- 1Power China Huadong Engineering Corporation Limited, Hangzhou, China
- 2School of Energy and Power Engineering, Huazhong University of Science and Technology, Wuhan, China
Hydrogen is regarded as the premier energy source for future sustainability and renewability. However, its distinct physicochemical properties render it prone to explosions in the event of a leak. Therefore, there is a need for more comprehensive research dealing with hydrogen leakage, explosion scenarios, and risk assessment. This paper provides an overview of the current hydrogen policies adopted in China. It reviews the processes of hydrogen refueling station construction and the thermophysical mechanisms of liquid hydrogen leakage. In this regard, the effects of various factors, including leakage rate, leakage time, leakage hole size, wind direction and speed, and building location, on the hydrogen leakage rate are analyzed and evaluated. Additionally, the impacts of different factors on hydrogen explosion overpressure are reported, including hydrogen concentration, wind speed, obstacles, and ignition position, in addition to the current applications of quantitative risk assessment methods in hydrogen refueling stations. Finally, the limitations of current research on liquid hydrogen leakage and explosion accidents are highlighted, along with the shortcomings of current risk assessment methods for liquid hydrogen refueling stations.
1 Introduction
Hydrogen has been adopted as a renewable source of energy and a potential fuel. In the past few years, high-pressure hydrogen has been widely used in fuel cell vehicles. Hydrogen has the highest mass-energy density compared to the other established energy sources, as shown in Table 1. Thus, it has emerged as a hot topic in recent research studies and a main focus of practical applications worldwide (Midilli et al., 2005; Aditiya and Aziz, 2021). Hydrogen refueling station (HRS) is one of the most important basic infrastructures for fuel cell vehicles. Compared to gas hydrogen refueling stations, liquid hydrogen refueling stations have the advantages of high energy density, low transportation costs, and high purity of hydrogen storage.
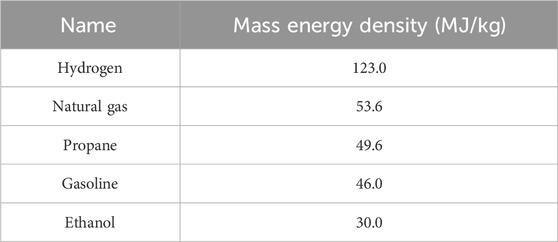
Table 1. Energy density of different fuel masses (Qian, 2021).
At present, China’s hydrogen refueling stations are in the stage of accelerated development, and how high the risk level of hydrogen refueling stations has become the most concerned issue of the public. In this paper, hydrogen policies in China was analyzed. Accordingly, the hydrogen safety issues was figured. Research survey on liquid hydrogen leakage was carried out.
This study designed the hydrogen fueling station scenario according to the Chinese standard specifications and hydrogen fuel cell buses demand and calculated and analyzed the accidents frequencies, risk indicators, components failure frequencies, and physical models of consequences, resulting in more targeted recommendations for component precautionary measures and safety precautionary distance combining Quantitative Risk Assessment method with HyRAM developed by Sandia National Laboratories in the US.
2 Hydrogen policies in China
Hydrogen fuel cells have been developed and presented as a key technology for various energy applications. They are characterized by a wide capacity range and zero emissions, and they are highlighted as one of the primary applications of hydrogen energy. In particular, hydrogen fuel cells have emerged as one of the preferred technologies in the development of alternative energy vehicles.
China’s Medium- and Long-term Plan for the Development of Hydrogen Energy Industry (2021–2035), released in 2022 (Zhang et al., 2022), clearly highlights that hydrogen energy is a crucial component of the country’s future energy system. It states that hydrogen energy is a major cornerstone and a key technology to adopt toward achieving the country’s low-carbon and green transformation In light of the carbon peaking goal in 2030, China’s annual production of hydrogen is expected to reach 37.15 million tons. This is predicted to increase under the carbon neutrality goal by 2060 to approximately 130 million, according to the “China Hydrogen Energy and Fuel Cell Industry White Paper 2020” (Alliance, 2021).
According to the Energy Saving and New Energy Vehicle Technology Roadmap 2.0, there will be 100,000 hydrogen fuel cell-driven vehicles on the road by 2025 and around one million vehicles by 2035. In this regard, there is a need to establish hydrogen refueling stations as crucial infrastructures to allow for hydrogen refueling into on-board high-pressure cylinders (Chen et al., 2021; Kuroki et al., 2018; Chen et al., 2022). Up to March 2022, China has established 264 hydrogen refueling stations, ranking first in the world. Figure 1 illustrates the evolution trend in the country’s established hydrogen refueling station count from 2016 to 2022, demonstrating the country’s rapid growth in this field in recent years (Liu et al., 2024).
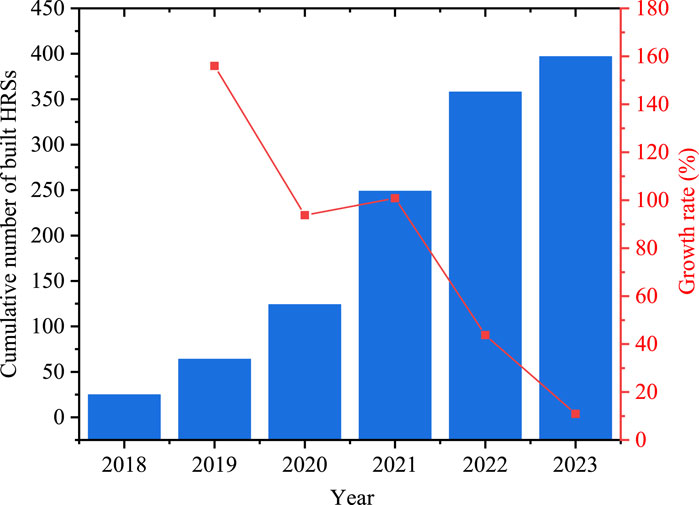
Figure 1. Number of established hydrogen refueling stations in China (Liu et al., 2024).
Although hydrogen is an environmentally friendly green energy source, it has not yet been harnessed extensively. The primary obstacle to the growth of hydrogen energy applications is the storage method. At room temperature and pressure, hydrogen has a large volume and is not economically viable. Overall, the most common forms of hydrogen storage are high-pressure gas hydrogen and low-temperature liquid hydrogen. On this basis, hydrogen refueling stations in China and abroad are primarily established in the form of high-pressure gas hydrogen refueling stations and liquid hydrogen refueling stations, with the latter constituting around 30% of the total number (Genovese and Fragiacomo, 2023).
The first liquid hydrogen refueling station in China was officially completed in 2021 in Pinghu City in the Zhejiang Province. Compared to gas hydrogen refueling stations, liquid hydrogen refueling stations have the advantages of high energy density, low transportation costs, and high purity of hydrogen storage (Genovese and Fragiacomo, 2023). However, the liquid hydrogen industry in China is still facing major challenges hindering its promotion and advancement. In terms of technical support and standards, multiple guidance and requirements reports were presented and released by China’s General Administration of Market Supervision (National Standards Commission), including the GB/T40045-2021 ″Hydrogen Vehicle Fuel Liquid Hydrogen” (Author Anonymous, 2024a), the GB/T40060-2021 ″Liquid Hydrogen Storage and Transportation Technical Requirements” (Author Anonymous, 2024b), and the GB/T40061-2021 ″Technical Specification for Liquid Hydrogen Production System” (Author Anonymous, 2024c). The publication of these three national standards for liquid hydrogen is a significant milestone in the development of China’s hydrogen refueling station infrastructure as well as a major step toward promoting hydrogen energy applications and implementations in the country.
3 Hydrogen safety issues
Although hydrogen energy offers numerous advantages, a significant drawback of its applications is that leaks are difficult to detect due to the lack of color and odor. Due to its inherent physical characteristics, hydrogen leakage might pose major safety risks in practical applications. Table 2 lists some of the physical characteristics of hydrogen compared to those of natural gas and gasoline vapor. As shown in the table, hydrogen has a very low ignition energy of only 0.02 MJ, which is about 1/10 of that of natural gas and gasoline vapor. Its flammability range is relatively wide, reaching 4% and 75% at its lowest and highest points. In terms of diffusion, hydrogen has a diffusion coefficient of 6.11 × 10−5 m2/s, which is 4 and 12 times that of natural gas and gasoline vapors, respectively. Additionally, hydrogen can be mixed with air very quickly to form a flammable cloud (Genovese and Fragiacomo, 2023). Once this hydrogen cloud ignites, it may burn or even explode. This results in tremendous temperatures and overpressure that could pose serious safety issues and become a danger for the lives of people and the operation of equipment nearby (Molkov, 2012).

Table 2. Comparison of the physical properties of hydrogen, natural gas, and gasoline vapors (Genovese and Fragiacomo, 2023).
As the safety concerns presented above are due to the specific characteristics of hydrogen, they also apply to liquid hydrogen. Because liquid hydrogen has a storage temperature as low as 20 K, a leak will severely damage station equipment and endanger the lives of personnel due to the low temperature characteristics of liquid hydrogen. Moreover, when it is evaporated into ambient hydrogen, the volume of liquid hydrogen can expand to 840 times its original volume. As a result, an area of high hydrogen concentration is established, leading to hypoxia symptoms.
At present, China considers hydrogen a hazardous chemical due to its combustion and explosion traits. Thus, it sets strict regulations in place for hydrogen preparation, storage, transportation, and use. However, uncontrollable factors, such as accidental impacts, material failures, and operational errors, can pose additional safety risks. In this context, the explosion of a hydrogen storage tank at a plant in Gangwon Province, South Korea, in May 2019, the flash explosion of a hydrogen refueling unit at a petrochemical company in Zhuhai, China, in January 2020, and the bursting and combustion accident of a hydrogen tanker truck due to a ruptured hose in August 2021 in Liaoning, China, are just a few examples of the scale and severity of fire and explosion accidents caused by hydrogen.
Considering the recent evolution in technology and standards, China’s hydrogen refueling stations are currently in an advanced stage of development. The main concern is how high the risk associated with hydrogen refueling stations is and what the extent of the danger imposed by these stations is. Thus, there is a need to further improve and enrich the research related to hydrogen safety. This has practical implications for promoting the future development of China’s liquid hydrogen refueling stations design and establishment.
4 Thermophysical mechanisms of liquid hydrogen leakage
Overall, liquid hydrogen leakage and diffusion involve complex flow and heat and mass transfer mechanisms. This primarily depends on the storage pressure and temperature, in addition to whether the release scenario is impact, confined, or in an open environment. When there is a leakage incident, a variety of physical phenomena may occur, including air condensation, heat and cold convection, flash evaporation, and evaporation from the liquid pool. It is noted here that, due to the relatively low density of liquid hydrogen, the effluent velocity is high during the effluent phase, reaching 50 m/s at a gauge pressure of 1 bar and 100 m/s at a gauge pressure of 4 bar. As liquid hydrogen has a storage pressure that typically exceeds atmospheric pressure, its temperature is likely to be higher than the atmospheric pressure when it is released into the atmosphere, corresponding to its boiling point. For every 1 K increase in the superheating temperature, approximately 2% of the liquid hydrogen is predicted to evaporate immediately. This leads to a volume expansion rate of roughly 120%/K.
Furthermore, due to the significant temperature difference between the hydrogen and the surrounding air, the liquid hydrogen that remains after flash evaporation will quickly vaporize and absorb heat. Then, it will liquefy and solidify when the air temperature falls below the freezing point of oxygen and nitrogen. As a result, a combination of solid-liquid oxygen and nitrogen particles exist, along with a multi-phase hydrogen mist. As illustrated in Figure 2, the liquid pool and the solid-liquid surfaces undergo mass and heat exchanges. In the process, a dynamic equilibrium is attained when the liquid hydrogen mass evaporated due to the heat received from the ground or other object is equal to the mass of liquid hydrogen released. If liquid hydrogen is crashed before it is heated and diluted by the ambient air and undergoes evaporation, nitrogen-oxygen particles may deposit with some liquid hydrogen to form a liquid pool before undergoing an expansion.
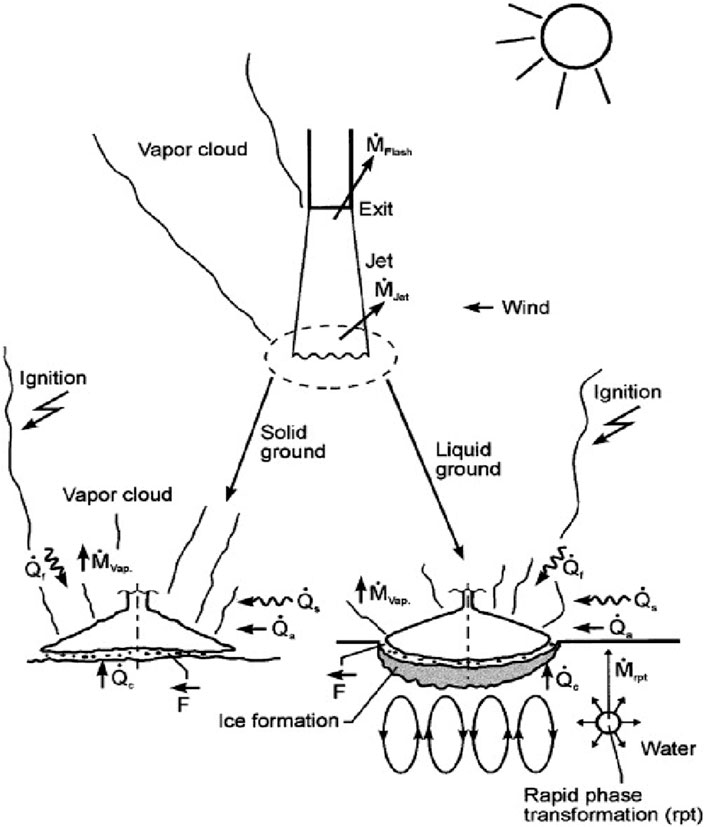
Figure 2. The set of physical phenomena occurring upon the release of a cryogenic liquid with the various mass flows M and heat flows Q (subscripts a: atmospheric; c: convection; f: flame; s: solar) (Verfondern and Dienhart, 2007).
Moreover, the cryogenic plume mixture starts to return to the gas phase outside the near-field region. Initially, the gaseous hydrogen and air plume will be much colder than the ambient air. Due to air condensation, the density of the cryogenic hydrogen cloud will initially be greater than the air’s density, exhibiting a dense gas behavior. However, the respective density starts to decrease as the hydrogen cloud is diluted by air. As a result, the hydrogen density becomes less than that of the air as the gas cloud climbs upward.
5 Research on liquid hydrogen leakage
5.1 Experimental research
Based on a small-scale liquid hydrogen spill experiment conducted by the U.S. Bureau of Mines in 1960, the flammable vapor cloud and the visible hydrogen cloud were reported in separate locations (Zabetakis and Burgess, 1959). The performed experiment involved lighting fires above a liquid hydrogen dewar at several spill rates. In 1980, a large-scale liquid hydrogen flooding experiment was carried out at the White Sands Proving Ground in New Mexico, by the National Aeronautics and Space Administration (NASA) (Witcofski and Chirivella, 1984). In this experiment, a liquid pool was established on the ground in 35 s by passing a volume of 5.7 m3 of liquid hydrogen through a diffuser. The aim of this investigation was to determine the diffusion pattern of the flammable cloud following a rapid leak of liquid hydrogen on a relatively large scale. Figure 3 depicts the form of the visible hydrogen cloud created based on the evaporation of the liquid hydrogen in the experiment. The findings of the experiment demonstrated that the flammable cloud had moved quickly along the ground. In this process, hydrogen would quickly mix with air due to multiple phenomena: the momentum created by the liquid hydrogen leakage, the rapid expansion formed by the phase change, and the turbulence resulting from the thermal instability of the gas cloud. As a result, the hydrogen cloud heats up to become positively buoyant, which would determine its rate of ascent.
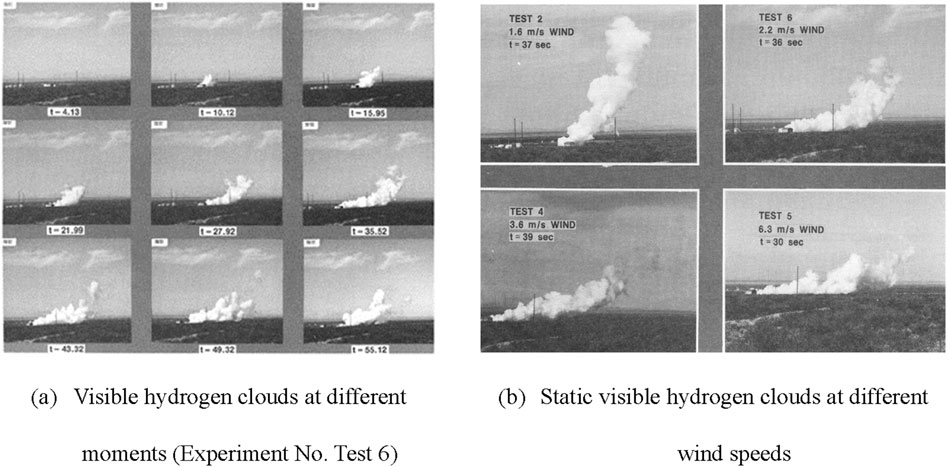
Figure 3. Images of liquid hydrogen spillage reported by the experiment conducted by NASA (Midilli et al., 2005). (A) Visible hydrogen clouds at different moments (Experiment No. Test 6). (B) Static visible hydrogen clouds at different wind speeds.
Furthermore, the German Federal Institute for Materials (BAM) (Statharas et al., 2000) carried out a diffusion experiment to investigate liquid hydrogen leakage in a building in 1996. The aim of the experiment was to increase knowledge about liquid hydrogen leakage accidents and their impacts. In the investigation, the liquid pool size, ground temperature, and liquid pool evaporation rate were measured. Based on the evaluation, it was reported that heat exchange between the ground and the gas cloud resulted in a relatively weaker “heavy gas” effect of the cloud. As a result, a more complex and diversified diffusion process is obtained. Additionally, the gas cloud was significantly impacted by the wind direction due to the building’s obstruction effect.
In addition, research was carried out by the UK’s Health and Safety Laboratory (HSL) in 2010 to investigate the dispersion of liquid hydrogen that is leaking in the presence of buildings (Willoughby and Royle, 2014; Hooker et al., 2011). In order to study the diffusion properties of liquid hydrogen leakage and carry out igniting experiments on a gas cloud, HSL performed a small-scale liquid hydrogen leakage experiment in open space. Some of the experimental results obtained are displayed in Figure 4. The figure presents solid deposits of nitrogen and oxygen forming on concrete floors that have been sufficiently cooled by liquid hydrogen. Additionally, it is noted that a combustible cloud caused by the rupture of a 1-inch pipe reaches at least 9 m downwind from the release point. In this specific experiment, liquid hydrogen was released both vertically and horizontally along a concrete floor in a large space and at a certain height from the floor.
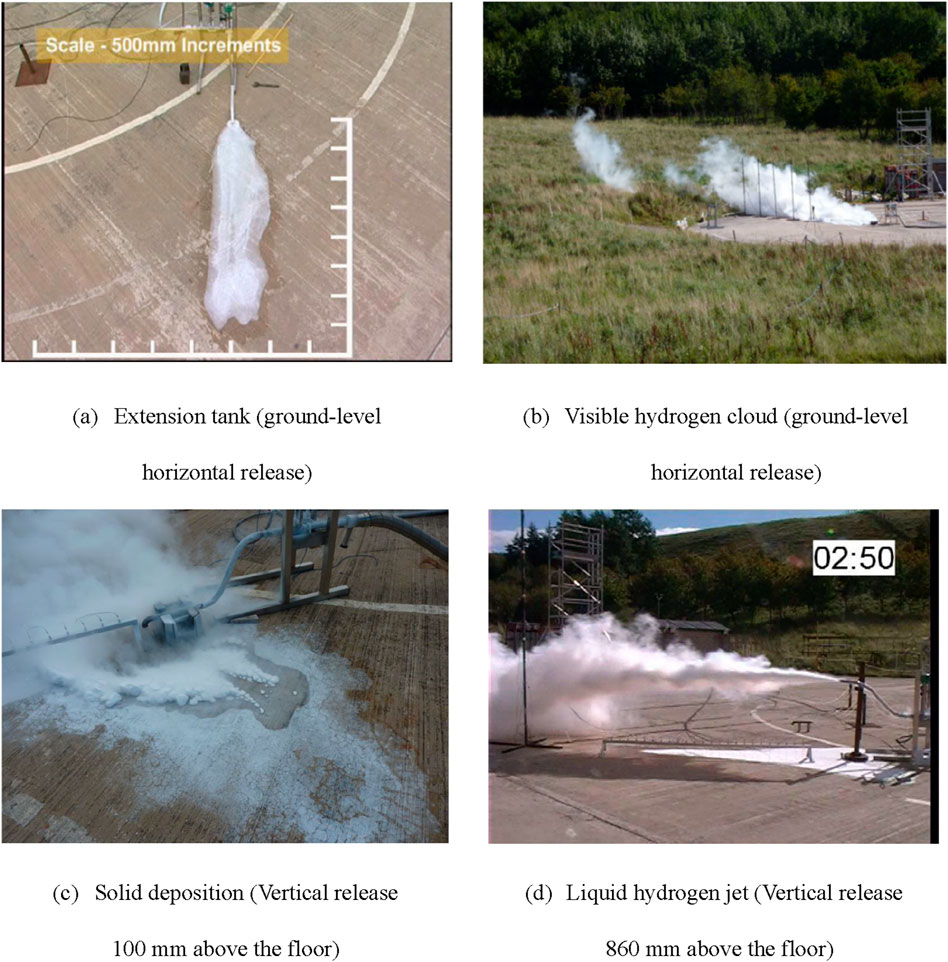
Figure 4. Schematic illustration of liquid hydrogen leaks reported by HSL experiments (Willoughby and Royle, 2014). (A) Extension tank (ground-level horizontal release), (B) Visible hydrogen cloud (ground-level horizontal release), (C) Solid deposition (Vertical release 100 mm above the floor), (D) Liquid hydrogen jet (Vertical release 860 mm above the floor).
5.2 Numerical research
Based on a comprehensive literature review, it is obvious that experimental studies presented on liquid hydrogen leakage are very limited due to its great practical difficulties, high danger factor, and large costs. To address this, most researchers investigate the leakage problem using numerical computations. As the Computational Fluid Dynamics (CFD) model can more accurately replicate the actual situation, multiple researchers have utilized CFD to simulate the two-phase flow of the liquid hydrogen leakage process along with the diffusion process of a low-temperature hydrogen cloud. Due to the large computational domain, the mixture model is often employed, treating homogeneous two phase flow. Consequently, the governing equations yield Equations 1–3.
where ρm represents the mix density. n and
where
In most of the studies presented dealing with large-scale liquid hydrogen spillage incidents, researchers have concentrated on the growth of the liquid pool and the dispersion behavior of the flammable cloud under various environmental conditions. For instance, Middha et al. (2011) replicated NASA’s liquid hydrogen spillage experiments by establishing a pool model that can accurately represent the process of liquid hydrogen spillage diffusion and evaporation in the CFD program FLACS. The model predictions were found to be in good agreement with the experimental results. In their study, they reported that atmospheric stability has a greater impact on the combustible cloud’s diffusive behavior. In this regard, increasing atmospheric stability can reduce atmospheric turbulence and the mixing between hydrogen and the surrounding air. This, in turn, is necessary for the development of a large enough buoyancy only under stable atmospheric conditions. Overall, decreasing the atmospheric turbulence and decreasing the mixing between hydrogen and the surrounding air through diffusion behavior enhances the atmospheric stability. This results in a combustible cloud with a buoyancy force that allows it to rise only in stable atmospheric conditions, which separates it from the ground.
Holborn et al. (2020) employed FLACS to simulate large-scale liquid hydrogen releases. Based on this study, they reported that the downwind combustible distance and the height of the combustible cloud are affected by the spill rate, duration, and wind speed. Additionally, the primary factors influencing the combustible mass were highlighted, including the spillage rate and duration. In this regard, it was noted that the combustible clouds made of materials with a relatively higher thermal conductivity, such as wet sand, exhibited a higher peak combustible mass. Also, a higher wind speed forces the cloud to move along the ground, increasing the downwind combustible distance. On the other hand, lower wind speeds cause the cloud’s head to move away from the ground due to the buoyancy effect.
Moreover, a three-dimensional CFD model of large-scale liquid hydrogen spillage was constructed by Liu et al. (2019), employing FLUENT. The model was verified using NASA tests. Overall, it was demonstrated that taking air humidity into account improved the numerical predictions to be in very good agreement with the experimental data. It is noted here that the atmospheric moisture condensation increases the vapor cloud’s buoyancy. This, in turn, encourages the diffusion of the combustible cloud in a vertical direction. Thus, the combustible cloud diffusion formed by the large-scale liquid hydrogen spillage increased in altitude with the increase in air humidity. At the same time, there is a decrease in the distance of separation from the ground in the downwind direction.
In their study, Tang et al. (2020) investigated the evaporation and diffusion processes of liquid hydrogen utilized as a vehicle fuel following spillage in open-air, garage, and tunnel scenarios. They employed a three-dimensional transient CFD model with multicomponent (hydrogen, air) phase transitions. Additionally, the morphology of the combustible cloud in the hydrogen diffusion in the different scenarios was examined, along with the duration. As a result, the open-air scenario exhibited the longest duration and was characterized by the largest volume of flammable cloud among the three scenarios investigated. Moreover, it was highlighted that the garage scenario has the longest combustible cloud diffusion period, bringing a variety of risks. Thus, all the cars in the garage could be in danger. Also, below the height of 1.5 m, the tunnel scenario achieves the maximum downwind hazard distance among the scenarios. This scenario also yields the maximum downwind hazard distance below a height of 1.5 m.
Furthermore, as the cryogenic hydrogen cloud has an initial velocity, its motion tendency differs from that of a large-scale flooding event. It is noted here that it is impossible to build a liquid pool at the ground due to the small leakage of the modest flow of persistent liquid hydrogen jets. In their study, Hansen (2020) employed the pseudo-source method to examine the quasi-steady-state cloud created by the leakage of a liquid hydrogen horizontal jet. Instead of rising to the sky and disappearing as in the case of a compressed hydrogen release, it was shown that the cryogenic hydrogen cloud descended in the near field as a dense gas.
In another study, Pu et al. (2019) used data from the liquid hydrogen release experiments conducted at HSL to create a three-dimensional transient CFD model. The developed model accounted for the phase transition between liquid hydrogen and air. In the investigation, they examined the diffusion behavior of cryogenic liquid hydrogen and methane after leakage, considering a similar inlet Froude number. On this basis, they reported that the cold effect cloud reached equilibrium faster than the combustible cloud. With Froude number increases from 0.47 to 3.72, liquid hydrogen represents a downward trend while liquid methane shows a downwind trend. For combustible clouds, the movements of hydrogen are larger than that of methane in both downwind and vertical direction on a quasi-stable state. Cryogenic liquid hydrogen was released as a heavy mixture in the near field while being dispersed as a light gas in the far field. It was noted that the hydrogen cloud flammability distance is always greater than that of the methane cloud.
In their work, Ichard et al. (2012) employed FLACS to simulate the vertical and horizontal jet experiments of HSL. They found that when liquid hydrogen leaks downward in a vertical direction, the air condenses and solidifies. As a result, the flow field is altered, causing an upward velocity that increases cloud buoyancy by releasing heat. However, in the case of horizontal leakage, there are no major impacts on the flow away from the release point because the region of condensation and solidification is close to the release point. Giannissi and Venetsanos (2018) used ADREA-HF to examine the effects of ambient humidity and the condensation of air constituents (nitrogen and oxygen) on the diffusion of liquid hydrogen leakage. They reported that the low-temperature cloud’s action swirls the liquid or solid particles of water, nitrogen, and oxygen into the cloud to increase its density. However, the positive buoyancy of condensation and solidification effects outweighs the effect of increasing the density of the mixture.
Generally, effects of leakage rate, time, hole size, wind direction, and speed on hydrogen leakage are interconnected and significantly influence safety risks. A higher leakage rate results in a greater volume of hydrogen escaping in a shorter time, increasing the potential for hazardous concentrations. The duration of the leak also plays a critical role; prolonged leaks can lead to higher accumulation levels. Larger hole sizes facilitate faster and more substantial hydrogen release, exacerbating risks. Wind direction affects the dispersion of hydrogen; if aligned with the leak, it can carry the gas away, reducing local concentration, while opposing wind can cause buildup. Finally, wind speed influences the dilution of hydrogen; higher speeds promote rapid dispersion, lowering the risk of ignition, whereas low speeds may allow for dangerous concentrations to form in localized areas.
6 Consequences of hydrogen leakage explosions
When hydrogen leaks into a flammable cloud and takes a long time to ignite, an explosion may occur. To address this, a large block of studies has been presented in the literature, where scientists have examined flammable gas explosions using experiments, theoretical analysis, and numerical simulation tools. On this basis, researchers have gathered a good understanding of the explosion and the corresponding elements contributing to the process. Moreover, the ignition of combustible clouds results in a relatively small flame propagation speed, a little amount of heat release, and a negligible explosion overpressure only in the case of laminar or transitional flow combustion. It is noted here that when obstacles are encountered during flame propagation, an enhancement in the mixed gas turbulence within the combustible cloud is observed. This leads to the expansion of the flame front and the acceleration of the combustion process. As a result, a significant amount of heat is released, causing the gas volume to expand rapidly and inducing a sharp overpressure increase. This phenomenon further enhances the degree of turbulence, causing more gas to participate in the combustion process and creating a cyclic excitation effect (Li, 2015), as presented in Figure 5.
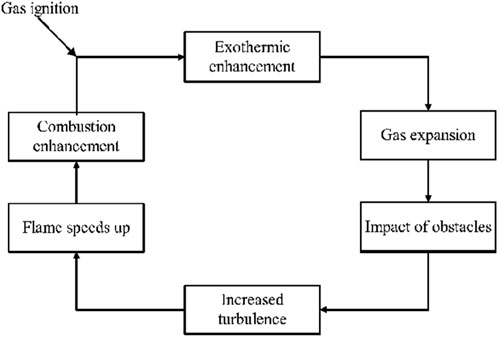
Figure 5. Cyclic excitation effect of the explosion (Li, 2015).
In addition, Groethe et al. (2007) carried out large-scale hydrogen explosion experiments to examine the impact of different obstructions on the explosion in various situations, as highlighted in Figure 6. In the open space experiments conducted, a 300 m3 hemispherical facility was considered with initial hydrogen concentrations ranging from 15% to 30%. Also, a number of cylindrical obstacles were used in some experiments to examine the possibility of turbulence-enhanced explosions. However, the results showed that the presence of obstacles did not enhance the explosion intensity. On the other hand, different results were reported for the small-scale experiments while using the same volume of obstacles. The discrepancy in the findings is likely because such obstacles resulted in low levels of turbulence in large spaces. This makes it challenging to consider small-scale experiments in the evaluation of the safety hazards of large-scale accidents. Moreover, tunnel experiments were conducted in a 78.5-meter-long vehicle tunnel with a cross-sectional area of 3.74 m2, aiming to investigate the effects of homogeneous gas mixing explosions and hydrogen-releasing explosions. It was found that the restriction of the tunnel to hydrogen resulted in a significant increase in the hazard compared to unrestricted spaces. Also, it was shown that proper tunnel ventilation could significantly reduce the hazard. Furthermore, semi-restricted experiments were conducted in a 37 m3 facility with two aluminum plates, placed 10 mm apart. The experiments aimed to simulate possible gaps in the hydrogen facility and study the effect of partial restrictions on the explosion. However, the size of the plates and the gaps chosen in this experiment did not result in an explosion enhancement. Finally, protective wall experiments were conducted to investigate the attenuation of the blast wave by employing a protective structure around the hydrogen facility with a wall of 4 m high and 10 m wide. As a result, the explosion intensity was reduced to an extent of at least twice the height of the wall.
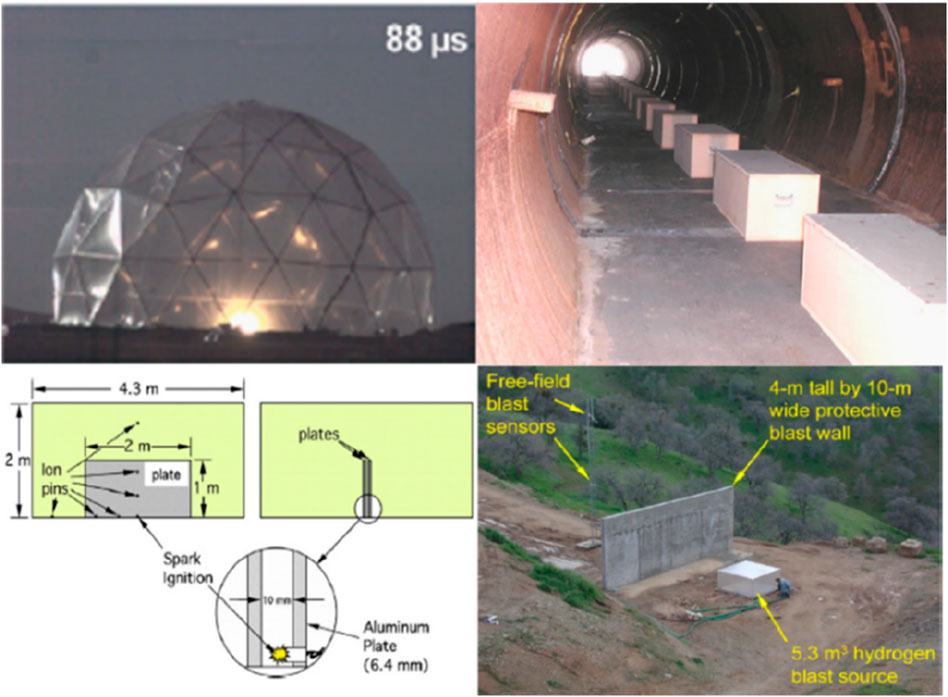
Figure 6. Different experimental scenarios considered by Groethe et al. (2007).
To study the risks associated with hydrogenation stations, Tanaka et al. (2007) conducted diffusion and explosion experiments in a full-size hydrogenation station model. In the experiments, high-pressure hydrogen was released from nozzles of a size ranging from 0.8 to 8.0 mm in the hydrogenator location and the storage chamber, respectively. The confinement experiments demonstrated that the hydrogen concentration had a significant impact on the overpressure. In this regard, a hydrogen concentration of 15% resulted in the minimal size of the hazardous area created by the explosion in the storage chamber. On the other hand, increasing the hydrogen concentration to 30% caused the station’s overpressure to exceed the allowable limit. It is noted here that leakage-related explosions at the hydrogenator location will only create a smaller hazardous area close to the nozzle. This indicates that the open area’s explosive intensity and hazardous area are lower than those in the confined space. In a connected study, Shiruill et al. (2012) conducted an explosion experiment using a premixed hydrogen cloud with dimensions of 5.4 m × 6.0 m × 2.5 m in a virtual hydrogenation station. The study findings demonstrated that the highest explosion overpressure was achieved when the ignition occurred between the two hydrogenators. Additionally, the local high pressure was recorded in the case of the explosion overpressure in crowded areas like the tube bank and the high pressure storage area.
Based on the conducted review, it is noted that most researchers have studied the effects of hydrogen leakage explosions using theoretical analysis approaches or numerical simulation methods. This is because of the significant cost and high risk of conducting experiments with actual hydrogen leakage explosions. Generally, the vapor cloud explosion model is used in theoretical analysis approaches to estimate the consequences of an accident and evaluate the impacts of the explosion. Typically, empirical or semi-empirical formulations of the diffusion model are used to obtain the hydrogen concentration distribution and convert it to an equivalent chemical gas cloud. Because of its validated approach, model simplicity, and modest computational requirements, the Gaussian diffusion model is one of the most extensively used diffusion models in such evaluations (Jiang, 2009).
Nevertheless, the main categories of vapor cloud explosion models can be divided into: i) The TNT equivalent method, which is based on the empirical measurement of explosion intensity and is not very suitable for gas explosions (Song et al., 2005), ii) TNO multi-energy method, which is biased toward the explosion by obstacles, where the boundaries can be adjusted by the user using multiple coefficients that characterize the degree of constraints (van den Berg, 1985), and iii) Baker-Strehlow method, which classifies three levels of obstacle congestion as low, medium, and high. Among these methods, the Strehlow approach is primarily used to estimate the far-field pressure and is marginally inadequate for local prediction. In the evaluation method, it splits the degree of obstacle congestion into three levels: low, medium, and high (Zhang et al., 2010). Li et al. (2012) conducted a quantitative analysis of the hydrogen leakage explosion accident at a hydrogen refueling station at a pressure of 35 MPa using the unified diffusion model and adopting the Baker-Strehlow method. It was reported that the vapor cloud explosion impact distance increased with the increase in hydrogen storage pressure, leakage aperture, and wind speed. Additionally, an enhanced algebraic model based on acoustic theory was presented by Pu et al. (2018). In this regard, the enhanced algebraic model significantly increases the computational efficiency when dealing with engineering applications of hydrogen safety evaluation. It has superior accuracy when conducting medium- and large-scale hydrogen/air explosion predictions, with acceptable results when considering small-scale situations.
FLACS software is one of the major tools used in numerical simulation research dealing with gas diffusion, combustion, and explosion applications, particularly in modifying the hydrogen diffusion and explosion model. Numerous experiments have been conducted to verify and validate the applicability of using the FLACS software in the study of hydrogen safety issues (Middha et al., 2007; Middha and Hansen, 2009). Using, FLACS, a large number of researchers have studied different aspects of the hydrogen explosion accident (Qian, 2021; Kim et al., 2013; Liang et al., 2019; Li et al., 2015). Considering a hydrogen refueling station in South Korea as a research object, Kim et al. (2013) conducted simulation research on hydrogen explosion incidents. They examined the features of the explosion’s pressure distribution at various sites in the hydrogen storage tanks, production facilities, and hydrogenators. Based on the investigation, they recommended placing explosion-proof walls close to the area of the tanks where a significant amount of hydrogen is stored. This is because the results highlighted that the explosion hazard increases with an increase in the volume of the gas cloud. Thus, placing such walls can effectively block the propagation of the explosion overpressure. In a connected investigation, a study on the leaking explosion process of China’s first renewable energy hydrogen refueling station was carried out by Liang et al. (2019). The findings reported that the maximum explosion hazard distance shows an inverted V curve with the increase of the wind speed in the case where the wind direction and leakage are in opposite directions after the hydrogen explosion accident hazards, compared to the case where the two are in the same direction. This was demonstrated following a simulation analysis of the renewable energy hydrogen refueling station leakage explosion process, aiming to examine the effects of various factors on the accident’s consequences. Furthermore, when a hydrogen storage tank with a 90 MPa pressure leaks toward a pipe trailer, the explosive threats are relatively high because the blockage degree is large. This results in a higher explosion hazard area along with a fatal area. In their investigation, Li et al. (2015) conducted high-pressure hydrogen leakage and explosion accident simulations of the Shanghai World Expo hydrogen refueling station. On this basis, they reported that higher wind speeds and congestion increase the explosion intensity, with wind speeds exceeding 9 m/s contributing to the explosion. Nevertheless, in situations where the explosion-proof wall is not capable of effectively hindering the spread of an overpressure wave, it is recommended to implement supplementary safety precautions for the compressor. Qian (2021) established a low-temperature compressed hydrogen refueling station model. The model was used to simulate the effects of a hydrogen leakage explosion with and without protective walls. The results showed that installing a protective wall can significantly reduce the risks of high temperatures and overpressures. Thus, it is capable of delaying the propagation of overpressure waves to a point behind the wall. The wall height is determinate, and the distance between the walls should be limited, as a large distance will increase the range of hazards related to high temperatures and overpressures.
7 Quantitative risk assessment method in hydrogen refueling stations
The risk assessment method was first introduced in the insurance industry in the 1930s. As the world’s industrialization accelerated, this method was used in the 1960s to evaluate the safety of buildings, businesses, and other establishments due to the persistent rise in leaks, fires, and explosions in the chemical industry. Since then, many risk assessment techniques have been developed based on research and development efforts, including qualitative, semi-quantitative, and quantitative risk assessment methods.
The qualitative risk assessment approach refers to the assessment of the likelihood of the occurrence of a specific accident and the impacts of the corresponding consequences. These impacts are generally described using qualitative terms, such as low, medium, and high. On the other hand, semi-quantitative risk assessment involves the use of a scoring mechanism to express the likelihood and severity of the consequences’ impacts, considering a semi-quantitative approach due to the lack of complete information on the incident. Overall, a large block of studies in the literature have examined qualitative or semi-quantitative risk assessment techniques due to the lack of databases on hydrogen events and accidents. In the assessments conducted by Kikukawa et al. (2008); Kikukawa et al. (2009), FMEA (Failure Mode and Effects Analysis) and HAZOP (Hazard and Operability Analysis) were employed. Using these two analysis forms, they identified a number of accident scenarios at a 70 MPa fuel cell vehicle refueling station and a liquid hydrogen refueling station. Then, a risk matrix, shown in Table 3, was used to assess the risk level of the accident scenarios. As a result, it was noted that for both the 70 MPa and the 35 MPa refueling stations, a safety distance of 6 m is sufficient. In the evaluation, the liquid hydrogen refueling station was given the essential precautions to lower the danger, and the effectiveness and application of the safety measures were demonstrated.
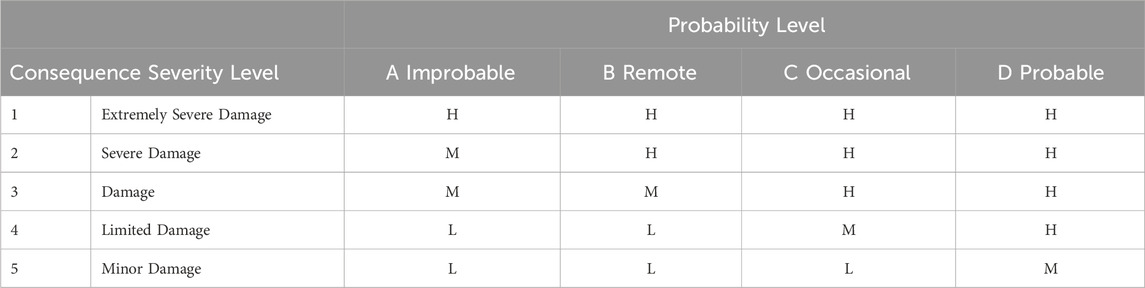
Table 3. Risk matrix presented by Li et al. (2015).
In addition, Nakayama et al. (2016) conducted a Hazard Identification (HAZID) study to identify potential scenarios and qualitatively assess the risks associated with a hybrid gasoline-hydrogen fueling station. Based on their study, they suggested multiple improvements, including the installation of protective walls, the implementation of hydrogen detectors, and using emergency shut-off valves for a variety of special hybrid risk scenarios. Moreover, the safety of the high-pressure storage equipment at a hydrogen refueling station in California was examined and evaluated by Casamirra et al. (2009), employing the FMEA, HAZOP, and FTA (Fault Tree Analysis) approaches. On this basis, it was reported that the station is characterized by excellent safety performance. After conducting a risk assessment of a high-pressure hydrogen storage and refueling station using the semi-quantitative analysis method HAZOP-LOPA (Layers Of Protection Analysis), Zhang (2019) concluded that there was a high risk associated with high-pressure hydrogen storage cylinders. By comparing the assessment’s findings against the ALARP (As Low As Reasonable) criteria, the risks were evaluated, and it was highlighted that immediate precautions are needed.
The quantitative risk assessment (QRA) technique is capable of expressing and quantifying the accident frequency and the consequence severity, as well as evaluating the level of risk associated with a particular operation or facility. As risks are incredibly unforeseen and common to happen, a thorough and integrated examination of hazards is necessary in various operations. Adopting the QRA approach, the following four queries are answered: i) What kinds of accidents are most likely to happen? ii) How likely are they to happen? iii) What are the potential consequences? and iv) Is the overall risk acceptable?
In recent years, due to the rapid expansion of hydrogen refueling stations and the rising frequency of hydrogen incident occurrences, a large number of researchers have applied quantitative risk assessment (QRA) approaches in different safety studies of hydrogen refueling stations. It is noted that, at present, gaseous hydrogen refueling stations are the primary subject of QRA studies dealing with hydrogen refueling stations. For instance, Zhiyong et al. (2010); Zhiyong et al. (2011) carried out QRA evaluations on various kinds of gaseous hydrogen refueling stations. In the assessments, they assessed the risks to employees, clients, and third parties at the stations. The results highlighted that the hydrogen refueling machine and the compressor are the main risk points. Thus, actions must be taken to stop the hydrogen from leaking continuously. Such actions include lifting the compressor and erecting an enclosure around it. Additionally, a city hydrogen refueling station was the subject of a QRA study conducted by Gye et al. (2019). The findings indicated an intolerable risk. On this basis, safety precautions were implemented, such as the installation of a hydrogen leak detection system. This will reduce both the social and personal risks to meet the ALARP standard, with a reduction in the individual risk to one-tenth of its initial level. In a QRA study on the most recent hydrogen refueling station model in Japan, Suzuki et al. (2021) reported that jet fires are the main source of risk and that countermeasures need to be implemented, such as using firewalls or reducing the time during which hydrogen is released continuously.
Furthermore, Yoo et al. (2021) established a hydrogen refueling process based on the design drawings of real gaseous hydrogen refueling stations and liquid hydrogen refueling stations reported in the literature. They aimed at comparing the risks of both types of stations. Then, a QRA study was conducted for each case. The findings highlighted that catastrophic pipeline trailer and liquid hydrogen storage tank ruptures are the most serious incidents in both cases. It was shown that both scenarios can result in dangerous fires and explosions, with the fires in gaseous hydrogen refueling stations and the explosions in liquid hydrogen refueling stations having the greater impact. Overall, the risk of gaseous hydrogen refueling stations is higher than that of liquid hydrogen refueling stations. However, both station types require implementing additional safety measures to lower the risk, such as installing detachable couplings. In this regard, integrating hydrogen sensors and the corresponding automatic emergency shutdown devices can lower the risk below acceptable standards. These findings are consistent with the assessment results for personal and societal risks. Also, it is clear that there are substantial differences between the risks associated with gaseous hydrogen and liquid hydrogen refueling stations. As such, the findings of the risk assessment conducted on gaseous hydrogen refueling stations cannot be extended directly to characterize liquid hydrogen refueling stations.
It is concluded that FTA, which has high data needs, may systematically discover root causes in hydrogen leakage analysis; Event Tree Analysis (ETA) is a useful tool for analyzing outcomes and is simple to visualize, but it necessitates a thorough comprehension of causality; Although it requires a lot of processing power, Monte Carlo simulation can handle a large range of uncertainty and produce probability distributions; Although difficult and computationally demanding to build, Bayesian networks are effective at handling uncertainty and complexity and integrating with expert knowledge; While risk matrices are clear-cut and easy to comprehend, allowing for quick assessment, they are very subjective and challenging to thoroughly analyze. Bayesian networks, on the other hand, are efficient at handling uncertainty, complexity, and combining expert knowledge, but they are difficult to build and computationally intensive. A more thorough evaluation of the danger of hydrogen leaking can be obtained by combining several models. Although they are quite subjective, risk matrices are straightforward and easy to understand.
8 Conclusion and future perspectives
The review and analysis conducted in this study revealed major gaps in the current research on explosion incidents due to liquid hydrogen leaks, along with flaws related to the risk assessment methods of liquid hydrogen refueling station:
(1) More complex models can be built to carry out numerical simulation of liquid hydrogen leakage accidents occurring in liquid hydrogen refueling stations from multiple perspectives and directions. This is because only the leakage in the direction of the key equipment has been simulated when creating a plume model of liquid hydrogen jet leakage from a real liquid hydrogen refueling station.
(2) The frequency of hydrogen leakage events in the quantitative risk assessment study of actual liquid hydrogen refuelling stations is primarily based on data from foreign literature published in the last 10 years. It is discovered that there is still a dearth of basic leakage data on hydrogen, and the values used by various literatures vary as well. Since there are very few liquid hydrogen leakage data, in particular, it is imperative to create a database of basic leakage frequency of hydrogen equipment appropriate for China’s national conditions.
(3) Although some researchers have applied quantitative risk assessment for the safety evaluation of hydrogen refueling stations, most of the presented work has considered gas hydrogen refueling stations, with only a few investigations dealing with actual liquid hydrogen refueling stations.
(4) For China, it is essential to set appropriate national standards, remove hydrogen from the list of hazardous chemicals, and incorporate it into fuels in order to encourage the successful construction of hydrogen refueling stations. These actions will help China’s civil hydrogen energy utilization and hydrogen refueling station industries grow.
(5) New types of hydrogen leakage protection measures have yet to be researched, e.g. mobile air curtains, water curtains for emergency conditions, etc.
It should be highlighted that multidisciplinary research and cooperation between government, business, and academia are crucial for advancing the security and effectiveness of hydrogen filling stations. Multidisciplinary research fosters the integration of expertise from several domains and can address the safety and efficiency issues with hydrogen fuel filling stations from several angles. Research findings may be translated, technology can be made more useful, and hydrogen fuel filling station safety and operational efficiency can all be guaranteed through collaboration between academics and industry. In addition to having a major role to play in developing pertinent legislation, the government may guarantee that all parties have common interests by supporting the development of hydrogen infrastructure financially and politically.
Author contributions
YR: Conceptualization, Writing–original draft. WY: Conceptualization, Investigation, Writing–original draft. JP: Conceptualization, Investigation, Writing–original draft. JH: Conceptualization, Investigation, Methodology, Writing–review and editing. JG: Formal Analysis, Resources, Writing–original draft. XZ: Writing–review and editing. JC: Methodology, Supervision, Writing–review and editing. SC: Conceptualization, Writing–review and editing.
Funding
The author(s) declare that no financial support was received for the research, authorship, and/or publication of this article.
Conflict of interest
Authors YR, JG, XZ, and SC were employed Power China Huadong Engineering Corporation Limited.
The remaining authors declare that the research was conducted in the absence of any commercial or financial relationships that could be construed as a potential conflict of interest.
Publisher’s note
All claims expressed in this article are solely those of the authors and do not necessarily represent those of their affiliated organizations, or those of the publisher, the editors and the reviewers. Any product that may be evaluated in this article, or claim that may be made by its manufacturer, is not guaranteed or endorsed by the publisher.
References
Aditiya, H. B., and Aziz, M. (2021). Prospect of hydrogen energy in Asia-Pacific: a perspective review on techno-socio-economy nexus. Int. J. Hydrogen Energy 46 (71), 35027–35056. doi:10.1016/j.ijhydene.2021.08.070
Alliance, C. H. E. (2021). China’s hydrogen energy and fuel cell industry white paper 2020. Beijing, China: People’s Daily Press.
Author Anonymous (2024a). “Fuel specification for hydrogen powered vehicles—liquid hydrogen(LH2),” in GB/T 40045-2021. (in Chinese).
Author Anonymous (2024b). “Technical requirements for storage and transportation of liquid hydrogen,” in GB/T 40060-2021. (in Chinese).
Author Anonymous (2024c). “Technical specification for liquid hydrogen production system,” in GB/T 40061-2021. (in Chinese).
Casamirra, M., Castiglia, F., Giardina, M., and Lombardo, C. (2009). Safety studies of a hydrogen refuelling station: determination of the occurrence frequency of the accidental scenarios. Int. J. Hydrogen Energy 34 (14), 5846–5854. doi:10.1016/j.ijhydene.2009.01.096
Chen, J., Gao, X., Shao, S., Hu, H., Xie, J., Li, N., et al. (2021). Numerical investigation of the vortex tube performance in novel precooling methods in the hydrogen fueling station. Int. J. Hydrogen Energy 46 (7), 5548–5555. doi:10.1016/j.ijhydene.2020.11.070
Chen, J., Xiao, L., Wu, Y., Gao, X., Chen, H., Xie, J., et al. (2022). Dynamic simulation of the potential of integrating a turbo-expander in a hydrogen refueling station. Appl. Therm. Eng. 202, 117889. doi:10.1016/j.applthermaleng.2021.117889
Genovese, M., and Fragiacomo, P. (2023). Hydrogen refueling station: overview of the technological status and research enhancement. J. Energy Storage 61, 106758. doi:10.1016/j.est.2023.106758
Giannissi, S. G., and Venetsanos, A. G. (2018). Study of key parameters in modeling liquid hydrogen release and dispersion in open environment. Int. J. Hydrogen Energy 43 (01), 455–467. doi:10.1016/j.ijhydene.2017.10.128
Groethe, M., Merilo, E., Colton, J., Chiba, S., Sato, Y., and Iwabuchi, H. (2007). Large-scale hydrogen deflagrations and detonations. Int. J. Hydrogen Energy 32 (13), 2125–2133. doi:10.1016/j.ijhydene.2007.04.016
Gye, H., Seo, S., Bach, Q., Ha, D., and Lee, C. J. (2019). Quantitative risk assessment of an urban hydrogen refueling station. Int. J. Hydrogen Energy 44 (2), 1288–1298. doi:10.1016/j.ijhydene.2018.11.035
Hansen, O. R. (2020). Liquid hydrogen releases show dense gas behavior. Int. J. Hydrogen Energy 45 (02), 1343–1358. doi:10.1016/j.ijhydene.2019.09.060
Holborn, P. G., Benson, C. M., and Ingram, J. M. (2020). Modelling hazardous distances for large-scale liquid hydrogen pool releases. Int. J. Hydrogen Energy 45 (43), 23851–23871. doi:10.1016/j.ijhydene.2020.06.131
Hooker, P., Willoughby, D. B., and Hall, J. (2011). “Experimental releases of liquid hydrogen,” in International Conference on Hydrogen Safety, Derbyshire, United Kingdom, 12 Sept. 2011 (Health and Safety Laboratory), 1–10.
Ichard, M., Hansen, O. R., Middha, P., and Willoughby, D. (2012). CFD computations of liquid hydrogen releases. Int. J. Hydrogen Energy 37 (22), 17380–17389. doi:10.1016/j.ijhydene.2012.05.145
Jiang, C. (2009). Accident investigation and analysis techniques. Chemical Industry Press. (in Chinese).
Kikukawa, S., Mitsuhashi, H., and Miyake, A. (2009). Risk assessment for liquid hydrogen fueling stations. Int. J. Hydrogen Energy 34 (2), 1135–1141. doi:10.1016/j.ijhydene.2008.10.093
Kikukawa, S., Yamaga, F., and Mitsuhashi, H. (2008). Risk assessment of Hydrogen fueling stations for 70MPa FCVs. Int. J. Hydrogen Energy 33 (23), 7129–7136. doi:10.1016/j.ijhydene.2008.08.063
Kim, E., Park, J., Cho, J. H., and Moon, I. (2013). Simulation of hydrogen leak and explosion for the safety design of hydrogen fueling station in Korea. Int. J. Hydrogen Energy 38 (3), 1737–1743. doi:10.1016/j.ijhydene.2012.08.079
Kuroki, T., Sakoda, N., Shinzato, K., Monde, M., and Takata, Y. (2018). Dynamic simulation for optimal hydrogen refueling method to Fuel Cell Vehicle tanks. Int. J. Hydrogen Energy 43 (11), 5714–5721. doi:10.1016/j.ijhydene.2018.01.111
Li, J. (2015). Investigation on strategy of fast refueling and consequence of leakage-explosion of high pressure hydrogen in hydrogen station. Zhejiang University. (in Chinese).
Li, J., Zhao, Y., and Zheng, J. (2015). Simulation and analysis on leakage and explosion of highpressure hydrogen in hydrogen refueling station. J. Zhejiang Univ. Eng. Sci. 49 (7), 1389–1394. (in Chinese).
Li, Z., Pan, X., and Ma, J. (2012). Quantitative assessment on hydrogen releases of hydrogen refueling station by consequence modeling. J. Tongji Univ. Nat. Sci. 40 (2), 286–291. (in Chinese).
Liang, Y., Pan, X., Zhang, C., Xie, B., and Liu, S. (2019). The simulation and analysis of leakage and explosion at a renewable hydrogen refuelling station. Int. J. Hydrogen Energy 44 (40), 22608–22619. doi:10.1016/j.ijhydene.2019.05.140
Liu, C., Lin, H., and Hu, M. (2024). Development status and outlook of hydrogen powered fuel cell vehicle market in China. South. energy Constr. 11 (2), 162–171. (in Chinese). doi:10.16516/j.ceec.2024.2.16
Liu, Y., Wei, J., Lei, G., Wang, T., Lan, Y., Chen, H., et al. (2019). Modeling the development of hydrogen vapor cloud considering the presence of air humidity. Int. J. Hydrogen Energy 44 (03), 2059–2068. doi:10.1016/j.ijhydene.2018.11.123
Middha, P., and Hansen, O. R. (2009). Using computational fluid dynamics as a tool for hydrogen safety studies. J. Loss Prev. Process Industries 22 (3), 295–302. doi:10.1016/j.jlp.2008.10.006
Middha, P., Hansen, O. R., Groethe, M., and Arntzen, B. J. (2007). “Hydrogen explosion study in a confined tube: FLACS CFD simulations and experiments,” in 21st International Colloquium of Dynamics of Explosions and Reactive Systems (ICDERS), Poitiers, France, GexCon AS, July 23–27, 2007, 1–6.
Middha, P., Ichard, M., and Arntzen, B. J. (2011). Validation of CFD modelling of LH2 spread and evaporation against large-scale spill experiments. Int. J. Hydrogen Energy 36 (03), 2620–2627. doi:10.1016/j.ijhydene.2010.03.122
Midilli, A., Ay, M., Dincer, I., and Rosen, M. (2005). On hydrogen and hydrogen energy strategies: I: current status and needs. Renew. Sustain. Energy Rev. 9 (3), 255–271. doi:10.1016/j.rser.2004.05.003
Molkov, V. (2012). Fundamentals of Hydrogen Safety Engineering II - eBooks and textbooks from bookboon.com. Northern Ireland. Energy Eng.
Nakayama, J., Sakamoto, J., Kasai, N., Shibutani, T., and Miyake, A. (2016). Preliminary hazard identification for qualitative risk assessment on a hybrid gasoline-hydrogen fueling station with an on-site hydrogen production system using organic chemical hydride. Int. J. Hydrogen Energy 41 (18), 7518–7525. doi:10.1016/j.ijhydene.2016.03.143
Pu, L., Shao, X., Li, Q., and Li, Y. (2018). A simple and effective approach for evaluating unconfined hydrogen/air cloud explosions. Int. J. Hydrogen Energy 43 (21), 10193–10204. doi:10.1016/j.ijhydene.2018.04.041
Pu, L., Tang, X., Shao, X., Lei, G., and Li, Y. (2019). Numerical investigation on the difference of dispersion behavior between cryogenic liquid hydrogen and methane. Int. J. Hydrogen Energy 44 (39), 22368–22379. doi:10.1016/j.ijhydene.2019.05.219
Qian, He (2021). Study on cryo-compressed hydrogen releases and explosions. Shandong University. (in Chinese).
Shiruill, L. C., Roberts, T. A., Royle, M., Willoughby, D., and Gautier, T. (2012). Safety studies on high-pressure hydrogen vehicle refuelling stations: releases into a simulated high-pressure dispensing area. Int. J. Hydrogen Energy 37 (08), 6949–6964. doi:10.1016/j.ijhydene.2012.01.030
Song, Y., Yu, L., and Li, C. (2005). TNT equivalent method predicts the consequence that some petrochemical industry equipment explodes. J. Saf. Sci. Technol. (03), 66–68. (in Chinese).
Statharas, J. C., Venetsanos, A. G., Bartzis, J. G., Würtz, J., and Schmidtchen, U. (2000). Analysis of data from spilling experiments performed with liquid hydrogen. J. Hazard. Mater. 77 (1-3), 57–75. doi:10.1016/s0304-3894(00)00252-1
Suzuki, T., Shiota, K., Izato, Y., Komori, M., Sato, K., Takai, Y., et al. (2021). Quantitative risk assessment using a Japanese hydrogen refueling station model. Int. J. Hydrogen Energy 46 (11), 8329–8343. doi:10.1016/j.ijhydene.2020.12.035
Tanaka, T., Azuma, T., Evans, J. A., Cronin, P., Johnson, D., and Cleaver, R. (2007). Experimental study on hydrogen explosions in a full-scale hydrogen filling station model. Int. J. Hydrogen Energy 32 (13), 2162–2170. doi:10.1016/j.ijhydene.2007.04.019
Tang, X., Pu, L., Shao, X., Lei, G., and Wang, X. (2020). Dispersion behavior and safety study of liquid hydrogen leakage under different application situations. Int. J. Hydrogen Energy 45 (55), 31278–31288. doi:10.1016/j.ijhydene.2020.08.031
van den Berg, A. C. (1985). The multi-energy method: a framework for vapour cloud explosion blast prediction. J. Hazard. Mater. 12 (01), 1–10. doi:10.1016/0304-3894(85)80022-4
Verfondern, K., and Dienhart, B. Pool spreading and vaporization of liquid hydrogen[J]. Int. J. Hydrogen Energy, 2007,32(13):2106–2117. doi:10.1016/j.ijhydene.2007.04.015
Willoughby, D., and Royle, M. (2014). Releases of unignited liquid hydrogen. Derbys. Health Saf. Exec.
Witcofski, R., and Chirivella, J. (1984). Experimental and analytical analyses of the mechanisms governing the dispersion of flammable clouds formed by liquid hydrogen spills. Int. J. Hydrogen Energy 5 (9), 425–435. doi:10.1016/0360-3199(84)90064-8
Yoo, B., Wilailak, S., Bae, S., Gye, H. R., and Lee, C. J. (2021). Comparative risk assessment of liquefied and gaseous hydrogen refueling stations. Int. J. Hydrogen Energy 46 (71), 35511–35524. doi:10.1016/j.ijhydene.2021.08.073
Zabetakis, M. G., and Burgess, D. S. (1959). Research on the hazards associated with the production and handling of liquid hydrogen. Washington: Bureau of Mines.
Zhang, L. (2019). Research on risk evaluation of fuel cell vehicle hydrogen refueling station. Chongqing University. in Chinese.
Zhang, Q., Chen, W., and Ling, W. (2022). Policy optimization of hydrogen energy industry considering government policy preference in China. Sustain. Prod. Consum. 33, 890–902. doi:10.1016/j.spc.2022.08.017
Zhang, W., Lv, D., and Wang, J. (2010). Comparison of vapor cloud explosion (VCE) consequences prediction models. Industrial Saf. Environ. Prot. 36 (4), 48–49. (in Chinese).
Zhiyong, L., Xiangmin, P., and Jianxin, M. (2010). Quantitative risk assessment on a gaseous hydrogen refueling station in Shanghai. Int. J. Hydrogen Energy 35 (13), 6822–6829. doi:10.1016/j.ijhydene.2010.04.031
Keywords: China hydrogen energy policy, liquid hydrogen leakage, explosive overpressure, risk assessment, hydrogen refueling stations
Citation: Rong Y, Yuan W, Peng J, Hou J, Gao J, Zhang X, Chen J and Chen S (2024) An review of research on liquid hydrogen leakage: regarding China’s hydrogen refueling stations. Front. Energy Res. 12:1408338. doi: 10.3389/fenrg.2024.1408338
Received: 12 April 2024; Accepted: 23 August 2024;
Published: 03 September 2024.
Edited by:
Tong Liu, Wuhan Institute of Technology, ChinaReviewed by:
Libin Lei, Guangdong University of Technology, ChinaKunal Mondal, Oak Ridge National Laboratory (DOE), United States
Copyright © 2024 Rong, Yuan, Peng, Hou, Gao, Zhang, Chen and Chen. This is an open-access article distributed under the terms of the Creative Commons Attribution License (CC BY). The use, distribution or reproduction in other forums is permitted, provided the original author(s) and the copyright owner(s) are credited and that the original publication in this journal is cited, in accordance with accepted academic practice. No use, distribution or reproduction is permitted which does not comply with these terms.
*Correspondence: Jianye Chen, amlhbnllX2NoZW5AaHVzdC5lZHUuY24=