and deformation of the CO2 stored in geological landforms.
The study of sedimentary basin for securely storing injected CO2 gas through the geochemical reaction has been carried out through models which focus on the transport and reaction kinetics of the CO2. It was observed that parameters of significant effects were the reactive surface of the mineral, the in-situ pH of the water with dissolved CO2, and the apparent activation energies utilized to model reaction rates to the temperature of the storage reservoirs (Black et al., 2015). A mathematical tool was utilized to examine the Joule-Thompson (JT) effects on CO2 geo-sequestration, assuming a constant gas injection rate. It was observed that if the reservoir pressure is less than 0.2 MPa, the JT effect is experienced, which further decreases the temperature of the reservoir, leading to the formation of gas hydrates with the moisture or residual water. The presence of CH4 gas in the landforms counteracts the cooling effects, though high-pressure and low-temperature combinations are likely to result in gas hydrate formation (Böser and Belfroid, 2013; Loeve et al., 2014; Twerda et al., 2018; Mathias et al., 2010). Using a numerical simulation, Birkholzer et al. (2009) investigated the impact of large-scale CO2 storage with an injection rate of 1.52 million tons annually in an open saline aquifer. The CO2 plume migration is relatively small, at about 2 km, and is concentrated on the top of the saline aquifer due to the buoyancy effect, according to their results, which show that there is significant pressure buildup in the formation of more than 100 km away from the injection zone. It has been definitively determined through various models that depleted gas reservoirs are preferable to depleted oil reservoirs because they offer a greater storage capacity per pore volume and higher gas compressibility (Barrufet et al., 2010; Stein et al., 2010; Bachu, 2007 reported that coal beds of depth between 300 and 900 m were ideal for CO2 sequestration. In recent years, the use of computational models, numerical analysis, and artificial intelligence approaches has become pivotal in selecting ideal sites for CO2 sequestrations, Table 2 details the recent studies published on CO2 storage and sequestration studies using theoretical approaches.
3.5 CO2 capture and storage pilot studies
The carbon capture and sequestration techniques presented in this article have been applied globally on different scales to control carbon dioxide emissions. Due to the high initial cost and energy-intensive nature of the operations, most of the CCS initiatives have been abandoned and discontinued (Huaman and Jun 2014). However, a large number of companies and research facilities have come up with a pilot and experimental set-up to capture carbon dioxide. Table 3 summarises the pilot and experimental applications of carbon capture technologies. Chen and Shen. (2024) highlight that selecting CCUS project sites is challenging due to government policies, ecological considerations, and economic conditions. This creates a multi-criteria decision-making problem, where the information used may be unreliable, leading to inaccuracies and uncertainties that affect the risk management process in decision-making. To counteract these shortcomings, an E-VIKOR method that caters for incomplete and fuzzy available information for multiple decision-making was utilized for selecting CCU storage sites in Guangdong province. Using the information given, the computational approach has the capacity to identify suitable sites under different risk preferences. A more accurate approach to CCU selection is crucial, especially for countries such as China, which have small CCUS projects (Zhang, 2021).
3.5.1 Sequestration in deep saline aquifers/reservoirs
The most known successful initiative of storing CO2 in geological locations is in the Sleipner gas field in the North Sea. This project was commissioned in 1996, and 1 Mt of CO2 is sequestrated annually. The CO2 is injected into the Utsira formation, a saltwater-bearing sand layer 1,000 m below the sea floor. This formation, characterized by its large high-permeability sand body, spans a depth of 500 to 1,500 m and covers an area of 26,000 km2. It is capped by a shale layer 50–100 m thick, which extends beyond the current CO2 injection zone. Due to its low permeability, the shale layer effectively seals the CO2. The cap rock is not uniform but features various domal and anticline structures that act as traps or channels, influencing CO2 migration within the reservoir (Chadwick, 2013; Williams and Chadwick, 2021). Simulation studies were also carried out in the geological settings of Sleipner Vest Field in the Norwegian part of the North Sea. In this study, the injection schemes’ aquifer characteristics for improving permanent sequestration and flow conditions on CO2 storage were carried out. It was observed that heterogeneity of the sediments improved the trapping of CO2, whereas the homogenous formations enhanced CO2 dissolution, as the fluid flow is faster in this type of porous media. Moreover, cyclic injections had more impact on the heterogeneous porous media as they increased the capillary pressure, which aided the entrapment of CO2 in smaller-sized pores, leading to dissolution in the brine later in its storage. In addition, the CO2 storage efficiency was observed to increase with the vertical-to-horizontal permeability ratio of geological formations as higher ratios increase the integrated gas’s solubility (Khudaida and Das, 2020).
A pilot project known as the Northern Reef Trend, which employed pipeline transportation and pumping of CO2 into depleted carbonate reservoirs, was initiated in 2013. It was estimated that over 1 million tons of CO2 would be stored in 5 years (Fagorite et al., 2023). A study was carried out in the Alberta Basin to identify and characterize a geological storage site of more than 800 m. The criteria used to select the site were the ease of accessing the site for CO2 storage (injectivity and permeability), cost of the whole procedure (economics), storage of the gas (containment), protection of the environment from containment, and the total volume of the pore space (capacity). It was found that the Heartland Redwater Leduc Reef was the best site for CO2 containment (Gunter et al., 2009). A feasibility study was carried out on the Northern Michigan Basin (CarbonSAFE–Northern Michigan Basin CS-NMB) storage complex. In this study, the Integrated CCS Pre-feasibility, Site Characterization, and Storage Complex feasibility were accessed. It was demonstrated that the complex had the capacity to store over 50 million metric tons of CO2 (Kelley et al., 2018).
Numerical modeling has been carried out with a multiphase fluid flow of sustained CO2 injection into the deep sand aquifers of Wyoming’s Powder River basin. It was observed that CO2 migration and residence results in an unplanned impact of brine displacement on an extended scale, potentially moving to adjacent sealing layers. The basin aquifer was computed to be able to store CO2 for over 1,000 years (McPherson and Cole, 2000). Another modeling study was carried out on the Alberta Basin, focussing on the interaction between the charged CO2 and the carbonate rock in the aquifer. It was observed that CO2 leads to rapid dissolution of the calcite and dolomite precipitation, which might be essential in CO2 sequestration (Ghafoori et al., 2017).
3.5.2 Sequestration in oil/gas wells
There are numerous oil/gas recovery-enhancing projects simultaneously sequestrating carbon dioxide. A pilot test study was carried out in the Chang 9 tight reservoir of Baibao oilfield located at the Ordos Basin in China for the CO2 –EOR. Two procedures were applied: injection through nine wells and through two newly drilled wells via continuous injection (CI) and water alternative gas injection (WAG). The simulation results showed that WAG increased oil production by 7.9% and 16.2% for the two- and nine-injection processes, respectively. In contrast, CO2 storage in the WAG procedure was decreased by 9.6% and 15% for the 2-and nine-CO2 injection well scenarios, respectively. Overall, it was observed that the WAG has a negative effect on CO2 storage (Ren et al., 2023). Another oil-enhancing project in operation is Project Salah in Algeria, which stores 1.2 mega tonnes of CO2 annually. This operation began in 2004 and is run by Statoil and British Petroleum (BP). Salah region has approximately 20 m of sandstone in the Krechba gas field with four gas production and three injection wells. Dynamic models, geomechanical, geological, micro-seismic data, and time-lapse seismic have been utilised. In 2011, injection was stopped, and CO2 was safely migrated to the overburdened rock from the reservoir; up to about 4 million tons of CO2 had been sequestrated (Riddiford et al., 2003; Vasco et al., 2018). Statiol ASA initiated Project Snøhvit in Norway in 2007. In this project, CO2 obtained from an LNG unit was injected into a 2,600 m deep reservoir to extract crude oil without the use of offshore oil extracting equipment (Onyia and Osuma, 2010).
The Weyburn project, situated in the Williston Basin of Saskatchewan in Canada, commenced CO2 injection in the year 2000. In this project, approximately 3,000–5,000 tons of CO2 are injected into the Utsira injection well per day by the Dakota Gasification Company, with operation expected to continue up to the year 2030, by which 20 mega tonnes of CO2 will have been stored. The main composition of the reservoir is carbonate stone, which is made of limestone and dolomite. In Alberta Canada, a company known as Enhance Energy is in charge of a CO2 injection project where 14.6 mega tonnes of CO2 are stored per year and a total capacity of 2 Giga tons. In Estevan, the SaskPower Boundary Dam plant has been projected to have the capacity to sequestrate 1 million tons of CO2 per annum. In this project, 90% of CO2 is pumped into a sandstone deposit in the Weyburn field (Whittaker and Rostron, 2003; Jensen et al., 2009). In the project, Frio brine, CO2 gas was injected into the Frio sandstone reservoir in two phases. By the completion of the second phase in 2006, 3200t of CO2 had been sequestrated (Gomez 2006). In Ketzin, Germany, a project of CO2 injection into the aquifer beneath sandstone using conduit was carried out from 2008 to 2009 (Kalam et al., 2021). In the United States, Project Port Arthur was initiated in Texas in the year 2013, with approximately 222,000 tons of CO2 being injected in the first 5 months, leading to an increase in oil recovery from 1.6 to 3.1 million barrels (Folger, 2014). In Mississippi, United States 3,54 million tons of CO2 were injected into 3,000 m deep sandstone formation in project Cranfield by the year 2012. This was an EOR and CO2 sequestration project. The geochemical reactions in the reservoir occurred at a very slow rate. The potential for mineral trapping was restricted because the reservoir rocks contained only small amounts of reactive minerals needed to convert CO2 into carbonate minerals. Lu et al. (2013), Young (2012).
3.5.3 Sequestration in unmineable coal seams
The projects on using unmineable coal seams for sequestration have been gaining attention over the years. In the Japan Yubari project, field tests were carried out on the Island of Hokkaido in a 5–6 m thick coal seam at a depth of 900 m. The tests were carried out between the years 2004 and 2007. Water injection and N2 flooding tests were carried out to improve the injection of CO2 into the seams. The swelling of the coal matrix was observed to create a high-stress zone close to the injection well (Fujioka et al., 2010). A pilot demonstration of CO2 storage in unmineable coal beds was carried out in the San Juan Fairway Basin of northern New Mexico, United States. Injection wells were drilled into the cretaceous Fruitland coalbed for CH4 production using various monitoring, verification, and accounting methods (MVA). The project site consists of 31 coalbeds for the CH4 production located in nine different section areas. The lowest Canyon well was injected to a depth of 3,000 feet. Comprehensive geological characterization and reservoir modeling have been conducted to replicate and understand the reservoir’s behaviour. The injections in the coal seams were unsuccessful, but the venture laid the foundation for further research and demonstration in this field (Koperna et al., 2012; Godec et al., 2014). The Alberta Research Council Inc (ARC) of Edmonton, Alberta, Canada, led the Canada Consortium to work with the China United Coalbed Methane Corp (CUCBM) to develop China’s coalbed CO2/CH4 technologies. Three micro-pilot tests at three different CH4 reservoirs coalbed were carried out in order to reduce the investment risk. Phase 1 was a single micro-pilot design to quantify reservoir properties based on the existing knowledge of gas storage capacity, thickness of the coal seams, and static permeability. Phase 2 was to move from a single well to a five-spot pilot with five wells. While in phase 3, the 5-spot pilot was expanded into a nine-pattern commercial demonstrated. A commercial 5-spot 160-acre pattern was employed for the conceptual full-scale operation assessment. The assessment results were favourable, forecasting a return on investment within 9 years and a real rate of return of 12% (Wong et al., 2007; Wong et al., 2010; Connell et al., 2014). A pilot study was carried out on the storage of CO2 in coal seams in the Upper Silesian Coal Basin in Poland, which was known as the RECOPOL project financed by the European Commission. Between 2004 and 2005, about 760 tons of CO2 had been injected into the reservoir. An injection well was 150 m deep drilled on the coalbed. About 10% of the injected CO2 was subsequently released back into the atmosphere. The injection was abandoned in the year 2007. The assessment was done to ascertain whether the injected CO2 was adsorbed onto the coal or it was present as free gas in pore space. From the measurement carried with water pressurization and decrease of well pressure, it was concluded that a large amount of CO2 was absorbed by coal (Pagnier et al., 2005; van Bergen et al., 2009; Stańczyk et al., 2023). The Powder River Basin, Wyoming was also accessed to expand the CO2 sequestration in the coalbed for methane release. It was noted that the Paleocene Fort Union formation, with a depth of (91–610 m) has ideal coal for this purpose. Lignite-sub bituminous coal has a very large volume of biogenic methane with a thickness of 15–45 m (Montgomery, 1999).
The findings from these projects highlight the dual benefits of combining CO2 sequestration with enhanced hydrocarbon recovery, offering a pragmatic solution to reducing atmospheric CO2 levels while also extending the productive life of oil and gas fields. However, the variability in outcomes across different geological settings highlights the importance of site-specific assessments and continued innovation in sequestration technology. While challenges remain, particularly in optimizing storage capacity and ensuring long-term stability, these projects have demonstrated significant progress. As technology advances, the integration of sequestration into fossil fuel recovery processes will likely play a critical role.
4 CO2 leakage and the risks associated with the technology
Although CO2 capture and storage present a viable process to mitigate the effects of global warming, its success largely relies on storage security. As with any technology that inherently carries risk, CCS comes with risks and challenges. One of the major risks associated with CCS is the possibility of a leakage releasing CO2 back into the atmosphere, thus negating the initial benefits of the process. Leakage can occur during geological storage due to a failure of reservoir integrity or during transportation due to pipeline and storage tank failure. Any leakage, whether gradual or catastrophic, of anthropogenic CO2 can have a global or local impact and may present risks to public health and cause adverse environmental effects (Vinca et al., 2018). A leakage above 0.01% per year of CO2 is deemed ineffective in reducing anthropogenic CO2 emissions (Miocic et al., 2019).
The leakage of CO2 that results in its release into the atmosphere has a global impact; elevated concentrations of CO2 can cause headaches, dizziness, loss of consciousness, and even fatalities by asphyxiation of humans and animals. Leakage into the underground environment has a local impact that causes pollution in the surrounding area of the storage reservoir, changing the quality of aquatic systems, soil, and vegetation. Various studies have reported that an elevated concentration of CO2 causes a change in pH, redox potential, chemical composition and mineral properties of soil and water, a change in enzyme and microbial profiles, and influences the availability of nutrients for plants (Lions et al., 2014; Zhao et al., 2017).
CO2 storage activities can trigger seismic activity that can cause earthquakes of small to moderate magnitude (Zoback and Gorelick, 2012; Verdon et al., 2013). The injection of CO2 at large volumes into the reservoir may alter the state of subsurface equilibrium and trigger seismicity. Such reactions can destroy the caprock, damage infrastructure, and even cause casualties in the surrounding areas (Cheng et al., 2023). Only a few CO2 injection-induced seismic activities have been reported. The injection of a supercritical CO2-rich mixture induced 19 detectable events with a maximum moment magnitude MW-0.5 at the CO2 CRC Otway Project (Victoria, Australia) (Glubokovskikh et al., 2022). The 18 earthquakes of magnitude exceeding MW3.0 at Cogdell oil field, Texas (United States), that occurred between 2006 and 2012 were attributed to the injection of supercritical CO2 (Gan and Frohlich, 2013).
4.1 Leakage through active wells/during injection
Upon injection, much of the CO2 dissolves into the brine, which becomes denser and sinks to the bottom, while some remain buoyant (Alexander and Boodlal, 2014; Duguid et al., 2021). The difference between CO2 and the brine density causes CO2 to migrate to the surface of the storage structure. Injection of CO2 into the reservoir requires a wellbore drilled through the seal formation, an impermeable layer, and the upward migration might lead to undesired leakage of CO2 if the integrity of the injection wells is compromised. The injection well must have functional barriers and components (i.e., tubing, annulus, casing, cement, pecker material) that provide isolation between each geological interval, between the well annuli, and between the well at the surface and the external environment. Leakage is particularly high during the first injection of the CCS reservoir field due to the lack of understanding of the geomechanical reactions to CO2 injection in a geological site (Gholami et al., 2021). Failure of the injection well can occur at the tubing, casing, or packer. To ensure the integrity of the injection well, its operational design should consider parameters such as pressure, thermal stresses, corrosion-resistant materials, and injection rates. It should be properly maintained throughout its life cycle (40+ years) to prevent cracking, corrosion, and degradation of materials and continual integrity for a planned abandonment for over 10,000 years (Smith et al., 2011).
4.2 Leakage through abandoned wells
The geological formation for CO2 storage may have pre-existing or abandoned wells, which can likely be a conduit for CO2 and brine leakage (Torsæter et al., 2017). It is estimated that 29 million abandoned wells globally were used for fossil fuel extraction industries. Abandoned wells require an effective sealing system for CO2 storage as it traps CO2 within the reservoir (Pawar et al., 2009). Leakage through abandoned wells is identified as one of the critical risks in geological CO2 sequestration. This leakage pathway is more likely in a depleted reservoir, which has been used previously for commercial oil and gas exploration production. Although the current sealing technology for closure and abandoned is sufficient to contain CO2, well integrity undergoes continuous degradation over time due to geomechanical and geochemical effects, making it a major challenge to maintain over a long period (Ide et al., 2006; Vrålstad et al., 2019; Raimi et al., 2021). The older wells in the Gulf of Mexico were found to have a 50% probability of sustained casing pressure, indicating failure in at least one of the barrier elements. Studies have shown that more than 50% of wells that are 15 years old or older have at least one casing string with sustained casing pressure (Shadravan and Amani, 2015).
4.3 Leakage through faults and fractures
CO2 storage can induce geo-mechanical reactions, which can compromise the storage integrity of the reservoir (Miocic et al., 2019). The geo-mechanical reaction can be a result of either pressure build-up within the reservoir or thermal cycles during the injection process. The increase in pressure within the storage reservoir when injecting CO2 due to a combination of viscous forces opposing fluid displacement associated with the multiphase flow of CO2 and compression of the filling fluids (de Coninck and Benson, 2014; Vilarrasa et al., 2019). The magnitude of pressure build-up is determined by the thickness and permeability. Injecting CO2 at a lower temperature than the temperature of the reservoir rock lowers the total stress in the injection layer (i.e., caprock layer) and reservoir, causing negative volumetric strains that can propagate to the surface (Gor and Prévost, 2013; Khurshid and Fujii, 2021; Vilarrasa et al., 2014; Sokama-Neuyam et al., 2022). The thermal effects make the stress concentration around the wellbore more tensile in all directions (Perdomo et al., 2013; Goodarzi et al., 2013). The change in the effective stress field in the rock reservoir can reactivate or create fracture networks in the sealing caprocks. Fractures running through a caprock that would otherwise be impermeable can thus provide a pathway for CO2 leakage and are generally rapid (Nicol et al., 2011; Verdon et al., 2013). It is, therefore, crucial to perform a geo-mechanical analysis of the storage rock to determine the fracture pressure of the cap rock to avoid over-pressurizing the storage rock and minimize the risk of CO2 leakage.
Risk assessment studies have reported that leakage through fractures and faults is more problematic than through active and abandoned wells, as fractures and faults impact cannot be generalized and predicted (Goodarzi et al., 2013; Li and Laloui, 2017). Moreover, only a few technical methods are available to resolve their impact (Turrell et al., 2022). An assessment report of the CO2 storage in the North Sea reported a low-level leakage of active and abandoned wells (leakage probability of ≤0.005 per year) with a leakage rate of up to 10 tonnes per day whilst leakage rate from the faults and fractures ranged from 50 to 1,500 tonnes per day (Jewell and Senior, 2012).
5 Monitoring of CO2 leakage
5.1 Storage reservoir monitoring
The security of CO2 sequestration projects is a major concern; thus, monitoring CO2 in storage is a critical component and a regulatory requirement for all CSS projects. Monitoring systems are designed to detect and quantify any CO2 leakage within a defined area of surveillance to ensure the performance, reliability, and safety of the process. Monitoring allows the leaks to be detected long before damage ensues; thus, they can be observed to predict their long-term consequences and to plan the most efficient intervention without the need for unnecessary immediate operational shutdowns. Although the monitoring programs of different CO2 storage projects share the same objectives, their design and selection are largely influenced by the risk assessments, the regulatory requirements, and the needs of the geological sites (Dean et al., 2020).
The monitoring program requires an assessment of the natural conditions of the geological site before implementation of the CCS projects to establish baseline monitoring. The baseline assessment includes hydrogeological, geochemical, and geophysical analysis of the site and the nearby injection and operations (Barth et al., 2015). Thoroughly assessing hydrological characteristics such as groundwater levels, flow direction and velocity as well as water-bearing formation, its thickness, and hydraulic conductivity ensures that the CCS project does not negatively impact water resources around the storage site (Edet and Okereke, 1997; Brydie et al., 2014). The geochemical characteristics such as pH, alkalinity, dissolved inorganic carbon, total dissolved solids, and trace elements are essential in understanding the interaction of the injected CO₂ with the host rock, and the surrounding fluids (Jeong et al., 2017). The geomechanical parameters that determine in-situ stress, fracture density and orientation, caprock integrity and permeability of the rock formation, induced seismicity potential and the thermal properties are essential in predicting the behaviour and the response of the storage land and the subsurface to CO2 injection (Khalilidermani and Knez, 2024). Any changes following CO2 injection in the operational phase through to site closure that deviates from the established baseline conditions and the physical movement of injected CO2, changes in gas and fluid compositions, and reactions of CO2 with formation waters and reservoir rocks can be actively monitored to confirm the effectiveness of site storage. Monitoring tools of various approaches (i.e., geophysical, geochemical, and satellite) are deployed at the subsurface to monitor the migration of CO2 and the stability of the caprock, near the surface to monitor soil, groundwater, and the atmosphere to monitor levels of atmospheric CO2 around the site in the event of suspected leakage (Barth et al., 2015; Fawad and Mondol, 2021). Tools available for the oil and gas industry, which monitor pressure, temperature, and passive and active seismic, are readily applied in CO2 monitoring, while tools that monitor geochemical and geophysical activity may require modification for CO2 monitoring (Vermeul et al., 2016).
Geological carbon storage should have a comprehensive monitoring plan that integrates complementary monitoring techniques to increase assurance to regulatory bodies and the public, as each method has limitations and disadvantages. Various geophysical monitoring methods listed in Table 4 can detect subsurface leakage of CO2 to provide information for risk assessment and mitigate potential risks (Fawad and Mondol, 2021). Whilst geophysical properties can be monitored remotely using wireline logs, geochemical properties require sampling of gas and fluid from the storage site either with down-hole tools (e.g., u-tubes), at the wellhead, or via the use of down-hole sensors for in situ measurements where possible. As with geophysical monitoring, geochemical monitoring requires establishing a baseline by determining natural conditions, such as alkalinity, pH, dissolved constituents in formation waters concentrations of major and minor cations, anions and trace elements, and stable isotope ratios of water and selected prior to CO2 injection. Continual monitoring during the injection phase would reveal changes in gas and fluid compositions due to the physical movement of injected CO2 and reactions of CO2 with formation waters and reservoir rocks.
Leakage of CO2 from the reservoir can reduce pH due to CO2 dissolving in the formation water, forming carbonic acid, and could lead to increased levels of trace metals. Monitoring CO2 leakage by a change in pH represents a challenge as this can occur naturally with organic matter or biological processes occurring in the environment (Esposito and Benson, 2011; Humez et al., 2013; Waarum et al., 2017). Consequently, geochemical analysis employs chemical tracers that are injected with the CO2 into the reservoir. Chemical tracers are well established in the hydrocarbon and geothermal industry for optimum production. They are now proposed as an effective means of detecting and quantifying CO2 leaks to the surface from geological CO2 storage sites. However, there is limited experience with using chemical tracers to detect CO2 leakage (Roberts et al., 2017). Chemical tracers are selected according to their public and environmental safety, suitability for monitoring and analysis, behaviour, and stability in the environment (interaction with environmental conditions), and they should allow differentiation from natural or background sources. Chemical tracers such as stable isotopes of carbon (13C) and oxygen (18O), sulfur hexafluoride (SF6), perfluorocarbons (PFCs), and Noble gases (He, Ne, Ar, Kr, and Xe) have been successfully applied in CO2 monitoring in storage (Myers et al., 2013; Flude et al., 2016; Ju et al., 2019; Kim et al., 2020; Flohr et al., 2021).
5.2 Wellbore monitoring
Given the risk profile of the wellbore, it is crucial to monitor CO2 leakage through active or abandoned wells. Changes in temperature and pressure are common methods of monitoring the migration of CO2 from the reservoir. Traditionally, downhole sensors are permanently installed within the wells to monitor temperature and pressure. CO2 that migrates upwards changes to a gaseous phase with a large volumetric expansion, causing pressure increase (Buscheck et al., 2019). CO2 leakage can cause a significant change in temperature due to the Joule–Thomson effect that may be easily detectable (Zeidouni et al., 2017; Mao et al., 2017; Islam and Sun, 2017).
5.3 Application of computational approaches in monitoring
The advancement of computational technologies in CCS has significantly enhanced the management and storage of CO2 in geological landforms. The application of Artificial Intelligence and Machine Learning allows for numerical simulations and data analysis which enables more efficient monitoring processes, and accurate prediction of CO2 behaviour and risks as well as determining the feasibility of CO2 storage. Studies have described various ML algorithms that can be applied to CCS monitoring to ensure the safety and long-term stability effectiveness of CO2 storage, with Neural Networks being the widely used machine learning (Sinha et al., 2020; Anyosa et al., 2021; Yao et al., 2023; Ma et al., 2024). Artificial Neural Network (ANN) is more applicable in geochemical monitoring and can be used to predict CO2 plume migration and changes in pressure, temperature and saturation within geological formation which is essential for optimizing injection strategies. Moreover, they can analyse time series data from geochemical sensors and predict any deviation that may occur from expected behaviour that can indicate CO2 leakage (Kim et al., 2017; Thanh et al., 2019). Li B. et al. (2018) demonstrated the predictive capabilities of the constructed ANN model in the integrity of injection well throughout its lifespan. The study analyzed the well attribute data (i.e., well type, well age, CO2 exposed period, well construction details and materials), well operation histories and regulatory changes of 510 CO2 wells at two CO2 EOR fields, West Hastings oil field and Oyster Bayou oil field, United States. The study by Tillero (2024) demonstrated that the ANN model predicts variation with time of trapping indices and CO2 cumulative injected volume for CO2 storage in deep saline aquifers in the Bunter sandstone formation in the Southern North Sea area in the United Kingdom. The study by Song and Wang (2021) demonstrated the capabilities of the ANN model to predict the optimum well design accurately and to evaluate storage efficiency to maximize CO2 sequestration in Pohang Basin, South Korea using datasets of 10- and 30-year injection scenarios. Convolutional Neural Networks (CNN), which is based on the Deep Learning method, is more effective in geomechanical monitoring and can be used in the interpretation of seismic data to detect subsurface CO₂ plumes and deformation, fractures and faults as well as changes in surrounding vegetation due to CO2 migration (Chen et al., 2019; Zhong et al., 2019; Zhou et al., 2019). Zhou et al. (2018) and Zhou et al. (2019) demonstrated the detection capabilities and subsurface application of CNN architecture by developing a detection model based on CNN which directly learns the mapping relation between seismic data and CO2 leakage mass at potential CO2 storage site at Kimberlina, California. The comparative study of Thanh et al. (2024) revealed that the CNN model emerged as the most precise in estimating the contact angle within the CO2-brine-mineral system which is vital for optimising CO₂ sequestration in geological formations. The effectiveness and predictive capabilities of models require a large set of data which presents a challenge for CCS due to limited historical data and the complexity of the geological landforms. Neural networks have, however, powerful learning capabilities and do not require prior knowledge from multiple input features. They nonetheless provide detailed insights into the performance of CO2 in storage helping to ensure the safety and effectiveness of CCS projects (Yan et al., 2021; Al-Hawary et al., 2023).
6 Challenges associated with carbon capture and storage
The CCS project faces many significant barriers that may impede its adoption and implementation. Some of the key challenges include.
6.1 Economic viability
As of 2023, it was estimated that the cost of the CCS technology varies from USD 15 – 130 USD per metric ton of carbon dioxide (tCO₂) for industrial processes producing highly concentrated gas streams, but much higher for direct air capture technology at a range from 100 – 345 USD per ton of CO₂ (Statista, 2023). The cost of the CCS technology is initially high due to the complex design of the technology and the infrastructure requirement, which may render the project economically infeasible and deter investments and financing for the full implementation of CCS projects on a larger scale. Although the cost declines with the maturity of the project, the technology requires a significant energy demand to operate; thus the sustainability of the project may prove economically inviable (Hardisty et al., 2011; Wang et al., 2021; Subraveti et al., 2023). The cost of CCU technologies is projected to decrease in the future as the experience in operating the technology accumulates, and the market grows, as was observed with renewable energy technology.
6.2 Regulatory framework
The legal and regulatory framework is considered a significant component of the CCS project, as they are required to adhere to governmental and international regulations to ensure environmental safety, operation reliability, and sustainable and effective long-term management from their deployment throughout the project lifetime. The regulation often entails site selection, operation protocol, closure and post-closure storage site closure, and risk evaluation. CCS is still in an early development phase; nonetheless, several countries have developed legal and regulatory frameworks, which have laid the foundation for other countries yet to establish their frameworks. The CCS projects can face a complex and time-consuming process in securing a permit; this could present a major challenge for CCS projects, which can prolong the project deployment and increase the overall expenses (Kerr et al., 2009; Romasheva and Cherepovitsyna, 2019; IEA, 2022).
6.3 Public perception
One of the key issues in the adoption of any technological project is social acceptance as this can affect residents and local stakeholders. Studies on public opinion on CCS have reported mixed levels of awareness and acceptance of the technology, which is characterised as low to moderate due to CCS being a young technology (Vercelli et al., 2013). Misconceptions and public concerns about the safety and environmental risks can lead to opposition and resistance from communities and stakeholders and, thus, may influence the necessary license to operate and the success of the project (Cohen et al., 2014; Braun, 2017; Tcvetkov et al., 2019). Engaging with the public and communicating the technology is important, discussing its pros and cons and making it understandable, facilitating public participation in decision-making processes (Vercelli et al., 2013; Vasilev et al., 2019).
Addressing these barriers requires a collaborative effort from governments, industry stakeholders, academic institutions, and society to address technological, economic, regulatory, and social challenges associated with CCS implementation. Policymakers play a crucial role in providing supportive frameworks, financial incentives, and regulatory certainty to facilitate the widespread adoption of CCS as a vital strategy to mitigate climate change.
7 Conclusion and perspective
Carbon dioxide capture and storage are promising strategies for reducing emissions and combating climate change. Over time, carbon capture technologies have evolved from traditional methods like adsorption and absorption to advanced techniques such as cryogenic, enzyme-based, and hydrate-based separation. Most current technologies are small-scale and onsite, making them difficult to deploy widely. Emerging methods, like DAC, which extracts CO2 directly from the air, are gaining attention. SMART technology enhances these processes by improving performance, enabling adaptive control, and allowing real-time monitoring, offering greater efficiency and flexibility compared to traditional methods. Storing carbon dioxide underground in landforms is generally more cost-effective than seafloor storage due to easier access and lower infrastructure costs. Machine learning algorithms and computational studies have significantly improved CCS technologies by optimizing CO2 behavior predictions, capturing processes, and analyzing large datasets for better decision-making. Effective monitoring is essential for maintaining the safety, security, and long-term containment of CO2 storage sites. Future research will need ongoing collaboration with regulatory bodies, industry partners, and research institutions to address the technical, economic, and regulatory challenges associated with the successful implementation of CCS (Carbon Capture and Storage). A major obstacle is the insufficient financial support for CCS initiatives, as they are unlikely to be profitable in the near term, which discourages investment. Consequently, without government financial aid, advancing these technologies will be challenging. Government policies, like a carbon tax, could help accelerate the adoption of CCS technologies. Additionally, utilizing carbon is considered a more practical and cost-effective method for managing CO2 emissions.
Author contributions
PN: Conceptualization, Data curation, Formal Analysis, Methodology, Resources, Validation, Writing–original draft, Writing–review and editing. RB: Conceptualization, Formal Analysis, Validation, Writing–original draft, Writing–review and editing. LM: Methodology, Writing–review and editing.
Funding
The author(s) declare that financial support was received for the research, authorship, and/or publication of this article. Publication fees provided by Durban University Technology and University of South Africa.
Conflict of interest
Author PN was employed by Mintek. Author RB was employed by Rand Water.
The remaining author declares that the research was conducted in the absence of any commercial or financial relationships that could be construed as a potential conflict of interest.
Publisher’s note
All claims expressed in this article are solely those of the authors and do not necessarily represent those of their affiliated organizations, or those of the publisher, the editors and the reviewers. Any product that may be evaluated in this article, or claim that may be made by its manufacturer, is not guaranteed or endorsed by the publisher.
References
Abdullatif, Y., Sodiq, A., Mir, N., Bicer, Y., Al-Ansari, T., El-Naas, M. H., et al. (2023). Emerging trends in direct air capture of CO2: a review of technology options targeting net-zero emissions. R. Soc. Chem. Adv. 13 (9), 5687–5722. doi:10.1039/d2ra07940b
Adu, E., Zhang, Y., and Liu, D. (2019). Current Situation of Carbon dioxide capture, storage, and enhanced oil recovery in the oil and gas industry. Can. J. Chem. Eng. 97, 1048–1076. doi:10.1002/cjce.23393
Agartan, E., Trevisan, L., Cihan, A., Birkholzer, J., Zhou, Q., and Illangasekare, T. H. (2015). Experimental study on effects of geologic heterogeneity in enhancing dissolution trapping of supercritical CO2. Water Resour. Res. 51 (3), 1635–1648. doi:10.1002/2014wr015778
Ahmadi, M., Janakiram, S., Dai, Z., Ansaloni, L., and Deng, L. (2018). Performance of mixed matrix membranes containing porous two-dimensional (2D) and three-dimensional (3D) fillers for CO2 separation: a review. Membranes 8 (3), 50. doi:10.3390/membranes8030050
Ajayi, T., Gomes, J. S., and Bera, A. (2019). A review of CO2 storage in geological formations emphasizing modeling, monitoring and capacity estimation approaches. Petroleum Sci. 16, 1028–1063. doi:10.1007/s12182-019-0340-8
Alexander, D., and Boodlal, D. (2014). Evaluating the effects of CO2 injection in faulted saline aquifers. Energy Procedia 63, 3012–3021. doi:10.1016/j.egypro.2014.11.324
Al Hameli, F., Belhaj, H., and Al Dhuhoori, M. (2002). CO2 sequestration overview in geological formations: Trapping mechanisms matrix assessment. Energies 15 (20), 7805. doi:10.3390/en15207805
Al-Hawary, S. I. S., Ali, E., Kamona, S. M. H., Saleh, L. H., Abdulwahid, A. S., Al-Saidi, D. N., et al. (2023). Prediction of geomechanical bearing capacity using autoregressive deep neural network in carbon capture and storage systems. Heliyon 9 (11), e21913. doi:10.1016/j.heliyon.2023.e21913
Ali, M., Jha, N. K., Pal, N., Keshavarz, A., Hoteit, H., and Sarmadivaleh, M. (2022). Recent advances in carbon dioxide geological storage, experimental procedures, influencing parameters, and future outlook. Earth-Science Rev. 225, 103895. doi:10.1016/j.earscirev.2021.103895
Al-Khafaji, H. F., Meng, Q., Hussain, W., Mohammed, R. K., Harash, F., and AlFakey, S. A. (2023). Predicting minimum miscible pressure in pure CO2 flooding using machine learning: method comparison and sensitivity analysis. Fuel 354, 129263. doi:10.1016/j.fuel.2023.129263
Al-Mamoori, A., Rownaghi, A. A., and Rezaei, F. (2018). Combined capture and utilization of CO2 for syngas production over dual-function materials. ACS Sustain. Chem. Eng. 6 (10), 13551–13561. doi:10.1021/acssuschemeng.8b03769
Aminu, M. D., Nabavi, S. A., Rochelle, C. A., and Manovic, V. (2017). A review of developments in carbon dioxide storage. Appl. Energy 208, 1389–1419. doi:10.1016/j.apenergy.2017.09.015
Ammendola, P., Raganati, F., and Chirone, R. (2017). CO2 adsorption on a fine activated carbon in a sound assisted fluidized bed: thermodynamics and kinetics. Chem. Eng. J. 322, 302–313. doi:10.1016/j.cej.2017.04.037
Anyosa, S., Bunting, S., Eidsvik, J., Romdhane, A., and Bergmo, P. (2021). Assessing the value of seismic monitoring of CO2 storage using simulations and statistical analysis. Int. J. Greenh. Gas Control 105, 103219. doi:10.1016/j.ijggc.2020.103219
Appriou, D., Bonneville, A., Zhou, Q., and Gasperikova, E. (2020). Time-lapse gravity monitoring of CO2 migration based on numerical modeling of a faulted storage complex. Int. J. Greenh. Gas Control 95, 102956. doi:10.1016/j.ijggc.2020.102956
Arlota, C., and Costa, H. K. M. (2021). Climate change, carbon capture and storage (CCS), energy transition, and justice: where we are now, and where (should be) we headed? Carbon Capture Storage Int. Energy Policy Law, 385–393.
Ashraf, W. M., and Dua, V. (2023). Machine learning based modelling and optimization of post-combustion carbon capture process using MEA supporting carbon neutrality. Digit. Chem. Eng. 8, 100115. doi:10.1016/j.dche.2023.100115
Awag, M., Mackay, E., and Ghanbari, S. (2024). Impact of CO2 solubility on design of single well tracer tests to evaluate residual saturation during carbon capture and storage. Adv. Geo-Energy Res. 11 (1), 6–19. doi:10.46690/ager.2024.01.02
Ayalew, M. E. (2021). The role of nanotechnology for energy storage, conservation and post combustion CO2 capture in industry: a review. Int. J. Mater. Sci. Appl. 10 (3), 55. doi:10.11648/j.ijmsa.20211003.12
Babar, M., Bustam, M. A., Ali, A., Maulud, A. S., Shafiq, U., Mukhtar, A., et al. (2019). Thermodynamic data for cryogenic carbon dioxide capture from natural gas: a review. Cryogenics 102, 85–104. doi:10.1016/j.cryogenics.2019.07.004
Babu, A., Viswanadhan, S. K., and Veluswamy, H. P. (2024). Investigation of semi-clathrate formation kinetics for post-combustion CO2 capture in the presence of amino acids. Gas Sci. Eng. 125, 205292. doi:10.1016/j.jgsce.2024.205292
Bachu, S. (2007). Carbon dioxide storage capacity in uneconomic coal beds in Alberta, Canada: Methodology, potential and site identification. Int. J. Greenh. Gas Control 1 (3), 374–385. doi:10.1016/s1750-5836(07)00070-9
Bachu, S., and Adams, J. J. (2003). Sequestration of CO2 in geological media in response to climate change: capacity of deep saline aquifers to sequester CO2 in solution. Energy Convers. Manag. 44 (20), 3151–3175. doi:10.1016/S0196-8904(03)00101-8
Baek, S. H., Kanzaki, Y., Lora, J. M., Planavsky, N., Reinhard, C. T., and Zhang, S. (2023). Impact of Climate on the Global capacity for enhanced rock weathering on croplands. Earth’s Future 11, e2023EF003698. doi:10.1029/2023ef003698
Barnard, M. (2019). Best carbon capture facility in world emits 25 times more CO2 than sequestered. Available at: https://cleantechnica.com/2019/06/12/best-carbon-capture-facility-in-world-emits-25-times-more-co2-than-sequestered/.CleanTechnica (Accessed June 12, 2019).
Barooah, M., Kundu, S., Kumar, S., Katare, A., Borgohain, R., Uppaluri, R. V., et al. (2024). New generation mixed matrix membrane for CO2 separation: transition from binary to quaternary mixed matrix membrane. Chemosphere 141653. doi:10.1016/j.chemosphere.2024.141653
Barrufet, M. A., Bacquet, A., and Falcone, G. (2010). Analysis of the storage capacity for CO2 sequestration of a depleted gas condensate reservoir and a saline aquifer. J. Can. Pet. Technol. 49, 23–31. doi:10.2118/139771-pa
Barth, J. A., Mader, M., Myrttinen, A., Becker, V., van Geldern, R., and Mayer, B. (2015). “Advances in stable isotope monitoring of CO2 under elevated pressures, temperatures and salinities: selected results from the project CO2 ISO-LABEL,” in Geological storage of CO2–long term security aspects, 59–71. GEOTECHNOLOGIEN Science Report No. 22.
Baskaran, D., Saravanan, P., Nagarajan, L., and Byun, H. S. (2024). An overview of technologies for Capturing, Storing, and utilizing carbon Dioxide: technology Readiness, large-scale Demonstration, and cost. Chem. Eng. J. 151998. doi:10.1016/j.cej.2024.151998
Beerling, D. J., Kantzas, E. P., Lomas, M. R., Wade, P., Eufrasio, R. M., Renforth, P., et al. (2020). Potential for large-scale CO2 removal via enhanced rock weathering with croplands. Nature 583, 242–248. doi:10.1038/s41586-020-2448-9
Bemani, A., Baghban, A., and Mohammadi, A. H. (2020). An insight into the modeling of sulfur content of sour gases in supercritical region. J. Petroleum Sci. Eng. 184, 106459. doi:10.1016/j.petrol.2019.106459
Bertz, M., Davidson, O., De Coninck, H., Loss, M., and Meyer, L. (2005). IPCC special report on carbon dioxide capture and storage. Cambridge University Press. United States of America by Cambridge University Press, New York: Intergovernmental Panel on Climate Change.
Bierbaumer, S., Nattermann, M., Schulz, L., Zschoche, R., Erb, T. J., and Winkler, C. K. (2023). Enzymatic conversion of CO2: from natural to artificial utilization. Chemical Reviews 123 (9), 5702–5754. doi:10.1021/acs.chemrev.2c00581
Birkholzer, J. T., Zhou, Q., and Tsang, C. F. (2009). Large-scale impact of CO2 storage in deep saline aquifers: a sensitivity study on pressure response in stratified systems. Int. J. Greenh. Gas Control 3, 181–194. doi:10.1016/j.ijggc.2008.08.002
Black, J. R., Carroll, S. A., and Haese, R. R. (2015). Rates of mineral dissolution under CO2 storage conditions. Chem. Geol. 399, 134–144. doi:10.1016/j.chemgeo.2014.09.020
Blomen, E., Hendriks, C., and Neele, F. (2009). Capture technologies: improvements and promising developments. Energy Procedia 1, 1505–1512. doi:10.1016/j.egypro.2009.01.197
Bloomberg (2019). Company overview of CO2 technology centre mongstad DA. Available at: https://www.bloomberg.com/research/stocks/private/snapshot.asp?privcapid=104816137 (Accessed January 9, 2019).
Bobicki, E. R., Liu, Q., Xu, Z., and Zeng, H. (2012). Carbon capture and storage using alkaline industrial wastes. Prog. Energy Combust. Science| 38 (2), 302–320. doi:10.1016/j.pecs.2011.11.002
Böser, W., and Belfroid, S. (2013). Flow assurance study. Energy Procedia 37, 3018–3030. doi:10.1016/j.egypro.2013.06.188
Braun, C. (2017). Not in my backyard: CCS sites and public perception of CCS. Risk Anal. 37 (12), 2264–2275. doi:10.1111/risa.12793
Brydie, J., Jones, D., Jones, J. P., Perkins, E., Rock, L., and Taylor, E. (2014). Assessment of baseline groundwater physical and geochemical properties for the Quest carbon capture and storage project, Alberta, Canada. Energy Procedia 63, 4010–4018. doi:10.1016/j.egypro.2014.11.431
Buckingham, J., Reina, T. R., and Duyar, M. S. (2022). Recent advances in carbon dioxide capture for process intensification. Carbon Capture Sci. and Technol. 2, 100031. doi:10.1016/j.ccst.2022.100031
Budinis, S., Krevor, S., Dowell, N. M., Brandon, N., and Hawkes, A. (2018). An assessment of CCS costs, barriers and potential. Energy Strategy Rev. 22, 61–81. doi:10.1016/j.esr.2018.08.003
Bui, M., Adjiman, C. S., Bardow, A., Anthony, E. J., Boston, A., Brown, S., et al. (2018b). Carbon capture and storage (CCS): the way forward. Energy and Environ. Sci. 11 (5), 1062–1176. doi:10.1039/c7ee02342a
Bui, M., Fajardy, M., and Mac Dowell, N. (2018a). Bio-energy with carbon capture and storage (BECCS): opportunities for performance improvement. Fuel 213, 164–175. doi:10.1016/j.fuel.2017.10.100
Buscheck, T. A., Mansoor, K., Yang, X., Wainwright, H. M., and Carroll, S. A. (2019). Downhole pressure and chemical monitoring for CO2 and brine leak detection in aquifers above a CO2 storage reservoir. Int. J. Greenh. Gas Control 91, 102812. doi:10.1016/j.ijggc.2019.102812
Cao, C., Liu, H., Hou, Z., Mehmood, F., Liao, J., and Feng, W. (2020). A review of CO2 storage in view of safety and cost-effectiveness. Energies 13 (3), 600. doi:10.3390/en13030600
Castellani, B. (2023). Potential pathway for reliable long-term CO2 storage as clathrate hydrates in marine environments. Energies 16 (6), 2856. doi:10.3390/en16062856
Castellani, B., Morini, E., Filipponi, M., Nicolini, A., Cotana, F., and Rossi, F. (2014). Engineering, and process aspects of hydrate-based technology for energy applications. 14th CIRIAF national congress: energy, environment and sustainable development, Perugia, Italy.
Castillo-Reyes, O., Hu, X., Wang, B., Wang, Y., and Guo, Z. (2023). Electromagnetic imaging and deep learning for transition to renewable energies: a technology review. Front. Earth Sci. 11, 1159910. doi:10.3389/feart.2023.1159910
Cavalcante, T. D. M., Souza, A. C., Hajibeygi, H., Carvalho, D. K., and Lyra, P. R. (2024). Simulation of two-phase flow in 3D fractured reservoirs using a projection-based Embedded Discrete Fracture Model on Unstructured tetrahedral grids (pEDFM-U). Adv. Water Resour. 187, 104679. doi:10.1016/j.advwatres.2024.104679
Celia, M. A., Bachu, S., Nordbotten, J. M., and Bandilla, K. W. (2015). Status of CO2 storage in deep saline aquifers with emphasis on modelling approaches and practical simulations. Water Resour. Res. 51 (9), 6846–6892. doi:10.1002/2015wr017609
Chadwick, R. A. (2013). “Offshore CO2 storage: Sleipner natural gas field beneath the North Sea,” in Geological storage of carbon dioxide (CO2) (Woodhead Publishing), 227–253e. doi:10.1533/9780857097279.3.227
Chen, M. R., and Shen, K. W. (2024). Risk prioritization by Z-VIKOR method under incomplete reliable information and its application in CCUS project site selection. Appl. Soft Comput. 154, 111357. doi:10.1016/j.asoc.2024.111357
Chen, Q., Zhang, F., Qiu, F., Wang, Z., Liu, Y., Zhang, Q., et al. (2021). Quantitatively determining gas content using pulsed neutron logging technique in closed gas reservoir. J. Petroleum Sci. Eng. 198, 108149. doi:10.1016/j.petrol.2020.108149
Chen, Y., Zhang, G., Bai, M., Zu, S., Guan, Z., and Zhang, M. (2019). Automatic waveform classification and arrival picking based on convolutional neural network. Earth Space Sci. 6 (7), 1244–1261. doi:10.1029/2018ea000466
Cheng, Y., Liu, W., Xu, T., Zhang, Y., Zhang, X., Xing, Y., et al. (2023). Seismicity induced by geological CO2 storage: a review. Earth Sci. Rev. 239, 104369. doi:10.1016/j.earscirev.2023.104369
Cheung, O. (2021). Nanotechnology and metal-organic frameworks for carbon capture. Research Outreach, (126).
Chevron Australia (2019). Gorgon project – an Australian icon. Available at: https://australia.chevron.com/our-businesses/gorgon-project (Accessed January 9, 2019).
Cohen, J. J., Reichl, J., and Schmidthaler, M. (2014). Re-focussing research efforts on the public acceptance of energy infrastructure: a critical review. Energy 76, 4–9. doi:10.1016/j.energy.2013.12.056
Cole, D. R., Chialvo, A. A., Rother, G., Vlcek, L., and Cummings, P. T. (2010). Supercritical fluid behaviour at nanoscale interfaces: implications for CO2 sequestration in geologic formations. Philos. Mag. 90 (17-18), 2339–2363. doi:10.1080/14786430903559458
Connell, L. D., Pan, Z., Camilleri, M., Meng, S., Down, D., Carras, J., et al. (2014). Description of a CO2 enhanced coal bed methane field trial using a multi-lateral horizontal well. Int. J. Greenh. Gas Control 26, 204–219. doi:10.1016/j.ijggc.2014.04.022
Conner, A., Chace, D., Abou-Saleh, J., Kim, Y., McNeil, C., Gerst, J., et al. (2017). Developing best practices for evaluating fluid saturations with pulsed neutron capture logging across multiple active CO2-EOR fields. Energy Procedia 114, 3636–3648. doi:10.1016/j.egypro.2017.03.1495
Consoli, C. (2019). Bioenergy and carbon capture and storage. Global CCS Institute, 1–14. Available at: https://www.globalccsinstitute.com/wp-content/uploads/2019/03/BECCS-Perspective_FINAL_PDF.pdf.
Constable, S. (2013). Review paper: instrumentation for marine magnetotelluric and controlled source electromagnetic sounding. Geophys. Prospect. 61, 505–532. doi:10.1111/j.1365-2478.2012.01117.x
Corrente, N. J., Hinks, E. L., Kasera, A., Liu, J., and Neimark, A. V. (2024). Deformation of nanoporous carbons induced by multicomponent adsorption: insight from the SAFT-DFT model. J. Phys. Chem. C 128 (20), 8458–8466. doi:10.1021/acs.jpcc.4c00833
Cousins, A., Huang, S., Cottrell, A., Feron, P. H. M., Chen, E., and Rochelle, G. T. (2015). Pilot-scale parametric evaluation of concentrated piperazine for CO2 capture at an Australian coal-fired power station. Greenh. Gases Sci. Technol. 5, 7–16. doi:10.1002/ghg.1462
Cowan, R. M., Ge, J. J., Qin, Y. J., McGregor, M. L., and Trachtenberg, M. C. (2003). CO2 capture by means of an enzyme-based reactor. Annals of the New York Academy of Sciences 984 (1), 453–469. doi:10.1111/j.1749-6632.2003.tb06019.x
Cowdhury, F. A., Yamada, H., Higashii, T., Goto, K., and Onoda, M. (2013). CO2 capture by tertiary amine absorbents A performance comparison study. Industrial and Eng. Chem. Res. 52 (24), 8323–8331. doi:10.1021/ie400825u
Cui, G., Zhang, L., Ren, B., Enechukwu, C., Liu, Y., and Ren, S. (2016). Geothermal exploitation from depleted high temperature gas reservoirs via recycling supercritical CO2: heat mining rate and salt precipitation effects. Appl. Energy 183, 837–852. doi:10.1016/j.apenergy.2016.09.029
Cui, G., Zhang, L., Tan, C., Ren, S., Zhuang, Y., and Enechukwu, C. (2017). Injection of supercritical CO2 for geothermal exploitation from sandstone and carbonate reservoirs: CO2–water–rock interactions and their effects. J. CO2 Util. 20, 113–128. doi:10.1016/j.jcou.2017.05.006
Cui, J., Bao, J., Ning, S., Li, B., Deng, W., Duan, X., et al. (2024). Molecular simulation of the impact of surface roughness on carbon dioxide adsorption in organic-rich shales. Unconv. Resour. 4, 100071. doi:10.1016/j.uncres.2023.100071
Czakiert, T., Krzywanski, J., Zylka, A., and Nowak, W. (2022). Chemical looping combustion: a brief overview. Energies 15 (4), 1563. doi:10.3390/en15041563
d’Almeida, K. S., Viela, P. C., Cardoso, R. A., Fernandes, R. F., and Souza, M. F. F. (2018). Ocorrencia de em campos petroliferos na margem leste brasileira. Empresa Pesqui. Energ.
Daniel, A. (2019). Iceland, Turning CO2 into Rock could be a big breakthrough for Carbon dioxide capture. Pulitzer Center on Crisis Reporting. May 06, 2019. Available at: https://pulitzercenter.org/stories/iceland-turning-co2-rock-could-be-big-breakthrough-carbon-capture.
Davoodi, S., Al-Shargabi, M., Wood, D. D., Rukavishnikov, V. S., and Minaev, K. M. (2023). Review of technological progress in carbon dioxide capture, storage, and utilization. Gas Sci. Eng. 117, 205070. doi:10.1016/j.jgsce.2023.205070
Dean, M., Blackford, J., Connelly, D., and Hines, R. (2020). Insights and guidance for offshore CO2 storage monitoring based on the QICS, ETI MMV, and STEMM-CCS projects. Int. J. Greenh. Gas Control 100, 103120. doi:10.1016/j.ijggc.2020.103120
Dechnik, J., Gascon, J., Doona, C. J., Janiak, C., and Sumby, C. J. (2017). Mixed matrix membranes. Angewandte Chemie International Edition, 56 (32), 9292–9310. doi:10.1002/anie.201701109
de Coninck, H., and Benson, S. M. (2014). Carbon dioxide capture and storage: issues and prospects. Annu. Rev. Environ. Resour. 39, 243–270. doi:10.1146/annurev-environ-032112-095222
De Silva, G. P. D., Ranjith, P. G., and Perera, M. S. A. (2015). Geochemical aspects of CO2 sequestration in deep saline aquifers: a review. Fuel 155, 128–143. doi:10.1016/j.fuel.2015.03.045
Dessert, C., Dupré, B., Gaillardet, J., François, L. M., and Allègre, C. J. (2003). Basalt weathering laws and the impact of basalt weathering on the global carbon cycle. Chem. Geol. 202 (3-4), 257–273. doi:10.1016/j.chemgeo.2002.10.001
Dikhanbaev, B., Dikhanbaev, A., Koshumbayev, M., Ybray, S., Mergalimova, A., and Georgiev, A. (2024). On the issue of neutralizing carbon dioxide at processing coal in boilers of thermal power plants. Energy 295, 130978. doi:10.1016/j.energy.2024.130978
Donskoy, I. (2023). Techno-economic efficiency estimation of promising integrated oxyfuel gasification combined-cycle power plants with carbon capture. Clean. Technol. 5 (1), 215–232. doi:10.3390/cleantechnol5010013
Duguid, A., Glier, J., Heinrichs, M., Hawkins, J., Peterson, R., and Mishra, S. (2021). Practical leakage risk assessment for CO2 assisted enhanced oil recovery and geologic storage in Ohio’s depleted oil fields. Int. J. Greenh. Gas Control 109, 103338. doi:10.1016/j.ijggc.2021.103338
DW documentary (2021). Norway and CO2 emissions. Beneath the waves - Norway and CO2 emmissions – DW – 11/21/2020.
EarthSky (2024). EarthSky. Available at: https://earthsky.org/earth/fastest-carbon-dioxide-surge-ever-during-year-of-extremes/.
Edet, A. E., and Okereke, C. S. (1997). Assessment of hydrogeological conditions in basement aquifers of the Precambrian Oban massif, southeastern Nigeria. J. Appl. Geophys. 36 (4), 195–204. doi:10.1016/s0926-9851(96)00049-3
Einloft, S., and Bernard, F. L. (2020). Encapsulated liquid sorbents for CO2 capture. Franciele Longaray Bernard. Adv. Carbon Capture 125–150. doi:10.1016/B978-0-12-819657-1.00006-2
Englezos, P., Ripmeester, J. A., Kumar, R., and Linga, P. (2008). “Hydrate processes for CO2 capture and scale-up using a new apparatus,” in Proceedings of the 6th international conference on gas hydrates (ICGH 2008) (Vancouver, British Columbia, Canada).
Esposito, A., and Benson, S. M. (2011). Remediation of possible leakage from geologic CO2 storage reservoirs into groundwater aquifers. Energy Procedia 4, 3216–3223. doi:10.1016/j.egypro.2011.02.238
Fagorite, V. I., Onyekuru, S. O., Opara, A. I., and Oguzie, E. E. (2023). The major techniques, advantages, and pitfalls of various methods used in geological carbon sequestration. Int. J. Environ. Sci. Technol. 20 (4), 4585–4614. doi:10.1007/s13762-022-04351-0
Fajardy, M., Morris, J., Gurgel, A., Herzog, H., Mac Dowell, N., and Paltsev, S. (2021). The economics of bioenergy with carbon capture and storage (BECCS) deployment in a 1.5°C or 2°C world. Glob. Environ. Change 68, 102262. doi:10.1016/j.gloenvcha.2021.102262
Fan, L. S., and Li, H. (2019). Chemical looping technology for clean energy utilization. John Wiley and Sons.
Fan, S., Yu, W., Yu, C., Wang, Y., Lang, X., Wang, S., et al. (2022). Investigation of enhanced exploitation of natural gas hydrate and CO2 sequestration combined gradual heat stimulation with CO2 replacement in sediments. J. Nat. Gas Sci. Eng. 104, 104686. doi:10.1016/j.jngse.2022.104686
Farmahini, A. H., Krishnamurthy, S., Friedrich, D., Brandani, S., and Sarkisov, L. (2018). From crystal to adsorption column: challenges in multiscale computational screening of materials for adsorption separation processes. Industrial and Eng. Chem. Res. 57 (45), 15491–15511. doi:10.1021/acs.iecr.8b03065
Fatima, S. S., Borhan, A., Ayoub, M., and Abd Ghani, N. (2021). Development and progress of functionalized silica-based adsorbents for CO2 capture. J. Mol. Liq. 338, 116913. doi:10.1016/j.molliq.2021.116913
Fawad, M., and Mondol, N. H. (2021). Monitoring geological storage of CO2: a new approach. Sci. Rep. 11, 5942. doi:10.1038/s41598-021-85346-8
Filgueira, R., Strohmeier, T., and Strand, Ø. (2019). Regulating services of bivalve molluscs in the context of the carbon cycle and implications for ecosystem valuation. Goods and services of marine bivalves, 231–251.
Flohr, A., Matter, J. M., James, R. H., Saw, K., Brown, R., Gros, J., et al. (2021). Utility of natural and artificial geochemical tracers for leakage monitoring and quantification during an offshore controlled CO2 release experiment. Int. J. Greenh. Gas Control 111, 103421. doi:10.1016/j.ijggc.2021.103421
Flude, S., Johnson, G., Gilfillan, S. M. V., and Haszeldine, R. S. (2016). Inherent tracers for carbon capture and storage in sedimentary formations: composition and applications. Environ. Sci. Technol. 50, 7939–7955. doi:10.1021/acs.est.6b01548
Folger, P. F. (2014). Carbon capture and sequestration: research, development, and demonstration at the. US Department of Energy. Available at: https://crsreports.congress.gov/.
Fridahl, M., and Lehtveer, M. (2018). Bioenergy with carbon capture and storage (BECCS): global potential, investment preferences, and deployment barriers. Energy Res. and Soc. Sci. 42, 155–165. doi:10.1016/j.erss.2018.03.019
Fujioka, M., Yamaguch, S., and Nako, M. (2010). CO2- ECBM field tests in the Ishikari coal basin of Japan. Int. Journal Coal Geol. 82 (3-4), 287–298. doi:10.1016/j.coal.2010.01.004
Gan, W., and Frohlich, C. (2013). Gas injection may have triggered earthquakes in the Cogdell oil field, Texas. Proc. Natl. Acad. Sci. 110, 18786–18791. doi:10.1073/pnas.1311316110
Gao, X., Yang, S., Shen, B., Tian, L., Li, S., Zhang, X., et al. (2023). Influence of reservoir spatial heterogeneity on a multicoupling process of CO2 geological storage. Energy and Fuels 37 (19), 14991–15005. doi:10.1021/acs.energyfuels.3c02784
Ge, J., Cowan, R. M., Tu, C., McGregor, M. L., and Trachtenberg, M. C. (2002). Enzyme-based CO2 capture for advanced life support. Life Support and Biosphere Sci. 8 (3-4), 181–189.
Gentzis, T. (2000). Subsurface sequestration of carbon dioxide-an overview from an Alberta (Canada) perspective. Int. J. Coal Geol. 43 (1-4), 287–305. doi:10.1016/s0166-5162(99)00064-6
Gerstenberger, M., Nicol, A., Stenhouse, M., Berryman, K., Stirling, M., Webb, T., et al. (2009). Modularised logic tree risk assessment method for carbon capture and storage projects. Energy Procedia 1 (1), 2495–2502. doi:10.1016/j.egypro.2009.02.012
Ghafoori, M., Tabatabaei-Nejad, S. A., and Khodapanah, E. (2017). Modeling rock-fluid interactions due to CO2 injection into sandstone and carbonate aquifer considering salt precipitation and chemical reactions. J. Nat. Gas Sci. Eng. 37, 523–538. doi:10.1016/j.jngse.2016.11.063
Gholami, R., Raza, A., and Iglauer, S. (2021). Leakage risk assessment of a CO2 storage site: a review. Earth-Science Rev. 223, 103849. doi:10.1016/j.earscirev.2021.103849
Gielen, D. (2003). Proceedings of the 2nd annual conference on carbon sequestration. Paris, France Alexandria, VA: International Energy Agency, 9 Rue de la Fédération, 5–8.
Global CCS Institute (2022). The global status of CCS: 2022 summary report. Melbourne: Global CCS Institute.
Glubokovskikh, S., Saygin, E., Shapiro, S., Gurevich, B., Isaenkov, R., Lumley, D., et al. (2022). A small CO2 leakage may induce seismicity on a sub-seismic fault in a good-porosity clastic saline aquifer. Geophys. Res. Lett. 49, e2022GL098062. doi:10.1029/2022gl098062
Godec, M., Koperna, G., and Gale, J. (2014). CO2-ECBM: a review of its status and global potential. Energy Procedia 63, 5858–5869. doi:10.1016/j.egypro.2014.11.619
Godec, M., Koperna, G., Petrusak, R., and Oudinot, A. (2014). Enhanced gas recovery and CO2 storage in gas shales: a summary review of its status and potential. Energy Procedia 66, 5849–5857. doi:10.1016/j.egypro.2014.11.618
Golze, D., Hirvensalo, M., Hernandez-Leon, P., Aarva, A., Etula, J., Susi, T., et al. (2022). Accurate computational prediction of core-electron binding energies in carbon-based materials: a machine-learning model combining density-functional theory and gw. Chem. Mater. 34 (14), 6240–6254. doi:10.1021/acs.chemmater.1c04279
Goodarzi, S., Settari, A., Zoback, M., and Keith, D. W. (2013). “Thermal effects on shear fracturing and injectivity during CO2 storage,” in Effective and sustainable hydraulic fracturing. Editors A. P. Bunger, J. McLennan, and R. Jeffrey (Rijeka: IntechOpen). Ch. 48.
Gor, G. Y., and Prévost, J. H. (2013). Effect of CO2 injection temperature on caprock stability. Energy Procedia 37, 3727–3732. doi:10.1016/j.egypro.2013.06.267
Gunter, W. D., Bachu, S., Buschkuehle, M., Michael, K., Ordorica-Garcia, G., and Hauck, T. (2009). Reduction of GHG emissions by geological storage of CO2: anatomy of the Heartland aquifer redwater carbon capture and geological storage project (HARP), Alberta, Canada. Int. J. Clim. Change Strategies Manag. 1 (2), 160–178. doi:10.1108/17568690910955621
Guofeng, W. A. N. G. (2023). Carbon dioxide capture, enhanced-oil recovery and storage technology and engineering practice in Jilin Oilfield, NE China. Petroleum Explor. Dev. 50 (1), 245–254. doi:10.1016/s1876-3804(22)60384-7
Gupta, M., Coyle, I., and Thambimuthu, K. (2003). CO2 capture technologies and opportunities in Canada - strawman document for CO2 capture and storage technology roadmap. Tech. Rep. Canmet Energy Technol. Cent. - Nat. Resour. Can. Calgary, 2003.
Habert, G., Miller, S. A., John, V. M., Provis, J. L., Favier, A., Horvath, A., et al. (2020). Environmental impacts and decarbonization strategies in the cement and concrete industries. Natl. Rev. Earth Environ. 1, 559–573. doi:10.1038/s43017-020-0093-3
Han, L., Zhang, Y., and Hu, J. (2024). Modeling and evaluating CO2 storage capacity in saline aquifer with modified brine density using electrolyte perturbed-channel statistical associating fluid theory. Energy and Fuels 38 (13), 11994–12004. doi:10.1021/acs.energyfuels.4c01757
Haq, B., Muhammed, H. S., Liu, J., and Chua, H. T. (2023). Enhanced natural gas production using CO2 injection: application to sustainable hydrogen production. Fuel 347, 128474. doi:10.1016/j.fuel.2023.128474
Harati, S., Gomari, S. R., Gasanzade, F., Bauer, S., Pak, T., and Orr, C. (2023). Underground hydrogen storage to balance seasonal variations in energy demand: impact of well configuration on storage performance in deep saline aquifers. Int. J. Hydrogen Energy 48 (69), 26894–26910. doi:10.1016/j.ijhydene.2023.03.363
Hardisty, P. E., Sivapalan, M., and Brooks, P. (2011). The environmental and economic sustainability of carbon capture and storage. Int. J. Environ. Res. Public Health 8, 1460–1477. doi:10.3390/ijerph8051460
Haszeldinez, R. S. (2009). Carbon capture and storage: how green can black be? Science 325 (5948), 1647–1652. doi:10.1126/science.1172246
Herzog, H., and Golomb, D. (2004). Carbon dioxide capture and storage from fossil fuel use. Encycl. Energy 1, 1–11.
Hildenbrand, A., Schlomer, S., and Kross, B. M. (2002). Gas breakthrough experiments on fine-grained sedimentary rocks. Geofluids 2 (1), 3–23. doi:10.1046/j.1468-8123.2002.00031.x
Holloway, S. (2005). Underground sequestration of carbon dioxide—a viable greenhouse gas mitigation option. Energy 30 (11-12), 2318–2333. doi:10.1016/j.energy.2003.10.023
Hosseinpour, M., Shojaei, M. J., Salimi, M., and Amidpour, M. (2023). Machine learning in absorption-based post-combustion carbon capture systems: a state-of-the-art review. Fuel 353, 129265. doi:10.1016/j.fuel.2023.129265
Hu, L., Clark, K., Alebrahim, T., and Lin, H. (2022). Mixed matrix membranes for post-combustion carbon capture: From materials design to membrane engineering. Journal of Membrane Science 644, 120140. doi:10.1016/j.memsci.2021.120140
Huaman, R. N. E., and Jun, T. X. (2014). Energy related CO2 emissions and the progress on CCS projects: a review. Renew. Sustain. Energy Rev. 31, 368–385. doi:10.1016/j.rser.2013.12.002
Huang, X., Zhao, Y. P., Wang, X., and Pan, L. (2019). Adsorption-induced pore blocking and its mechanisms in nanopores shale due to interactions with supercritical CO2. J. Petroleum Sci. Eng. 178, 74–81. doi:10.1016/j.petrol.2019.03.018
Humez, P., Lagneau, V., Lions, J., and Negrel, P. (2013). Assessing the potential consequences of CO2 leakage to freshwater resources: a batch-reaction experiment towards an isotopic tracing tool. Appl. Geochem. 30, 178–190. doi:10.1016/j.apgeochem.2012.07.014
Hung, H. V., Sugai, Y., Nguele, R., and Sasaki, K. (2019). Integrated workflow in 3D geological model construction for evaluation of CO2 storage capacity of a fractured basement reservoir in Cuu Long Basin, Vietnam. Int. J. Greenh. Gas Control 90, 102826. doi:10.1016/j.ijggc.2019.102826
Ide, S. T., Friedmann, S. J., and Herzog, H. J. (2006). CO2 leakage through existing wells: current technology and regulations. 8th international conference on greenhouse gas control technologies 1, 19–33.
Iglauer, S. (2017). CO2–water–rock wettability: variability, influencing factors, and implications for CO2 geostorage. Accounts Chem. Res. 50 (5), 1134–1142. doi:10.1021/acs.accounts.6b00602
Ignatusha, P., Lin, H., Kapuscinsky, N., Scoles, L., Ma, W., Patarachao, B., et al. (2024). Membrane separation technology in direct air capture. Membr. (Basel) 14 (2), 30. doi:10.3390/membranes14020030
International Energy Agency (IEA) (2022). Legal and regulatory frameworks for CCUS. Paris: IEA. Available at: https://www.iea.org/reports/legal-and-regulatory-frameworks-for-ccus.
Isahak, W. N. R. W., Ramli, Z. A. C., Hisham, M. W. M., and Yarmo, M. A. (2015). The formation of a series of carbonates from carbon dioxide: capturing and utilisation. Renew. Sustain. Energy Rev. 47, 93–106. doi:10.1016/j.rser.2015.03.020
Islam, A. W., and Sun, A. Y. (2017). Detecting CO2 leakage around the wellbore by monitoring temperature profiles: a scoping analysis. Int. J. Therm. Sci. 118, 367–373. doi:10.1016/j.ijthermalsci.2017.04.030
Isogai, H., and Nakagaki, T. (2024). Power-to-heat amine-based post-combustion CO2 capture system with solvent storage utilizing fluctuating electricity prices. Appl. Energy 368, 123519. doi:10.1016/j.apenergy.2024.123519
Jablonka, K. M., Charalambous, C., Fernandez, E. S., Wiechers, G., Monteiro, J., Moser, P., et al. (2023). Machine learning for industrial processes: forecasting amine emissions from a carbon capture plant. Sci. Adv. 9, eadc9576. doi:10.1126/sciadv.adc9576
Jensen, G. K., Nickel, E. H., Whittaker, S., and Rostron, B. J. (2009). Geological model and hydrogeological framework of an active CO2 sequestration project in the Weyburn–Midale area, Saskatchewan: leading to a further understanding of possible CO2 migration. Energy Procedia 1 (1), 2983–2989. doi:10.1016/j.egypro.2009.02.075
Jeong, S., Lee, H. A., and Yoon, H. O. (2017). Analytical methods for geochemical monitoring of CO2 capture and storage sites. Geoscinces J. 21, 631–643. doi:10.1007/s12303-017-0010-z
Jewell, S., and Senior, B. (2012). CO2 storage liabilities in the North Sea: an assessment of risks and financial consequences. Summ. Rep. DECC.
Ju, Y., Beaubien, S. E., Lee, S.-S., Kaown, D., Hahm, D., Lee, S., et al. (2019). Application of natural and artificial tracers to constrain CO2 leakage and degassing in the K-COSEM site, South Korea. Int. J. Greenh. Gas Control 86, 211–225. doi:10.1016/j.ijggc.2019.05.002
Juenger, M. C., Snellings, R., and Bernal, S. A. (2019). Supplementary cementitious materials: new sources, characterization, and performance insights. Cem. Concr. Res. 122, 257–273. doi:10.1016/j.cemconres.2019.05.008
Kalam, S., Olayiwola, T., Al-Rubaii, M. M., Amaechi, B. I., Jamal, M. S., and Awotunde, A. A. (2021). Carbon dioxide sequestration in underground formations: review of experimental, modeling, and field studies. J. Petroleum Explor. Prod. 11, 303–325. doi:10.1007/s13202-020-01028-7
Kelemen, P., Benson, S. M., Pilorge, H., Psarras, P., and Wilcox, J. (2019). An Overview of the status and challenges of CO2 storage in minerals and geological formations. Front. Clim. Negat. Emiss. Technol. 1, 1–9. doi:10.3389/fclim.2019.00009
Kelley, M., Haagsma, A., Champagne, P., Glier, J., Gupta, N., Yugulis, M. H., et al. (2018). Integrated pre-feasibility assessment for a northern Michigan basin CarbonSAFE CO2 storage complex battelle. Richland, WA (United States).
Kerr, T., Havercroft, I., and Dixon, T. (2009). Legal and regulatory developments associated with carbon dioxide capture and storage: a global update. Energy Procedia 1, 4395–4402. doi:10.1016/j.egypro.2009.02.254
Khalik, M. S., and Behzad, P. (2018). Recent advances on carbon dioxide capture through a hydrate-based gas separation process. Green Process. Technol. 11, 22–26. doi:10.1016/j.cogsc.2018.03.006
Khalilidermani, M., and Knez, D. (2024). Shear wave velocity applications in geomechanics with focus on risk assessment in carbon capture and storage projects. Energies 17, 1578. doi:10.3390/en17071578
Khokhar, A. A., Gudmundsson, J. S., and Sloan, E. D. (1998). Gas storage in structure H hydrates. Fluid Phase Equilibria 150, 383–392. doi:10.1016/s0378-3812(98)00338-0
Khudaida, K. J., and Das, D. B. A. (2020). Numerical analysis of the effects of supercritical CO2 injection on CO2 storage capacities of geological formations. Clean. Technol. 2, 333–364. doi:10.3390/cleantechnol2030021
Khurshid, I., and Fujii, Y. (2021). Geomechanical analysis of formation deformation and permeability enhancement due to low-temperature CO2 injection in subsurface oil reservoirs. J. Petroleum Explor. Prod. 11, 1915–1923. doi:10.1007/s13202-021-01133-1
Kim, M., Kim, K.-Y., Kim, C. Y., Chae, G.-T., Han, W. S., and Park, E. (2020). Characterizing tracer transport behavior in two-phase flow system: implications for CO2 geosequestration. Geophys. Res. Lett. 47, e2020GL089262. doi:10.1029/2020gl089262
Kim, S., Park, J., Seol, S. J., and Byun, J. (2024). Machine learning-based time-lapse 1D seismic full-waveform inversion with efficient training data generation in a carbon capture and storage monitoring. Geoenergy Sci. Eng. 238, 212852. doi:10.1016/j.geoen.2024.212852
Kim, Y., Jang, H., Kim, J., and Jeonghwan, L. (2017). Prediction of storage efficiency on CO2Sequestration in deep saline aquifers using artificial neural network. Appl. Energy 185, 916–928. doi:10.1016/j.apenergy.2016.10.012
Kim, Y. H., and Park, Y. G. (2023). A review of CO2 plume dispersion modeling for application to offshore carbon capture and storage. J. Mar. Sci. Eng. 12 (1), 38. doi:10.3390/jmse12010038
Koperna, G., Riestenberg, D., Kuuskraa, V., Rhudy, R., Trautz, R., Hill, G. R., et al. (2012). The SECARB anthropogenic test: a US integrated CO2 capture. Transp. storage test.
Kulshrestha, A., Kumar, R., and Sharma, K. P. (2024). Efficient carbon capture and mineralization using porous liquids comprising hollow nanoparticles and enzymes dispersed in fatty acid-based ionic liquids. ACS Sustain. Chem. and Eng. 12 (15), 5799–5808. doi:10.1021/acssuschemeng.3c07182
Kumar, D., and Kumar, D. (2018). “Coal usage and control of CO2 emissions,” in Sustainable Management of coal preparation (Woodhead Publishing).
Kumar, N., Sampaio, M. A., Ojha, K., Hoteit, H., and Mandal, A. (2022). Fundamental aspects, mechanisms and emerging possibilities of CO2 miscible flooding in enhanced oil recovery: a review. Fuel 330, 125633. doi:10.1016/j.fuel.2022.125633
Kumar, R., Mangalapuri, R., Ahmadi, M. H., Vo, D. V. N., Solanki, R., and Kumar, P. (2020). The role of nanotechnology on post-combustion CO2 absorption in process industries. Int. J. Low-Carbon Technol. 15 (3), 361–367. doi:10.1093/ijlct/ctaa002
Kuznetsov, V. A., Bozheeva, D. M., and Minakov, A. V. (2023). Entrained-flow oxy-gasification of pulverized coal in CO2-H2O-O2 environment. Environ. Sci. Pollut. Res. 30 (55), 117435–117447. doi:10.1007/s11356-023-30401-2
Lackner, K. S. (2003). Climate change: a guide to CO2 sequestration. Science 300 (5626), 1677–1678. doi:10.1126/science.1079033
Lackner, K. S. (2009). A guide to CO2 sequestration. Science 325 (5948), 1647–1652. doi:10.1126/science.1172246
Lei, Q., Li, L., Chen, H., and Wang, X. (2023). Emerging directions for carbon capture technologies: a synergy of high throughput theoretical calculations and machine learning. Environ. Sci. and Technol. 57, 17189–17200. doi:10.1021/acs.est.3c05305
Leonzio, G., and Shah, N. (2024). Recent advancements and challenges in carbon capture, utilization and storage. Curr. Opin. Green Sustain. Chem. 46, 100895. doi:10.1016/j.cogsc.2024.100895
Leung, D. Y. C., Caramanna, G., and Maroto-Valer, M. M. (2014). An overview of current status of carbon dioxide capture and storage technologies. Renew. Sustain. Energy Rev. 39, 426–443. doi:10.1016/j.rser.2014.07.093
Li, B., Duan, Y., Luebke, D., and Morreale, B. (2013). Advances in CO2 capture technology: a patent review. Appl. Energy 102, 1439–1447. doi:10.1016/j.apenergy.2012.09.009
Li, B., Zhou, F., Li, H., Duguid, A., Que, L., Xue, Y., et al. (2018b). Prediction of CO2 leakage risk for wells in carbon sequestration fields with an optimal artificial neural network. Int. J. Greenh. Gas Control 68, 276–286. doi:10.1016/j.ijggc.2017.11.004
Li, C., and Laloui, L. (2017). Impact of material properties on caprock stability in CO2 geological storage. Geomechanics Energy Environ. 11, 28–41. doi:10.1016/j.gete.2017.06.003
Li, F., Zhang, J., Oko, E., and Wang, M. (2015). Modelling of a post-combustion CO2 capture process using neural networks. Fuel 151, 156–163. doi:10.1016/j.fuel.2015.02.038
Li, F., Zhang, J., Shang, C., Huang, D., Oko, E., and Wang, M. (2018a). Modelling of a post-combustion CO2 capture process using deep belief network. Appl. Therm. Eng. 130, 997–1003. doi:10.1016/j.applthermaleng.2017.11.078
Lichtschlag, A., Pearce, C. R., Suominen, M., Blackford, J., Borisov, S. M., Bull, J. M., et al. (2021). Suitability analysis and revised strategies for marine environmental carbon capture and storage (CCS) monitoring. Int. J. Greenh. Gas Control 112, 103510. doi:10.1016/j.ijggc.2021.103510
Lin, X., Zhang, Y., Liu, H., Boczkaj, , Cao, Y., and Wang, C. (2024). Carbon dioxide sequestration by industrial wastes through mineral carbonation: current status and perspectives. J. Clean. Prod. 434, 140258. doi:10.1016/j.jclepro.2023.140258
Linderholm, C., Lyngfelt, A., and Dueso, C. (2013). Chemical-looping combustion of solid fuels in a 10 kW reactor system using natural minerals as oxygen carrier. Energy Procedia 37, 598–607. doi:10.1016/j.egypro.2013.05.147
Linga, P., Haligva, C., Nam, S. C., Ripmeester, J. A., and Englezos, P. (2009). Gas hydrate formation in a variable volume bed of silica sand particles. Energy fuels. 23, 5496–5507. doi:10.1021/ef900542m
Lions, J., Devau, N., de Lary, L., Dupraz, S., Parmentier, M., Gombert, P., et al. (2014). Potential impacts of leakage from CO2 geological storage on geochemical processes controlling fresh groundwater quality: a review. Int. J. Greenh. Gas Control 22, 165–175. doi:10.1016/j.ijggc.2013.12.019
Liu, J., Xie, L., Yao, Y., Gan, Q., Zhao, P., and Du, L. (2019). Preliminary study of influence factors and estimation model of the enhanced gas recovery stimulated by carbon dioxide utilization in shale. ACS Sustain. Chem. Eng. 7, 20114–20125. doi:10.1021/acssuschemeng.9b06005
Loeve, D., Hofstee, C., and Maas, J. G. (2014). Thermal effects in a depleted gas field by cold CO2 injection in the presence of methane. Energy Procedia 63, 5378–5393. doi:10.1016/j.egypro.2014.11.569
Long, N. V. D., Lee, J., Koo, K. K., Luis, P., and Lee, M. (2017). Recent progress and novel applications in enzymatic conversion of carbon dioxide. Energies 10 (4), 473. doi:10.3390/en10040473
Lu, J., Kordi, M., Hovorka, S. D., Meckel, T. A., and Christopher, C. A. (2013). Reservoir characterization and complications for trapping mechanisms at Cranfield CO2 injection site. Int. J. Greenh. Gas Control 18, 361–374. doi:10.1016/j.ijggc.2012.10.007
Lu, P., Apps, J., Zhang, G., Gysi, A., and Zhu, C. (2024). Knowledge gaps and research needs for modeling CO2 mineralization in the basalt-CO2-water system: a review of laboratory experiments. Earth-Science Rev. 254, 104813. doi:10.1016/j.earscirev.2024.104813
Ma, J., Zhou, Y., Zheng, Y., He, L., Wang, H., Niu, L., et al. (2024). Advances in geochemical monitoring technologies for CO2 geological storage. Sustainability 16, 6784. doi:10.3390/su16166784
Maniarasu, R., Rathore, S. K., and Murugan, S. (2023). A review on materials and processes for carbon dioxide separation and capture. Energy and Environ. 34 (1), 3–57. doi:10.1177/0958305x211050984
Mao, Y., Zeidouni, M., and Askari, R. (2017). Effect of leakage pathway flow properties on thermal signal associated with the leakage from CO2 storage zone Greenhouse. Gases Sci. Technol. 7, 512–529. doi:10.1002/ghg.1658
Martin, D., Lal, T., Sachdev, C. B., and Sharma, J. P. (2010). Soil organic carbon storage changes with climate change, landform and land use conditions in Garhwal hills of the Indian Himalayan mountains. Ecosyst. and Environ. 138 (1–2), 64–73. doi:10.1016/j.agee.2010.04.001
Masaoka, N., Kosugi, K. I., Yamakawa, Y., and Tsutsumi, D. (2016). Processes of bedrock groundwater seepage and their effects on soil water fluxes in a foot slope area. J. Hydrology 535, 160–172. doi:10.1016/j.jhydrol.2016.01.081
Massarweh, O., and Abushaikha, A. S. (2024). CO2 sequestration in subsurface geological formations: a review of trapping mechanisms and monitoring techniques. Earth-Science Rev. 253, 104793. doi:10.1016/j.earscirev.2024.104793
Mathias, S. A., Gluyas, J. G., Oldenburg, C. M., and Tsang, C. F. (2010). Analytical solution for Joule–Thomson cooling during CO2 geo-sequestration in depleted oil and gas reservoirs. Int. J. Greenh. Gas Control 4 (5), 806–810. doi:10.1016/j.ijggc.2010.05.008
Mathieson, A., Wright, I., Roberts, D., and Ringrose, P. (2009). Satellite imaging to monitor CO2 movement at Krechba, Algeria. Energy Procedia 1, 2201–2209. doi:10.1016/j.egypro.2009.01.286
McGrail, B. P., Freeman, C. J., Beeman, G. H., Sullivan, E. C., Wurstner, S. K., and Brown, C. F. (2010). Capture and sequestration of CO2 at the boise white paper mill. Tech. Rep. doi:10.2172/1013647
McGrail, B. P., Schaef, H. T., Glezakou, V. A., Dang, L. X., and Owen, A. T. (2009). Water reactivity in the liquid and supercritical CO2 phase: has half the story been neglected? Energy Procedia 1 (1), 3415–3419. doi:10.1016/j.egypro.2009.02.131
McLeod, J., Ferguson, I., Craven, J., Roberts, B., and Giroux, B. (2018). Pre-injection magnetotelluric surveys at the Aquistore CO2 sequestration site, Estevan, Saskatchewan. Can. Int. J. Greenh. Gas Control 74, 99–118. doi:10.1016/j.ijggc.2018.04.024
McPherson, B. J. O. L., and Cole, B. S. (2000). Multiphase CO2 flow, transport and sequestration in the Powder River Basin, Wyoming, USA. J. Geochem. Explor. 69, 65–69. doi:10.1016/s0375-6742(00)00046-7
Metz, B., Davidson, O., De Coninck, H. C., Loos, M., and Meyer, L. (2005). IPCC special report on carbon dioxide capture and storage. Cambridge, United Kingdom: Cambridge University Press.
Michael, K., Golab, A., Shulakova, V., EnnisKing, J., Allinson, G., Sharma, S., et al. (2010). Geological storage of CO2 in saline aquiferA review of the experience from existing storage operations. Int. J. Greenh. Gas Control 4, 659–667. doi:10.1016/j.ijggc.2009.12.011
Milad, B., Moghanloo, R. G., and Hayman, N. W. (2024). Assessing CO2 geological storage in Arbuckle Group in northeast Oklahoma. Fuel 356, 129323. doi:10.1016/j.fuel.2023.129323
Milano, M., and Fedi, M. (2023). Surface gravity response of CO2 storage in the Johansen deep reservoir. IEEE Trans. Geoscience Remote Sens. 61, 1–14. doi:10.1109/tgrs.2023.3330778
Miocic, J. M., Gilfillan, S. M. V., Frank, N., Schroeder-Ritzrau, A., Burnside, N. M., and Haszeldine, R. S. (2019). 420,000-year assessment of fault leakage rates shows geological carbon storage is secure. Sci. Rep. 9, 769. doi:10.1038/s41598-018-36974-0
Molahid, V. L. M., Kusin, F. M., and Syed Hasan, S. N. M. (2023). Mineralogical and chemical characterization of mining waste and utilization for carbon sequestration through mineral carbonation. Environ. Geochem. Health 45 (7), 4439–4460. doi:10.1007/s10653-023-01513-y
Mondal, M. K., Balsora, H. K., and Varshney, P. (2012). Progress and trends in CO2 capture/separation technologies: a review. Energy 46, 431–441. doi:10.1016/j.energy.2012.08.006
Monkman, S., Grandfield, K., and Langelier, B. (2018). “On the mechanism of using carbon dioxide as a beneficial concrete admixture,” in SP 329 proceedings twelfth international conference (Beijing, China: American Concrete Institute), 415–428.
Montana Golden triangle (2009). Montana Golden triangle. Available at: https://www.montana.edu/energy/carbon.html.
Montgomery, S. L. (1999). Powder River Basin, Wyoming: an expanding coalbed methane (CBM) play. AAPG Bull. 83 (8), 1207–1222. doi:10.1306/E4FD3333-1732-11D7-8645000102C1865D
Muller, L. J., Katelhon, A., Bachmann, M., Zimmermann, A., Sternberg, A., and Bardow, A. (2020). A guideline for life cycle assessment of carbon capture and utilization. Front. Energy Res. 8, 15. doi:10.3389/fenrg.2020.00015
Myers, M., Stalker, L., Pejcic, B., and Ross, A. (2013). Tracers – past, present and future applications in CO2 geosequestration. Appl. Geochem. 30, 125–135. doi:10.1016/j.apgeochem.2012.06.001
Nandi, M., and Uyama, H. (2014). Exceptional CO2 adsorbing materials under different conditions. Chem. Rec. 14, 1134–1148. doi:10.1002/tcr.201402062
Nassef, A. M. (2023). Improving CO2 absorption using artificial intelligence and modern optimization for a sustainable environment. Sustainability 15 (12), 9512. doi:10.3390/su15129512
Ndlovu, P. (2022). Carbon dioxide encapsulation in methane hydrates. Durban. South Africa: University of KwaZulu Natal. Ph.D thesis. School of Engineering.
Ndlovu, P., Babaee, S., and Naidoo, P. (2022). Review on CH4-CO2 replacement for CO2 sequestration and CH4/CO2 hydrate formation in porous media. Fuel 320, 123795. doi:10.1016/j.fuel.2022.123795
Ndlovu, P., Babaee, S., and Naidoo, P. (2023). Experimental study of CH4-CO2 replacement in gas hydrates in the presence of nitrogen and graphene nanoplatelets. J. Mol. Liq. 371, 121109. doi:10.1016/j.molliq.2022.121109
Ndlovu, P., Babaee, S., Naidoo, P., and Moodley, K. (2024a). Kinetics studies of Gas Hydrate for CO2 capture in the presence of nanoparticles. Industrial Eng. Chem. Res. 63 (9), 3867–3879. doi:10.1021/acs.iecr.3c04061
Ndlovu, P., Babaee, S., Naidoo, P., and Moodley, K. (2024b). Utilization of nanoparticles to improve the kinetics of CH4 gas hydrates in gas storage applications. Energy and Fuels 38, 4480–4491. doi:10.1021/acs.energyfuels.3c04584
Neal, P. R., Hou, W., and Allinson, W. G. (2014). Project-based storage capacity and project maturity. Energy Procedia 63, 5239–5246. doi:10.1016/j.egypro.2014.11.555
NETL Powder River Basin (2007). NETL Powder River Basin. Available at: https://netl.doe.gov/node/2982.
Nicol, A., Carne, R., Gerstenberger, M., and Christophersen, A. (2011). Induced seismicity and its implications for CO2 storage risk. Greenh. Gas. Control Technol. 4, 3699–3706. doi:10.1016/j.egypro.2011.02.302
Nkosi, N., and Tumba, K. (2023). Optimization and energy assessment of carbon dioxide hydrate-based fruit juice concentration process. Food Bioprocess Technol. 17, 1845–1861. doi:10.1007/s11947-023-03228-5
Nomeli, M. A. (2014). Geochemical reactions modelling of carbon dioxide: theory. College Park: Department of Mechanical Engineering, University of Maryland. PhD Thesis.
Nordbotten, J. M., Fernø, M., Flemisch, B., Juanes, R., and Jørgensen, M. (2024). Experimentally assessing the uncertainty of forecasts of geological carbon storage. Int. J. Greenh. Gas Control 135, 104162. doi:10.1016/j.ijggc.2024.104162
Olajire, A. A. (2010). CO2 capture and separation technologies for end-of-pipe applications - a review. Energy 35, 2610–2628. doi:10.1016/j.energy.2010.02.030
Olivier, J. G. J., Schure, K. M., and Peters, J. H. W. (2017). Trends in global CO2 and total greenhouse gas emissions. The Hague: Netherlands Environmental Assessment Agency.
Oloye, O., and O'Mullane, A. P. (2021). Electrochemical capture and storage of CO2 as calcium carbonate. ChemSusChem 14 (7), 1767–1775. doi:10.1002/cssc.202100134
Onyia, B. N., and Osuma, O. (2010). Logistics challenges involved in constructing of operating facilities in mega-projects: a case study of cost overrun in Snøhvit LNG project. Høgskolen i Molde. Master's thesis.
Ota, M., Abe, Y., Watanabe, M., Smith, R. L., and Inomata, H. (2005). Methane recovery from methane hydrate using pressurized CO2. Fluid Phase Equilib. 228, 553–559. doi:10.1016/j.fluid.2004.10.002
Ouyang, Q., Fan, S., Wang, Y., Lang, X., Wang, S., Zhang, Y., et al. (2020). Enhanced methane production efficiency with in situ intermittent heating assisted CO2 replacement of hydrates. Energy Fuel 34 (10), 12476–12485. doi:10.1021/acs.energyfuels.0c02562
Ozkan, M. (2021). Direct air capture of CO2: a response to meet the global climate targets. MRS Energy& Sustain. 8 (2), 51–56. doi:10.1557/s43581-021-00005-9
Ozkan, M., Nayak, S. P., Ruiz, A. D., and Jiang, W. (2022). Current status and pillars of direct air capture technologies. iScience 25 (4), 103990. doi:10.1016/j.isci.2022.103990
Pagnier, H. J. M., van Bergen, F., Kreft, E., Van Der Meer, L. G. H., and Simmelink, H. J. (2005). “Field experiment of ECBM-CO2 in the upper silesian Basin of Poland (RECOPOL),” in SPE europec featured at EAGE conference and exhibition?, Madrid, Spain (SPE), SPE–94079.
Pan, S. Y., Chen, Y. H., Fan, L. S., Kim, H., Gao, X., Ling, T. C., et al. (2020). CO2 mineralization and utilization by alkaline solid wastes for potential carbon reduction. Nat. Sustain. 3 (5), 399–405. doi:10.1038/s41893-020-0486-9
Paulo, C., Power, I. M., Stubbs, A. R., Wang, B., Zeyen, N., and Wilson, S. (2021). Evaluating feedstocks for carbon dioxide removal by enhanced rock weathering and CO2 mineralization. Appl. Geochem. 129, 104955. doi:10.1016/j.apgeochem.2021.104955
Pawar, R. J., Watson, T. L., and Gable, C. W. (2009). Numerical simulation of CO2 leakage through abandoned wells: model for an abandoned site with observed gas migration in Alberta. Can. Greenh. Gas. Control Technol. 9 (1), 3625–3632. doi:10.1016/j.egypro.2009.02.158
Perdomo, J., Tovar, J., Smith, F., Verlinden, V., McIntosh, K., and Ibukun, O. (2013). Thermal fracturing in high and low permeability sandstone reservoir in the North Sea. European Association of Geoscientists and Engineers.
Petrovic, B., Gorbounov, M., and Soltani, S. M. (2022). Impact of surface functional groups and their introduction methods on the mechanisms of CO2 adsorption on porous carbonaceous adsorbents. Carbon Capture Sci. and Technol. 3, 100045. doi:10.1016/j.ccst.2022.100045
Proietti, G., Cvetković, M., Saftić, B., Conti, A., Romano, V., and Bigi, S. (2022). 3D modelling and capacity estimation of potential targets for CO2 storage in the Adriatic Sea, Italy. Pet. Geosci. 28 (1), petgeo2020–117. doi:10.1144/petgeo2020-117
Pruess, K. (2008). On production behavior of enhanced geothermal systems with CO2 as working fluid. Energy Convers. and Manag. 49 (6), 1446–1454. doi:10.1016/j.enconman.2007.12.029
Rahimi, M., Moosavi, S. M., Smit, B., and Hatton, T. A. (2021). Toward smart carbon capture with machine learning. Cell Rep. Phys. Sci. 2, 100396. doi:10.1016/j.xcrp.2021.100396
Raimi, D., Krupnick, A. J., Shah, J. S., and Thompson, A. (2021). Decommissioning orphaned and abandoned oil and gas wells: new estimates and cost drivers. Environ. Sci. Technol. 55, 10224–10230. doi:10.1021/acs.est.1c02234
Ramar, V., and Balraj, A. (2022). Critical review on carbon-based nanomaterial for carbon capture: technical challenges, opportunities, and future perspectives. Energy and Fuels 36 (22), 13479–13505. doi:10.1021/acs.energyfuels.2c02585
Ramos, G. M. S., Barbosa, J. A., Araujo, A. F. L., Filho, O. J. C., Barreto, C. J. S., Oliveira, J. T. C., et al. (2023). Potential for permanent CO2 sequestration in depleted volcanic reservoirs in the offshore Camps Basin, Brazil. Int. J. Greenh. Gas Control 128, 103942. doi:10.1016/j.ijggc.2023.103942
Ratnakar, R. R., Chaubey, V., and Dindoruk, B. (2023). A novel computational strategy to estimate CO2 solubility in brine solutions for CCUS applications. Appl. Energy 342, 121134. doi:10.1016/j.apenergy.2023.121134
Ren, D., Wang, X., Kou, Z., Wang, S., Wang, H., Wang, X., et al. (2023). Feasibility evaluation of CO2 EOR and storage in tight oil reservoirs: a demonstration project in the Ordos Basin. Fuel 331, 125652. doi:10.1016/j.fuel.2022.125652
Renfrew, S. E., Starr, D. E., and Strasser, P. (2020). Electrochemical approaches toward CO2 capture and concentration. ACS Catal. 10 (21), 13058–13074. doi:10.1021/acscatal.0c03639
Rheinhardt, J. H., Singh, P., Tarakeshwar, P., and Buttry, D. A. (2017). Electrochemical capture and release of carbon dioxide. ACS Energy Lett. 2 (2), 454–461. doi:10.1021/acsenergylett.6b00608
Riaz, A., and Cinar, Y. (2014). Carbon dioxide sequestration in saline formations: Part I—review of the modeling of solubility trapping. J. Petroleum Sci. Eng. 124, 367–380. doi:10.1016/j.petrol.2014.07.024
Riddiford, F. A., Tourqui, A., Bishop, C. D., Taylor, B., and Smith, M. (2003). “A cleaner development: the in Salah Gas project, Algeria,” in Greenhouse gas control technologies-6th international conference (Pergamon), 595–600.
Roberts, J. J., Gilfillan, S. M. V., Stalker, L., and Naylor, M. (2017). Geochemical tracers for monitoring offshore CO2 stores. Int. J. Greenh. Gas Control 65, 218–234. doi:10.1016/j.ijggc.2017.07.021
Roberts, J. J., Wood, R. A., Wilkinson, M., and Haszeldine, S. (2015). Surface controls on the characteristics of natural CO2 seeps: implications for engineered CO2 stores. Geofluids 15 (3), 453–463. doi:10.1111/gfl.12121
Rogers, K. L., Neuhoff, P. S., Pedersen, A. K., and Bird, D. K. (2006). CO2 metasomatism in a basalt-hosted petroleum reservoir, Nuussuaq, West Greenland. Lithos 92 (1-2), 55–82. doi:10.1016/j.lithos.2006.04.002
Romasheva, N., and Cherepovitsyna, A. (2019). CCS projects: how regulatory framework influences their deployment. Resources 8 (4), 181. doi:10.3390/resources8040181
Sabil, K. M., and Partoon, B. (2018). Recent advances on carbon dioxide capture through a hydrate-based gas separation process. Curr. Opin. Green Sustain. Chem. 11, 22–26. doi:10.1016/j.cogsc.2018.03.006
Sahoo, P.C., Kumar, M., Puri, S. K., and Ramakumar, S. S. V. (2018). Enzyme inspired complexes for industrial CO2 capture: opportunities and challenges. Journal of CO2 Utilization 24, 419–429. doi:10.1016/j.jcou.2018.02.003
Said, S., Govindaraj, V., Herri, J. M., Ouabbas, Y., Khodja, M., Belloum, M., et al. (2016). A study on the influence of nanofluids on gas hydrate formation kinetics and their potential: application to the CO2 capture process. J. Nat. Gas Sci. Eng. 32, 95–108. doi:10.1016/j.jngse.2016.04.003
Saldi, G. D., Jordan, G., Schott, J., and Oelkers, E. H. (2009). Magnesite growth rates as a function of temperature and saturation state. Geochimica Cosmochimica Acta 73 (19), 5646–5657. doi:10.1016/j.gca.2009.06.035
Schuiling, R. D., and Krijgsman, P. (2006). Enhanced weathering: an effective and cheap tool to sequester CO2. Clim. Change 74 (1-3), 349–354. doi:10.1007/s10584-005-3485-y
Seiiedhoseiny, M., Ghasemzadeh, K., and Basile, A. (2024). “Membrane technology in integrated gasification combined cycles,” in Current trends and future developments on (bio-) membranes, 743–763.
Seo, Y., Lee, S., and Lee, J. (2013). Experimental verification of methane replacement in gas hydrate by carbon dioxide. Italian Association of Chemical Engineering 32. doi:10.3303/CET1332028
Shadravan, A., and Amani, M. (2015). “A decade of self-sealing cement technology application to ensure long-term well integrity,” in Paper presented at the SPE Kuwait oil and gas show and conference (Mishref, Kuwait). October 2015.
Shalaby, A., Elkamel, A., Douglas, P., Zhu, Q., and Zheng, P. Q. (2021). A machine learning approach for modelling and optimization of a CO2 post-combustion capture unit. Energy 215, 119113. doi:10.1016/j.energy.2020.119113
Shao, P., Ye, J., Shen, Y., Zhang, S., and Zha, J. (2024). Recent advancements in carbonic anhydrase for CO2 capture: a Mini Review. Gas Sci. Eng. 12, 205237. doi:10.1016/j.jgsce.2024.205237
Sharifian, R., Wagterveld, R. M., Digdaya, I. A., Xiang, C. X., and Vermaas, D. A. (2021). Electrochemical carbon dioxide capture to close the carbon cycle. Energy and Environ. Sci. 14 (2), 781–814. doi:10.1039/d0ee03382k
Sharma, T., Sharma, S., Kamyab, H., and Kumar, A. (2020). Energizing the CO2 utilization by chemo-enzymatic approaches and potentiality of carbonic anhydrases: a review. J. Clean. Prodoction 247, 119138. doi:10.1016/j.jclepro.2019.119138
Shell Canada (2019). Quest carbon capture and storage. Available at: https://www.shell.ca/en_ca/about-us/projects-and-sites/quest-carbon-capture-and-storage-project.html (Accessed January 10, 2019).
Shervani, S., Tansug, L. P., and Tezel, F. H. (2024). Microporous adsorbent-based mixed matrix membranes for CO2/N2 separation. Energies 17 (8), 1927. doi:10.3390/en17081927
Shi, Z. (2005). Carbon dioxide storage in natural gas reservoir. Oil & gas science and technology 60(3), 527–536. doi:10.2516/ogst:2005035
Siegelman, R. L., Kim, E. J., and Long, J. R. (2021). Porous materials for carbon dioxide separations. Nat. Mater. 20 (8), 1060–1072. doi:10.1038/s41563-021-01054-8
Silva, J. W. L., Santos, M. D., and Oliveira, G. P. (2024). Generalized functionals for qualification of geological carbon storage injection sites. Int. J. Greenh. Gas Control 135, 104167. doi:10.1016/j.ijggc.2024.104167
Silveira, B. H., Costa, H. K., and Santos, E. M. (2023). Bioenergy with carbon capture and storage (BECCS) in Brazil: a review. Energies 16 (4), 2021. doi:10.3390/en16042021
Sinha, S., de Lima, R. P., Lin, Y., Sun, A. Y., Symons, N., Pawar, R., et al. (2020). Normal or abnormal? Machine learning for the leakage detection in carbon sequestration projects using pressure field data. Int. J. Greenh. Gas Control 103, 103189. doi:10.1016/j.ijggc.2020.103189
Sloan, E. D. (2003). Fundamental principles and applications of natural gas hydrates. Nature 426, 353–359. doi:10.1038/nature02135
Smith, L., Billingham, M. A., Lee, C.-H., and Milanovic, D. (2011). Establishing and maintaining the integrity of wells used for sequestration of CO2. Int. J. Greenh. Gas Control 4, 5154–5161. doi:10.1016/j.egypro.2011.02.492
Snaebjörnsdóttir, S. O., Gislason, S. R., Galeczka, I. M., and Oelkers, E. H. (2018). Reaction path modelling of in-situ mineralization of CO2 at the CarbFix site at Hellisheidi, SW. Iceland. Geochim. Cosmochim. Acta 202, 348–366. doi:10.1016/j.gca.2017.09.053
Sodiq, A., Abdullatif, Y., Aissa, B., Ostovar, A., Nassar, N., El-Naas, M., et al. (2023). A review on progress made in direct air capture of CO2. Environ. Technol. and Innovation 29, 102991. doi:10.1016/j.eti.2022.102991
Sokama-Neuyam, Y. A., Aggrey, W. N., Boakye, P., Sarkodie, K., Oduro-Kwarteng, S., and Ursin, J. R. (2022). The effect of temperature on CO2 injectivity in sandstone reservoirs. Sci. Afr. 15, e01066. doi:10.1016/j.sciaf.2021.e01066
Soleimani, R., and Dehaghani, A. H. S. (2024). Unveiling CO2 capture in tailorable green neoteric solvents: an ensemble learning approach informed by quantum chemistry. J. Environ. Manag. 354, 120298. doi:10.1016/j.jenvman.2024.120298
Song, Y., He, H., Yan, Y., Zhai, L., Yao, J., and Wu, B. (2024). A Toolbox for generalized pumped storage power station based on terrain in ArcGIS Environment. Renew. Energy 220, 119590. doi:10.1016/j.renene.2023.119590
Song, Y., and Wang, J. (2021). Optimization of relief well design using artificial neural network during geological CO2 storage in Pohang Basin, South Korea. Appl. Sci. 11, 6996. doi:10.3390/app11156996
Stańczyk, K., Hildebrandt, R., Chećko, J., Urych, T., Wiatowski, M., Masum, S., et al. (2023). CO2 injection via a horizontal well into the coal seam at the experimental mine barbara in Poland. Energies 16, 7217. doi:10.3390/en16207217
Statista (2023). Estimated cost ranges for carbon capture and storage (CSS) and carbon dioxide removal (CDR) solutions as of 2023, by approach or technology. Available at: https://www.statista.com/statistics/1304575/global-carbon-capture-cost-by-technology (Accessed July 30, 2024).
Stein, M. H., Ghotekar, A. L., and Avasthi, S. M. (2010). “CO2 sequestration in a depleted gas field: a material balance study,” in Proceedings of the SPE EUROPEC/EAGE annual conference and exhibition (Barcelona, Spain), 14–17. June 2010.
Su, Z., and Yang, L. (2022). Peak shaving strategy for renewable hybrid system driven by solar and radiative cooling integrating carbon capture and sewage treatment. Renew. Energy 197, 1115–1132. doi:10.1016/j.renene.2022.08.011
Subraveti, S. G., Angel, R. E., Ramírez, A., and Roussanaly, S. (2023). Is carbon capture and storage (CCS) really so expensive? An analysis of cascading costs and CO2 emissions reduction of industrial CCS implementation on the construction of a bridge. Environ. Sci. Technol. 57 (6), 2595–2601. doi:10.1021/acs.est.2c05724
Sullivan, I., Goryachev, A., Digdaya, I. A., Li, X., Atwater, H. A., Vermaas, D. A., et al. (2021). Coupling electrochemical CO2 conversion with CO2 capture. Nat. Catal. 4 (11), 952–958. doi:10.1038/s41929-021-00699-7
Sun, R., Huang, R., Yang, J., and Wang, C. (2021). Magnetic copper smelter slag as heterogeneous catalyst for tetracycline degradation: process variables, kinetics, and characterizations. Chemosphere 285, 131560. doi:10.1016/j.chemosphere.2021.131560
Swift, R. S. (2001). Sequestration of carbon by soil. Soil Sci. 166, 858–871. doi:10.1097/00010694-200111000-00010
Tan, Q., Han, J., and Liu, Y. (2023). Examining the synergistic diffusion process of carbon capture and renewable energy generation technologies under market environment: a multi-agent simulation analysis. Energy 282, 128815. doi:10.1016/j.energy.2023.128815
Tang, L., Ding, G., Song, S., Wang, H., Xie, W., Zhou, Y., et al. (2023). Effect of confining pressure on CO2-brine relative permeability characteristics of sandstone in Ordos Basin. Water 15 (24), 4235. doi:10.3390/w15244235
Tcvetkov, P., Cherepovitsyn, A., and Fedoseev, S. (2019). Public perception of carbon capture and storage: a state-of-the-art overview. Heliyon 5 (12), e02845. doi:10.1016/j.heliyon.2019.e02845
Thanh, H. V., Sugai, Y., Nguele, R., and Sasaki, K. (2019). Integrated workflow in 3D geological model construction for evaluation of CO2 storage capacity of a fractured basement reservoir in Cuu Long Basin, Vietnam. Int. J. Greenh. Gas Control 90, 102826. doi:10.1016/j.ijggc.2019.102826
Thanh, H. V., Zhang, H., Rahimi, M., Ashraf, U., Migdady, H., Daoud, M. S., et al. (2024). Enhancing carbon sequestration: innovative models for wettability dynamics in CO2-brine-mineral systems. J. Environ. Chem. Eng. 12 (5), 113435. doi:10.1016/j.jece.2024.113435
Thonemann, N., Zacharopoulos, L., Fromme, F., and Nuhlen, J. (2022). Environmental impacts of carbon capture and utilization by mineral carbonation: a systematic literature review and meta life cycle assessment. J. Clean. Prod. 332, 130067. doi:10.1016/j.jclepro.2021.130067
Tillero, E. (2024). Machine learning-based modelling for geologic CO2 storage in deep saline aquifers. Case study of bunter sandstone in Southern North Sea. Int. J. Greenh. Gas Control 133, 104077. doi:10.1016/j.ijggc.2024.104077
Torsæter, M., Todorovic, J., Lavrov, A., Gawel, K., Lund, H., Roy, P., et al. (2017). Avoiding damage of CO2 injection wells caused by temperature variations. Int. J. Greenh. Gas Control 114, 5275–5286. doi:10.1016/j.egypro.2017.03.1645
Turrell, W. R., Berx, B., Bresnan, E., León, P., Rouse, S., Webster, L., et al. (2022). A review of national monitoring requirements to support offshore carbon capture and storage. Front. Mar. Sci. 9, 838309. doi:10.3389/fmars.2022.838309
Turvey, C. C., Wilson, S., Hamilton, J. L., Tait, A. W., McCutcheon, J., Beinlich, A., et al. (2018). Hydrotalcites and hydrated Mg-carbonates as carbon sinks in serpentinite mineral wastes from the Woodsreef chrysotile mine, New South Wales, Australia: controls on carbonate mineralogy and efficiency of CO2 air capture in mine tailings. Int. J. Greenh. Gas Control 79, 38–60. doi:10.1016/j.ijggc.2018.09.015
Twerda, A., Belfroid, S., and Neele, F. (2018). “CO2 injection in low pressure depleted reservoirs,” in Fifth CO2 geological storage workshop (European Association of Geoscientists and Engineers), 1–4.
Udebhulu, O. D., Aladeitan, Y., Azevedo, R. C., and De Tomi, G. (2024). A review of cement sheath integrity evaluation techniques for carbon dioxide storage. J. Petroleum Explor. Prod. Technol. 14 (1), 1–23. doi:10.1007/s13202-023-01697-0
Vakilifard, N., Kantzas, E. P., Edwards, N. R., Holden, P. B., and Beerling, D. J. (2021). The role of enhanced rock weathering deployment with agriculture in limiting future warming and protecting coral reefs. Environ. Res. Lett. 16, 094005. doi:10.1088/1748-9326/ac1818
van Bergen, F., Krzystolik, P., van Wageningen, N., Pagnier, H., Jura, B., Skiba, J., et al. (2009). Production of gas from coal seams in the Upper Silesian Coal Basin in Poland in the post-injection period of an ECBM pilot site. Int. J. Coal Geol. 77 (1-2), 175–187. doi:10.1016/j.coal.2008.08.011
Vasco, D. W., Bissell, R. C., Bohloli, B., Daley, T. M., Ferretti, A., Foxall, W., et al. (2018). “Monitoring and modeling caprock integrity at the in Salah carbon dioxide storage site, Algeria,” in Geological carbon storage: subsurface seals and caprock integrity, 243–269.
Vasco, D. W., Timothy, H., Dixon, T. H., Ferretti, A., and Samsonov, S. V. (2020). Monitoring the fate of injected CO2 using geodetic techniques. Lead. Edge 39 (1), 29–37. doi:10.1190/tle39010029.1
Vasilev, Y., Vasileva, P., and Tsvetkova, A. (2019). International review of public perception of CCS technologies. Sect. Ecol. Environ. Prot. 19 (5.1), 415–422. doi:10.5593/sgem2019/5.1/S20.052
Vercelli, S., Anderluccia, J., Memolib, R., Battistia, N., Mabonc, L., and Lombardi, S. (2013). Informing people about CCS: a review of social research studies. Energy Procedia 37, 7464–7473. doi:10.1016/j.egypro.2013.06.690
Verdon, J. P., Kendall, J.-M., Stork, A. L., Chadwick, R. A., White, D. J., and Bissell, R. C. (2013). Comparison of geomechanical deformation induced by megatonne-scale CO2 storage at Sleipner, Weyburn, and in Salah. Proc. Natl. Acad. Sci. 110, E2762–E2771. doi:10.1073/pnas.1302156110
Vermeul, V. R., Amonette, J. E., Strickland, C. E., Williams, M. D., and Bonneville, A. (2016). An overview of the monitoring program design for the FutureGen 2.0 CO2 storage site. Int. J. Greenh. Gas Control 51, 193–206. doi:10.1016/j.ijggc.2016.05.023
Vlarrasa, V., Carrera, J., Olivella, S., Rutqvist, J., and Laloui, L. (2019). Induced seismicity in geologic carbon storage. Solid earth. 10, 871–892. doi:10.5194/se-10-871-2019
Vilarrasa, V., Olivella, S., Carrera, J., and Rutqvist, J. (2014). Long-term impacts of cold CO2 injection on the caprock integrity. Int. J. Greenh. Gas Control 24, 1–13. doi:10.1016/j.ijggc.2014.02.016
Vinca, A., Emmerling, J., and Tavoni, M. (2018). Bearing the cost of stored carbon leakage. Front. Energy Res. 6. doi:10.3389/fenrg.2018.00040
Vishal, V., and Singh, T. (2016). Geologic carbon sequestration. Environ. Geosci. 16, 47. doi:10.1007/978-3-319-27019-7
Vrålstad, T., Saasen, A., Fjær, E., Øia, T., Ytrehus, J. D., and Khalifeh, M. (2019). Plug and abandonment of offshore wells: ensuring long-term well integrity and cost-efficiency. J. Petroleum Sci. Eng. 173, 478–491. doi:10.1016/j.petrol.2018.10.049
Waarum, I.-K., Blomberg, A. E. A., Eek, E., Brown, J., Ulfsnes, A., Carpenter, M., et al. (2017). CCS leakage detection technology - industry needs, government regulations, and sensor performance. Greenh. Gas. Control Technol. 114, 3613–3627. doi:10.1016/j.egypro.2017.03.1493
Waele, J. D. (2017). Karst processes and landforms. University of Italy. International Encyclopaedia of Geography. John Wiley and Sons. Ltd.
Wall, T. F. (2007). Combustion processes for carbon capture. Proc. Combust. Inst. 31 (1), 31–47. doi:10.1016/j.proci.2006.08.123
Wang, F., Harindintwali, J. D., Yuan, Z., Wang, M., Wang, F., Li, S., et al. (2021). Technologies and perspectives for achieving carbon neutrality. The Innovation 2 (4), 100180. doi:10.1016/j.xinn.2021.100180
Wang, X., and Song, C. (2020). Carbon capture from flue gas and the atmosphere: a perspective. Front. Energy Res. 8, 560849. doi:10.3389/fenrg.2020.560849
Wang, Y., Lei, Z., Xu, Z., Liu, Y., Pan, X., Wang, Y., et al. (2024a). A compositional numerical study of vapor–liquid-adsorbed three-phase equilibrium calculation in a hydraulically fractured shale oil reservoir. Phys. Fluids 36 (7). doi:10.1063/5.0214453
Wang, Y. Y., Wang, X. G., Dong, R. C., Teng, W. C., Zhan, S. Y., Zeng, G. Y., et al. (2023). Reservoir heterogeneity controls of CO2-EOR and storage potentials in residual oil zones: insights from numerical simulations. Petroleum Sci. 20 (5), 2879–2891. doi:10.1016/j.petsci.2023.03.023
Wang, Y. Z., Cao, R. Y., Jia, Z. H., Wang, B. Y., Ma, M., and Cheng, L. S. (2024b). A multi-mechanism numerical simulation model for CO2-EOR and storage in fractured shale oil reservoirs. Petroleum Sci. 21, 1814–1828. doi:10.1016/j.petsci.2024.02.006
Wen, G., Li, Z., Long, Q., Azizzadenesheli, K., Anandkumar, A., and Benson, S. M. (2023). Real-time high-resolution CO2 geological storage prediction using nested Fourier neural operators. Energy and Environ. Sci. 16 (4), 1732–1741. doi:10.1039/d2ee04204e
White, J. W., and Foxall, W. (2016). Assessing induced seismicity risk at CO2 storage projects: recent progress and remaining challenges. Int. J. Greenh. Gas Control 49, 413–424. doi:10.1016/j.ijggc.2016.03.021
White, S. K., Spane, F. A., Schaef, H. T., Miller, Q. R. S., White, M. D., Horner, J. A., et al. (2020). Quantification of CO2 mineralization at the wallula basalt pilot project. Environ. Sci. Technol. 54 (22), 14609–14616. doi:10.1021/acs.est.0c05142
Whittaker, S. G., and Rostron, B. (2003). “Geologic storage of CO2 in a carbonate reservoir within the Williston Basin, Canada: an update,” in Greenhouse gas control technologies-6th international conference (Pergamon), 385–390.
Wilkinson, M., Mouli-Castillo, J., Morgan, P., and Eid, R. (2017). Time-lapse gravity surveying as a monitoring tool for CO2 storage. Int. J. Greenh. Gas Control 60, 93–99. doi:10.1016/j.ijggc.2017.03.006
Williams, G. A., and Chadwick, R. A. (2021). Influence of reservoir-scale heterogeneities on the growth, evolution and migration of a CO2 plume at the Sleipner Field, Norwegian North Sea. Int. J. Greenh. Gas Control 106, 103260. doi:10.1016/j.ijggc.2021.103260
Winnefeld, F., Leeman, A., German, A., and Lothenbach, B. (2022). CO2 storage in cement and concrete by mineral carbonation. Current Opinion in Green and Sustainable Chemistry 38, 100672. doi:10.1016/j.cogsc.2022.100672
Witte, P. A., Konuk, T., Skjetne, E., and Chandra, R. (2023). Fast CO2 saturation simulations on large-scale geomodels with artificial intelligence-based Wavelet Neural Operators. Int. J. Greenh. Gas Control 126, 103880. doi:10.1016/j.ijggc.2023.103880
Wong, S., Law, D., Deng, X., Robinson, J., Kadatz, B., Gunter, W. D., et al. (2007). Enhanced coalbed methane and CO2 storage in anthracitic coals—micro-pilot test at South Qinshui, Shanxi, China. Int. J. Greenh. Gas Control 1 (2), 215–222. doi:10.1016/s1750-5836(06)00005-3
Wong, S., Macdonald, D., Andrei, S., Gunter, W. D., Deng, X., Law, D., et al. (2010). Conceptual economics of full scale enhanced coalbed methane production and CO2 storage in anthracitic coals at South Qinshui basin, Shanxi, China. Int. J. Coal Geol. 82 (3-4), 280–286. doi:10.1016/j.coal.2010.01.011
Wu, H., Lubbers, N., Viswanathan, H. S., and Pollyea, R. M. (2021). A multi-dimensional parametric study of variability in multi-phase flow dynamics during geologic CO2 sequestration accelerated with machine learning. Appl. Energy 287, 116580. doi:10.1016/j.apenergy.2021.116580
Wu, Q., and Li, C. (2023). Modeling and operation optimization of hydrogen-based integrated energy system with refined power-to-gas and carbon-capture-storage technologies under carbon trading. Energy 270, 126832. doi:10.1016/j.energy.2023.126832
Wu, X., Shen, J., Wang, M., and Lee, K. Y. (2020). Intelligent predictive control of large-scale solvent-based CO2 capture plant using artificial neural network and particle swarm optimization. Energy 196, 117070. doi:10.1016/j.energy.2020.117070
Wu, Y., and Li, P. (2020). The potential of coupled carbon storage and geothermal extraction in a CO2-enhanced geothermal system: a review. Geotherm. Energy 8 (1), 19. doi:10.1186/s40517-020-00173-w
Wu, Y., Zhang, H., Wang, S., and Zhen, L. (2023). Mathematical optimization of carbon storage and transport problem for carbon capture, use, and storage chain. Mathematics 11 (12), 2765. doi:10.3390/math11122765
Wu, M., Qin, Y., Zhang, Y., Zhu, S., Zhang, G., Lan, F., et al. (2004). Influence factors and feasibility evaluation on geological sequestration of CO2 in coal seams: a review. ACS omega 8 (19), 16561–16569. doi:10.1021/acsomega.3c01148
Xie, J., Yang, X., Qiao, W., Peng, S., Yue, Y., Chen, Q., et al. (2023). Investigations on CO2 migration and flow characteristics in sandstone during geological storage based on laboratory injection experiment and CFD simulation. Gas Sci. Eng. 117, 205058. doi:10.1016/j.jgsce.2023.205058
Yan, L. (2024). Advancements in controlled source electromagnetic methods for prospecting unconventional hydrocarbon resources in China. Surv. Geophys. 45, 239–276. doi:10.1007/s10712-023-09808-6
Yan, Y., Borhani, T. N., Subraveti, S. G., Pai, K. N., Prasad, V., Rajendran, A., et al. (2021). Harnessing the power of machine learning for carbon capture, utilisation, and storage (CCUS)–a state-of-the-art review. Energy Environ. Sci. 14 (12), 6122–6157. doi:10.1039/d1ee02395k
Yang, X., Buscheck, T. A., Mansoor, K., Wang, Z., Gao, K., Huang, L., et al. (2019). Assessment of geophysical monitoring methods for detection of brine and CO2 leakage in drinking water aquifers. Int. J. Greenh. Gas Control 90, 102803. doi:10.1016/j.ijggc.2019.102803
Yao, P., Yu, Z., Zhang, Y., and Xu, T. (2023). Application of machine learning in carbon capture and storage: an in-depth insight from the perspective of geoscience. Fuel 333, 126296. doi:10.1016/j.fuel.2022.126296
Yilo, N. K., Weitemeyer, K., Minshull, T. A., Attias, E., Marin-Moreno, H., Falcon-Suarez, I. H., et al. (2024). Marine CSEM synthetic study to assess the detection of CO2 escape and saturation changes within a submarine chimney connected to a CO2 storage site. Geophysical Journal International 236 (1), 183–206.
Yong, J. K., Stevens, G. W., Caruso, F., and Kentish, S. E. (2015). The use of carbonic anhydrase to accelerate carbon dioxide capture processes. J. Chem. Technol. and Biotechnol. 90 (1), 3–10. doi:10.1002/jctb.4502
You, J., Ampomah, W., Morgan, A., Sun, Q., and Huang, X. (2021). A comprehensive techno-eco-assessment of CO2 enhanced oil recovery projects using a machine-learning assisted workflow. Int. J. Greenh. Gas Control 111, 103480. doi:10.1016/j.ijggc.2021.103480
Young, M. (2012). Cranfield large scale CO2 injection--monitoring 3.5 million tons (No. DOE-SSEB-FC26-05NT42590-9). Peachtree Corners, GA United States: Southern States Energy Board.
Youns, Y. T., Manshad, A. K., and Ali, J. A. (2023). Sustainable aspects behind the application of nanotechnology in CO2 sequestration. Fuel 349, 128680. doi:10.1016/j.fuel.2023.128680
Yousef, A. M., El-Maghlany, W. M., Eldrainy, Y. A., and Attia, A. (2018). New approach for biogas purification using cryogenic separation and distillation process for CO2 capture. Energy 156, 328–351. doi:10.1016/j.energy.2018.05.106
Zaidi, S., Srivastava, N., and Khare, S. K. (2022). Microbial carbonic anhydrase mediated carbon capture, sequestration and utilization: a sustainable approach to delivering bio renewables. Bioresour. Technol. 365, 128174. doi:10.1016/j.biortech.2022.128174
Zajac, M., Skocek, J., Ben Haha, M., and Deja, J. (2022). CO2 mineralization methods in cement and concrete industry. Energies 15, 3597. doi:10.3390/en15103597
Zanjani, N. G., Moghaddam, A. Z., Nazari, K., and Taheri, M. M. (2012). Increasing the Storage capacity and selectivity in the formation of Natural gas hydrates using porous media. Chem. Eng. Technol. 35 (11), 1973–1980. doi:10.1002/ceat.201200089
Zeidouni, M., Tran, N. H., and Munawar, M. D. (2017). Interpretation of above-zone pressure influence time to characterize CO2 leakage. Greenh. Gases Sci. Technol. 7 (6), 1050–1064. doi:10.1002/ghg.1698
Zhang, H. (2021). Regulations for carbon capture, utilization and storage: comparative analysis of development in Europe, China and the Middle East. Resour. Conservation Recycl. 173, 105722. doi:10.1016/j.resconrec.2021.105722
Zhang, L., Ren, S., Ren, B., Zhang, W., Guo, Q., and Zhang, L. (2011). Assessment of CO2 storage capacity in oil reservoirs associated with large lateral/underlying aquifers: case studies from China. Int. J. Greenh. Gas Control 5 (4), 1016–1021. doi:10.1016/j.ijggc.2011.02.004
Zhang, L., Yang, L., Wang, J., Zhao, J., Dong, H., Yang, M., et al. (2017). Enhanced CH4 recovery and CO2 storage via thermal stimulation in the CH4/CO2 replacement of methane hydrate. Chem. Eng. J. 308, 40–49. doi:10.1016/j.cej.2016.09.047
Zhang, L. S., Zhou, S., Wang, L., Wang, L., and Li, J. (2013). Surfactant surface tension effects on promoting hydrate formation: an experimental study Fluorocarbon surfactant (Intechem-01) +SDS composite surfactant. J. Environ. Prot. 4, 42–48. doi:10.4236/jep.2013.45A005
Zhang, S., and DePaolo, D. L. (2017). Rates of CO2 mineralization in geological carbon storage. Accounts Chem. Res. 50, 2075–2084. doi:10.1021/acs.accounts.7b00334
Zhang, T., Shen, Z., He, L., Shen, W., and Li, W. (2022b). Strain field features and three-dimensional crustal deformations constrained by dense GRACE and GPS measurements in NE tibet. Remote Sens. 14, 2638. doi:10.3390/rs14112638
Zhang, Y., Cheng, V., Mallapragada, D. S., Song, J., and He, G. (2022a). A model-adaptive clustering-based time aggregation method for low-carbon energy system optimization. IEEE Trans. Sustain. Energy 14 (1), 55–64. doi:10.1109/tste.2022.3199571
Zhang, Y., Liu, J., Chen, L., Yang, Y., and Wang, Z. (2019). Chemical-looping combustion of coal with Fe2O3 and Mn3O4 as oxygen carriers: a comparative study. Fuel Process. Technol. 188, 40–49. doi:10.1016/j.applthermaleng.2019.113813
Zhang, Z., Pan, S. Y., Li, H., Cai, J., Olabi, A. G., Antony, E. L., et al. (2020). Recent advances in carbon dioxide utilization. Renew. Sustain. Energy Rev. 125, 109799. doi:10.1016/j.rser.2020.109799
Zhao, K., Jia, C., Li, Z., Du, X., Wang, Y., Li, J., et al. (2023). Recent advances and future perspectives in carbon capture, transportation, utilization, and storage (cctus) technologies: a comprehensive review. Fuel 351, 128913. doi:10.1016/j.fuel.2023.128913
Zhao, X., Deng, H., Wang, W., Han, F., Li, C., Zhang, H., et al. (2017). Impact of naturally leaking carbon dioxide on soil properties and ecosystems in the Qinghai-Tibet plateau. Sci. Rep. 7 (3001), 3001. doi:10.1038/s41598-017-02500-x
Zheng, J., Zhang, P., and Linga, P. (2017). Semi clathrate hydrate process for pre-combustion capture of CO2 at near ambient temperatures. Appl. Energy 194, 267–278. doi:10.1016/j.apenergy.2016.10.118
Zheng, J., Chong, Z. R., Qureshi, M. F., and Linga, P. (2020). Carbon dioxide sequestration via gas hydrates: A potential pathway toward decarbonization. Energy Fuels 34 (9), 10529–10546. doi:10.1021/acs.energyfuels.0c02309
Zhong, Z., Sun, A. Y., Yang, Q., and Ouyan, Q. (2019). A deep learning approach to anomaly detection in geological carbon sequestration sites using pressure measurements. J. Hydrology 573, 885–894. doi:10.1016/j.jhydrol.2019.04.015
Zhou, Q., Birkholzer, J. T., Tsang, C. F., and Rutqvist, J. (2008). A method for quick assessment of CO2 storage capacity in closed and semi-closed saline formations. Int. J. Greenh. gas control 2 (4), 626–639. doi:10.1016/j.ijggc.2008.02.004
Zhou, X., Liang, D. Q., Liang, S., Yi, L. Z., and Lin, F. H. (2015). Recovering CH4 from natural gas hydrates with the injection of CO2-N2 gas mixtures. Energy and Fuels 29, 1099–1106. doi:10.1021/ef5025824
Zhou, Z., Lin, Y., Wu, Y., Wang, Z., Dilmore, R., and Guthrie, G. (2018). “Spatial-temporal densely connected convolutional networks: an application to CO2 leakage detection,” in Paper presented at the 2018 SEG international exposition and annual meeting (California, USA: Anaheim). October 2018.
Zhou, Z., Lin, Y., Wu, Y., Wang, Z., Dilmore, R., Guthrie, G., et al. (2019). A data-driven CO2 leakage detection using seismic data and spatial–temporal densely connected convolutional neural networks. Int. J. Greenh. Gas Control 90, 102790. doi:10.1016/j.ijggc.2019.102790
Zhu, X., Xie, W., Wu, J., Miao, Y., Xiang, C., Chen, C., et al. (2022). Recent advances in direct air capture by adsorption. R. Chem. Soc. Rev. 51 (15), 6574–6651. doi:10.1039/d1cs00970b
Keywords: carbon dioxide capture, carbon dioxide sequestration, numerical and simulation approach, geological storage, monitoring CO2 leakage
Citation: Ndlovu P, Bulannga R and Mguni LL (2024) Progress in carbon dioxide capture, storage and monitoring in geological landform. Front. Energy Res. 12:1450991. doi: 10.3389/fenrg.2024.1450991
Received: 18 June 2024; Accepted: 30 August 2024;
Published: 15 October 2024.
Edited by:
Federica Raganati, National Research Council (CNR), ItalyReviewed by:
Sina Rezaei Gomari, Teesside University, United KingdomXinyuan Gao, China University of Petroleum, Beijing, China
Benmadi Milad, University of Oklahoma, United States
Copyright © 2024 Ndlovu, Bulannga and Mguni. This is an open-access article distributed under the terms of the Creative Commons Attribution License (CC BY). The use, distribution or reproduction in other forums is permitted, provided the original author(s) and the copyright owner(s) are credited and that the original publication in this journal is cited, in accordance with accepted academic practice. No use, distribution or reproduction is permitted which does not comply with these terms.
*Correspondence: Phakamile Ndlovu, cGhha2FtaWxlbmRsb3Z1QGdtYWlsLmNvbQ==