- Water Technologies Innovation Institute and Research Advancement, Jubail, Saudi Arabia
This study offers a thorough techno-economic evaluation of a hybrid desalination system merging Pressure Retarded Osmosis (PRO) with Seawater Reverse Osmosis (SWRO) process. The primary aim is to determine the feasibility and economic viability of the hybridized approach to conventional SWRO methods. Diverse scenarios are studied based on parameters such as PRO module costs, energy savings achievable and payback period. Our findings reveal the potential for energy savings through the PRO-SWRO hybrid system. For instance, in scenarios where PRO membrane costs are low, such as $450 per element, and electricity prices are relatively high at $0.12 per kilowatt-hour, energy savings of up to 7% are attainable compared to standalone SWRO setups. This potential could be amplified with increasing salinity levels in the feed solution and higher draw solution pressures. The study extends beyond theoretical analysis, offering practical solutions for the design and implementation of sustainable desalination solutions; by studying the interaction between various parameters and their impact; on both energy consumption and economic viability. The current study estimates the membrane break-even costs of a PRO-SWRO hybrid system by estimating and equating the Net Present Value (NPV) of the SWRO system (base configuration) with the NPV of the PRO-SWRO hybrid. This research lays a solid foundation for the development of hybrid desalination systems capable of addressing water scarcity challenges in a cost-effective and environmentally sustainable manner.
Introduction
The escalating global demand for freshwater and diminishing natural freshwater resources have prompted the exploration of innovative water desalination technologies. The need for efficient and sustainable desalination methods has never been more critical. As global populations grow and climate change exacerbates water scarcity, innovative solutions are essential to ensure a reliable supply of potable water. Seawater Reverse Osmosis (SWRO) has become the cornerstone of modern desalination, known for its ability to produce high-quality fresh water from seawater. However, the process is energy-intensive, with significant operational costs associated with high-pressure pumps and energy recovery devices (Altaee et al., 2014).
One promising approach is the hybridization of Pressure Retarded Osmosis (PRO) and Seawater Reverse Osmosis (SWRO) systems. This hybrid system aims to enhance the overall efficiency and sustainability of desalination processes by leveraging the strengths of both technologies.
PRO is an emerging technology that harnesses the osmotic pressure difference between two solutions of varying salinity to generate energy. In the context of desalination, PRO can be integrated with SWRO to improve energy recovery and reduce the overall energy consumption of the desalination process. The PRO-SWRO hybrid system capitalizes on the osmotic energy available from the brine concentrate produced by the SWRO process, which is typically discarded as waste (Altaee et al., 2014). By converting this waste brine into a source of renewable energy, the PRO system can significantly lower the energy requirements of the SWRO process, making the overall desalination process more sustainable and cost-effective (She et al., 2012).
Membrane development has been a focal point for both SWRO and PRO technologies. The efficiency and viability of these processes heavily depend on membrane properties such as permeability, selectivity, mechanical strength, and resistance to fouling. State-of-the-art thin-film composite membranes have considerable improvements in these areas, yet challenges remain (Yip and Elimelech, 2012).
The integration of PRO and SWRO systems presents several technical and economic challenges. Technologically, the development of membranes with high water permeability and selectivity is crucial for the efficient operation of both PRO and SWRO processes. Advances in membrane technology, such as the development of thin-film composite membranes, have shown promise in improving the performance and durability of these systems (Loeb, 1976). However, further research and development are needed to optimize membrane properties and address issues related to fouling and scaling, which can significantly impact the efficiency and lifespan of the membranes (Yip and Elimelech, 2012). (Rahman et al., 2023) discuss the latest developments in the PRO membrane covering the types of membranes, the materials used in the membranes and the possible limitations (Ruiz-Garcia et al., 2023). found that the water permeability coefficient (A) had a larger impact on the amount of energy generated than the salt permeability coefficient (B) and suggested that PRO membrane manufacturers should focus on increasing A, even if the value of B increases.
Economically, the feasibility of the PRO-SWRO hybrid system depends on various factors, including capital costs, operational and maintenance expenses, and the potential for energy savings. While the initial investment in hybrid systems can be substantial, the long-term benefits, such as reduced energy consumption and lower operational costs, can offset these initial expenditures. A comprehensive techno-economic analysis is essential to evaluate the cost-effectiveness of the PRO-SWRO hybrid system and identify the conditions under which it can be a viable alternative to conventional desalination technologies (Helfer et al., 2014).
Furthermore, the implementation of PRO-SWRO hybrid systems requires careful consideration of site-specific factors, such as the availability of seawater and freshwater sources, local energy costs, and environmental regulations. The adaptability of the hybrid system to different geographic and climatic conditions is a critical factor in its widespread adoption. Additionally, policy and regulatory frameworks play a significant role in promoting the development and deployment of advanced desalination technologies. Government incentives, subsidies, and supportive policies can encourage investment in PRO-SWRO hybrid systems and facilitate their integration into existing water management infrastructures (McGinnis et al., 2007).
The PRO-SWRO hybrid system represents a promising advancement in desalination technology, offering potential benefits in terms of energy efficiency and sustainability. However, realizing its full potential requires addressing technical challenges related to membrane development, optimizing economic factors, and navigating regulatory landscapes. Through continued research and innovation, the PRO-SWRO hybrid system can contribute to meeting the growing global demand for freshwater in an environmentally and economically sustainable manner.
The hybrid system typically consists of a SWRO unit that produces fresh water and a high-salinity brine stream, which is then directed to the PRO unit. In the PRO unit, this brine is brought into contact with a low-salinity feed solution, usually seawater or wastewater, across a semipermeable membrane (Cath et al., 2006; Thorsen and Holt, 2009).
The osmotic pressure difference drives water from the low salinity side to the high salinity side, generating hydraulic pressure that can be converted into mechanical energy. This energy can then be used to assist the SWRO process, reducing the overall energy demand. Key components of the system include high-performance membranes, energy recovery devices, pressure exchangers, and control systems to optimize flow rates and pressure conditions (Achilli et al., 2009; Lutchmiah et al., 2014).
The design and optimization of such hybrid systems require a thorough understanding of the thermodynamics and kinetics of osmotic processes. Computational models and simulations are often employed to predict system performance under various operating conditions. These models help in identifying the optimal configuration and operational parameters that maximize energy efficiency and water recovery rates (Ramon et al., 2011; Liang, 2023).
The potential of PRO systems in conjunction with desalination processes is also well-studied. For example, investigating the integration of PRO with reverse osmosis, highlights the synergies that can be achieved for improved desalination efficiency (Ruiz-García, 2024). A 2-year pilot plant study results of a SWRO-PRO hybrid system of 240 m3/d capacity and their results showed that PRO reduced the energy consumption of the SWRO system by nearly 20% when using seawater as the Draw Solution (DS) and wastewater as the Feed Solution (FS) (Ruiz-García et al., 2022). Further studies have examined the environmental and economic benefits of integrating PRO with the existing reverse osmosis system, providing a comprehensive overview of the feasibility and advantages of such integration (Matsuyama et al., 2021; Al-Zainati et al., 2022). A 2-year pilot plant study of a SWRO-PRO hybrid system with a capacity of 240 m³/day demonstrated that using seawater as the Draw Solution (DS) and wastewater as the Feed Solution (FS) reduced the energy consumption of the SWRO system by nearly 20% (Ruiz-García et al., 2022). Further research has explored the environmental and economic benefits of integrating PRO with existing reverse osmosis systems, offering a comprehensive overview of the feasibility and advantages of such integration (Matsuyama et al., 2021; Al-Zainati et al., 2022).
Additionally, recent advancements in membrane technology and system design have been reported. A study in focuses on innovative approaches to improve the energy recovery and overall performance of PRO systems, offering new perspectives on optimizing system design (Jiao et al., 2022; Ruiz-Garcia et al., 2023). Moreover, ongoing research continues to address the challenges related to fouling and scaling in PRO membranes, as discussed in several recent publications (Bharadwaj et al., 2016; Huang et al., 2023).
An experiment was conducted using treated wastewater and seawater using a 10-inch module from TOYOBO. They reported the cost of power generation and also how much power density is required to realize a commercially feasible power plant (Matsuyama et al., 2021).
Overall, these studies collectively underscore the significant progress made in the development and optimization of PRO systems. They provide a solid foundation for further research and development, aimed at overcoming current limitations and fully realizing the potential of PRO technology for sustainable energy and water resource management.
In this study, brine (BR) from a Seawater Reverse Osmosis (SWRO) system was considered as the DS, and seawater (SW) was considered as the FS, henceforth referred to as the (SW, BR) case unless otherwise mentioned. However, the current study aims not to generate electricity but to use the mechanical energy produced by the PRO system as a direct pumping energy provider for the high-pressure pump in the SWRO system. By this approach the energy transfer losses would be minimized since the osmotic pressure is directly used as mechanical energy directly without converting it into electricity.
Materials and methods
A bench scale test was performed using TOYOBO’s FP5230S3SI hollow fibre cellulose tri-acetate (CTA) 5-inch membrane module. The surface area of the membranes is 60 m2. The optimum pressure and the corresponding dilution were found for the (SW, BR) case (Figure 1). The findings were considered for a SWRO plant configuration producing permeate at a capacity of 2,000 m3/d.
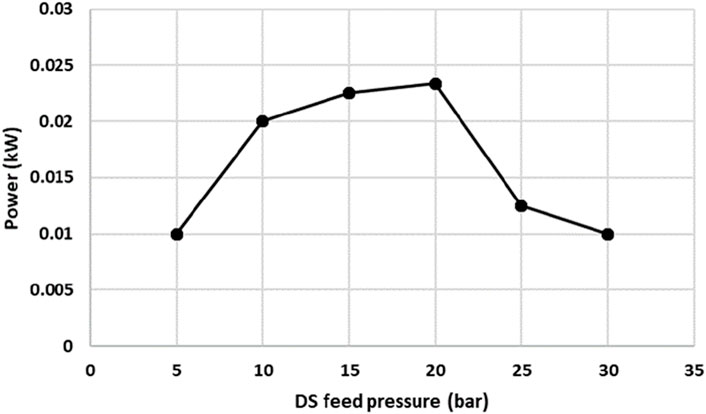
Figure 1. Optimization of DS feed pressure where 20 bar DS feed pressure shows the highest power generation.
Optimizing a PRO system involves balancing the osmotic pressure of the draw solution and the hydraulic pressure to optimize power generation. The system also accounted for the structural integrity of the membrane and other components under varying pressures. In summary, as the draw solution pressure increases, the power generation potential initially rises, reaches an optimal point, and then declines. The key is to find the pressure that maximizes the power output, considering the trade-off between water flux and pressure.
At low pressure (0 to a few bars), the water flux is high, leading to a rapid increase in hydraulic pressure. The potential for power generation is substantial due to the significant volume of water flowing through the membrane. Whereas in the moderate pressure range (15–20 bars), the water flux starts to decrease as the hydraulic pressure builds up, but there is still a considerable flow contributing to power generation.
Finally, at high pressure water flux diminishes as the hydraulic pressure nears the osmotic pressure difference. The power generation potential decreases because the driving force for water movement is reduced. These results matched well with previous studies (Al-Zainati et al., 2022).
The net power generated and the power consumed by the PRO system are calculated (Liang, 2023) using Equation 1.
Where α is an efficiency factor,
The optimization results were used to compare the PRO-SWRO hybrid configuration against a standalone SWRO configuration. The standalone SWRO configuration will be referred to as the base case from now on unless otherwise mentioned.
SWRO base configuration
The base configuration consists of a standard RO plant: a booster pump, a high-pressure pump and an Energy Recovery Device (ERD). Pre-treated seawater from a pre-treated seawater tank at 1 bar is pumped to the suction side of a high-pressure pump at 2.5 bar. The high-pressure pump pumps the seawater from 2.5 bar to 39 bar, which is further pressurized in an ERD device to 68 bar.
The salinity of the seawater was considered as 42,000 ppm and that of the brine was taken as 70,000 ppm. The temperature of all the streams was considered to be 35°C. The base configuration adopted is shown in Figure 2. The recovery of the system was taken to be 40% with 2,000 m3/d permeate and 3,000 m3/d brine produced. The processes bounded by the red dashed line were considered to be unchanged in the base case configuration and the PRO-SWRO hybrid configuration.
Hybrid SWRO-PRO configuration
Hybrid system consists of the SWRO system from the base case integrated with the PRO system. The ERD and the SWRO system are assumed to remain unchanged, with the same amount of permeate produced as in the base case. The processes enclosed by the red dashed line remain the same in the base case and the hybrid case. Furthermore, it is assumed that the pre-treatment configuration remains the same with the same quality of feed water supplied to the SWRO system as in the base case.
The energy gained in the PRO system due to the dilution of the DS is exchanged with the feed water to increase the head of the feed before the high-pressure pump. The pressure exchanged in the pressure exchanger (PX) can be estimated using the following formula (Ruiz-García, 2024) given in Equation 2 (Banchik and Lienhard 2012).
Where,
Techno-economic analysis
The cost of PRO membranes and their lifetime/replacement time are two variables that are not readily available, as PRO is not fully commercialized yet. Previous techno-economic studies estimated the commercial feasibility of PRO using metrics such as the power density, Levelized Cost of Electricity (LCoE), and the Levelized Cost of Water (LCoW). However, the current study estimates the membrane break-even costs of a PRO-SWRO hybrid system by estimating and equating the Net Present Value (NPV) of the SWRO system (base configuration) with the NPV of the PRO-SWRO hybrid. It has been assumed that the pre-treatment, ERD and SWRO membrane system remains the same in the SWRO base configuration and the PRO-SWRO hybrid configuration (see the area enclosed by the red dashed lines in Figures 2, 3). Capital expenditures (CAPEX) of the SWRO base configuration only includes the high-pressure pump and the booster pump, and the OPEX includes the power consumed by the high-pressure pump and the booster pump.
In the PRO-SWRO hybrid case, CAPEX includes the cost of PRO modules, the SWRO high-pressure pump, the pumps needed for pumping the DS and FS solutions, and the ERD. As the brine from SWRO is used as DS and the pre-treated seawater is used as FS, pre-treatment costs were not considered. The pumping power needed by the PRO DS pump, the PRO FS pump, and the high-pressure pump of SWRO, as well as the replacement of PRO membranes were considered as a part of the OPEX.
The Net Present Value (NPV) of the PRO-SWRO configuration and the SWRO base configuration was equated to determine the breakeven PRO module cost at different lifetimes of the PRO modules. The breakeven cost represents the upper limit on the cost and any PRO module cost above it would make the PRO-SWRO configuration infeasible when compared against the SWRO base configuration. However, any reduction in the PRO module cost to a value below the breakeven cost would render the PRO-SWRO configuration feasible, as savings would be realized over the life of the project.
The Net Present Value (NPV) was calculated as follows using Equation 3 and Equation 4
Where,
Calculation process flow chart
Figure 4 shows the steps involved in calculating the PRO membrane cost at the breakeven NPV value of the SWRO base case and the PRO-SWRO hybrid case. Any cost below the breakeven PRO membrane cost makes the PRO-SWRO hybrid case feasible and competitive against the SWRO base case. When the costs of the PRO membranes become quite low, the payback period also becomes small.
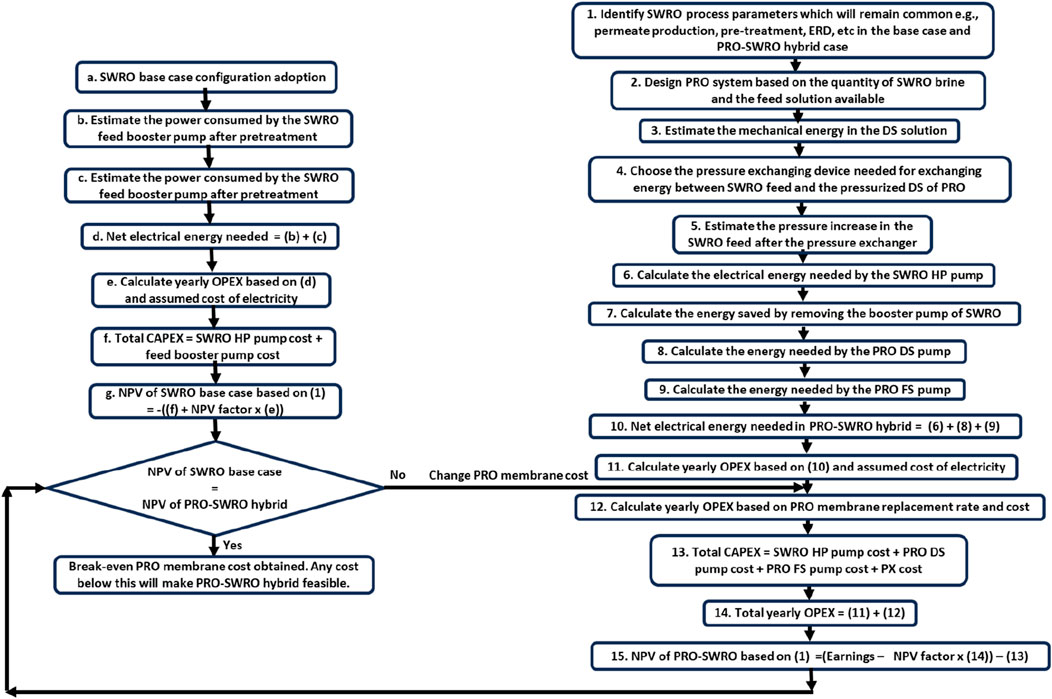
Figure 4. Calculation flow chart showing how the NPV of the SWRO base case and the PRO-SWRO hybrid case was calculated.
Results and discussions
Energy savings in the PRO-SWRO hybrid configuration
The pressure at which the DS is pumped into the PRO modules influences the amount of energy generated and eventually saved in the PRO-SWRO hybrid configuration. In the (SW, BR) case, when a pressure of 20 bar is used on the DS side, the amount of energy saved in the PRO-SWRO hybrid configuration is 7% when compared with the SWRO base case.
Breakeven costs of the PRO modules
Figure 5 shows the breakeven costs of PRO membrane modules at a 20 bar DS feed pressure, with varying lifetimes/replacement times for the PRO modules/membranes. At any given cost of electricity, an increase in the lifetime of the PRO module allows an increase in the breakeven cost of the PRO module due to the following reasons:
• the OPEX related to PRO membrane decreases, and
• as the NPV of the PRO-SWRO case has to match the NPV of the base case, the CAPEX of the PRO membranes has to increase.
Lower costs of electricity push the breakeven cost of PRO towards lower values, implying that the costs of the PRO modules need to be reduced for the PRO-SWRO system to become feasible. The breakeven PRO module costs at a 5-year life are 1431 $, 1149 $, and 185 $ at electricity costs of 0.16 $/kWh, 0.12 $/kWh, and 0.08 $/kWh, respectively.
As discussed, different scenarios were studied using the developed techno-economic model. Figure 6 shows the difference in the NPV values between the PRO-SWRO hybrid case and the SWRO base case. The DS feed pressure was 20 bar, the cost of electricity for usage was taken to be 0.12 $/kWh, the PRO membrane cost was considered as 450 $, and the PRO membrane life was taken as 5 years. The payback period is found to be between 16 and 17 years. On the other hand, when the cost of electricity is taken as 0.16 $/kWh (see Figure 7), the payback period is found to be between 10 and 11 years. If 0.08 $/kWh electricity is considered, the difference in the NPVs would always remain negative with payback never occurring. This implies that the cost at which electricity is available for running the plant plays an important role in determining the payback period, with higher values resulting in quicker payback.
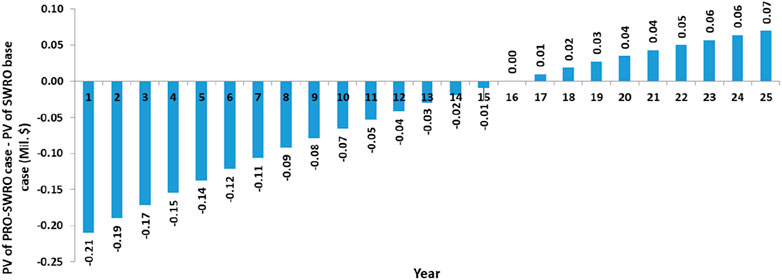
Figure 6. PRO-SWRO hybrid case with 20 bar DS feed pressure. The PRO membrane element cost was assumed as 450 $ and sourced electricity cost as 0.12 $/kWh. Payback occurs between 16–17 years. Y-axis shows the difference in the present value of the PRO-SWRO hybrid case and the SWRO base case.
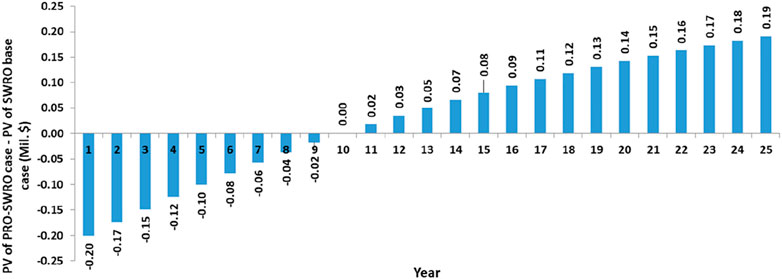
Figure 7. PRO-SWRO hybrid case with 20 bar DS feed pressure. The PRO membrane element cost was assumed as 450 $ and sourced electricity cost as 0.16 $/kWh. Payback occurs between 10–11 years. Y-axis shows the difference in the present value of the PRO-SWRO hybrid case and the SWRO base case.
Figure 7 highlights the importance of the development in membrane industry and its competence. The development of PRO membranes is in a similar position to where RO membranes were at several decades ago. To achieve similar success, the membrane industry must focus on several key areas:
Material innovation: Developing new materials that offer higher water permeability and selectivity, as well as greater resistance to fouling and degradation.
Manufacturing techniques: Improving manufacturing processes to reduce costs and enhance the quality and consistency of membranes.
Scale of production: Increasing the scale of production to achieve economies of scale, thereby reducing the unit cost of membranes.
PRO membrane cost
It was reported that; on nanofiltration (NF) reject (wastewater was used as NF feed) usage as the FS and SWRO brine as the DS in a PRO-SWRO system mentioned 5 $/m2 as the viable membrane cost (Sajna et al., 2024). Our calculations indicate values less than 2.2 $/m2 (1431 $/650 m2) as the viable cost for PRO membranes when the cost of electricity is 0.16 $/kWh. Personal communication with some commercial membrane providers indicated a cost varying between 9.2 $/m2 to 12.3 $/m2. As seawater was used as the FS and SWRO brine was used as the DS in our studies, the lower dilution flux from FS to DS side results in lower energy production, which lowers the energy savings achievable. This results in the estimation of low values for the PRO membrane cost which are a part of CAPEX in the NPV calculations. One way of overcoming this is by developing membranes that can achieve higher dilution fluxes even with seawater as the FS.
The study highlights the economic viability of PRO membranes in hybrid PRO-SWRO systems. Energy savings in this configuration are notably influenced by the draw solution (DS) pressure; for instance, using a 20 bar pressure on the DS side results in 7% energy savings compared to standard SWRO systems. Additionally, the study analyses the breakeven costs of PRO membrane modules concerning varying electricity costs influenced by global oil price fluctuations.
To optimize these systems, the study recommends focusing on material innovation, manufacturing techniques, and scaling production. These strategies aim to reduce unit costs and improve performance, mirroring the historical development path of Reverse Osmosis (RO) membranes.
Overall, these findings underscore the transformative potential of PRO-SWRO integration in enhancing energy efficiency and sustainability in desalination practices. This potential hinges on advancements in membrane technology and favorable electricity pricing strategies.
Conclusions and recommendations
In this study, the possible energy savings which can be realized in a PRO-SWRO hybrid system were evaluated and the economic feasibility was quantitatively analyzed. Implementing PRO for SWRO feed pressurization can yield substantial energy savings of up to 7%, particularly when utilizing pre-treated seawater as the feed solution and SWRO brine as the draw solution. The energy savings will be higher when treated wastewater is used as the FS. However, collocation of a wastewater treatment plant near a desalination plant is not always possible, implying that pre-treated seawater needs to be used in such cases.
Electricity cost plays an important role in the techno-economics of the PRO-SWRO system, with higher electricity costs making the system feasible and lower electricity costs making it infeasible.
When seawater is used as the FS and SWRO brine as the DS in the PRO system, the cost of the PRO membrane needs to be kept low to make up for the lower energy production in such a system. One way to overcome this is by developing new PRO membranes that allow higher fluxes even when the difference in the FS and DS salinities is not very high.
Our analysis extends beyond the general levelized cost of energy/electricity, demonstrating that despite potentially higher PRO membrane costs, financial savings remain feasible, especially in scenarios where electricity costs are high. In summary, the PRO-SWRO hybrid system has the transformative potential of enhancing energy efficiency and advancing sustainable desalination practices. The study aims to enhance energy efficiency in desalination practices by providing a comprehensive techno-economic analysis of a hybrid Pressure Retarded Osmosis (PRO) and Seawater Reverse Osmosis (SWRO) system. It demonstrates significant energy savings and economic feasibility through optimized integration.
Data availability statement
The raw data supporting the conclusions of this article will be made available by the authors, without undue reservation.
Author contributions
AmA: Conceptualization, Investigation, Methodology, Supervision, Validation, Writing–original draft, Writing–review and editing. AhA: Conceptualization, Data curation, Formal Analysis, Investigation, Methodology, Project administration, Resources, Software, Supervision, Validation, Writing–original draft, Writing–review and editing. SA: Data curation, Formal Analysis, Methodology, Project administration, Resources, Software, Supervision, Validation, Visualization, Writing–original draft, Writing–review and editing.
Funding
The author(s) declare that no financial support was received for the research, authorship, and/or publication of this article.
Conflict of interest
The authors declare that the research was conducted in the absence of any commercial or financial relationships that could be construed as a potential conflict of interest.
Publisher’s note
All claims expressed in this article are solely those of the authors and do not necessarily represent those of their affiliated organizations, or those of the publisher, the editors and the reviewers. Any product that may be evaluated in this article, or claim that may be made by its manufacturer, is not guaranteed or endorsed by the publisher.
Abbreviations
CAPEX, Capital Expenditure; DS, Draw Solution; ERD, Energy Recovery Device; FS, Feed Solution; NF, Nanofiltration; NPV, Net Present Value; OPEX, Operational Expenditure; PRO, Pressure Retarded Osmosis; SWRO, Seawater Reverse Osmosis
References
Achilli, A., Cath, T. Y., and Childress, A. E. (2009). Power generation with pressure retarded osmosis: an experimental and theoretical investigation. J. Membr. Sci. 343 (1-2), 42–52. doi:10.1016/j.memsci.2009.07.006
Altaee, A., Zaragoza, G., and van Tonningen, H. R. (2014). Comparison between forward osmosis-reverse osmosis and reverse osmosis processes for seawater desalination. Desalination 336, 50–57. doi:10.1016/j.desal.2014.01.002
Al-Zainati, N., Subbiah, S., Yadav, S., Altaee, A., Bartocci, P., Ibrar, I., et al. (2022). Experimental and theoretical work on reverse osmosis - dual stage pressure retarded osmosis hybrid system. Desalination 543, 116099. doi:10.1016/j.desal.2022.116099
Banchik, L. D., and Lienhard, J. H. (2012). “Thermodynamic analysis of a reverse osmosis desalination system using forward osmosis for energy recovery,” in Proceedings of the ASME 2012 international mechanical engineering congress and exposition. IMECE2012, November 9-15, Houston, Texas, USA.
Bharadwaj, D., Fyles, T. M., and Struchtrup, H. (2016). Multistage pressure-retarded osmosis. J. Non-Equilibrium Thermodyn. 41. doi:10.1515/jnet-2016-0017
Cath, T. Y., Childress, A. E., and Elimelech, M. (2006). Forward osmosis: principles, applications, and recent developments. J. Membr. Sci. 281 (1-2), 70–87. doi:10.1016/j.memsci.2006.05.048
Helfer, F., Lemckert, C., and Anissimov, Y. G. (2014). Osmotic power with pressure retarded osmosis: theory, performance and trends – a review. J. Membr. Sci. 453, 337–358. doi:10.1016/j.memsci.2013.10.053
Huang, Z., Yang, Z., Gao, X., and Gao, C. (2023). Power feasibility of single-staged full-scale PRO systems with hypersaline draw solutions. J. Water Process Eng. 63, 105561. doi:10.1016/j.jwpe.2024.105561
Jiao, Y., Song, L., Zhao, C., An, Y., Lu, W., He, B., et al. (2022). Membrane-based indirect power generation technologies for harvesting salinity gradient energy - a review. Desalination 525, 115485. doi:10.1016/j.desal.2021.115485
Liang, Y. Y. (2023). Review of analytical and numerical modeling for pressure retarded osmosis membrane systems. Desalination 560, 116655. doi:10.1016/j.desal.2023.116655
Loeb, S. (1976). Production of energy from concentrated brines by pressure-retarded osmosis. J. Membr. Sci. 1, 49–63. doi:10.1016/s0376-7388(00)82257-7
Lutchmiah, K., Verliefde, A. R. D., Roest, K., Rietveld, L. C., and Cornelissen, E. R. (2014). Forward osmosis for application in wastewater treatment: a review. Water Res. 58, 179–197. doi:10.1016/j.watres.2014.03.045
Matsuyama, K., Makabe, R., Ueyama, T., Sakai, H., Saito, K., Okumura, T., et al. (2021). Power generation system based on pressure retarded osmosis with a commercially-available hollow fiber PRO membrane module using seawater and freshwater. Desalination 499, 114805. doi:10.1016/j.desal.2020.114805
McGinnis, R. L., McCutcheon, J. R., and Elimelech, M. (2007). A novel ammonia-carbon dioxide osmotic heat engine for power generation. J. Membr. Sci. 305 (1-2), 13–19. doi:10.1016/j.memsci.2007.08.027
Rahman, S. N., Saleem, H., and Zaidi, S. J. (2023). Progress in membranes for pressure retarded osmosis application. Desalination 549, 116347. doi:10.1016/j.desal.2022.116347
Ramon, G. Z., Feinberg, B. J., and Hoek, E. M. V. (2011). Membrane-based production of salinity-gradient power. Energy and Environ. Sci. 4 (11), 4423–4434. doi:10.1039/c1ee01913a
Ruiz-García, A. (2024). Power feasibility of single-staged full-scale PRO systems with hypersaline draw solutions. J. Water Process Eng. 63, 105561. doi:10.1016/j.jwpe.2024.105561
Ruiz-García, A., Tadeo, F., and Nuez, I. (2022). Simulation tool for full-scale PRO systems using SWMMs. Desalination 541, 116025. doi:10.1016/j.desal.2022.116025
Ruiz-Garcia, A., Tadeo, F., and Nuez, I. (2023). Role of permeability coefficients in salinity gradient energy generation by PRO systems with spiral wound membrane modules. Renew. Energy 215, 118954. doi:10.1016/j.renene.2023.118954
Sajna, M. S., Elmakki, T., Schipper, K., Ihm, S., Yoo, Y., Park, B., et al. (2024). Integrated seawater hub: a nexus of sustainable water, energy, and resource generation. Desalination 571, 117065. doi:10.1016/j.desal.2023.117065
She, Q., Jin, X., Li, Q., and Tang, C. Y. (2012). Relating reverse and forward osmosis (RO and FO) membrane structural parameters to filtration performance. Environ. Sci. and Technol. 46 (8), 4673–4681.
Thorsen, T., and Holt, T. (2009). The potential for power production from salinity gradients by pressure retarded osmosis. J. Membr. Sci. 335 (1-2), 103–110. doi:10.1016/j.memsci.2009.03.003
Keywords: hybrid desalination, pressure retarded osmosis (PRO), osmotic energy, efficiency, sustainable, brine management
Citation: Ahmed A, Alghamdi A and Ahmed S (2024) Techno-economic analysis of a pressure retarded osmosis (PRO) - seawater reverse osmosis (SWRO) hybrid: a case study. Front. Energy Res. 12:1448402. doi: 10.3389/fenrg.2024.1448402
Received: 13 June 2024; Accepted: 08 August 2024;
Published: 22 August 2024.
Edited by:
Mohamed Farghali, Kobe University, JapanReviewed by:
Alejandro Ruiz-García, University of Las Palmas de Gran Canaria, SpainCopyright © 2024 Ahmed, Alghamdi and Ahmed. This is an open-access article distributed under the terms of the Creative Commons Attribution License (CC BY). The use, distribution or reproduction in other forums is permitted, provided the original author(s) and the copyright owner(s) are credited and that the original publication in this journal is cited, in accordance with accepted academic practice. No use, distribution or reproduction is permitted which does not comply with these terms.
*Correspondence: Ahmed Alghamdi, YWFsLWdoYW1kaTM0YWNjYkBzd2NjLmdvdi5zYQ==