- 1Department of Mechanical/Biomedical Engineering, Bells University of Technology, Ota, Nigeria
- 2Department of Mechanical Engineering, Federal University of Agriculture, Abeokuta, Nigeria
- 3Department of Mechanical Engineering, University of Calabar, Calabar, Nigeria
- 4Department of Mechanical Engineering, Covenant University, Ota, Nigeria
- 5Department of Mechanical Engineering, Federal University of Petroleum Resources, Effurun, Nigeria
- 6Department of Mechanical Engineering, University of South Africa, Science Campus, Florida, South Africa
- 7Department of Chemical Engineering, University of Strathclyde, Glasgow, United Kingdom
- 8Department of Chemical and Metallurgical Engineering, Tshwane University of Technology, Pretoria, South Africa
Given the increased natural resource consumption of contemporary energy conversion systems, as well as the emissions, waste disposal, and climate changes that accompany them, a critical review of new techniques - known as thermo-economic and thermo-environmental analyses - has been carried out for the evaluation and optimization of energy conversion processes, from the perspectives of thermodynamics, economics, and the environment. Such a review study is essential because of the energy system’s impacts on sustainability and performance management requirements, and more importantly, it is crucial to understand the whole picture of performance evaluation of energy systems from the sustainability perspective. The study evaluated the performance and optimization of energy systems and examined the different approaches that integrate the economic, environmental, and second law of thermodynamics for sustainable development. Moreover, to assess the technical, economic, and environmental worth of energy systems and guarantee that the chosen designs are well-suited to a sustainable development framework, a mix of thermodynamic, economic, and environmental indicators is taken into consideration. In this regard, thirteen sustainability indicators for the design, analysis, and performance improvement of energy systems from the viewpoints of thermodynamics, economics, and the environment are presented and discussed. The outcome of this study shows that (i) the sustainability of energy conversion systems can be enhanced with the use of exergy techniques assessment; (ii) by reducing energy losses, exergy efficiency initiatives can lessen their adverse effects on the environment; (iii) the best methods for efficient use of energy resources, low energy production costs, and less environmental impact can be provided by hybrid energy systems; and (iv) use of a single performance metric to optimize the energy process results in improbable outcomes. Hence, multi-criteria techniques should be utilized, allowing for a more comprehensive optimization and planning of sustainable energy systems. Researchers and field engineers working on energy systems’ design, modeling, assessment, and performance optimization would find great value in this comprehensive review study.
1 Introduction
Today, scientists and engineers are developing new technologies and more effective energy-harvesting systems to maximize the use of available energy resources. Because of this, studying and improving energy conversion systems is important from a scientific standpoint and is also necessary for the effective use of available energy resources (Oyedepo, 2014a). Any country’s ability to grow economically depends on its supply of energy. Social developments impact the energy system in various ways, but society is also affected by the energy system (Oyedepo, 2014b). The production and availability of energy significantly influence global economic and political development. A strong energy sector is essential for success in other socioeconomic domains. The link between natural resource availability and security for energy consumption is known as energy security. These days, having access to economically priced energy is crucial to the functioning of modern economies. The attainment of the Sustainable Development Goals (SDGs) is contingent upon every individual having access to modern, adequate, and efficient energy (Bishoge et al., 2020). Since then, a vast array of technical applications for energy have been created, making energy availability a crucial concern for civilization (Huggins, 2016).
Sustainable development has recently been recognised as the utmost task of this new age (Annamalai et al., 2018). The latter leads to several transnational consequences, particularly environmental contamination, climate change, reduction of natural deposits, natural decimation, and international injustice (Akbari et al., 2020). Human activities have added undesirably to sustainable growth. Additionally, it is known that a transformation to economic sustainability requires substantial variations in energy demands and resources (Mulvihill et al., 2011). However, in recent times, the lack of adequate energy supply and limited capital, followed by efforts to alleviate the hazard posed by contamination of the environment, has resulted in the growth of feasible engineering science (Das et al., 2019).
The prime objective of sustainable technology is to attain economic feasibility for scenic surroundings. Sustainable technology has a principal goal in environmental disciplines and the development of worldwide economies, generally linked alongside the design layout and breakdown of multifaceted, unified energy processes and economic sustainability. Lessening material and energy supply and minimising misuse is a significant environmental objective (Srebrenkoska et al., 2013). Sustainable energy is necessary for any strategies for global sustainability due to (i) the generality of energy usage, (ii) its demand in economic growth and standards of living, and (iii) the significant effects of energy processes and systems on the environment and its continued influence (Oyedepo, 2012). Increasing environmental issues and limited available non-renewable conventional energy resources have engendered new impulses in modern sustainable energy technologies. This is because resources for energy and their utilisation closely have to do with sustainable developments. The development of cleaner energy systems is one of the significant advances of this advanced century. Most forecasts demonstrate that combustion type of energy conversion systems remains the prime method for most energy consumption (Lieuwen and Yang, 2013). Hence, to accomplish sustainable progress, it is crucial to increase the efficiencies of energy processes that utilize sustainable energy (Hepbasli, 2008).
In the next 20 years, energy use worldwide is projected to rise considerably worldwide, with developing countries having the highest growth rates. Fossil fuels are anticipated to deliver a big part of the world’s energy, up to 75% of overall energy utilization in 2040 (Rao, 2015). Following the massive expansion in petroleum derivative use around the world, the ozone-depleting substance CO2 emanations to the environment are relied upon to increment by an average yearly development of 1.29% within the range of the years 2010–2040 or by as far as 45.8% by 2040 from 2010. Notwithstanding this ozone-harming substance, the tackling of the energy trapped inside a fuel by incineration generates contaminations like oxides of sulphur, nitrogen, and partly combusted fossil fuels that are brought into the air, the quantity dependent upon the effectiveness and efficiency of the technology utilized for transformation. A challenge emanates between raising the quality of life (assessed by gross national product per capita), and energy use per capita that can directly influence the surroundings provided sustainable energy conversion systems and operations are followed up.
Research in generating alternative energy sources has made more energy available to consumers. Different equipment and processes convert energy into different forms, as shown in Table 1. An IC engine converts the chemical energy from gasoline into heat, which in turn creates mechanical energy that moves a vehicle. The energy passes through a device that converts it, which produces another energy source. The output energy is always less than the input energy. However, the quantity of energy is conserved (Demirel, 2012). Figure 1 depicts a representation of the energy use and its conversion process.
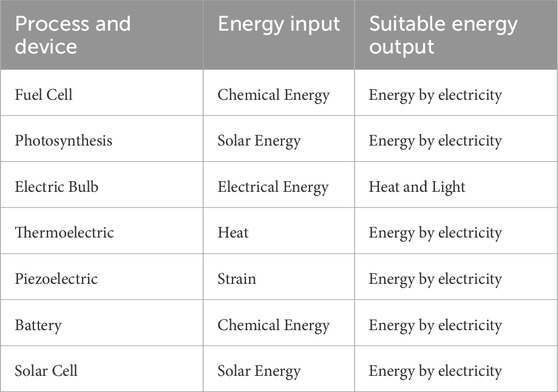
Table 1. Processes/devices for energy conversion (Demirel, 2012).
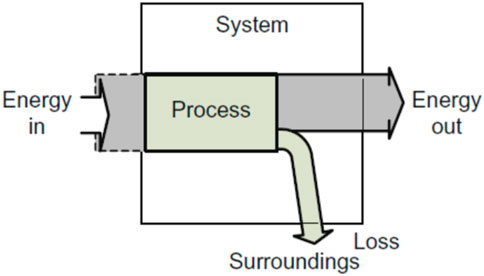
Figure 1. Diagram of energy use and its conversion process (Demirel, 2012).
The different forms of energy are grouped into three general categories: (i) primary energy (hydropower, fossil fuel, hydraulic energy, wind energy and solar energy), (ii) secondary energy (gasoline, diesel, and kerosene. Others are, ethanol fuel and biodiesel derived from biomass, thermal energy from solar collectors, and district heating from geothermal fluids), (iii) tertiary energy (electric energy, nuclear energy, gas, coal, and most of the different renewable energy source of energy, which are categorised as primary energy sources, contribute to the supply of tertiary energy when they are used to produce electricity). The processing and transformations that produce secondary and tertiary energy forms always involve energy losses, which are often significant for tertiary energy (Khartchenko and Kharchenko, 2014). The first two laws of thermodynamics control the energy dissipation during such transformations. The efficiency of the transformation process indicates how well the primary resource’s original energy can be converted to the secondary form (Michaelides, 2018).
Thermal efficiencies of energy transformation are typically far less than 100% because of inefficiencies caused by friction, heat loss, and other factors. For example, in a steam power station, only 35%–40% of the thermal energy can be transformed into electrical current. A typical gasoline automobile engine operates at about 25% efficiency. Energy is always conserved, according to the first law of thermodynamics. But some of the energy that was initially available is lost during energy transformations as waste heat that is not needed or used by human society.
Due to different energy conversion technologies based on differences in economic and environmental factors, energy utilization should be guided by principles whose validity has been proven beyond doubt (Giovanni, 2014). Hence, analysis and optimisation of energy systems are logical and vital for humanity from the socio-economic and environmental points of view.
Furthermore, the past 2 decades have witnessed tremendous transformation in energy technology, now a focal field for research and development studies. Extensive studies were carried out in energy system research to optimize energy conversion efficiency and advance the technologies that promote other energy sources. The main objective of such studies was to determine the degradation effect of exergy resources. To complement this drive, the research area of thermo-economic and thermo-environmental involves combining thermodynamic, economic and environmental issues in design, assessment and enhanced optimal performance of energy conversion systems and industrial process efficiencies without jeopardizing an energy system’s financial feasibility. The resulting improvement in efficiency has further decreased environmental impact due to improved system efficiency (von Spakovsky and Frangopoulos, 2011).
The sustainable utilization of energy is inherently connected to the systems’ effectiveness in transforming crude materials into useful products. The technology of these energy converters straightforwardly impacts the value and amount of their energy consumption, services, and the effluent dispersed to the atmosphere. In this regard, the consumption of sources of fuel, paying little mind to fossil fuel or the supposed renewable energy, should be led by utilizing an effective conversion system during its final use as well as in the entire process of energy transformation measures starting from the fuel source, through the energy conversion process till the waste disposal stage. Assessing each stage of energy usage is imperative in determining the extent of energy quality (de Oliveira, 2013).
In view of the above, the energy conversion system is recognised as a device that consumes fuel and other scarce natural resources. Consequently, it has an adverse effect on the atmosphere in the course of operation. Hence, the study on assessing and optimising energy systems must consider sustainability matters quantifiably. Accordingly, this paper focuses on the critical review of the enhancement and sustainability of energy systems from thermodynamic, thermo-economic and thermo-environmental perspectives, considering selected performance indicators as signposts of sustainability for energy conversion systems.
2 Thermodynamics assessment of energy conversion systems
Thermodynamics plays a vital role in dissecting frameworks and equipment where energy transformation occurs. Thermodynamics’ significance is across the board of human initiative (Dincer and Cengel, 2001). Thermodynamics science follows two central natural laws, the first and second (Oyedepo, 2014a). The thermodynamic first law emphasizes that energy as a thermodynamic property interacts and can transform from state to state. However, the energy is conserved. The first law is conventionally used to analyze energy consumption and plant operation. Unfortunately, it does not consider the energy quality, which is now remedied by the second law of thermodynamics. The thermodynamic second law affirms that energy has quantity and quality and that real processes exist in the path of reducing the quality of energy called exergy.
The energy system is designed to provide energy services to consumers by continuously evaluating its exergy performance (Granet and Bluestein, 2000). Exergy studies have been used to extract the maximum amount of useful work or performance of an operation as it moves from one equilibrium state to another (Ahmadi et al., 2011a). It is also considered the elementary notion by which the thermodynamic cycle is modelled and analyzed by the applications of the laws of thermodynamics (Zare and Hasanzadeh, 2016). Moreover, it thoroughly analyzed energy systems’ efficiencies, optimal performance and irreversibility sources (Saidur et al., 2007).
Surprisingly, the various means of generating and supplying power are unsustainable and costly, leading to an upsurge in energy demand; if appropriate actions are not taken, this increase in energy demand will be truncated with more energy waste, resulting in environmental pollution. Therefore, if carried out sustainably, increased energy demand, efficient energy systems, improved technologies, and environmental preservation dictate improvement in a nation’s industrial development (Saidur et al., 2010).
2.1 Analysis of energy and exergy of an energy systems
Presently, humankind faces incredible economic, environmental, and energy difficulties. A solid essential exists to more readily configure, investigate, evaluate and enhance energy measures, systems and utilization (Dincer et al., 2014). Accordingly, energy and exergy analysis are the two essential tools for designing and optimising energy systems to use effectively resource-constrained fossil fuels (Oyedepo et al., 2018). Researchers have delved into using exergy analysis to overcome the shortcomings of the first and foremost law of thermodynamics.
Moreover, studies reveal a correlation between sustainable development and exergy. Both will establish that the energy system is cost-effective, steady and non-threat to the environment. Hence, exergy analysis is broadly employed in the design of our energy equipment (Hepbasli, 2008). The exergy idea is dependent on the first two thermodynamic laws. Exergy assessment shows the positions of energy deterioration in a procedure and can result in enhanced technology. Exergy analysis mainly detects energy losses in a system and the magnitude of such loss. It also identifies energy efficiency to optimize the system (Zare, 2020).
With the exergy analysis concept, it is possible to gauge energy transformation operation processes on a thermodynamics aspect and the eco-environmental impact of the process in the study. This inclusive approach to energy conversion is essential for sustainable energy resource utilization (de Oliveira, 2013). Exergy methods can help improve the sustainability of energy systems. Studies have indicated that enhancing the exergy efficiency of energy systems can reduce environmental impacts by cutting down on energy wastage (Oyedepo, 2014a; Rao, 2015; Ahmadi et al., 2011a; Zare and Hasanzadeh, 2016). A measure to assess the relationship between exergy efficiency and environmental sustainability is the Exergetic Sustainability Index (ESI). This indicator helps to determine the capacity and effective utilization and preservation of energy resources (Dincer et al., 2014; Oyedepo et al., 2018; Rosen, 2021). Figure 2 illustrates this qualitatively by demonstrating how, as exergy efficiency increases, sustainability increases and environmental impact decreases. Exergy efficiency becomes closer to 100%, sustainability approaches infinity since the process approaches reversibility, while environmental impact approaches zero because exergy is converted from one form to another without any loss. Exergy efficiency approaches 0% since exergy resources are used to accomplish nothing, hence, sustainability approaches zero. On the other hand, environmental impact approaches infinity because increasing resources must be used and increasing exergy wastes are emitted for a fixed service (Rosen, 2021).
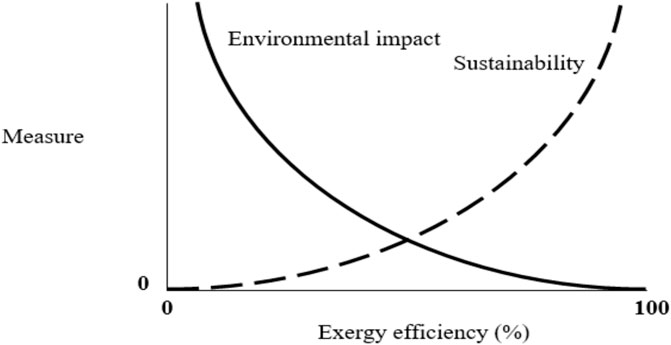
Figure 2. Qualitative depiction of relation of environmental impact and sustainability with exergy efficiency (Rosen, 2021).
Environmental pollution from fossil fuels has led to numerous studies on energy-efficient conversion and eco-friendly renewable energy sources (Rosen, 2021). An energy-efficient system assures sustainable development. A deliberate improved system as a function of exergy analysis will usher in reliability, sustainability and cost-effectiveness (Hepbasli, 2008). A correlation exists among exergy analysis, sustainable development, and ecological and environmental management (Rosen, 2009). The analysis of exergy aids in projecting thermodynamics into ecological and environmental impact on devices in consideration. The essence of exergy analysis investigates the useful energy that does work on the system. It looks into the primary source of irreversibility in the cycle and improves it to prevent exergy destruction.
Another essential feature of the second law of thermodynamic (exergy) concept is its capability to be integrated with economic and environmental constraints. These integrated methods, called “thermo-economic and thermo-environmental” approaches, are powerful tools to identify, quantify, and interpret economic losses and environmental burdens of energy systems at the component level. Precisely, the thermo-economic method can effectively address the shortcomings of techno-economic analysis by accounting for thermodynamic losses (Al-Qayim, 2019). In addition, the thermo-environmental method can reliably cope with the drawbacks of Life Cycle Assessment (LCA) analysis in the sustainability assessment of energy systems by allocating the environmental burdens at the component level and measuring the environmental burdens of intermediate products. This unique combination of exergy, economy, and environment can reliably assess the thermodynamic productivity, economic viability, environmental safety, and overall sustainability of energy and material conversion processes (Figure 3) (Aghbashlo et al., 2022).
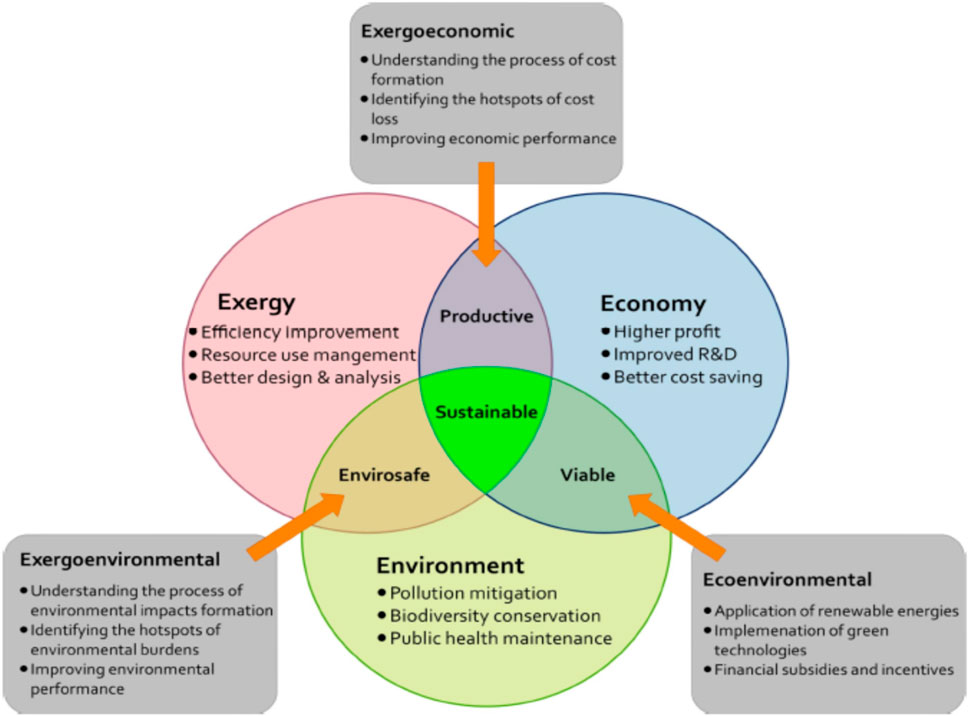
Figure 3. Opportunity interactions among exergy, economy, and environment (Aghbashlo et al., 2022).
Additionally, numerous studies on the exergy assessment of energy operations have been carried out to study the variability of energy systems for power generation or industrial processes. Among the explored studies are gas turbines (Rosen, 2021; Aghbashlo et al., 2022), steam power plants, combined cycle power plants, refrigeration systems, ORC and Kalina cycles, wind energy and solar energy systems, among others (Ahmadi et al., 2011a; Saidur et al., 2010; Rosen, 2021; Aghbashlo et al., 2022; Waheed et al., 2018).
2.1.1 Gas turbine engine
The demand for gas turbine engines is increasing due to their low cost, high flexibility, high reliability without complexity, short delivery time, and fast starting and loading but low efficiency. Several analyses have therefore been conducted to improve the low thermal efficiency in Simple Gas Turbine (SGT) (Rosen, 2009). In recent times, efforts to improve the power generation efficiency of SGTs have been through gas-to-gas recuperation, steam injection (STI), evaporation cycle, chemical recuperation, inlet air cooling (IAC) and combined cycle. These efforts have led to an increase in output power, thermal efficiency and work-back ratio (Waheed et al., 2018).
A typical gas turbine unit comprises a low-pressure turbine (LPT) and a high-pressure turbine (HPT). The LPT consist of four segments with an effectual blade for cooling and negligible leak. The HPT is positioned within two combustors possessing a single stage. During the initial expansion, most of the exhaust gases diffuse to the second combustor (Aghbashlo et al., 2022). Numerous researchers have worked on the thermodynamic assessment of different gas turbines (Wang and Chiou, 2004; Farzaneh-Gord and Deymi-Dashtebayaz, 2009). The thermodynamic investigation of gas turbines revealed that ambient temperature, humidity, pressure ratio, turbine inlet temperature (TIT) (around 500°C) and nature of fuel are the essential factors affecting gas turbine performance. The enormous exhaust temperature shows that energy is being flared uncontrollably into the environment. In the work of Sulaiman et al. (2017), the effect of decreasing entry air cooling of the compressor with energy wasted in the gas pressure drop station on a basic gas turbine power plant was studied. They proposed recuperating misused energy within exhaust through the heat-regaining steam generator and adding steam into the combustion compartment. The least specific fuel consumption of 0.15 kg/kWh, the highest energetic efficiency of 47.35%, and high-enhanced capacity in thermal efficiency along with power output were reported. Fagbenle et al. (2014) applied an exergy study to numerous gas turbine power equipment in Nigeria. They stated that the energetic efficiency improved as the turbine inlet temperature increased.
Oyedepo et al. (2015c) evaluated a selection of gas turbine performance in Nigeria utilizing energy and exergy analyses. The study revealed that the maximum proportion of energy loss happened in the combustion channel, ranging from 33.30% to 39.94%. The exergy investigation additionally confirmed the combustion compartment as the utmost exergy critical part contrasted with other cycle constituents. The exergy ruin in the fire chamber ranged from 86.04% to 94.66%. Its energetic proficiency is lesser than other workings. A rise in the entry turbine temperature increased the exergetic effectiveness in response to an upsurge in the turbine’s outlet power and losses in the combustion chamber.
Zhang et al. (2018) suggested eight arrangements of gas turbines fuelled by biomass gasification and simulated their performance. They described that exergy effectiveness ranged between 22.2% and 37.2%. De Souza-Santos also investigated the functioning of a gas turbine fuelled by syngas from the environment and the pressurized gasifying of sugarcane bagasse (De Souza-Santos, 1999). They reported that the syngas obtained from pressurised vaporization produce greater effectiveness than the climatic one. Gümüş (Gümüş and Atmaca, 2013) investigated the exergy effect on mixed fuel and diesel in a diesel engine. Exergy, energy and irreversibility increase as engine speeds increase for both energy sources. Mixed fuel proved to possess a higher exergy efficiency than diesel fuel. The operation of some gas turbine devices was studied by Almutairi et al. (Granet and Bluestein, 2000) on diverse load requirements and weather circumstances applying two configurations. The reheat systems boosted output power and reduced exergy efficiency compared to the elementary gas turbo. Athari et al. (2015a) conducted an exergy analysis on a simple gas turbine. It was observed that the combustor’s improved exergy efficiency was achieved once the compressor pressure ratio was increased. The compressor pressure ratio is seen as a focal factor determining both energy and exergy efficiencies of both steam injection gas turbines alone and steam injection gas turbines with inlet fogging cooler.
Ahmadi et al. (2013) performed an all-inclusive thermodynamic simulation and multipurpose optimisation of a multi-operational system comprising an absorption chiller, ejector refrigeration system, heat regain steam generator, and an electrolyser that gives numerous products: energy, hot water boiler, refrigeration, and hydrogen (Figure 4). Exergy and environmental studies were performed. A multipurpose optimisation method based on a rapid and elite non-dominant categorization genomic process was applied to assess the system’s optimal design factors. The two goals tasks used across the streamlining study were the framework’s complete expense rate, which shaped the expense related to fuel, part procuring and environmental effect, and the system’s exergy effectiveness. The framework’s all-out expense rate was scaled down while the cycle exergy effectiveness was expanded utilizing a developmental calculation (evolutionary algorithm). A shut structure condition about the connection amid exergy proficiency and the overall cost was inferred. A sensitivity investigation was also accomplished to evaluate the impacts of a few plan boundaries’ total exergy destruction rate on the system, carbon dioxide discharge, and exergy effectiveness. The major conclusions made were that an increase in heat recovery steam generator pressures increased exergy efficiency of the system and decreased the system’s rate of total cost. In contrast, a rise in heat recovery steam generator throttle point temperatures reduced the exergy efficiency of the system.
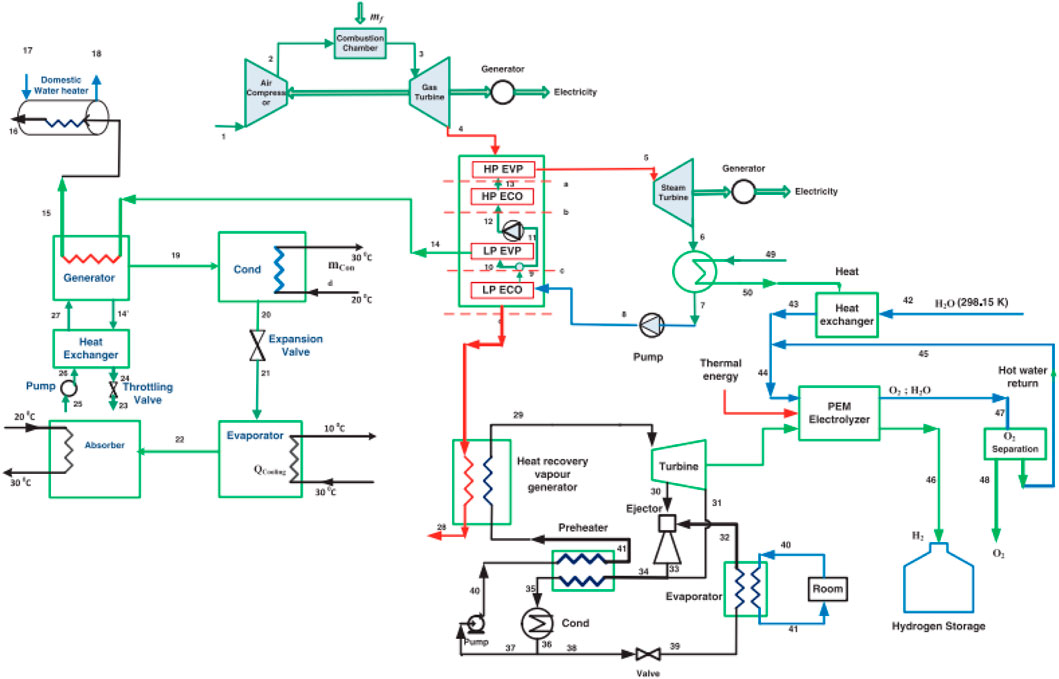
Figure 4. Multigeneration energy system (Ahmadi et al., 2013).
Ahmadi et al. (2012) executed an all-inclusive thermodynamic modelling of a trigeneration operation for heating system, chilling, and energy generation. The trigeneration operation comprises a gas turbine cycle, an ORC, a single-effect absorption chiller and a native water boiler (Figure 5). An exercise-based environmental analysis was undertaken, and parameters that assessed ecological effect and sustainability were gauged. The trigeneration system’s exergy efficiency was discovered to be greater than classic mixed heating and power systems. The outcome also shows that the trigeneration system’s CO2 emissions are less than those mentioned above. The exergy results revealed that the exergy obliteration was most elevated in the combustion chamber because of the temperature contrast between the working fluid and combustion temperature. The study concludes that the entry temperature of the turbine, the pressure proportion of the compressor, and the turbine’s isentropic efficiency significantly affect the system’s performance. Moreover, raising the inlet temperature of the turbine diminishes the expense of the surrounding effect, basically by lessening the concentration transfer rate of the combustion chamber.
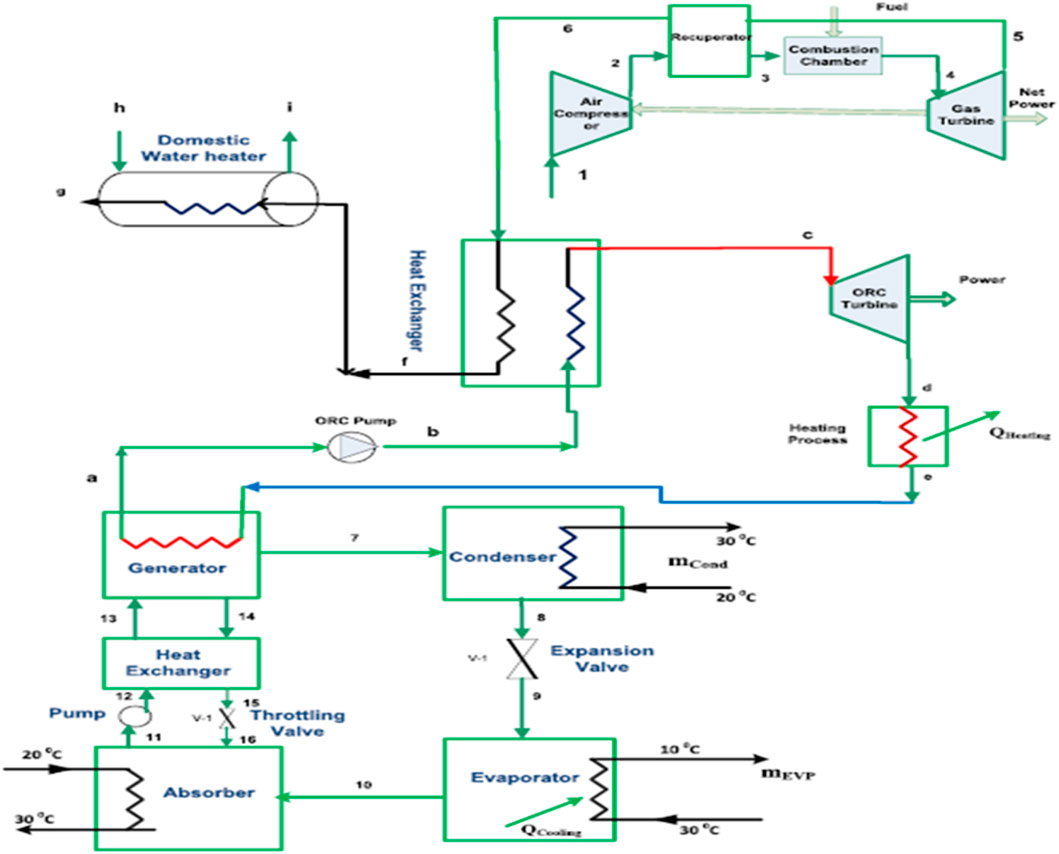
Figure 5. Representation of a Multigeneration system for steaming, refrigeration plus power generation (Ahmadi et al., 2012).
2.1.2 Steam power plant
Steam injection technology enhances the functioning of a basic gas turbine. Ghazikhani et al. (2005) studied the impact of steam injection at the Mashhad Power Plant with a gas turbine, GE-F5. They observed an increase of 10% in the system’s thermal efficiency while the back-work ratio improved by 15%. Bouam et al. (2008) enhanced a gas turbine under Sahara conditions by injecting steam into the combustion chamber when the efficiency of the steam gas turbine was held constant at 50% while the ambient temperature was varied from ISO conditions to 50°C. This study shows that steam injection makes it possible to achieve high efficiency. Ziółkowski et al. (2012) also investigated the effects of steam injection on a gas turbine performance at the PGE Gorzow power plant using COM-GAS code and Aspen plus software. Agarwal and Mishra, (2011) reviewed current and future sustainable gas turbine technologies by comparing their thermodynamic characteristic and concluded that mixed air steam technologies offered superior performances compared to other technologies. Comparative exergoeconomic analysis of a steam injection gas turbine fuelled with biomass gasification was also studied to assess the influences and performance of operating variables with and without fogging inlet cooling (Jana and De, 2014).
Keçebaş and Gokgedik (2015) performed conventional and advanced exergy analyses of an existing geothermal binary power system for power generation (Figure 6). Details about the exergy destruction in the system and its parts were analysed through advanced analysis to investigate the interactions between the system components and the actual performance of the reasonable improvements. The obtained results showed that the primary improved components were in the order of CON 1, TURB 1 and VAP 2 for the conventional analysis, as shown in the schematic, while for the advanced exergy analysis, the order of improvement was CON 1, CON 2 and PRE-HE 1. From the study, the conventional exergy analysis results were found to be more qualified than the conventional exergy analysis. Following the improvements made to the system, the modified exergy efficiency increased to 18.26%. However, the total system efficiency was found to be about 9.60% in the real conditions.
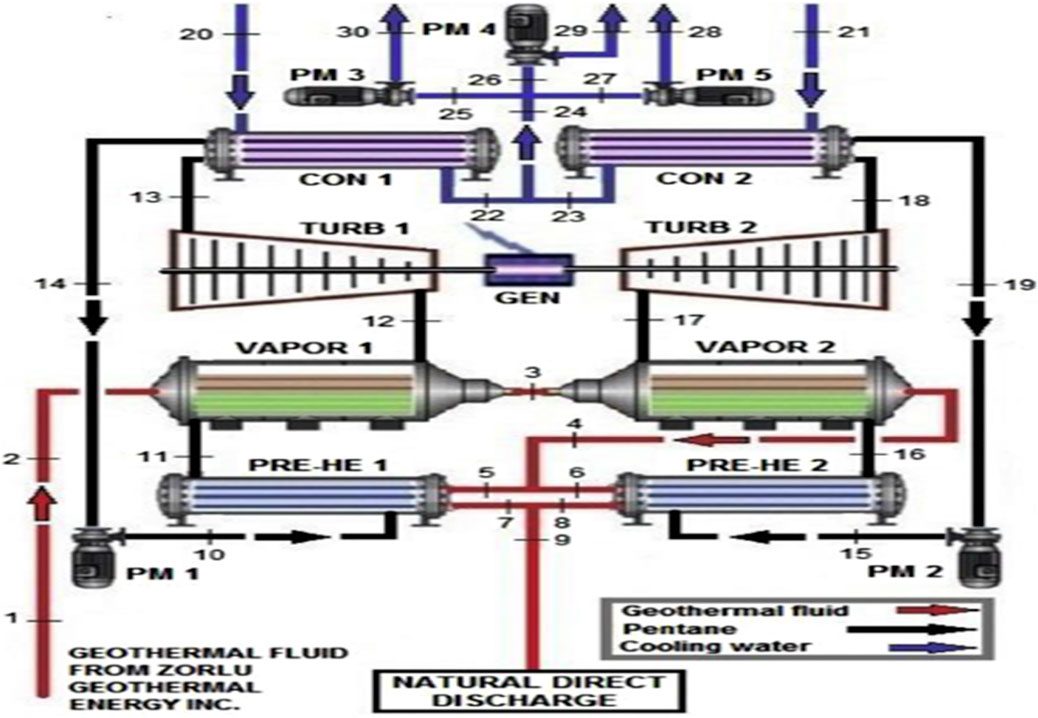
Figure 6. Geothermal binary power system for power generation (Keçebas and Gokgedik, 2015).
2.1.3 Combined cycle power plant
Recently, attempts to advance the capacity and effectiveness of simple gas turbine power generators were carried out through different combined heat and power cycle (CHP) methods (Ahmadi et al., 2011b). For instance, Boonnasa et al. (2006) improved a mixed cycle power plant in Bangkok using a steam absorption chiller to chill inlet air to 16°C and 100% humidity. This gives a 14% increase in the plant’s power output. Traverso and Massardo (2002) evaluated the function of four diverse combined cycle types of machinery. Humid air-water injection turbine was found to be more attractive than other technologies.
Moreover, some studies were focused on biomass-fuelled gas turbine systems for improved performance. For instance, Vera et al. (2011a) studied a CHP-gas turbine system with a capacity of 70 kWe and 150 kWth for an olive mill. Vera et al. (2011b) reported that there is higher thermal efficiency for the externally fuel gas turbine (EFGT) in comparison to the internally fuel gas turbine (IFGT) on a 30 kWe CHP system due to the extra required work for syngas compression in IFGT. Iora and Silva (2013) studied a biomass-fuelled 50 kWe CHP-EFGT and obtained an efficiency of 21%. Also, the CHP-EFGT system is fuelled by wastes from poultry, with a part at the turbine exit planned to dry the fuel and another for steam generation (Bianchi et al., 2006). For instance, biomass gasification fuelled on EFGT-Rankine combined cycle with steam injection and fog inlet cooling was investigated by (Athari et al. (2016a), Athari et al. (2016b). Besides, Gholamian et al. (2016) used a biomass-fuelled mutual cycle involving a conventional EFGT and a supercritical CO2 cycle. They reported an increase from 26.95% to 38.18% for exergy efficiency through EFGT combined with a supercritical CO2 cycle. Almutairi et al. (2015) observed the combustion chamber in a mixed-cycle power plant as the primary source of irreversibility due to poor air-fuel mixing and fuel oxidation. Therefore, adding an air preheater and reducing the air-fuel ratio were recommended to solve the cycle’s high exergy destruction. Doseva and Chakyrova, (2015) investigated the cogeneration system’s exergy efficiency of an internal combustion engine for use with biogas from a wastewater treatment plant. It was observed that the focal cause of irreversibility in the cogeneration plant emanated from the combustion chamber due to friction, temperature variation and chemical reaction.
Oko and Njoku (2017) explored the thermodynamic viability of upgrading an existing 650 MW combined thermal power plant (Figure 7) by adding an organic Rankine cycle unit. Energy and exergy methods were utilised to thermodynamically analyse the performance of the integrated gas, steam, and organic fluid-cycle power plant. The choice of organic refrigerant greatly enhanced the system’s performance.
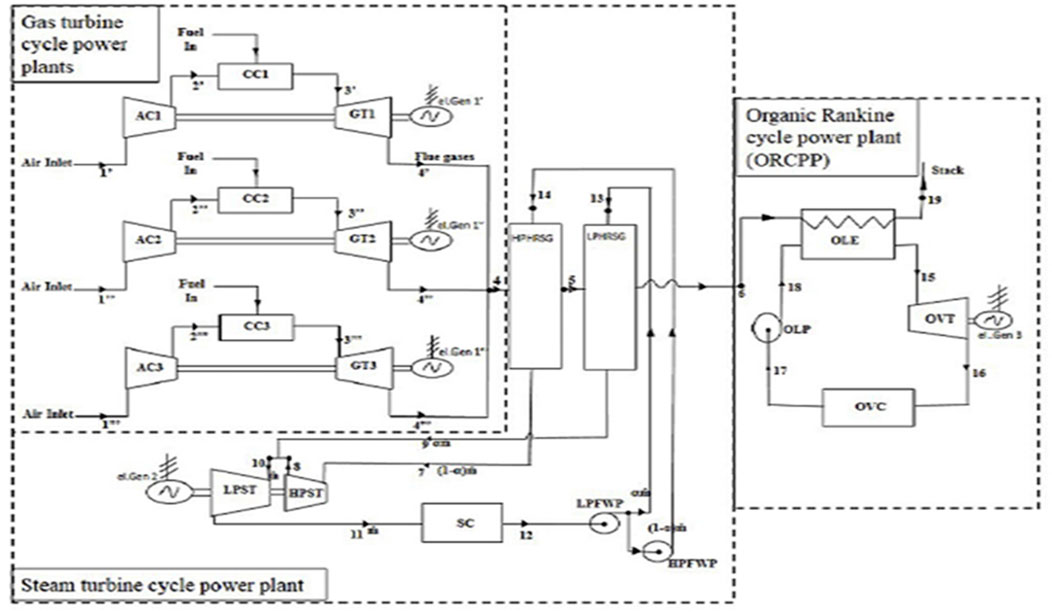
Figure 7. Schematic layout of Integrated Combined Thermal Power Plant with Organic Rankine Cycle (Oko and Njoku, 2017).
Manente, (2016) built a detailed off-design model of a 390 MW three-pressure level natural gas combined cycle (Figure 8) to assess the different integration schemes of solar energy, which keep the equipment of the combined cycle unchanged or include new equipment which comprises the steam turbine and heat recovery steam generator. Power-boosting and fuel-saving operation strategies were analysed to search for the highest annual efficiency and solar share. The results showed that without modifying the existing equipment, the maximum incremental power output from solar at design solar irradiance is limited to 19 MW. Depending on solar share and extension of tube banks in the heat recovery steam generator, high solar radiation-to-electrical efficiencies in the 24%–29% range were achievable in the integrated solar combined cycle. Compared to power-boosting, the fuel-saving strategy shows lower thermal efficiencies of the integrated solar combined cycle due to the efficiency drop of the gas turbine at reduced loads.
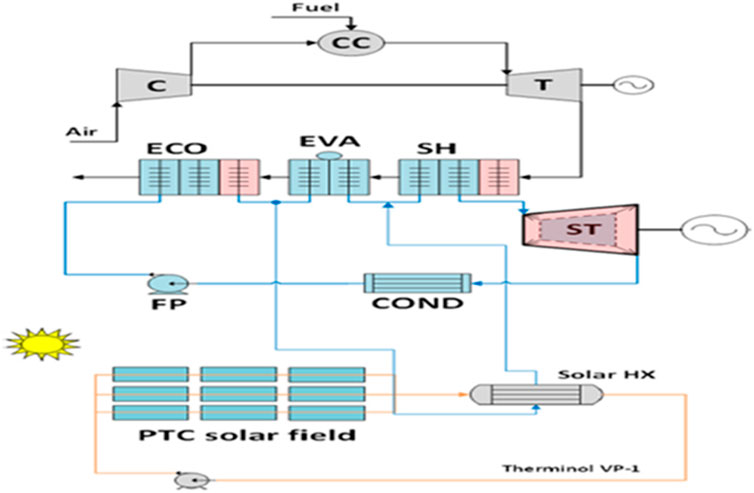
Figure 8. Schematic layout of integrated solar combine cycle (Manente, 2016).
Another form of integrated combined cycle is a biomass integrated gasification combined cycle (BIGCC). The BIGCC, as a power generation process, incorporates a biomass gasification system with a combined cycle power plant. This process is an attractive alternative for power generation compared to the conventional CHP processes due to high thermal efficiency and energy output, smaller production of greenhouse gases, and reduced generation of solid wastes. Moreover, A BIGCC process without carbon capture and storage (CCS) may contain four central operating units: an air separation unit (ASU), a gasifier, a syngas cooling and clean-up system, and a combined cycle power plant (Santos et al. (2016)]. Figure 9 shows a simplified scheme of a BIGCC process without CCS. Due to the promising benefits of BIGCC over the firing-based conventional power plants and coal-firing-based IGCC plants, several types of research from the second law of thermodynamics perspectives have been conducted to improve its performance. Bhattacharya et al. (Santos et al., 2016) investigated a 50 MWe BIGCC system integrated to supplement biomass firing. The study showed Second law (exergetic) efficiency of 36.86% for the entire power plant. Khanmohammadi et al. (Bhattacharya et al., 2011) studied integrating combined EFGT, ORC and biomass gasifier for CHP applications. Zhang et al. (Oyedepo et al., 2015c) used an IFGT-based CHP combined cycle powered by biomass gasification and coupled with a ground source heat pump. A Second law (exergetic) efficiency of 13.6% was obtained. A novel BIGCC based on IFGT, and oxy-fuel combustion was proposed by Xiang et al. (Khanmohammadi et al., 2019).
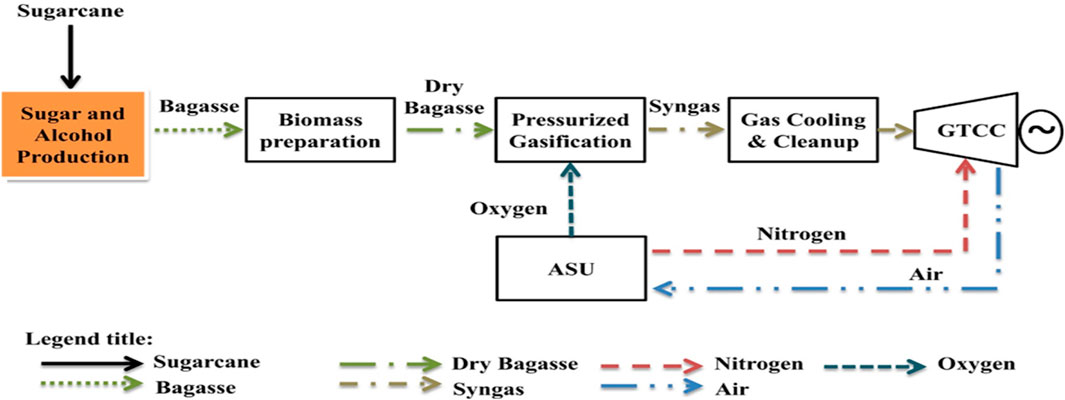
Figure 9. Simplified diagram of the Biomass Integrated Gasification Combined Cycle (B-IGCC) [Santos et al. (2016)].
2.1.4 Refrigeration systems
Refrigeration systems are widely used for cooling purposes in industrial and residential sectors. These systems have been recognised to consume a high proportion of energy. Because of climate change resulting from the greenhouse effect and depletion of the ozone layer many world unions have recommended decreasing climate effects by improving the system’s efficiency and changing the existing refrigerants with ozone-friendly and eco-friendly ones (Xiang et al., 2019; Bolaji, 2010; Gill and Singh, 2018).
Lugo-Leyte et al. (Borokinni et al., 2018) studied the refrigeration cycle’s energy and exergetic analysis via R134a as the operating fluid. The equipment’s irreversibilities, the temperature of the refrigerated spaced and the exergetic operation cost in function of the environmental temperature were reported. The energy and exergy analysis of R502, R404A and R507 was also investigated by Arora and Kaushik (Lugo-Leyte et al., 2013). They computed the exergy destruction, exergetic efficiency, coefficient of performance and efficiency defects in the system. Based on exergy concept, Ahamed et al. (Keçebas and Gokgedik, 2015) stated that the major parameters that affect a vapour compression system are evaporating and condensation temperatures, undercooling and compressor pressure. Kizilkan et al. (Arora and Kaushik, 2008) presented that major irreversibility happened in the compressor and then the condenser, the evaporator, and the throttling valve for diverse compressor frequencies. Similarly, the exergy study of plant refrigeration with R407C, R507 and R417A as the working fluids by varying the compressor speed was examined by Aprea et al. (Kizilkan et al., 2010). The result obtained indicated that the compressor contributed better to the overall irreversibility, while R407C performed better than R507 and R417A. The total energy and exergy performance of a domestic refrigeration cycle working with R413A is consistently better than that of R12 when the performance of R413A in an unmodified R12 system was evaluated by Padilla et al. (Aprea et al., 2003).
Kalaiselvam and Saravan (Padilla et al., 2010) also investigated the total exergy losses on different scroll compressors using R22, R417A and R407C as the working fluid. They suggested that R417A, being eco-friendly, can be used as an alternative to other refrigerants. Agarwal et al. (Kalaiselvam and Saravan, 2009) investigated the performance of mechanically subcooled simple vapour compression refrigeration systems based on energy and exergy analysis. Compatibility of alternative low GWP and zero ODP HFOs R1234yf and R1234ze were investigated to replace the HFC 134a. Results of the study revealed that R1234ze was the best alternate refrigerant considered in the analysis and can replace R134a as the COP and exergetic efficiency of R1234ze were 1.87% and 1.88% higher than that of R134a for 30°C of sub-cooling. On the other hand, R1234yf offered lower performance than R134a. According to the study, the condenser and evaporator components had the highest and lowest exergy destruction sites, respectively (Agarwal et al., 2021).
Al-Sayyab and Abdulwahid (Yataganbaba et al., 2015) used R1234yf, R1234ze, R245fa, and R227ea as alternative refrigerants to R134a for exergy study of ten vapour compression cycles. The study results revealed that the maximum no-reversibility among the system mechanisms happened in the compressor, followed by the throttling valve, flash tank, and condenser. At the same time, the evaporator possessed the least no-reversibility. R245fa possessed the least exergy destruction with high exergetic efficiency and high COP with respect to other refrigerants. Hence, this refrigerant has the potential to serve as an alternative refrigerant to R134a. From the study by (Al-Sayyab and Abdulwahid, 2019), R454B was found to be the satisfactory fluid for use in the ground source heat pump. Menlik et al. (Bobbo et al., 2019) presented the second law of thermodynamic analysis, the potential of R22, and its alternatives, R407C and R410A, in a VCRS. Results of the study revealed that R407C was a better alternative to R22 than R410A. The highest exergy-destroyer component of VCRS was the condenser. Gill and Singh (Menlik et al., 2013) experimentally inspected a vapour compression refrigeration framework’s thermodynamic performance utilizing a combination of R134a and LPG refrigerant as a swap for R134a. From the investigation, the proposed combination of R134a and LPG performed in a way that is better than R134a from insightful exergy and energy examinations.
2.1.5 Organic Rankine Cycle (ORC) and Kalina Cycle (KC)
Organic Rankine Cycle (ORC) and Kalina Cycle (KC) are new energy conversion technologies. These technologies convert low-temperature waste heat from industries (or solar energy sources) into useful energy (heat or power) (Kim et al., 2013). Based on the desire for effective energy utilization and preservation of the environment from pollution, various researchers have worked on performance improvement and economic assessment of ORC and KC.
Gill and Singh, (2017) and Kim et al. (2012) investigated the energy and exergy system performance characteristics of the ammonia-water Rankine cycle using low-temperature heat sources and ammonia-water mixture heat exchangers with and without regenerator. Similarly, Kim et al. (2012) reported the pinch point characteristics in heat exchangers and ammonia-water-based power cycle condensers. Moreover, studies on integrating ORC/KC with other heat sources were studied. For instance, (Lolos and Rogdakis, 2009; Kim et al., 2014) studied a Kalina cycle’s performance with solar energy as a heat source. Ogriseck, (2009) analysed the integration of Kalina power in a combined heat and power plant in Germany.
Ogriseck, (2009) compared and analysed the Kalina Cycle’s performance and bottoming transcritical organic Rankine cycle in the cryogenic cogeneration system for engine exhaust heat recovery. Yue, et al. (2015) and Sun et al. (2012) carried out energy, and exergetic analysis for solar-boosted KC with an auxiliary superheater to utilise low-grade heat sources. Sun et al. (2014) investigated a 150 kWe EFGT system fuelled by a downdraft gasifier and combined with an ORC to maximise the electrical energy generation. They reported an electrical efficiency, generated power and biomass consumption of 20.7%, 200 kWe and 217 kg/h. Vera and Jurado, (2018) compared ORC and KC’s low-temperature waste heat recovery performance. At the expense of higher pressure, KC generated more power with higher efficiency.
Furthermore, an investigation on KC driven by low-grade geothermal energy integrated with thermoelectric generators to convert heat into electricity directly has been carried out (Varga and Palotai, 2017). The study enhanced the net power by 7.3%, with efficiencies comparable to the conventional KCs. Zare and Palideh, (2018) developed a novel Organic Rankine cycle (ORC) (see Figure 10). The ORC system’s thermodynamic performance was investigated to study the system’s operational improvement. The study results indicated that ORC integration and intercooled cycle gas turbines could recover waste heat. From the study, the maximum enhancements of output power and thermal efficiency were 6.08% and 2.14%, respectively. Based on the outcome of the investigation, it was established that both room temperature and operating conditions of gas turbines are principal factors influencing the operating performances of the ORC system. Koç et al. (Liu et al., 2018) performed energy, exergy, and parametric analysis on the Organic Rankine Cycle (ORC) with a simple and recuperative ORC case study using a gas turbine-based combined cycle. The study showed high power output at quasi 9 MW for simple ORCs that employed methanol and recuperative ORCs using trans-2-butane as working fluids. Recuperative ORC was observed to have the potential for reduction in CO2 emission.
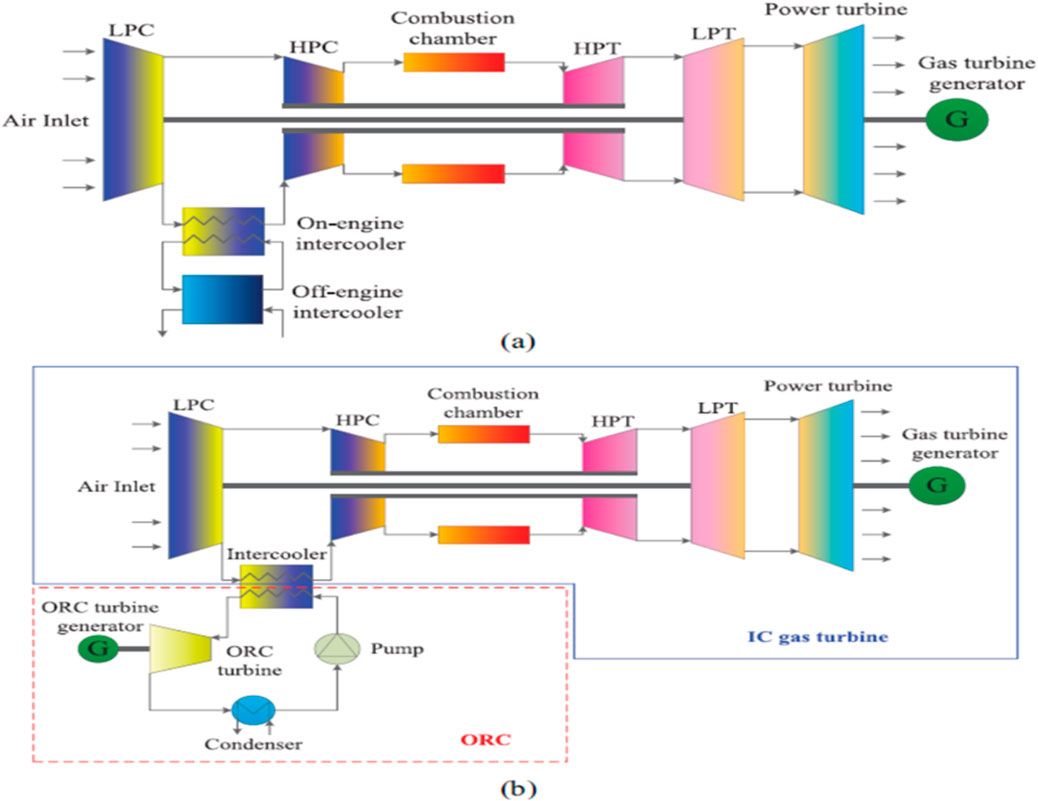
Figure 10. Schematic diagram of the IC gas turbine (A) without and (B) with ORC power generation system (Zare and Palideh, 2018).
2.1.6 Renewable energy systems
Renewable energy systems are gaining users’ attention as an incomplete or total substitution of fossil fuel-based sources. Numerous studies have been conducted on using renewable sources to replace fossil fuels. The superior substitutes are solar and biomass energy, which replace power generation’s thermal energy because of their carbon-neutral nature and environmental beneficence (Koç et al., 2020). Moreover, these renewable energy resources are easily accessible to humanity worldwide and also available in abundance (Seyam et al., 2020). In his study, Hepbasli, (2008) presented a comprehensive review of exergetic analysis and performance evaluation of different renewable energy resources (RERs). The RERs studied are solar, wind, geothermal, and biomass energy systems. The paper concluded that such a study is of great importance and relevant to engineers and scientists applying the second law of thermodynamics in the design, simulation and performance improvement of RERs.
This section presents a thermodynamic performance assessment of the selected renewable energy systems based on energy and exergy analyses. The focus is on integrated renewable energy sources with the conventional energy system.
2.1.6.1 Integrated solar energy system
Solar energy is a form of renewable energy that converts the sun entering the earth’s surface into heat or electricity. Integrated solar-assisted polygeneration systems have emerged as an effective and sustainable alternative for meeting thermal, cooling, power and freshwater demands (Khani et al., 2022). Because of this, numerous studies on integrated solar-assisted plants with conventional thermal power plants have been carried out (Alta et al., 2010; Oztop et al., 2013; Akyuz et al., 2012). Assareh et al. (2023) proposed an innovative power generation system based on solar and biomass energy resources. The system consists of a biomass unit, a solar unit, a waste heat recovery unit, and a hydrogen liquefaction unit. The power was generated by two gas turbines and two Rankine cycles. Results of the study showed that solar energy with waste-heat recovery reduces CO2 emissions by 30.5% while increasing the system’s electrical power output by 44%. Roshanzadeh et al. (2023) conducted a study focusing on utilizing solar cooling systems to achieve low inlet air temperature and generate high electricity yields. The study simulated the thermodynamic behavior of a combined cycle power plant with integrated solar-driven inlet air cooling for Tehran, Phoenix, and Houston during warm-hot seasons. Results of the study revealed that a considerable reduction in the output power was realized during hot ambient conditions due to the lower density of the air and lower mass flow rate to the turbines. The output power decreased from 306.6 to 260.8 MW as the ambient temperature increased from 15 to 45°C.
Moreover, Ghorbani et al. (Ratlamwala et al., 2011) developed and analysed an integrated system capable of producing 65.2 MW of heating, 1.87 MW of power and 83.2 kg/s of freshwater. Servam et al. (Koç et al., 2020) assessed the performance of a novel integrated large-scale combined cycle power plant (CCPP). This system depends on renewable sources such as solar radiation and seawater from the Atlantic Ocean to provide clean and sustainable energy. The integrated power plant consists of six subsystems: solar farm, Gas turbine cycle, Rankine cycle, multi-effect desalination, electrolyser, and hydrogen liquefaction subsystem. The combined cycle was studied thermodynamically to investigate thermal and exergy performance. The integrated system’s overall thermal efficiency was 88.12%, while the exergetic efficiency was 23.05%. The combined system has the energy cost of 14.59 $/MWh, which was found to be economical due to the system’s multiple services. Also, the emissions were significantly low, making this power plant environmentally benign. Alibaba et al. (Ghorbania et al., 2018) developed an optimal thermodynamic, exergo-economic and exergo-environmental design for the geothermal power plant used as a complement to concentrated solar power (CSP) and then combined energy-exergy-economic-environmental analysis was conducted. In the study, a standalone geothermal cycle and hybrid Geothermal-Solar cycle were investigated to generate the heating/cooling power. The study revealed that the exergo-economic analysis of the hybrid power plant has the highest investment cost when related to the solar power plant. It also had the lowest exergy degradation cost with zero environmental impact. The highest environmental rate was associated with the solar power plant. However, its environmental destruction rate was minimal because it does not consume fuel. Sarhaddi et al. (Alibaba et al., 2020) did an exhaustive energy and exergy investigation to assess a commonplace PV cluster’s electrical performance, componentwise exergy destruction, and exergy efficiency. The consequences of the investigation demonstrated that the PV exhibit temperature greatly affects the exergy efficiency. The investigation reasoned that a PV cluster’s exergy proficiency could be improved if the heat could be eliminated from the PV array surface.
Yuanyuan and Yongping (Sarhaddi et al., 2009) presented a thermodynamic and economic analysis for an integrated solar combined cycle (ISCC) system with two pressure-level DSG solar fields (ISCC–2DSG) (Figure 11). The impacts of solar multiples on the system’s performance with or without consideration of thermal storage were studied. In that order, the solar thermal energy produced from two solar fields in the ISCC–2DSG system was only used to supply latent heat for low and high-pressure water vaporisation. The analysis characterised several such ISCC–2DSG systems’ annual thermodynamic performances, using different solar multiple values but identical design parameters in the power subsystem. The results showed that the capacity factor increases with a more significant solar multiple due to more outstanding electricity production for ISCC–2DSG systems without thermal storage. The capacity factors were more substantial than those without thermal storage for the systems with thermal storage, following the effective utilisation of the surplus solar thermal energy generated. The results concluded that the system’s levelized electricity cost with thermal storage was considerably higher for a given solar multiple than without thermal storage.
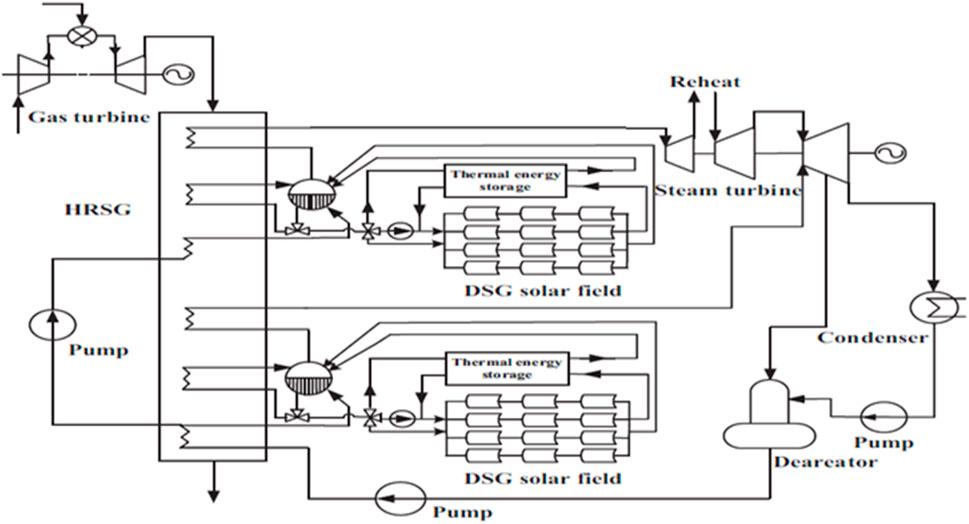
Figure 11. Schematic diagram of an ISCC system with two DSG solar fields with thermal storage (Sarhaddi et al., 2009).
Al-Ali and Dincer (Yuanyuan and Yongping, 2015) proposed a thermodynamic analysis of a multi-generation integrated geothermal-solar (GS) system that consists of the geothermal-solar cycle, organic Rankine cycle 1, organic Rankine cycle 2 and a single absorption chiller that produces electricity, space heating, hot water, industrial process heat and cooling. Results obtained show the energy efficiencies for single-generation and multi-generation systems were 16.4% and 78%, respectively. The exergy efficiencies yield 26.2% and 36.6%, respectively. Adibhatla and Kaushik (Al-Ali and Dincer, 2014) performed energy, exergy and economic analyses of a conceptual power plant cycle formed by adding solar energy to the steam cycle of a natural gas-based combined cycle power plant (Figure 12). The solar integration idea was made at a moderate temperature level using a direct steam generation technique with parabolic trough collectors. The simulated results showed that the solar field’s energy and exergy efficiencies were 53.79% and 27.39%, respectively. The results have indicated that the plant output was increased by 7.84%, with the solar field operating at the design point for a 50 MW nominal solar field-rated capacity. The results showed that the levelized cost of electricity generation decreased from 7.4 to 6.7 cents/kW.
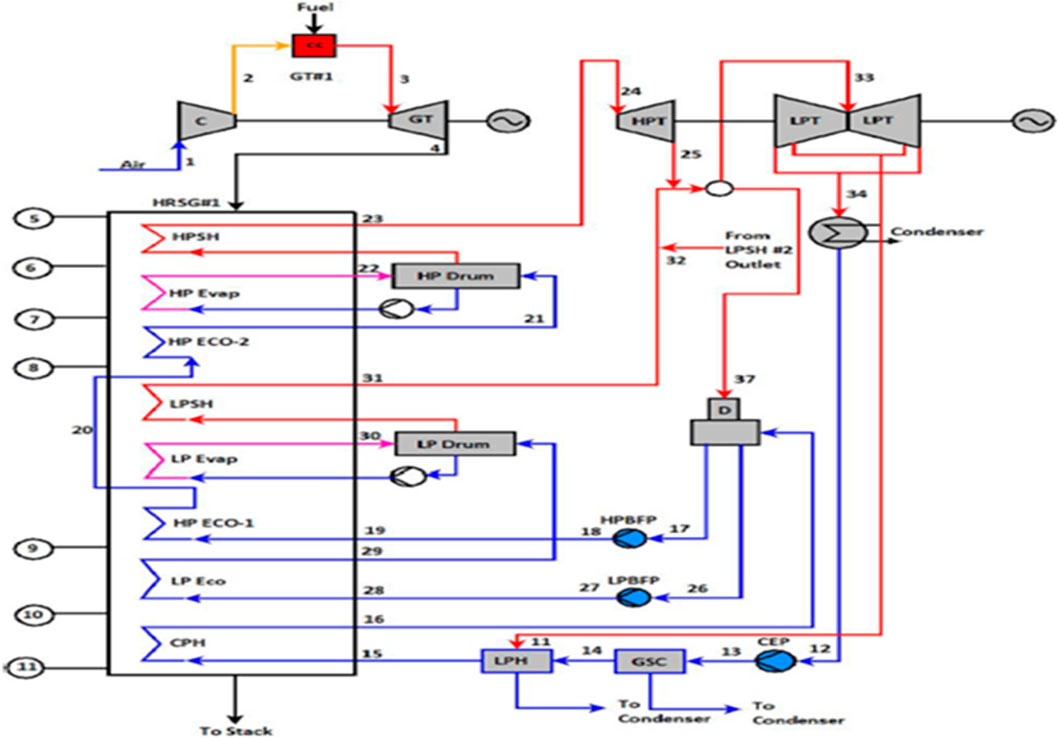
Figure 12. Integrated solar direct steam generation combined cycle power plant (Al-Ali and Dincer, 2014).
2.1.6.2 Integrated biomass energy system
Reducing natural resources for fossil fuel has shifted attention to sustainable renewable energy integrated systems (Gholamian et al., 2016). The economic policy international bodies support research into utilizing renewable energy to minimize environmental pollution posed by fossil fuel. Biomass is being explored as renewable energy. With the increase in the depletion of fossil fuels and greenhouse gas (GHG) emissions, highly efficient energy utilisation systems have drawn increasing attention, especially renewable and sustainable energy integration systems (Gholamian et al., 2016). The current global energy policies promote research to enhance the utilisation of renewable energy sources, largely to minimise environmental problems and improve the national energy security of countries dependent on the use of imported fossil fuels. Biomass is currently one of the most popular renewable energy sources. This is because biomass has great potential as a clean, renewable feedstock for producing modern energy carriers (Adibhatla and Kaushik, 2017).
Buentello-Montoya and Zhang (2019) analysed the thermodynamic efficiencies of biomass gasification from char-activated char catalysts. The work introduced an equilibrium model for the thermodynamic analysis and assessed the effect of the reactor temperature, reaction time and equivalence ratio based on the gas quality. The result showed that there is a thermodynamic advantage and reduction in exergy destruction due to high reforming temperature. The result is in tandem with Echegaray et al. (Adibhatla and Kaushik, 2017) as the exergetic efficiency of the gasification process lessened when all measured working parameters were increased.
There was also the attempt to generate electricity through a combination of solar heat and biomass-fired power systems (Khalid et al., 2015). Thermodynamic performance of the hybrid solar heat–biomass-fired plant was carried out, which resulted in an improved efficiency of 20%. The exergetic conversion efficiency of the combined heat power plant was estimated to attain 21% (Buentello-Montoya and Zhang, 2019) (Figure 13). A cost assessment of the system was investigated for a case study considering a typical apartment block on a Greek Island, assuming Parabolic Trough Collector (PTC) area of 50 m2. The savings in fuel oil and electricity consumption accounted for an Internal Rate of Return (IRR) of around 12%, with a payback period of 7 years (Asim et al., 2020).
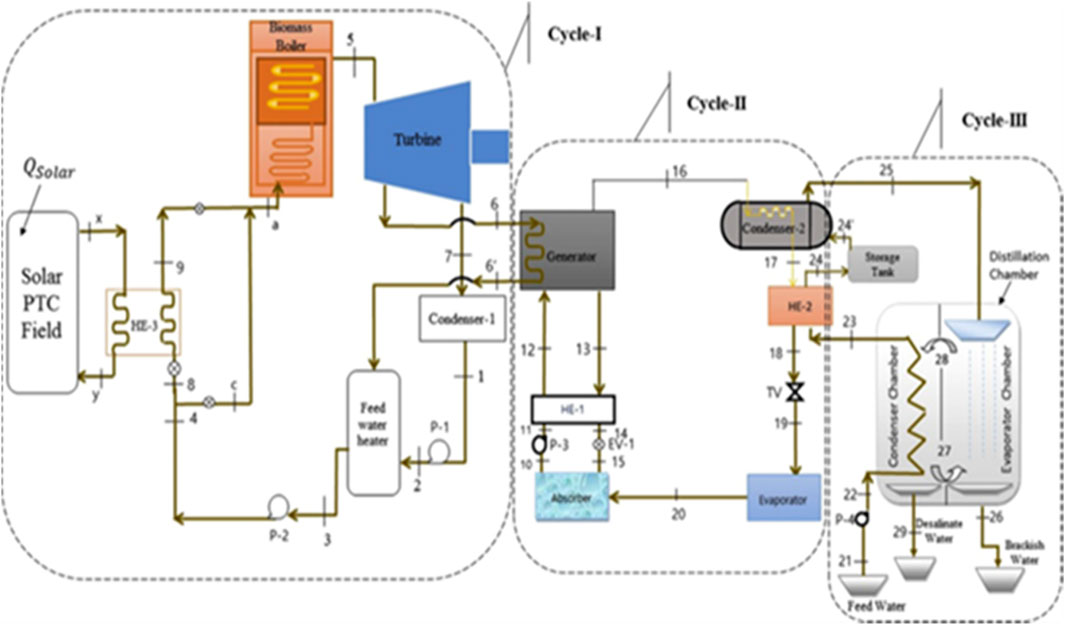
Figure 13. The combined ORC–VCC cycle and biomass boiler and PTC heating water circuits (Buentello-Montoya and Zhang, 2019).
A thermodynamic evaluation and optimisation of a hybrid solar-biomass (HSB) system in a polygeneration process for combined power, cooling and desalination (Figure 14) was investigated by Sahoo et al. (2017) purposely to identify the effects of various operating parameters. Primary energy savings (PES) of the polygeneration process in the HSB system was achieved to 50.5%. The energy output from this system was increased to 78.12% compared to that of simple power plants (Chen et al., 2020).
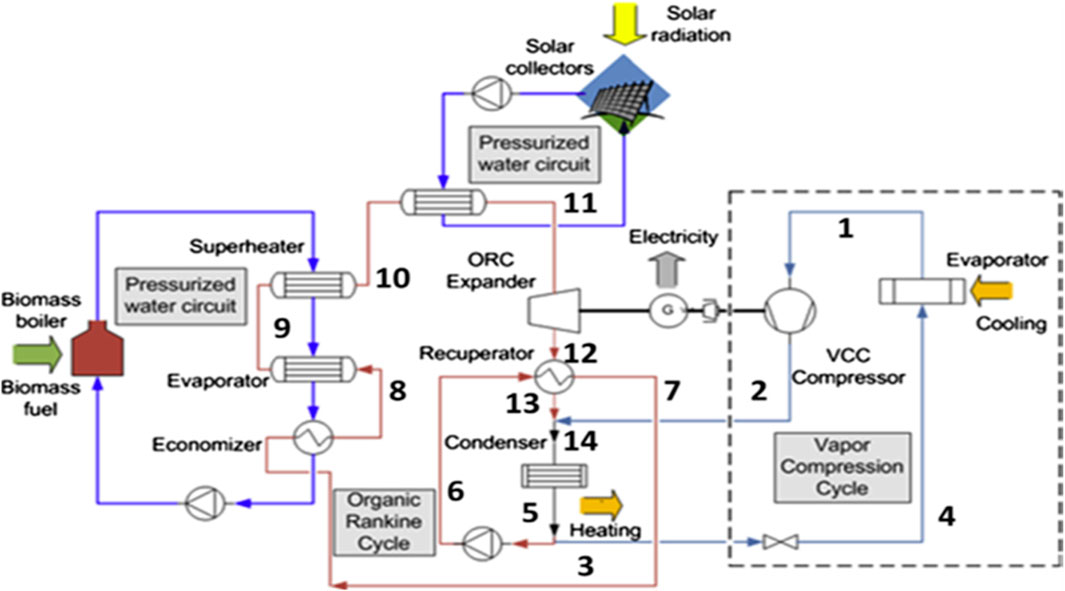
Figure 14. Schematic diagram of hybrid solar-biomass power plant with cooling and desalination in polygeneration process (Karellas and Braimakis, 2016).
2.2 Thermodynamic optimisation of energy systems
In order to improve the performance of an energy conversion system, thermodynamic analysis of energy systems offers a comprehensive, systems-based approach that offers a methodological scientific framework for arriving at realistic, integrated solutions to complex energy systems problems. Modern energy systems are evolving quickly and exhibit increasingly complex features. This is mostly because modern energy systems have other purposes in addition to energy conversion and supply. Aside from providing energy, energy systems should also secure their resources to ensure long-term operation, minimize their effects on the environment and ecology during construction and operation, provide energy at a reasonable cost to maximize benefits to a larger population, and help mitigate carbon emissions that contribute to global warming. In an effort to meet some of the aforementioned goals, new energy technologies are always developing. With the implementation of these technologies, renewable energy has been increasingly exploited and used, the energy efficiency of conventional power generation has been continuously improved, and energy end-consumers have become more energy-efficient and environmentally benign (Sahoo et al., 2017). According to Benja et al. (Kopanos et al., 2017), a thermodynamic optimization aims to minimise the thermodynamic inefficiencies: exergy destruction and loss in energy system components.
Many researchers have contributed valuable publications on the thermodynamic optimisation of a variety of energy systems via hybrid systems or waste heat recovery technology. These endless sustainable energy technologies can reduce fossil fuel consumption and decrease carbon emissions when its operations are optimised and controlled. Vera et al. (Sun et al., 2014) reported a value of 15.6% for exergy efficiency at base case operating conditions, which was increased to 18% by optimisation. They also reported a parametric optimisation result for a pilot system that generates power, exergy and energy efficiencies reaching up to 491 kW, 35.6% and 6.48%, respectively. Not too long ago, Prananto et al. (Bejan et al., 1996) investigated the electricity-generating performance of a KC by recovering the heat of the unused brine discharged from a geothermal power plant. In an optimised condition, 48 kg/s of unused brine was reported to generate up to 1.66 MW of electricity. In addition, Ghaebi et al. (Prananto et al., 2018) proposed and assessed geothermal power by combining KC with an ejector refrigeration cycle. In optimised conditions, the cycle has the potential to produce 2.3 MW of net power and 1.1 MW of refrigeration. Ahmadi et al. (Ghaebi et al., 2018; Ahmadi et al., 2015) optimised a transcritical CO2 power cycle operated by a geothermal energy source with LNG as its heat sink and an irreversible Carnot refrigerator. Ahmadi et al. (2016a) also studied the exergetic and sustainable multi-objective optimisation on a nano-scale Braysson cycle operating with Maxwell–Boltzmann gas. A solar-geothermal combined cooling, heating and power plant, becoming more popular as an efficient alternative to fossils, was optimised using multiple criteria by Boyaghchi and Chavoshi (Ahmadi et al., 2016b). Boyaghchi and Sabaghian, (2017) also wrote a similar work optimising the Kalina power cycle on a parabolic trough solar collector. Ahmadi and Dincer (Boyaghchi and Sabaghian, 2016) also optimised the cogeneration of a combined cooling, heating and power plant using exergo environmental principles through a Multimodal Genetic Algorithm. Ahmadi and Dincer, (2010) made significant contributions to the multi-objective optimisation of gas turbine power plants for increased efficiency of the thermodynamic system. Further studies by Barzeger et al. (Ahmadi and Dincer, 2011) focused on a closely related study investigating the multi-objective optimisation of a gas turbine power plant with a preheater using evolutionary algorithms. Shamoushaki et al. (Barzegar- Avval et al., 2011) built on this work by applying multiple objective optimisations of gas turbine power plants in Aliabad Katoul by evolutionary algorithms to improve efficiency.
3 Thermoeconomic assessment of energy systems
Economic studies of an energy system allow using costing equations, elaboration of estimation and optimisation algorithms, cost optimisation and low-cost energy systems and operations for thermoeconomic evaluation (Ahmadi and Dincer, 2011; Shamoushaki et al., 2017; Palazzo, 2013). Thermoeconomic studies, on the other hand, provide a balance between system efficiency and cost. Therefore, in designing and evaluating an energy system, the oil price, yearly equipment purchase cost (EPC), and functioning and management are valuable indicators for economic benefit analysis of energy systems by assessing several energy generation devices (Khanmohammadi et al., 2019; Goncalves and Arrieta, 2010; Boukelia et al., 2016). Hence, thermoeconomics is the art of saving natural resources that connects engineering and costing accounts through the Second Law of Thermodynamics. Energy systems are obtained from a set of sub-assemblies that network with one another environment and consume external resources that are transformed into products (Bakhshmand et al., 2015). Almutairi et al. (Valero and Cuadra, 2002) stated that the thermos-economic impact analysis of some power plants shows that the cost of destroying exergy is reduced. The suitability of thermo-economic study ranges to alternative energy areas of usefulness, such as heat engines, dewatering plants, refrigeration systems, etc. (Almutairi et al., 2016; Esen et al., 2007).
3.1 Thermoeconomic analysis and optimisation of energy systems
Thermoeconomics combines thermodynamics with principles of analytical accounting, and the main aim is minimising cost. In contrast to typical fiscal study, thermoeconomic provides a choice to examine and advance the operation of individual parts in the energy system (Taner, 2015). Hence, thermoeconomic appeared as a vital instrument to optimise the work of energy systems from both thermodynamic and economic perspectives. In thermoeconomics, the individual cost of part and stream is connected to its exergy subject. Capital expenditure of the mechanisms and the ecological influence are considered methodically for thermoeconomic optimisation of energy systems (Jamil et al., 2020). For energy transformation operations, accounting as a practice studies unit costs as it relates to energy. In view of this, several authors have suggested that budgets are preferably spread amid turnouts if management accounting is built on the thermodynamic exergy quantity. This is justified because exergy, but not energy, is often assigned economic value (Kopanos et al., 2017).
Numerous research studies on the relationship between thermodynamic (exergy) and economics analysis to evaluate quality output and optimisation of energy systems are stated in the literature. Pellegri (Dincer and Rosen, 2007) carried out a thermoeconomic and environmental study on sugarcane and ethanol for electricity generation in cogeneration plants. It was observed that a reduction in entropy resulted in a more efficient cogeneration operation, a reduction in exergy destruction and a better thermoeconomic and environmental operation. Oyedepo et al. (Pellegrini and de Oliveira Junior, 2011) conducted exergy estimation and quality assessment of carefully chosen gas turbine energy stations in Nigeria. From the study, the unit cost of electricity produced in the carefully chosen power plants varied from cents 1.98/kWh to cents 5.66/kWh. The result confirms that the combustion chamber had the highest exergy destruction cost in comparison to other components, as further confirmed by (Oyedepo et al., 2015a). Imran et al. (Athari et al., 2015b) introduced the thermo-economic improvement of fundamental ORC and regenerative ORC for waste heat recovery systems at consistent heat source conditions. In the examination, optimization was performed for five distinctive working fluids. The after-effects of the examination demonstrated that R245fa was the best working fluid under considered conditions, and basic ORC has a low explicit investment cost and thermal efficiency, which is in contrast to regenerative ORC. The sensitivity analysis revealed that evaporation pressure has promising effects on thermal efficiency and specific investment costs (Imran et al., 2014).
Bakhshmand et al. (Singh and Kaushik, 2014) investigated the thermos-economic analysis and optimisation of a triple-pressure combined cycle power station with one reheat stage (Figure 15). The total cost rate of the plate formed the optimisation objective function. The results showed that the optimisation process brought an increase of about 2.9% in energetic and exergetic efficiencies and a reduction of about 8.9% in the total thermos-economic cost.
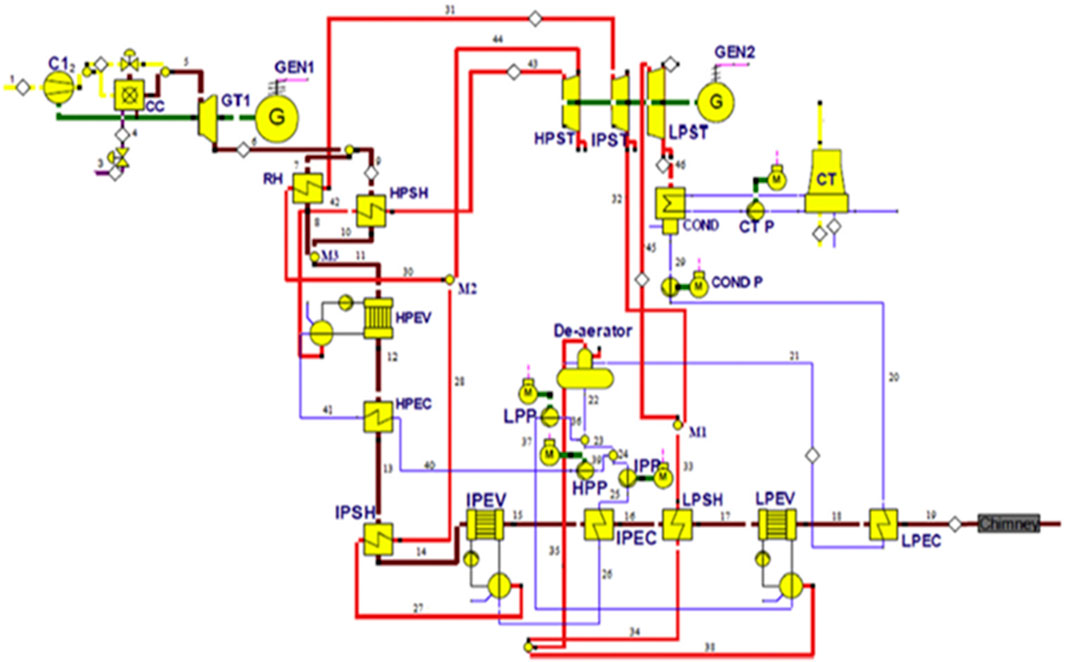
Figure 15. Schematic diagram of combined power plant with triple-pressure levels and one reheating stage (Singh and Kaushik, 2014).
4 Thermo-environmental analysis of energy systems
Recently, the environmental effects of unsustainable ways of energy consumption have been a serious concern, and the Second Law of thermodynamic analysis has been employed as an improvement method to (i) reduce atmospheric discharges and increase the life span of natural means through improving effectiveness and (ii) evaluate the probable influences of emissions. Thermo-environmental analysis combines the first two laws of thermodynamic examination and environment estimation to analyse thermodynamic efficiency and environmental impacts of energy systems components [Oyedepo et al. (Bakhshmand et al., 2015)]. The amalgamation of exergy and environmental studies unveils the interconnections among thermodynamic actions and environmental influences amid energy systems’ constituents (Oyedepo et al., 2018).
Environmental impact for energy utilisation results in climate change, acid rain and ozone depletion. Carbon dioxide emissions cause harmful environmental effects when the resources are used. In this regard, estimating carbon dioxide emissions is a substantial part of making an environmental assessment. At the point when the exergy efficiency, overall cost of the equipment and CO2 emissions are utilized for the improvement of mixed cycle power generation station with accompanying burning, the outcomes show that the cost of exergy destruction and losses diminished as the turbine inlet temperature expanded alongside burning compartment, the significant source of irreversibility (Keçebas and Gokgedik, 2015). The CO2 discharge could be limited by upgrading the efficiency of the components and bringing down the fuel rate of flow. Almutairi et al. (Granet and Bluestein, 2000) also stated that variation in ambient temperature demonstrates climatic circumstances about the location of a gas turbine. They further noted that the rise in ambient temperature reduces both exergetic efficiency and overall power outflow.
4.1 Thermo-environmental analysis and optimisation of energy systems
Research has shown that global warming is the utmost ecological task confronting the globe these days. Energy systems play a chief part in the emission of greenhouse gases. For example, about 21.2% of greenhouse gases are solely released by power stations (Oyedepo et al., 2015b). The need to understand the linkages between the first two laws of thermodynamics (energy and exergy) analysis and the ecological effect becomes more noteworthy due to the relations between exergy and the environment revealing the causal forms impacting environmental transformation. Recently, it has been shown that raising the exergy efficiency will reduce conditions for energy capacity and emissions. Moreover, exergy has been linked to ecological influence, as such a proportion from departing from the condition of a framework from the surrounding (Oyedepo et al., 2016; Baumgärtner and Arons, 2003).
The environmental analysis looks into the costs related to the movements of contaminants as well as the exergetic and economic costs of mass concentration and energy flows in energy devices (Jørgensen and Svirezhev, 2004). The rise of the exergy efficiency of these developments can decrease the environmental effect of energy transformation progressions. An increase in exergy efficiency will lessen the consumption of resources and consequently reduce the waste and the noxious discharge to the environment. This infers an enhancement in the ecological efficiency of these operations (de Oliveira, 2013).
Numerous studies related to the linkages between the first and second laws of thermodynamic analysis (exergy), and environmental impacts to assess the performance and optimisation of energy systems are reported (Pellegrini and de Oliveira Junior, 2011; Oyedepo et al., 2015b; Ahmadi and Dincer, 2010; Ahmadi and Dincer, 2011; Ameri et al., 2016). Owebor et al. (Amrollahi et al., 2011) carried out an energy, thermo-environmental and fiscal study for a projected municipal waste-propelled power station. The projected plant consisted of vaporization, solid oxide fuel cells, gas and steam turbines, absorption refrigeration, and organic Rankine cycles (Figure 16). Results of the study revealed that the fuel noxious release factor, explicit CO2 discharge and sustainability exponent were 0.00097, 148.23 kgCO2/MWh and 6.56, correspondingly. Moreover, the energy-economic sustainability exponent was shown, which considered the efficiency of the energy change progressions and its commercial effect on the people in terms of cost and social, economic state. Oyedepo et al. (2015b) presented an all-inclusive thermodynamic simulation and exergoenvironomic performance analysis for Nigeria’s designated gas turbine power station. In the study, the exergo-environomic parameters computed were carbon (IV) oxide emission in kg per MWh of power generated, sustainability index, consumption number, price flow rate of environmental effects in $/h and overall rate of cost of products in $/hr. Results of the study revealed that the combustion chamber was the utmost exergy-damaging section linked to other cycle components. However, the exergy ruin of this module is lessened by the growing gas turbine inlet temperature (GTIT). Moreover, it was observed that the thermodynamic inefficiency was responsible for the environmental power of gas turbine components. Hence, the study showed that CO2 discharges and the cost of environmental impact decreased with increasing GTIT.
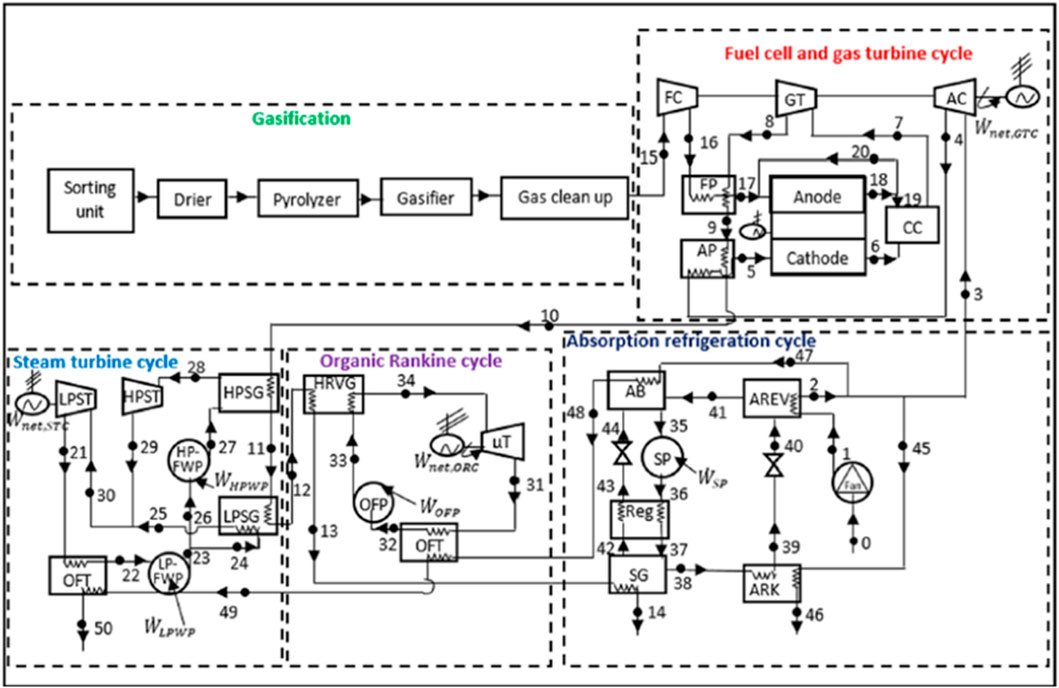
Figure 16. Schematics of an integrated gasification, SOFC, GT, ST, ORC and AR cycles (Amrollahi et al., 2011).
Ameri et al. (Jørgensen and Svirezhev, 2004) presented the exergoeconomic and ecological maximization of a selected huge steam generating station. The impact of additional air on exergy efficiency and noxious emissions were studied. Furthermore, the optimization process was presented by exergetic efficiency, standardized CO2 emissions of the plant, and three diverse cost functions, including electricity costs, environmental impacts, and total plant costs. Aftereffects of the study revealed that the cost of electricity generation and the cost of environmental impacts diminished by 20.25% and 49.6%, respectively, at the optimum operating conditions of the plant. A thorough thermoeconomic and thermoenvironomic modeling and analysis of selected gas turbine power plants in Nigeria were presented by Oyedepo et al. (Pellegrini and de Oliveira Junior, 2011) utilizing the first and second laws of thermodynamics (exergy) principle. The consequences of the study uncovered that CO2 emissions varied somewhere in the range of 100.18 and 408.78 kg CO2/MWh for the selected power plants, while the cost rate of the green effect varied from 40.18 $/h to 276.97 $/h. It was additionally shown that CO2 discharges and the consequence of environment influence diminished with growing GTIT. Meanwhile, the sustainability index of the plants increased with increasing GTIT.
Maraver et al., (2014) showed the thermodynamic streamlining of ORCs, combined alongside absorption or adsorption refrigeration components, for consolidated CCHP production from biomass burning. In the study, system modelling with the prime objective of proffering optimization procedures with the condition of operation of such systems was carried out. Furthermore, the energy and ecological execution of the distinctive ideal CCHP machine were explored. The study established that the trigeneration plant is capable of being designed in an energy-environmentally effective route with an n-pentane restorative ORC and a volumetric sort expander. Ahmadi et al. (Keçebas and Gokgedik, 2015) investigated exergoeconomic and environmental analyses for a mixed cycle and examined the impacts of additional burning on the effectiveness of bottoming cycle and CO2 discharges. Memon et al. (2015) performed parametric-based thermoenvironmental and exergoeconomic analyses of a combined cycle power plant. Abam et al. (Memon et al., 2015) proposed an in-service turbine gas device, burned by gas to be modified with a steam turbine (ST), organic Rankine cycle (ORC), gas turbine (GT) for refrigeration and electricity generation, an adapted Kalina cycle (KC) for electricity development and refrigeration, and a vapour absorption system (VAS) for refrigeration (Figure 17). The integrated multi-generation plant (IMP) was to be burned by biomass-based syngas as a supplementary firing source. Exergoeconomic and environmental analysis was carried out on the IMP. The after-effects of the study demonstrated that the planned system could propel the existing energy evolution predicament in the energy sector of Nigeria and workable energy approach to match the Agreement of Paris and Sustainable Development Goals (SDGs) strategy.
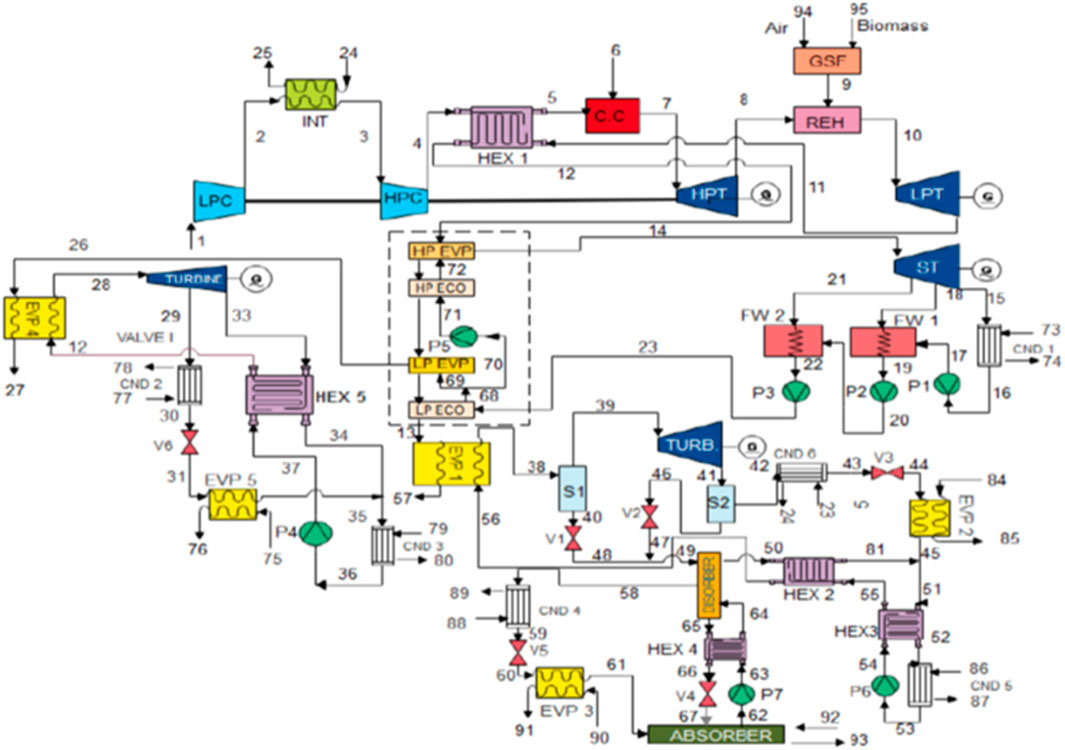
Figure 17. The proposed integrated multi-generation plant (Memon et al., 2015).
5 Assessment of sustainability indices (indicators) of energy systems
One of the fundamental ideas of sustainable development is sustainable energy development (SED). It ensures affordable, accessible energy for all while adhering to social and economic development requirements and the environment. Increasing the share of alternative energy sources in the energy mix, such as renewables, improving energy efficiency, and lowering greenhouse gas and air pollution emissions, are some of the actions that help implement the SED (Abam et al., 2020a). The evaluation criteria for the energy system’s sustainability must consider the resource, environmental, social, and economic facets.
The process of converting energy from sources like fossil fuels and renewables into easily utilised forms, like electrical energy, propulsive energy, heating, and cooling, is known as energy conversion. The primary goal of the energy conversion systems sustainability evaluation is to ensure that options have been thoroughly considered and assessed for their potential short- and long-term impacts on the environment, economy, society, and other factors (Deng et al., 2022). In other words, when new and innovative energy systems are introduced for transportation, electric power generation, or industrial operations, it is prudent and essential to conduct a thorough sustainability assessment before moving forward with the execution of these innovative concepts. This is so because sustainability refers to an energy supply that is affordable, readily available, and likely has little to no impact on the environment (Chamchine et al., 2022).
Establishing a strong and comprehensive set of indicators to track the advancement of sustainable energy development is now essential, as sustainable development has made sustainable energy development a key goal of international policy. United Nations Sustainable Development Goal (SDG 7) calls for support in ensuring everyone can access affordable, clean energy (UN, 2015). This demonstrates the dedication to clean energy and sustainability. Affordable and sustainable electricity is essential for the growth of business, communications, agriculture, healthcare, education, and transportation. Energy constraints impede human development and economic growth, especially in developing countries.
Setting up various suitable performance indicators is a prerequisite for measuring the effectiveness of energy conversion systems. A mix of thermodynamic, economic, and environmental indicators is considered to assess the technical, financial, and societal worth of the energy systems and guarantee that the chosen designs are well-suited to a sustainable development framework. Numerous general energy system performance indicators and new ones are included in the performance indicators below (Spelling, 2013). The sustainability indices of the energy systems listed below are examined based on the economic, environmental, and first and second laws of thermodynamics.
5.1 Enthalpic efficiency indicator (thermal efficiency)
Enthalpic change values (ΔH) are used to quantify “heat content,” which is how the indicator gauges the efficiency of the process inputs and outputs. For every process, the enthalpic efficiency ratio can be calculated by dividing the ΔH value of the process’s useable output by its inputs’ ΔH value. Equation 1 can be used to compute enthalpic efficiency (Patterson, 1996):
where,
ηΔH = enthalpic efficiency
For instance, a basic gas turbine has an enthalpic efficiency of roughly 33%. The enthalpic efficiency indicator evaluates the “useful” output. The conversion rate of chemical energy (ΔH) into electricity in this process is only 33%; the remaining 67% is lost as “waste” heat to the environment. When computing energy in enthalpic terms, the total output equals the total inputs if each activity’s “waste” output is combined with the “useful” output from all processes. This is essentially another way of saying the first law of thermodynamics, which states that energy cannot be generated or destroyed during any type of conversion. Because of this, enthalpic efficiency is frequently referred to as first-law efficiency.
5.2 Thermal discharge index (TDI)
The number of thermal energy units released into the environment for every unit of electrical energy generated by a plant is known as the thermal discharge index of the power system. The following can be used to express it in power units (Ofodu and Abam, 2002).
Every thermal power plant must have an index that is not zero to comply with the second law of thermodynamics; however, the index should be as low as feasible to maximize plant efficiency and maintain low pollution levels (Rajput, 2003; Labele-Alawa and Asuo, 2011; Abam et al., 2012). The thermal discharge index can be described as follows in terms of thermal efficiency, a crucial determinant of the index (Ofodu and Abam, 2002).
where Pth is the thermal energy input and
5.3 Overall exergetic efficiency (OEE)
Exergy plays a significant role in identifying efficiency improvements and decreases in thermodynamic losses related to energy conversion processes. By lowering energy losses, exergy efficiency initiatives can lessen their adverse effects on the environment. Naturally, minimizing irreversibility or exergy loss results in an energy conversion process’s most significant increase in exergy efficiency (Inoussah et al., 2017).
The overall exergy efficiency of an energy system can be calculated as the ratio of the total useful exergy output to the total exergy input. Equation 3 gives the expression for overall exergetic efficiency:
5.4 Exergy improvement potential (EIP)
An energy conversion system’s exergy improvement potential measures how much and how quickly the system could be optimized. It is a thermodynamic method that combines effectiveness and exergy losses to provide a more comprehensive parameter of the system’s performance (Rivero et al., 2004). By creating a hierarchy of the system’s components and identifying its essential points, the exergy improvement potential enables the application of measures where they will be most effective.
The system’s efficiency and exergy losses yield the exergetic improvement potential. Equation 4 is used to compute it (Hammond, 2004):
where ExIP is the exergetic improvement potential, ε is the exergetic efficiency (%), and I is the exergy loss or irreversibility rate. This is computed by using Equation 5
For a control volume at a steady state Equation 6 is used to compute, the exergetic efficiency
where the rates at which the fuel is supplied and the product is generated are denoted by ĖF and ĖP, respectively. ĖD and ĖL denote the exergy destruction and loss rates, respectively.
In any real energy conversion process (irreversible), exergy is degraded, and the exergy efficiency is consequently less than unity. According to Van Gool, (1992), the maximum improvement in the exergy efficiency for a process or system is achieved when
5.5 Environmental effect factor (EEF)
An indicator of the extent of environmental harm caused by waste exergy destruction is termed the environmental effect factor (EEF). The EEF is a crucial thermo-sustainability indicator as it indicates whether environmental damage results from the destruction of waste exergy. According to Equation 7, the EEF is defined as (Aydin, 2013; Abam et al., 2018):
5.6 Exergetic sustainability index (ESI)
When evaluating the energy system’s sustainability, the exergetic sustainability index (ESI) is a crucial objective parameter among the sustainability indicators. This index has values between the intervals of 0 and ∞. The higher efficiency of the energy system means a low waste-exergy ratio and low environmental effect factor; as a result, there is a higher ESI. Studies have indicated that enhancing exergy efficiency can reduce environmental impacts by reducing energy wastage. These procedures result in higher exergy efficiency and lower exergy losses within the bounds of exergy methods (Midilli and Dincer, 2009). For example, an excellent fuel economy enables the energy conversion process to support exergy-based sustainability since it lowers resource requirements and environmental implications. Hence, ESI helps to assess the capacity and effective utilization and preservation of energy resources.
The relation between exergy efficiency and exergetic sustainability index is given by Equation 8:
Where,
ηex is the exergy efficiency, and it is defined as the ratio of useful, final, value and input value, i.e.,
ESI is also defined as the extent of sustainability and can also be calculated as a reciprocal of the EEF and it is given by Equation 9 (Abam et al., 2018):
5.7 Depletion number
Reducing the damaging emissions into the environment from energy conversion systems (which burn fossil fuels) has been shown to improve system efficiency, extending the life span of the fuel resources and boosting sustainability. Researchers have established the links among sustainability, exergy efficiency, and environmental effects (Connelly and Koshland, 1997).
Studies have shown that efficient fuel consumption is characterized by a depletion number defined and computed by using Equation 10:
Where Ėdest is the exergy destruction, and Ėxin is the exergy input by fuel consumption. The relationship between the depletion number and the exergy efficiency is given by Equation 11:
According to Altayib, (2011), the sustainability of the fuel resource can be expressed by a sustainability index (SI) as the inverse of the depletion number and is computed by using Equation 12:
5.8 Environmental impact factor (EIF)
This factor indicates whether the exergy losses damage the environment. The environmental impact factor is defined as the inverse of the exergetic sustainability index and computed by using Equation 13:
A high environmental effect factor translates into a low exergetic sustainability index, while a low environmental impact factor translates into a high exergetic sustainability index. When evaluating the environmental impact and sustainability of energy processes, the exergy technique of study is a useful tool. According to research, combustion is a highly irreversible process with a small exergy sustainability index and low exergy efficiency when directly producing heat at a low temperature. As a result, it greatly impacts the environment and raises CO2 emissions (Gojak and Bajc, 2019).
5.9 Waste exergy ratio (WER)
The energy conversion system transforms energy from primary sources into conveniently used forms. During the conversion processes, some exergy is destroyed in the engine components, and some exergy is lost by hot exhaust gases that are discharged into the environment. Hence, the total waste exergy is calculated as the sum of both the destructed exergy and loss exergy of the system. For a given energy system, total waste exergy (WE) is evaluated using Equation 14, while waste exergy ratio (WER) is expressed as the ratio of the total WE to the overall exergy input, and it is shown in Equation 15 (Aydin, 2013):
5.10 Exergetic utility index (EUI)
The exergetic utility index (EUI) measures the extent of exergy resource utilization in an energy system regarding the same system’s net output. It is a function of combustion chamber efficiency, ηCC, net output, Ẇnet, exergy input, Ėxin and the exergy efflux to the environment, Ėxout. EUI shows the degree to which exergy of the resource input is used for work production. EUI is calculated using Equation 16 (Abam et al., 2020b):
The small values of EUI presaged poor process conversion, especially for multi-generation energy systems.
5.11 Exergo-thermal index (ETI)
The thermal effect of an energy system on the environment while converting energy is measured by the exergo-thermal index (ETI). Low ETI values can be attained by continually powering other low-heat bottoming cycles with high-temperature flue gas from energy conversion systems. The expression for ETI is given by using Equation 17 (Aydin, 2013; Abam et al., 2018):
where β is the thermal pollution factor (enviro-thermal conservation factor) and can be computed by using Equation 18:
Tref is the environmental temperature, and TFg is the temperature of the exhaust stream (fluid gases). Research has shown that the ETI increases with an increase in TFg; the low values of ETI are desired and connote less environmental impact (Abam et al., 2020b).
5.12 The specific energy costs (C′)
This is an economic–thermodynamic indicator, and it is defined as the ratio of total energy system costs (including all costs for investment and operation) C and the final energy demand FE (Klemm and Wiese, 2022). It is computed using Equation 19:
When the absolute cost of an energy system is considered for optimization purposes, the specific energy cost (ce) is expressed as the ratio of the average annual cost of energy produced (TCav) to the average annual supplied energy amount (Eav) during the life cycle of the energy system, expressed by Equation 20 (Su, 2020):
5.13 Consistency indicators for energy systems
By replacing non-renewable resources with renewable ones, energy consistency seeks to transform the energy production and use paradigm. Using renewable energy sources rather than fossil fuels is one well-known example. Moving from fossil fuels to renewable energy sources is the most common definition of energy consistency. Therefore, it is possible to view the share of renewables (SoR) as a suitable metric of energy consistency (Samadi et al., 2017). This is computed by using Equation 21:
An energy source cannot be used more than its rate of regeneration in order to be considered sustainable. In this case, the usable life tuse of materials utilized in an energy system in proportion to its regeneration time treg could be used to evaluate the system’s consistency. This can be obtained by using Equation 22:
In order to obtain a meaningful value of consistency energy indicator, all materials used within an energy system, for example, from the concrete in the foundation of a power plant up to the fuel consumption, must be taken into account.
6 Conclusions
This paper extensively appraises the thermodynamics, thermo-economic and thermo-environmental analysis and enhancement of energy conversion systems for sustainable development. Also discussed are several appropriate performance indicators applied by researchers for assessing the performance of energy systems. A combination of thermodynamic, economic and environmental indicators was considered to evaluate the technical, financial and societal value of energy systems and thus ensure that the selected designs fit well within a sustainable development framework. Based on the outcome of this review study, it can be concluded that:
• The sustainability of the energy conversion system can be enhanced by assessing exergy techniques. Based on the exergetic approach, as exergy efficiency is getting closer to 100%, sustainability is getting closer to infinity because the process is getting closer to reversibility, and the environmental effect is getting closer to zero as energy is transformed without any loss.
• By reducing energy losses, exergy efficiency initiatives can lessen their adverse environmental effects. These techniques result in lower exergy losses and higher exergy efficiency within the context of exergy approaches.
• It is evident that when exergy loss or irreversibility is kept to a minimum, a process or system will increase its exergy efficiency to the greatest extent possible.
• By presenting a hierarchy of the energy system’s components, the exergy improvement potential indicator allows for determining the system’s crucial points and applying measures where they will have the most significant impact.
• Sustainable energy development depends on the availability of affordable, reliable energy from environmentally friendly sources.
• The best methods for efficient use of energy resources, low energy production costs, and less environmental impact are provided by hybrid energy systems (i.e., integrating conventional and renewable energy systems) and adopting multi-generation technologies.
• Maintaining the current conventional energy systems, which rely on fossil fuels and thermal energy conversion, seriously impacts climate change and global warming. As such, if we are to fulfil and surpass sustainable development goals worldwide, a paradigm shift in the energy-producing sector is needed.
• While some performance indicators are appropriate for assessing different facets of energy sustainability, none can capture the total energy sustainability of energy systems. Therefore, using a single performance metric to optimize the energy process results in improbable outcomes. Multi-criteria techniques, which allow for a more comprehensive optimization and planning of sustainable energy systems, should be utilized to prevent this.
• Direct low-temperature heat production through combustion has a low exergy sustainability index and low exergy efficiency and is irreversible. As a result, it has a significant environmental impact and produces considerable CO2 emissions. If at all possible, such combustion processes ought to be reduced or prevented.
An energy-efficient system assures sustainable development. A deliberate improved system as a function of exergy analysis will usher in energy systems’ reliability, sustainability and cost-effectiveness. Hence, a study like this aids in evaluating the exhibition of energy transformation measures on a thermodynamics premise and by adding fiscal and ecological perspectives and effects of the studied procedures. This comprehensive review of energy system performance and optimization would not only assist in effective energy properties use but also be one of the utmost characteristics in the establishment of justifiable methods of energy resource utilization.
Author contributions
SO: Writing–review and editing, Writing–original draft, Resources, Project administration, Methodology, Conceptualization. MW: Writing–review and editing, Writing–original draft, Validation, Methodology, Investigation. FA: Writing–review and editing, Project administration, Methodology, Investigation. JD: Writing–original draft, Validation, Methodology. OS: Writing–review and editing, Validation, Methodology, Investigation. OA: Writing–review and editing, Validation, Investigation. TS: Writing–review and editing, Validation. AP: Writing–review and editing, Validation, Methodology. OK: Writing–review and editing, Validation. PB: Writing–review and editing, Validation.
Funding
The author(s) declare that no financial support was received for the research, authorship, and/or publication of this article.
Conflict of interest
The authors declare that the research was conducted in the absence of any commercial or financial relationships that could be construed as a potential conflict of interest.
Publisher’s note
All claims expressed in this article are solely those of the authors and do not necessarily represent those of their affiliated organizations, or those of the publisher, the editors and the reviewers. Any product that may be evaluated in this article, or claim that may be made by its manufacturer, is not guaranteed or endorsed by the publisher.
References
Abam, F. I., Diemuodeke, O. E., Ekwe, E. B., Alghassab, M., Samuel, O. D., Khan, Z. A., et al. (2020a). Exergoeconomic and environmental modeling of integrated polygeneration power plant with biomass-based syngas supplemental firing. Energies 13, 6018–6027. doi:10.3390/en13226018
Abam, F. I., Diemuodeke, O. E., Ekwe, E. B., Alghassab, M., Samuel, O. D., Khan, Z. A., et al. (2020b). Exergoeconomic and environmental modeling of integrated polygeneration power plant with biomass-based syngas supplemental firing. Energies 13, 6018. doi:10.3390/en13226018
Abam, F. I., Ekwe, B. E., Samuel, O., Effiom, S. O., and Afangideh, C. B. (2018). Performance and thermo-sustainability analysis of non-hybrid organic Rankine cycles (ORCs) at varying heat source and evaporator conditions. Aust. J. Mech. Eng., 1–14. doi:10.1080/14484846.2017.1373585
Abam, F. I., Ugot, I. U., and Igbong, D. I. (2012). Performance analysis and components irreversiblities of a (25 MW) gas turbine power plant modeled with a spray cooler. Am. J. Eng. Appl. Sci. 5 (1), 35–41. doi:10.3844/ajeassp.2012.35.41
Adibhatla, A., and Kaushik, S. C. (2017). Energy, exergy and economic (3E) analysis of integrated solar direct steam generation combined cycle power plant. Sustain. Energy Technol. Assessments 20, 88–97. doi:10.1016/j.seta.2017.01.002
Agarwal, S., Arora, A., and Arora, B. B. (2021). Energy and exergy investigations of r1234YF and r1234ZE as r134A replacements in mechanically subcooled vapour compression refrigeration cycle. J. Therm. Eng. 7 (1), 109–132. doi:10.18186/thermal.846561
Agarwal, S. K. S. S., and Mishra, R. S. (2011). Performance improvement of a simple gas turbine cycle through integration of inlet air evaporative cooling and steam injection. J. Sci. industrial Res. 70, 544–533.
Aghbashlo, M., Hosseinzadeh-Bandbafha, H., Shahbeik, H., and Tabatabaei, M. (2022). The role of sustainability assessment tools in realizing bioenergy and bioproduct systems. Biofuel Res. J. 35, 1697–1706. doi:10.18331/brj2022.9.3.5
Ahmadi, M. H., Ahmadi, M. A., Pourfayaz, F., Bidi, M., and Açıkkalp, E. (2016b). Multi-objective optimization and exergetic-sustainability of an irreversible nano scale Braysson cycle operating with Maxwell–Boltzmann gas. Alex. Eng. J. 55, 1785–1798. doi:10.1016/j.aej.2016.03.034
Ahmadi, M. H., Ahmadi, M. A., and Sadatsakkak, S. A. (2015). Thermodynamic analysis and performance optimization of irreversible Carnot refrigerator by using multi-objective evolutionary algorithms (MOEAs). Renew. Sustain. Energy Rev. 51, 1055–1070. doi:10.1016/j.rser.2015.07.006
Ahmadi, M. H., Mehrpooya, M., and Pourfayaz, F. (2016a). Thermodynamic and exergy analysis and optimization of a transcritical CO2 power cycle driven by geothermal energy with liquefied natural gas as its heat sink. Appl. Therm. Eng. 109, 640–652. doi:10.1016/j.applthermaleng.2016.08.141
Ahmadi, P., and Dincer, I. (2010). Exergoenvironmental analysis and optimization of a cogeneration plant system using Multimodal Genetic Algorithm (MGA). Energy 35, 5161–5172. doi:10.1016/j.energy.2010.07.050
Ahmadi, P., and Dincer, I. (2011). Thermodynamic and exergoenvironmental analyses, and multi-objective optimization of a gas turbine power plant. Appl. Therm. Eng. 31, 2529–2540. doi:10.1016/j.applthermaleng.2011.04.018
Ahmadi, P., Dincer, I., and Rosen, M. (2011b). Exergy, exergoeconomic and environmental analyses and evolutionary algorithm based multi-objective optimization of combined cycle power plants. Energy 36 (10), 5886–5898. doi:10.1016/j.energy.2011.08.034
Ahmadi, P., Dincer, I., and Rosen, M. A. (2012). Exergo-environmental analysis of an integrated organic Rankine cycle for trigeneration. Energy Convers. Manage 64, 447–453. doi:10.1016/j.enconman.2012.06.001
Ahmadi, P., Dincer, I., and Rosen, M. A. (2013). Thermodynamic modeling and multi-objective evolutionary-based optimization of a new multigeneration energy system. Energy Convers. Manag. 76, 282–300. doi:10.1016/j.enconman.2013.07.049
Ahmadi, P., Rosen, M. A., and Dincer, I. (2011a). Greenhouse gas emission and exergo-environmental analyses of a trigeneration energy system. Int. J. Greenh. Gas. Control 5, 1540–1549. doi:10.1016/j.ijggc.2011.08.011
Akbari, M., Khodayari, M., Danesh, M., Davari, A., and Padash, H. (2020). A bibliometric study of sustainable technology research, Cogent Bus. and Manag. 7:1751906. doi:10.1080/23311975.2020.1751906
Akyuz, E., Coskun, C., Oktay, Z., and Dincer, I. (2012). A novel approach for estimation of photovoltaic exergy efficiency. Energy 44 (1), 1059–1066. doi:10.1016/j.energy.2012.04.036
Al-Ali, M., and Dincer, I. (2014). Energetic and exergetic studies of a multigenerational solar geothermal system. Appl. Therm. Eng., 71, 16–23. doi:10.1016/j.applthermaleng.2014.06.033
Alibaba, M., Pourdarbani, R., Manesh, M. H. K., Ochoa, G. V., and Forero, J. D. (2020). Thermodynamic, exergo-economic and exergo-environmental analysis of hybrid geothermal-solar power plant based on ORC cycle using emergy concept. Heliyon 6, 037588–e3812. doi:10.1016/j.heliyon.2020.e03758
Almutairi, A., Pilidis, P., and Al-Mutawa, N. (2015). Energetic and exergetic analysis of combined cycle power plant: Part-1 operation and performance. Energies 8 (12), 14118–14135. doi:10.3390/en81212418
Almutairi, A., Pilidis, P., and Al-Mutawa, N. (2016). Exergoeconomic and sustainability analysis of reheat gas turbine engine. Am. J. Energy Res. 4, 1–10. doi:10.12691/ajer-4-1-1
Al-Qayim, K. A. Integrated solar thermal combined cycle for power generation in Iraq (2019), IOP Conf. Ser. Mater. Sci. Eng., 518, 4. 042002. doi:10.1088/1757-899x/518/4/042002
Al-Sayyab, A. K. S., and Abdulwahid, M. A. (2019). Energy-exergy analysis of multistage refrigeration system and flash gas intercooler working with ozone-friendly alternative refrigerants to R134a. J. Adv. Res. Fluid Mech. Therm. Sci. 63 (2), 188–198.
Alta, D., Bilgili, E., Ertekin, C., and Yaldiz, O. (2010). Experimental investigation of three different solar air heaters: energy and exergy analyses. Appl. Energy 87, 2953–2973. doi:10.1016/j.apenergy.2010.04.016
Altayib, K. (2011). Energy, exergy and exergoeconomic analyses of gas-turbine based systems. Canada: University of Ontario Institute of Technology. MSc Thesis.
Ameri, M., Mokhtari, H., and Bahrami, M. (2016). Energy, exergy, exergoeconomic and environmental (4E) optimization of a large steam power plant: a case study. J. Sci. Technol. Trans. Mech. Eng. 40, 11–20. doi:10.1007/s40997-016-0002-z
Amrollahi, Z., Ertesvag, I., and Bolland, O. (2011). Thermodynamic analysis on post-combustion CO2 capture of natural-gas fired power plant. Int. J. Greenh. Gas. Control 5, 422–426. doi:10.1016/j.ijggc.2010.09.004
Annamalai, S., Santhanam, M., Selvaraj, S., Sundaram, M., Pandian, K., and Pazos, M. (2018). “Green technology”: bio-stimulation by an electric field for textile reactive dye contaminated agricultural soil. Sci. Total Environ. 624, 1649–1657. doi:10.1016/j.scitotenv.2017.10.047
Aprea, C., de Rossi, F., Greco, A., and Renno, C. (2003). Refrigeration plant exergetic analysis varying the compressor capacity. Int. J. Energy Res. 27, 653–669. doi:10.1002/er.903
Arora, A., and Kaushik, S. (2008). Theoretical analysis of a vapour compression refrigeration system with R502, R404A and R507A. Int. J. Refrig. 31, 998–1005. doi:10.1016/j.ijrefrig.2007.12.015
Asim, M., Saleem, S., Imran, M., Leung, M. K. H., Hussain, S. S. A., Miró, L. S., et al. (2020). Thermo-economic and environmental analysis of integrating renewable energy sources in a district heating and cooling network. Energy Effic. 13, 79–100. doi:10.1007/s12053-019-09832-9
Assareh, E., Agarwal, N., Paul, M. C., Ahmadi, P., Ghodrat, M., and Lee, M. (2023). Investigation and development of a novel solar-biomass integrated energy system for clean electricity and liquid hydrogen production. Therm. Sci. Eng. Prog. 42 (1), 101925. doi:10.1016/j.tsep.2023.101925
Athari, H., Soltani, S., B¨olükbas¸i, A., Rosen, M. A., and Morosuk, T. (2015a). Comparative exergoeconomic analyses of the integration of biomass gasification and a gas turbine power plant with and without fogging inlet cooling. Renew. Energy 76, 394–400. doi:10.1016/j.renene.2014.11.064
Athari, H., Soltani, S., Rosen, M. A., Gavifekr, M. K., and Morosuk, T. (2016a). Exergoeconomic study of gas turbine steam injection and combined power cycles using fog inlet cooling and biomass fuel. Renew. Energy 96, 715–726. doi:10.1016/j.renene.2016.05.010
Athari, H., Soltani, S., Rosen, M. A., Mahmoudi, S. M. S., and Morosuk, T. (2015b). Comparative exergoeconomic analyses of gas turbine steam injection cycles with and without fogging inlet cooling. Sustainability 7, 12236–12257. doi:10.3390/su70912236
Athari, H., Soltani, S., Rosen, M. A., Mohammad, S., Mahmoudi, S., and Morosuk, T. (2016b). Gas turbine steam injection and combined power cycles using fog inlet cooling and biomass fuel: a thermodynamic assessment. Renew. Energy 92, 95–103. doi:10.1016/j.renene.2016.01.097
Aydin, H. (2013). Exergetic sustainability analysis of LM6000 gas turbine power plant with steam cycle. Energy 57, 766–774. doi:10.1016/j.energy.2013.05.018
Bakhshmand, S., Saray, R., Bahlouli, K., Eftekhari, H., and Ebrahimi, A. (2015). Exergoeconomic analysis and optimization of a triple-pressure combined cycle plant using evolutionary algorithm. Energy 93, 555–567. doi:10.1016/j.energy.2015.09.073
Barzegar- Avval, H., Ahmadi, P., Ghaffarizadeh, A., and Saidi, M. (2011). Thermo-economic-environmental multiobjective optimization of a gas turbine power plant with preheater using evolutionary algorithm. Int. J. Energy Res. 35, 389–403. doi:10.1002/er.1696
Baumgärtner, S., and Arons, J. D. S. (2003). Necessity and inefficiency in the generation of waste: a thermodynamic analysis. J. Industrial Ecol. 7 (2), 113–123. doi:10.1162/108819803322564389
Bejan, A., Tsatsaronis, G., and Moran, M. (1996). Thermal design and optimization. Canada: John Wiley and Sons, Inc, 558.
Bhattacharya, A., Manna, D., Paul, B., and Datta, A. (2011). Biomass integrated gasification combined cycle power generation with supplementary biomass firing: energy and exergy based performance analysis. Energy 36, 2599–2610. doi:10.1016/j.energy.2011.01.054
Bianchi, M., Cherubini, F., Pascale, A. De, Peretto, A., and Elmegaard, B. (2006) Cogeneration from poultry industry wastes: indirectly fired gas turbine application, 31, 1417–1436.
Bishoge, O. K., Kombe, G. G., and Mvile, B. N. (2020). Renewable energy for sustainable development in sub-Saharan African countries: challenges and way forward. J. Renew. Sustain. Energy 12, 052702. doi:10.1063/5.0009297
Bobbo, S., Fedele, L., Curcio, M., Bet, A., De Carli, M., Emmi, G., et al. (2019). Energetic and exergetic analysis of low global warming potential refrigerants as substitutes for R410A in ground source heat pumps. Energies 12 (3538), 3538–3616. doi:10.3390/en12183538
Bolaji, B. O. (2010). Exergetic performance of a domestic refrigerator using R12 and its alternative refrigerants. J. Eng. Sci. Technol. 5 (4), 435–446.
Boonnasa, S., Namprakai, P., and Muangnapo, T. (2006). Performance improvement of the combined cycle power plant by intake air cooling using an absorption chiller. Energy 31, 2036–2046. doi:10.1016/j.energy.2005.09.010
Borokinni, F. O., Bolaji, B. O., and Ismail, A. A. (2018). Eksperimentalna analiza svojstava ekološki prihvatljivih radnih tvari R510A i R600a u modificiranome parnome kompresorskome rashladnom sustavu. NASE MORE 65 (1), 11–17. doi:10.17818/nm/2018/1.2
Bouam, A., Aïssani, S., and Kadi, R. (2008). Gas turbine performances improvement using steam injection in the combustion chamber under Sahara conditions. Oil and Gas Sci. Technology– Rev. IFP 63 (2), 251–261. doi:10.2516/ogst:2007076
Boukelia, T. E., Arslan, O., and Mecibah, M. S. (2016). ANN-based optimization of a parabolic trough solar thermal power plant. Appl. Therm. Eng. 107, 1210–1218. doi:10.1016/j.applthermaleng.2016.07.084
Boyaghchi, F. A., and Chavoshi, M. (2017). Multi-criteria optimization of a micro solar-geothermal CCHP system applying water/CuO nanofluid based on exergy, exergoeconomic and exergoenvironmental concepts. Appl. Therm. Eng. 112, 660–675. doi:10.1016/j.applthermaleng.2016.10.139
Boyaghchi, F. A., and Sabaghian, M. (2016). Multi objective optimisation of a Kalina power cycle integrated with parabolic trough solar collectors based on exergy and exergoeconomic concept. Int. J. Energy Technol. Policy 12, 154–180. doi:10.1504/ijetp.2016.075673
Buentello-Montoya, D., and Zhang, X. (2019). An energy and exergy analysis of biomass gasification integrated with a char-catalytic tar reforming system. Energy fuels. 33 (9), 8746–8757. doi:10.1021/acs.energyfuels.9b01808
Chamchine, A. V., Makhviladze, G. M., and Vorobyev, O. G. (2022). Thermodynamic indicators for integrated assessment of sustainable energy Technologies. Int. J. Low Carbon Technol. 1 (1), 69–78. doi:10.1093/ijlct/1.1.69
Chen, H., Xue, K., Wu, Y., Xu, G., Jin, X., and Liu, W. (2020). Thermodynamic and economic analyses of a solar-aided biomass-fired combined heat and power system. Energy 214, 119023. doi:10.1016/j.energy.2020.119023
Connelly, L., and Koshland, C. P. (1997). Two aspects of consumption: using an exergy-based measure of degradation to advance the theory and implementation of industrial ecology. Resour. Conservation Recycl. 19 (3), 199–217. doi:10.1016/s0921-3449(96)01180-9
Das, P., Dutta, S., Singh, K. K. K. K., and Maity, S. (2019). Energy saving integrated membrane crystallization: a sustainable technology solution. Sep. Purif. Technol. 228, 115722. doi:10.1016/j.seppur.2019.115722
Demirel, Y. (2012). Energy production, conversion, storage, conservation, and coupling. Springer-Verlag London Limited, 180–190.
Deng, D., Li, C., Zu, Y., Liu, L. Y. J., Zhang, J., and Wen, S. (2022). A systematic literature review on performance evaluation of power system from the perspective of sustainability. Front. Environ. Sci. 10 (10), 1–20. doi:10.3389/fenvs.2022.925332
De Souza-Santos, M. L. (1999). A feasibility study of an alternative power generation system based on biomass gasification/gas turbine concept. Fuel 78 (5), 529–538. doi:10.1016/s0016-2361(98)00181-1
Dincer, I., and Cengel, Y. A. (2001). Energy, entropy and exergy concepts and their roles in thermal engineering. Entropy 3, 116–149. doi:10.3390/e3030116
Dincer, I., Midilli, A., and Kucuk, H. (2014). Progress in exergy, energy, and the environment. Springer International Publishing Switzerland, 850–870.
Dincer, I., and Rosen, M. A. (2007). Exergy: energy, environment and sustainable development. Oxford OX2 8DP, UK: Elsevier, Linacre House, Jordan Hill, 473.
Doseva, N., and Chakyrova, D. (2015). Energy and exergy analysis of cogeration system with biogas engines. J. Therm. Eng. 1 (3), 391–401. doi:10.18186/jte.75021
Echegaray, M. E., Castro, M. R., Mazza, M. G. D., and Rodriguez, G. I. R. A. (2016). Exergy analysis of syngas production via biomass thermal gasification. Int. J. Thermo. 19 (3), 178–184. doi:10.5541/ijot.5000182576
Esen, H., Inalli, M., Esen, M., and Pihtili, K. (2007). Energy and exergy analysis of a ground-coupled heat pump system with two horizontal ground heat exchangers. Build. Environ. 42 (10), 3606–3615. doi:10.1016/j.buildenv.2006.10.014
Fagbenle, R. O., Adefila, S. S., Oyedepo, S. O., and Exergy, O. M. (2014). Exergoeconomic and exergoenvironomic analyses of selected gas turbine power plants in Nigeria. ASME Int. Mech. Eng. Congr. Expo., 1–13. doi:10.1115/IMECE2014-40311
Farzaneh-Gord, M., and Deymi-Dashtebayaz, M. (2009). A new approach for enhancing performance of a gas turbine (case study: khangiran refinery). Appl. Energy 86, 2750–2759. doi:10.1016/j.apenergy.2009.04.017
Ghaebi, H., Parikhani, T., Rostamzadeh, H., and Farhang, B. (2018). Proposal and assessment of a novel geothermal combined cooling and power cycle based on Kalina and ejector refrigeration cycles. Appl. Therm. Eng. 130, 767–781. doi:10.1016/j.applthermaleng.2017.11.067
Ghazikhani, M., Manshoori, N., and Tafazoli, D. (2005). “Experimental investigation of steam injection in Ge-F5 gas turbine nox reduction applying vodoley system,” in Second international conference on applied thermodynamics. Istanbul, Turkey, 18–20.
Gholamian, E., Mahmoudi, S. M. S., and Zare, V. (2016). Proposal, exergy analysis and optimization of a new biomass-based cogeneration system. Appl. Therm. Eng. 93, 223–235. doi:10.1016/j.applthermaleng.2015.09.095
Ghorbania, B., Mehrpooya, M., and Sadeghzadeh, M. (2018). Developing a tri-generation system of power, heating, and freshwater (for an industrial town) by using solar flat plate collectors, multi-stage desalination unit, and Kalina power generation cycle. Energy Convers. Manag. 165, 113–126. doi:10.1016/j.enconman.2018.03.040
Gill, J., and Singh, J. (2017). Energy analysis of vapor compression refrigeration system using mixture of R134a and LPG as refrigerant. Int. J. Refrig. 84, 287–299. doi:10.1016/j.ijrefrig.2017.08.001
Gill, J., and Singh, J. (2018). Component-wise exergy and energy analysis of vapor compression refrigeration system using mixture of R134a and LPG as refrigerant. Heat Mass Transf. 54 (5), 1367–1380. doi:10.1007/s00231-017-2242-x
Giovanni, P. (2014). Energy conversion and management: principles and applications. Cham Heidelberg New York Dordrecht London: Springer, 150–178.
Gojak, M., and Bajc, T. (2019). Thermodynamic sustainability assessment for heating of residential building. E3S Web Conf. 111 04028, 04028–4036. CLIMA 2019. doi:10.1051/e3sconf/201911104028
Goncalves, L. P., and Arrieta, F. R. P. (2010). An exergy cost analysis of a cogeneration plant. Rev. Eng. Térmica 9 (1-2), 28–34. doi:10.5380/reterm.v9i1-2.61927
Granet, I., and Bluestein, M. (2000). Thermodynamics and heat power. 6th Ed. Upper Saddle River: Prentice Hall, 838.
Gümüş, M., and Atmaca, M. (2013). Energy and exergy analyses applied to a CI engine fueled with diesel and natural gas. Energy Sources, Part A Recovery, Util. Environ. Eff. 35 (11), 1017–1027. doi:10.1080/15567036.2010.516312
Hammond, G. P. (2004). Engineering sustainability: thermodynamics, energy systems, and the environment. Int. J. Energy Res. 28, 613–639. doi:10.1002/er.988
Hepbasli, A. (2008). A key review on exergetic analysis and assessment of renewable energy resources for a sustainable future. Renew. Sustain. energy Rev. 12 (3), 593–661. doi:10.1016/j.rser.2006.10.001
Huggins, R. A. (2016). Energy storage - fundamentals, materials and applications. 2nd Edition. Springer International Publishing Switzerland, 30–50.
Imran, M., Park, B. S., Kim, H. J., Lee, D. H., Usman, M., and Heo, M. (2014). Thermo-economic optimization of Regenerative Organic Rankine Cycle for waste heat recovery applications. Energy Convers. Manag. 87, 107–118. doi:10.1016/j.enconman.2014.06.091
Inoussah, M. M., Adolphe, M. I., and Daniel, L. (2017). Assessment of sustainability indicators of thermoelectric power generation in Cameroon using exergetic analysis tools. Energy Power Eng. 9, 22–39. doi:10.4236/epe.2017.91003
Iora, P., and Silva, P. (2013). Innovative combined heat and power system based on a double shaft intercooled externally fired gas cycle. Appl. Energy 105, 108–115. doi:10.1016/j.apenergy.2012.11.059
Jamil, M. A., Shahzad, M. W., and Zubair, S. M. (2020). A comprehensive framework for thermoeconomic analysis of desalination Systems. Energy Convers. Manag. 222, 113188–113220. doi:10.1016/j.enconman.2020.113188
Jana, K., and De, S. (2014). Biomass integrated gasification combined cogeneration with or without CO2 capture-a comparative thermodynamic study. Renew. Energy 72, 243–252. doi:10.1016/j.renene.2014.07.027
Jørgensen, S. E., and Svirezhev, Y. M. (2004). Towards a thermodynamic theory for ecological systems. Amsterdam: Elsevier, 380.
Kalaiselvam, S., and Saravan, R. (2009). Exergy Analysis of scroll compressors working with R22, R407C, and R417A as refrigerant for HVAC system. Therm. Sci. 13, 175–184. doi:10.2298/tsci0901175k
Karellas, S., and Braimakis, K. (2016). Energy–exergy analysis and economic investigation of a cogeneration and trigeneration ORC–VCC hybrid system utilizing biomass fuel and solar power. Energy Convers. Manag. 107, 103–113. doi:10.1016/j.enconman.2015.06.080
Keçebas, A., and Gokgedik, H. (2015). Thermodynamic evaluation of a geothermal power plant for advanced exergy analysis, Energy. 88, 746–755. doi:10.1016/j.energy.2015.05.094
Khalid, F., Dincer, I., and Rosen, M. A. (2015). Energy and exergy analyses of a solar-biomass integrated cycle for multi-generation. Sol. Energy 112, 290–299. doi:10.1016/j.solener.2014.11.027
Khani, N., Manesh, M. H. K., and Onishi, V. C. (2022). 6E analyses of a new solar energy-driven polygeneration system integrating CO2 capture, organic Rankine cycle, and humidification-dehumidification desalination. J. Clean. Prod. 379, 1–29. doi:10.1016/j.jclepro.2022.134478
Khanmohammadi, S., Saadat-Targhi, M., Al-Rashed, AAAA, and Afrand, M. (2019). Thermodynamic and economic analyses and multi-objective optimization of harvesting waste heat from a biomass gasifier integrated system by thermoelectric generator. Energy Convers. Manage 195, 1022–1034. doi:10.1016/j.enconman.2019.05.075
Khartchenko, N. V., and Kharchenko, V. M. (2014). “6000 broken sound parkway NW, suite 300,” in Advanced energy systems. 2nd edition (Boca Raton: CRC Press Taylor and Francis Group), 215–230.
Kim, K. H., Han, C. H., and Kim, K. (2012). Effects of ammonia concentration on the thermodynamic performances of ammonia-water based power cycles. Thermochim. Acta 530, 7–16. doi:10.1016/j.tca.2011.11.028
Kim, K. H., Han, C. H., and Kim, K. (2013). Comparative exergy analysis of ammonia-water based Rankine cycles with and without regeneration. Int. J. Exergy. 12, 344–361. doi:10.1504/ijex.2013.054117
Kim, K. H., Ko, H. J., and Kim, K. (2014). Assessment of pinch point characteristics in heat exchangers and condensers of ammonia-water based power cycles. Appl. Energy 113, 970–981. doi:10.1016/j.apenergy.2013.08.055
Kizilkan, O., Kabul, A., and Yakut, A. K. (2010). Exergetic performance assessment of a variable speed R404a refrigeration system. Int. J. Energy Res. 34, 463–475. doi:10.1002/er.1553
Klemm, C., and Wiese, F. (2022). Indicators for the optimization of sustainable urban energy systems based on energy system modeling. Energy, Sustain. Soc. 12 (3), 3–20. doi:10.1186/s13705-021-00323-3
Koç, Y., Yağlı, H., and Kalay, I. (2020). Energy, exergy, and parametric analysis of simple and recuperative organic Rankine cycles using a gas turbine–based combined cycle. J. Energy Eng. 146 (5), 1–20. doi:10.1061/(asce)ey.1943-7897.0000693
Kopanos, G. M., Liu, P., Michael, C., and Georgiadis, M. C. (2017). Advances in energy systems engineering. Springer International Publishing Switzerland, 837.
Labele-Alawa, B. T., and Asuo, J. M. (2011). Optimization of the system performance of a gas turbine plant. Int. J. Appl. Sci. Technol. 1 (6), 250–255.
Lieuwen, T. C., and Yang, V. (2013). Gas turbine emissions. New York, NY: Cambridge University Press 32 Avenue of the Americas, 150–210. 10013-2473, USA.
Liu, W., Zhang, X., Zhao, N., Shu, C., Zhang, S., Ma, Z., et al. (2018). Performance analysis of organic Rankine cycle power generation system for intercooled cycle gas turbine. Adv. Mech. Eng. 10 (8), 1–12. doi:10.1177/1687814018794074
Lolos, P. A., and Rogdakis, E. D. (2009). A Kalina power cycle driven by renewable energy sources. Energy 34, 457–464. doi:10.1016/j.energy.2008.12.011
Lugo-Leyte, R., Salazar-Pereyra, M., Ru´ız-Ram´ırez, O. A., Zamora-Mata, J. M., and Torres-Gonz´alez, E. V. (2013) Exergoeconomic operation cost analysis to theoretical compression refrigeration cycle of HFC-134a, 12. Mexico: Revista Mexicana de Ingenier´ıa Qu´ımica, 361–370.
Manente, G. (2016). High performance integrated solar combined cycles with minimum modifications to the combined cycle power plant design. Energy Convers. Manag. 111, 186–197. doi:10.1016/j.enconman.2015.12.079
Maraver, D., Quoilin, S., and Royo, J. (2014). Optimization of biomass-fuelled combined cooling, heating and power (CCHP) systems integrated with subcritical or transcritical organic rankine cycles (ORCs). Entropy 16, 2433–2453. doi:10.3390/e16052433
Memon, A. G., Memon, R. A., Harijan, K., and Uqaili, M. (2015). Parametric based thermo-environmental and exergoeconomic analyses of a combined cycle power plant with regression analysis and optimization. Energy Convers. Manag. 92, 19–35. doi:10.1016/j.enconman.2014.12.033
Menlik, T., Demircioğlu, A., and Özkaya, M. G. (2013). Energy and exergy analysis of R22 and its alternatives in a vapour compression refrigeration system, Int. J. Exergy. 12 1, 11–30. doi:10.1504/ijex.2013.052568
Michaelides, E. E. (2018). Energy, the environment, and sustainability, in 6000 broken sound parkway NW, suite 300. Boca Raton: CRC Press Taylor and Francis Group, 230–245.
Midilli, A., and Dincer, I. (2009). Development of some exergetic parameters for PEM fuel cells for measuring environmental impact and sustainability. Int. J. Hydrogen Energy 34, 3858–3872. doi:10.1016/j.ijhydene.2009.02.066
Mulvihill, M. J., Beach, E. S., Zimmerman, J. B., and Anastas, P. T. (2011). “Green chemistry and green engineering: a framework for sustainable technology development,”. Editors A. Gadgil, and D. M. Liverman, 36, 271–293. doi:10.1146/annurevenviron-032009-095500Annu. Rev. Environ. Resour.
Ofodu, J. C., and Abam, D. P. S. (2002). Exergy analysis of afam thermal power plant. NSE Tech. Trans. 37 (3), 14–28.
Ogriseck, S. (2009). Integration of Kalina cycle in a combined heat and power plant, a case study. Appl. Ther. Eng. 29, 2843–2848. doi:10.1016/j.applthermaleng.2009.02.006
Oko, C. C., and Njoku, I. H. (2017). Performance analysis of an integrated gas-steam- and organic fluid-cycle thermal power plant, Energy. 122: 431–443. doi:10.1016/j.energy.2017.01.107
Owebor, K., Oko, C. O. C., Diemuodeke, E. O., and Ogorure, O. J. (2019). Thermo-environmental and economic analysis of an integrated municipal waste-to-energy solid oxide fuel cell, gas, steam, organic fluid- and absorption refrigeration cycle thermal power plants. Appl. Energy 239, 1385–1401. doi:10.1016/j.apenergy.2019.02.032
Oyedepo, S. O. (2012). On energy for sustainable development in Nigeria. Renew. Sustain. Energy Rev. 16, 2583–2598. doi:10.1016/j.rser.2012.02.010
Oyedepo, S. O. (2014a). Thermodynamic performance analysis of selected gas turbine power plants in Nigeria. Ota, Nigeria: Covenant University, 1–282. Ph.D Thesis.
Oyedepo, S. O. (2014b). Towards achieving energy for sustainable development in Nigeria. Renew. Sustain. Energy Rev. 34, 255–272. doi:10.1016/j.rser.2014.03.019
Oyedepo, S. O., Fagbenle, R. O., Adefila, S. S., and Alam, M. M. (2015a). Exergy costing analysis and performance evaluation of selected gas turbine power. Plants Cogent Eng. 2, 2–21. doi:10.1080/23311916.2015.1101048
Oyedepo, S. O., Fagbenle, R. O., Adefila, S. S., and Alam, M. M. (2015b). Thermoeconomic and thermoenvironomic modeling and analysis of selected gas turbine power plants in Nigeria. Energy Sci. Eng. 3 (5), 423–442. doi:10.1002/ese3.79
Oyedepo, S. O., Fagbenle, R. O., Adefila, S. S., and Alam, M. M. (2016). Exergoenvironomic modelling and performance assessment of selected gas turbine power plants. World J. Eng. 13 (2), 149–162. doi:10.1108/wje-04-2016-020
Oyedepo, S. O., Fagbenle, R. O., Adefila, S. S., Alam, M. M., and Dunmade, I. S. (2018). Thermo-economic and environmental assessment of selected gas turbine power plants in Nigeria. Prog. Industrial Ecol. – An Int. J. 12 (4), 361–384. doi:10.1504/pie.2018.097164
Oyedepo, S. O., Fagbenle, R. O., Adefila, S. S. and M. M., and Alam, M. M. (2015c). Performance evaluation of selected gas turbine power plants in Nigeria using energy and exergy methods. World J. Eng. 12 (2), 161–176. doi:10.1260/1708-5284.12.2.161
Oztop, H. F., Bayrak, F., and Hepbasli, A. (2013). Energetic and exergetic aspects of solar air heating (solar collector) systems. Renew. Sustain. Energy Rev. 21, 59–83. doi:10.1016/j.rser.2012.12.019
Padilla, M., Revellin, R., and Bonjour, J. (2010). Exergy analysis of R413A as replacement of R12 in a domestic refrigeration system. Energy Convers. Manag. 51, 2195–2201. doi:10.1016/j.enconman.2010.03.013
Palazzo, P. (2013). Thermoeconomics and exergy method in environmental engineering. J civil. Environ. Eng. 3, e110. doi:10.4172/2165-784X.1000e110
Patterson, M. G. (1996). What is energy efficiency? Energy Policy 24 (5), 377–390. doi:10.1016/0301-4215(96)00017-1
Pellegrini, L. F. ., and de Oliveira Junior, S. (2011). Combined production of sugar, ethanol and electricity: thermoeconomic and environmental analysis and optimization. Energy. 36(6), 3704–3715. doi:10.1016/j.energy.2010.08.011
Prananto, L. A., Zaini, I. N., Mahendranata, B. I., Juangsa, F. B., and Aziz, M. (2018). Use of the Kalina cycle as a bottoming cycle in a geothermal power plant: case study of the Wayang Windu geothermal power plant. Appl. Therm. Eng. 132, 686–696. doi:10.1016/j.applthermaleng.2018.01.003
Rao, A. (2015). Sustainable energy conversion for electricity and coproducts: principles, technologies, and equipment. Hoboken, New Jersey, Canada: John Wiley and Sons, Inc., 130–137.
Ratlamwala, T. A. H., Gadalla, M. A., and Dincer, I. (2011). Performance assessment of an integrated PV/T and triple effect cooling system for hydrogen and cooling production. Int. J. Hydrogen Energy 36, 11282–11291. doi:10.1016/j.ijhydene.2010.11.121
Rivero, R., Garcia, M., and Urquiza, J. (2004). Simulation, exergy analysis and application of diabatic distillation to a tertiary amyl methyl ether production unit of a crude oil refinery. Energy 29, 467–489. doi:10.1016/j.energy.2003.10.007
Rosen, M. A. (2009). “Applications of exergy to enhance ecological and environmental understanding and stewardship,” in Proceedings of the 4th IASME/WSEAS international conference on energy and environment (Stevens Point Wisconsin United States: World Scientific and Engineering Academy and Society (WSEAS)), 146–152.
Rosen, M. A. (2021). Exergy analysis as a tool for addressing climate change. Eur. J. Sustain. Dev. Res. 5 (2), em0148–10. doi:10.21601/ejosdr/9346
Roshanzadeh, B., Asadi, A., and Mohan, G. (2023). Technical and economic feasibility analysis of solar inlet air cooling systems for combined cycle power plants. Energies 16, 5352–5423. doi:10.3390/en16145352
Sahoo, U., Kumar, R., Pant, P. C., and Chaudhary, R. (2017). Development of an innovative polygeneration process in hybrid solar-biomass system for combined power, cooling and desalination. Appl. Therm. Eng. 120, 560–567. doi:10.1016/j.applthermaleng.2017.04.034
Saidur, R., Ahamed, J. U., and Masjuki, H. H. (2010). Energy, exergy and economic analysis of industrial boilers. Energy Policy 38, 2188–2197. doi:10.1016/j.enpol.2009.11.087
Saidur, R., Sattar, M. A., Masjuki, H. H., Ahmed, S., and Hashim, U. (2007). An estimation of the energy and exergy efficiencies for the energy resources consumption in the transportation sector in Malaysia. Energy Policy 35 (8), 4018–4026. doi:10.1016/j.enpol.2007.02.008
Samadi, S., Gröne, M.-C., Schneidewind, U., Luhmann, H.-J., Venjakob, J., and Best, B. (2017). Sufficiency in energy scenario studies: taking the potential benefits of lifestyle changes into account. Technol. Forecast. Soc. Change 124, 126–134. doi:10.1016/j.techfore.2016.09.013
Santos, V. E. N., Ely, R. N., Szklo, A. S., and Magrini, A. (2016). Chemicals, electricity and fuels from biorefineries processing Brazil׳s sugarcane bagasse: production recipes and minimum selling prices. Renew. Sustain. Energy Rev. 53, 1443–1458. doi:10.1016/j.rser.2015.09.069
Sarhaddi, F., Farahat, S., Ajam, H., and Behzadmehr, A. (2009). Exergetic optimization of a solar photovoltaic array. J. Thermodyn. 2009. Article ID 313561, 1 – 12. doi:10.1155/2009/313561
Seyam, S., Dincer, I., and Agelin-Chaab, M. (2020). Development of a clean power plant integrated with a solar farm for a sustainable community. Energy Convers. Manag. 225, 113434–113520. doi:10.1016/j.enconman.2020.113434
Shamoushaki, M., Ghanatir, F., Ehyaei, M., and Ahmadi, A. (2017). Exergy and exergoeconomic analysis and multi-objective optimisation of gas turbine power plant by evolutionary algorithms. Case study: Aliabad Katoul power plant. Int. J. Exergy 22, 279–307. doi:10.1504/ijex.2017.083160
Singh, O. K., and Kaushik, S. C. (2014). Exergoeconomic analysis of a Kalina cycle coupled coal-fired steam power plant. Int. J. Exergy 14 (1), 38–59. doi:10.1504/ijex.2014.059512
Spelling, J. D. (2013). Hybrid solar gas-turbine power plants - a thermoeconomic analysis. Stockholm, Sweden: KTH Royal Institute of Technology School of Industrial Engineering and Management Department of Energy Technology, 100 44. PhD Thesis.
Srebrenkoska, V., Fidancevska, E., Jovanov, V., and Angusheva, B. (2013). “Sustainable technology and natural environment,” in Proceedings of XXI international scientific and professional meeting “ecological truth” ECO-IST’13. Serbia: University of Belgrade, 238–242. Technical Faculty. Serbia, 2013.
Su, Y. (2020). Smart energy for smart built environment: a review for combined objectives of affordable sustainable green. Sustain. Cities Soc. 53, 101954–102015. doi:10.1016/j.scs.2019.101954
Sulaiman, M. A., Waheed, M. A., Adesope, W. A., and Noike, A. (2017). Techno-economic investigation of different alternatives of improving simple gas turbine integration options. Niger. J. Technol. 36, 849–857. doi:10.4314/njt.v36i3.27
Sun, F., Ikegami, Y., and Jia, B. (2012). A study on Kalina solar system with an auxiliary superheater. Renew. Energy 41, 210–219. doi:10.1016/j.renene.2011.10.026
Sun, F., Zhou, W., Ikegami, Y., Nakagami, K., and Su, X. (2014). Energy-exergy analysis and optimization of the solar-boosted Kalina cycle system 11 (KCS-11). Renew. Energy 66, 268–279. doi:10.1016/j.renene.2013.12.015
Taner, T. (2015). Optimisation processes of energy efficiency for a drying plant: a case of study for Turkey. Appl. Therm. Eng. 80, 247–260. doi:10.1016/j.applthermaleng.2015.01.076
Traverso, A., and Massardo, A. F. (2002). Thermoeconomic analysis of mixed gas-steam cycles. Appl. Therm. Eng. 22: 1–21. doi:10.1016/s1359-4311(01)00064-3
UN (2015). Transforming our world: the 2030 agenda for sustainable development. Report A/RES/70/1. United State of America: United Nations. Available at: https://sustainabledevelopment.un.org/post2015/transformingourworld/publication.
Valero, A., and Cuadra, C. T. (2002) “Thermoeconomic analysis, exergy, energy system analysis and optimization – vol. II - thermoeconomic analysis,” in Encyclopedia of life support systems. Paris, France: EOLSS, 1–15.
Van Gool, W. (1992). Exergy analysis of industrial processes. Energy 17 (8), 791–803. doi:10.1016/0360-5442(92)90123-h
Varga, Z., and Palotai, B. (2017). Comparison of low temperature waste heat recovery methods. Energy 137, 1286–1292. doi:10.1016/j.energy.2017.07.003
Vera, D., and Jurado, F. (2018). Biomass gasification coupled to an EFGT-ORC combined system to maximize the electrical energy generation: a case applied to the olive oil industry, 144, 41–53.
Vera, D., Jurado, F., and Carpio, J. (2011a). Study of a downdraft gasifier and externally fired gas turbine for olive industry wastes. Fuel Process Technol. 92 (10), 1970–1979. doi:10.1016/j.fuproc.2011.05.017
Vera, D., Jurado, F., de Mena, B., and Schories, G. (2011b). Comparison between externally fired gas turbine and gasifier-gas turbine system for the olive oil industry. Energy 36 (12), 6720–6730. doi:10.1016/j.energy.2011.10.036
von Spakovsky, M. R., and Frangopoulos, C. A. (2011). Exergy, energy system analysis and optimization – vol. III - analysis and optimization of energy systems with sustainability considerations. Encycl. Life Support Syst. (EOLSS).
Waheed, M. A., Ajayi, O. O., and Ohijeagbon, O. D. (2018). Techno-economic analysis of NERC’s feed-in tariff for sustained grid-connected renewable power supply: case of 3 selected sites of northern Nigeria. Proc. World Congr. Eng.
Wang, F. J., and Chiou, J. (2004). Integration of steam injection and inlet air cooling for a gas turbine generation system. Energy Convers. Manag. 45, 15–26. doi:10.1016/s0196-8904(03)00125-0
Xiang, Y., Cai, L., Guan, Y., Liu, W., He, T., and Li, J. (2019). Study on the biomass-based integrated gasification combined cycle with negative CO2 emissions under different temperatures and pressures. Energy 179, 571–580. doi:10.1016/j.energy.2019.05.011
Yataganbaba, A., Kilicarslan, A., and Kurtbas, I. (2015). Exergy analysis of R1234yf and R1234ze as R134a replacements in a two evaporator vapour compression refrigeration system. Int. J. Refrig. 60, 26–37. doi:10.1016/j.ijrefrig.2015.08.010
Yuanyuan, L., and Yongping, Y. (2015). Impacts of solar multiples on the performance of integrated solar combined cycle systems with two direct steam generation fields. Appl. Energy 160, 673–680. doi:10.1016/j.apenergy.2015.08.094
Yue, C., Han, D., Pu, W., and He, W. (2015). Comparative analysis of a bottoming transcritical ORC and a Kalina cycle for engine exhaust heat recovery. Energy Convers. Manage 89, 764–774. doi:10.1016/j.enconman.2014.10.029
Zare, V. (2020). Role of modeling approach on the results of thermodynamic analysis: concept presentation via thermoeconomic comparison of biomass gasification-fueled open and closed cycle gas turbines. Energy Convers. Manag. 225, 113479. doi:10.1016/j.enconman.2020.113479
Zare, V., and Hasanzadeh, M. (2016). Energy and exergy analysis of a closed Brayton cycle-based combined cycle for solar power tower plants. Energy Convers. Manage 128, 227–237. doi:10.1016/j.enconman.2016.09.080
Zare, V., and Palideh, V. (2018). Employing thermoelectric generator for power generation enhancement in a Kalina cycle driven by low-grade geothermal energy. Appl. Therm. Eng. 130, 418–428. doi:10.1016/j.applthermaleng.2017.10.160
Zhang, X., Li, H., Liu, L., Bai, C., Wang, S., Song, Q., et al. (2018). Exergetic and exergoeconomic assessment of a novel CHP system integrating biomass partial gasification with ground source heat pump. Energy Convers. Manage 156, 666–679. doi:10.1016/j.enconman.2017.11.075
Ziółkowski, P., Lemański, M., Badur, J., and Nastałek, L. (2012). Power augmentation of PGE gorzow’s gas turbine by steam injection – thermodynamic overview. Rynek Energii 1 (98), 161–167.
Nomenclature
Abbreviated terms
ASU Air Separation Unit
BIGCC Biomass Integrated Gasification Combined Cycle
CC Combustion Chamber
CCPP Combined Cycle Power Plant
CCS Carbon Capture Storage
CHP Combined Heat and Power
CON Condenser
COP Coefficient of Performance
CSP Concentrated Solar Power
DSG Direct Steam Generation
EEF Environmental Effect Factor
EFGT Extended Fuel Gas Turbine
EIF Environmental Impact Factor
EIP Exergy Improvement Potential
ESI Exergetic Sustainability Index
ETI Exergo-Thermal Index
EUI Exergetic Utility Index
GHG Green House Gas
GT Gas Turbine
GWP Global Warming Potential
HFC Hydrofluorocarbon
HSB Hybrid Solar- Biomass
HPT High Pressure Turbine
IAC Inlet Air Cooling
IGCC Integrated Gas Combined Cycle
IFGT Internally Fuel Gas Turbine
IRR Internal Rate of Return
ISCC Integrated Solar Combined Cycle
ISO International Standards Organisation
KC Kalina Cycle
LCA Life Cycle Assessment
LPT Low Pressure Turbine
MW Mega Watts
ODP Ozone Depletion Potential
ORC Organic Rankine Cycle
PES Primary Energy Saving
PRE-HE Pre- Heater
PTC Parabolic Trough Collector
PV Photovoltaic
RERs Renewable Energy Resources
SED Sustainable Energy Development
SGT Simple Gas Turbine
SoR Share of Renewables
STI Steam Injection
TDI Thermal Discharge Index
TIT Turbine Inlet Temperature
Turb Turbine
VAP Vapour
VCC Vapour Compression Cycle
WER Waste Exergy Ratio
Greek letters
ηΔH Enthalpic Efficiency
Pth Thermal energy input
β Thermal pollution factor
C′ Specific Energy Costs
Keywords: thermodynamics, sustainability indices, thermo-economic, thermoenvironmental, energy systems, sustainable development
Citation: Oyedepo SO, Waheed MA, Abam FI, Dirisu JO, Samuel OD, Ajayi OO, Somorin T, Popoola API, Kilanko O and Babalola PO (2025) A critical review on enhancement and sustainability of energy systems: perspectives on thermo-economic and thermo-environmental analysis. Front. Energy Res. 12:1417453. doi: 10.3389/fenrg.2024.1417453
Received: 14 April 2024; Accepted: 25 October 2024;
Published: 03 January 2025.
Edited by:
Michael Carbajales-Dale, Clemson University, United StatesReviewed by:
Moses Omolayo Petinrin, University of Ibadan, NigeriaDebabrata Barik, Karpagam Academy of Higher Education, India
Copyright © 2025 Oyedepo, Waheed, Abam, Dirisu, Samuel, Ajayi, Somorin, Popoola, Kilanko and Babalola. This is an open-access article distributed under the terms of the Creative Commons Attribution License (CC BY). The use, distribution or reproduction in other forums is permitted, provided the original author(s) and the copyright owner(s) are credited and that the original publication in this journal is cited, in accordance with accepted academic practice. No use, distribution or reproduction is permitted which does not comply with these terms.
*Correspondence: Sunday O. Oyedepo, c29veWVkZXBvQGJlbGxzdW5pdmVyc2l0eS5lZHUubmc=