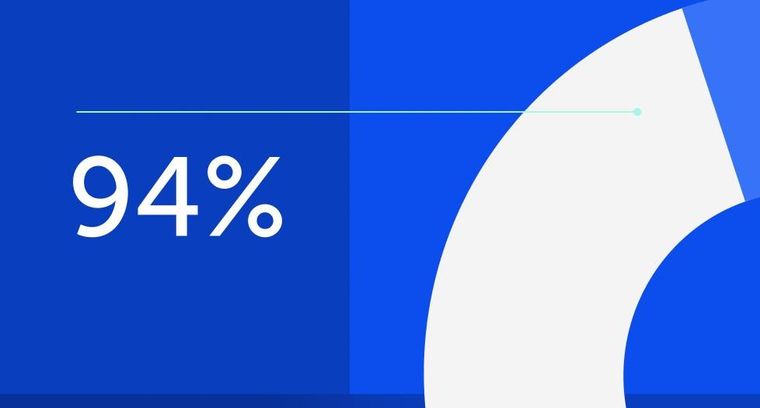
94% of researchers rate our articles as excellent or good
Learn more about the work of our research integrity team to safeguard the quality of each article we publish.
Find out more
ORIGINAL RESEARCH article
Front. Energy Res., 14 May 2024
Sec. Sustainable Energy Systems
Volume 12 - 2024 | https://doi.org/10.3389/fenrg.2024.1397037
This article is part of the Research TopicAdvancing Urban Sustainability: Integrating Renewable Energy for Accelerated Zero-Carbon Community TransitionsView all 7 articles
Supermarkets in Nigeria rely on diesel generators for electricity due to the unreliability of the national grid. The recent removal of petroleum subsidies in 2023 has increased fuel prices by 60%. This research examines the technical, economic, and environmental viability of employing solar PV/battery storage/generator systems to generate electricity for high-load supermarkets. The case study was conducted in the Market Square (MS) supermarket in Port Harcourt (PH) city, Nigeria. The MS supermarket had a load demand of 59.8 kW/day for an energy audit in 2022. The average solar radiation and temperature for PH city were 4.21 kWh/m2 and 25.3°C, respectively. The hybrid system was simulated with the HOMER Pro software. The simulation revealed that the optimum baseline (BL) system for the present price of a liter of diesel in Nigeria ($0.63 USD/L) was a solar PV/Battery/Generator. The optimal BL system produced 401,599 kWh/year, which was more than adequate to cover the yearly load requirement of 204,765 kWh/year and left a surplus of 173,195 kWh/year. The BL system had a levelized cost of electricity of $0.106 USD/kWh, a net present cost of $232,533, and Operation and Maintenance cost of $7,928. When the overall environmental impact of the optimal BL system was assessed, it contributed 10,935 kg CO2-eq/year of global warming potential, 1,611 kg O3-eq/year of smog formation, and 72.2 kg SO2-eq/year of acidification potential to the environment. Sensitivity analysis shows that the BL system could have a net present value of $710,364, a 38% internal rate of return, and a 2-year simple payback period over a 25-year life if the excess energy is sold to the grid. Also, LCOE increases with fuel price or discount rate increase, while CO2 falls as all renewable hybrid system configurations become more advantageous. The potential reduction in CO2 emission in the proposed system highlights the environmental benefits compared to traditional ones. This finding will guide decisions on adopting hybrid energy solutions for supermarkets in Nigeria. This analysis offers crucial insights for energy sector decision-makers seeking to balance reliability, cost-effectiveness, and environmental sustainability in a volatile market.
Electricity is a fundamental necessity across all sectors (manufacturing, education, health, finance, agriculture, mining, construction, etc.) and is therefore indispensable for the growth of any economy. The International Energy Agency (IEA) estimates that around 675 million people worldwide lack access to electricity (Basic energy access lags amid renewable opportunities, 2024). Of particular concern are those living in sub-Saharan Africa, which account for 80% of this figure, highlighting the considerable disparity in energy access (Access to electricity SDG7: Data and Projections Analysis, 2024). This disparity is further exacerbated in Nigeria, where rapid population growth outpaces energy generation, illustrating the severe obstacles West African country faces in achieving universal access to energy (For the first time in decades and the number of people without access to electricity is set to increase in 2022—Analysis, 2023). Lagging energy access and the unreliability of the national grids have contributed to sluggish economic growth in Nigeria. This energy shortage has hindered the improvement of living standards for a sizable portion of the Nigerian population, as it compromises the accessibility of vital provisions, including education and healthcare.
A 2022 World Bank study estimates that just 8% of West African rural settlements, or roughly 42% of the overall population, have access to electricity. The region’s limited access to electricity directly contributes to its acute energy poverty. Further aggravating the problem of energy scarcity in the area are the high electricity costs for households in sub-Saharan Africa. This energy shortfall exacerbates these communities’ development issues, impeding economic growth and limiting educational and healthcare opportunities. Improving the availability of reliable and cost-effective electricity is crucial for supporting long-term development and improving living conditions in West Africa (Mobilizing Clean Energy to Address Energy Poverty in West Africa The Climate Reality Project, 2023; Doris Dokua Sasu, 2024).
Nigeria, located in Western Africa, has a population of approximately 220 million. The population is expected to grow by more than 40% to reach 370 million by 2050, at an annual growth rate of 2.78% (Nigeria Population, 2023). The country consumes 24.61 billion kWh of electricity annually, averaging 113 kWh per person (Energy consumption in Nigeria, 2023). According to Worldometer, 53.9% of Nigerians live in cities, with a median age of 17.2 (Nigeria Demographics, 2023). The growing population and increasing affluence will cause energy demand, especially in metropolitan areas, to rise quickly. Nigeria can be energy self-sufficient, with facilities capable of producing 29 billion kWh of electricity (or 119% of its consumption); yet the energy produced is sold to other nations or unused (Energy consumption in Nigeria, 2023). For the country to optimize the developmental advantages of its energy output, resources should be allocated toward infrastructure investment and providing incentives for domestic consumption.
Nigeria faces an unstable national grid and relies heavily on premium motors (PMS) and diesel generators and is the largest importer of such generators in Africa (Nigeria leads Africa as the highest generator importer - Nairametrics, 2023). This reliance is highlighted by the fact that 37 of the country’s 43 federal universities use generators as an alternative source of energy (Heavy use of generators puts Nigeria’s climate plans in jeopardy - Punch Newspapers, 2023). The German Development Agency GIZ reports that 86% of businesses in Nigeria own or share a generator, contributing to 46% of the country’s electricity production (Ozoegwu and Akpan, 2021). Notably, Nigeria spent $243.6 million on electrical generator and converter imports in 2016 alone (Heavy use of generators puts Nigeria’s climate plans in jeopardy - Punch Newspapers, 2023), and small and medium-sized businesses and families spend $21.8 billion a year on gasoline and diesel to power their generators. This reliance on diesel- and petrol-powered generators, which account for 75% of consumed electricity and 25 GW of installed capacity, starkly contrasts with the mere 5 GW supplied by the national grid (Nigeria relies on generators for 75% electricity—Report, 2023). In 2020, Nigeria emitted 111.9 million tons of CO2 (Energy consumption in Nigeria, 2023). Nigeria’s heavy reliance on generators strains the economy and contributes to environmental deterioration and carbon emissions.
The removal of petroleum subsidies in Nigeria (Amnesty International, 2023) has led to a surge in diesel costs, consequently increasing the cost of living and exerting a considerable financial burden on Nigerians. Because the citizens relied on diesel generators to generate electricity, the rise in diesel costs has increased electricity costs. The increased cost of electricity has impacted businesses and households, impeding economic development and growth. Commercial centers in Nigeria are the largest electricity consumers, primarily due to the need for refrigeration and air conditioning systems to maintain product freshness and customer comfort. These electrical equipment’s contribute significantly to urban areas’ energy demand and carbon footprint. In response to these challenges, there is an increasing demand for cost-effective and sustainable energy alternatives to fossil-fuel-based power generation. Nigeria generates only 3% of its 29.4 billion kWh of electricity from solar and biomass energy, 21.7% from hydropower, and 78.1% from fossil fuels (Energy consumption in Nigeria, 2023).
Microgrids present a hopeful alternative for providing electricity to African communities and transitioning to sustainable energy. They offer the advantages of reduced expenses, more adaptability, and simplified incorporation of renewable energy sources (How microgrids can electrify rural Africa | Africa at LSE, 2024; Accelerating access to energy in Africa with solar microgrids: A study - Schneider Electric Blog, 2024). Microgrids in underdeveloped nations can mitigate power supply intermittently by storing excess energy during periods of high generation and discharging it during periods of low or no generation. Additionally, they function as contingency systems in the event of grid disturbances, improving dependability and facilitating consistent power availability (Bukari et al., 2021). The excess energy can be sold to nearby commercial centers and business hubs. Mini-grid and microgrid developers prioritize energy-efficient applications using local business hubs to boost excess energy demand and create adequate revenue for financial viability. By prioritizing energy-efficient applications, developers of mini-grids may ensure that the surplus energy produced from renewable sources is utilized efficiently. Not only does this enhance stability and provide consistent power availability, but it also stimulates economic development in local regions by selling excess energy to businesses (Energy and Environment Partnership EEP NDF, 2018).
Microgrids involve the combination of various sources of energy, both renewable and non-renewable. Microgrids, powered by solar panels, wind turbines, batteries, and diesel generators, offer communities or facilities a reliable and sustainable energy supply (How microgrids can electrify rural Africa Africa at LSE, 2024). Renewable energy resources might offer long-term cost savings and environmental benefits, reducing reliance on fossil fuels and carbon emissions (Bukari et al., 2021). The promotion of renewable energy infrastructure can generate employment and attract foreign investment, bolstering the national economy (Llera Sastresa et al., 2010). With a tropical climate and an annual sunlight duration of 4 months, Nigeria has the potential for solar photovoltaic (PV) systems. The solar resource’s potential is significant, with a solar radiation range between 4 and 6 kWh/m2 (Okoro and Chineke, 2021; Page, 2012). Integrating PV systems could improve energy accessibility, reduce reliance on diesel generators and contribute to reducing greenhouse gas emissions (GHG), thereby mitigating the adverse effects of climate change.
Solar photovoltaic (PV) applications directly convert solar radiation into electrical energy. The predominant approach for achieving this objective is using silicon solar cells, known as solar modules. The solar module is the power generating unit, comprising multiple solar cells electrically interconnected on a base plate (Ajao et al., 2011). A solar PV system consists of several essential components comprising arrays of solar cells, inverter equipment that converts direct current (DC) to alternating current (AC) and supplies regulated voltage and current outputs, a charge controller that automatically operates the entire system, and battery storage that is optional for stand-alone systems (Ijeoma et al., 2023). The interconnection of these systems facilitates renewable energy generation.
Renewable energy systems are limited due to their unpredictable nature and potential unreliability. On overcast days, a stand-alone solar energy system may not deliver electricity continuously due to fluctuations in weather conditions. Similarly, an independent wind energy system may not provide sufficient energy for an extended period due to cut-in wind speed. Therefore, integrating multiple renewable energy sources and storage systems is crucial for a steady and dependable power supply (Adaramola et al., 2014; Wind and solar are not intermittent they are unreliable et al., 2024; The Reliability Debate: Renewables Sustainable Review, 2024). This combination reduces the intermittent nature of each system, resulting in a more reliable energy system. The diesel generator or storage unit can serve as a contingency power source in cases of insufficient renewable sources (Come et al., 2021; Can diesel power plants be integrated with renewable energy sources? by Brenda Rose Medium, 2024; Power Plant Generator Woodstock Power Company, 2024).
Using multiple energy sources in hybrid energy systems enhances power supply consistency and reliability, mitigating weather changes and maintaining a consistent electrical supply even when a single energy source is not operating at maximum capacity (Adaramola et al., 2014; Come et al., 2021). Numerous studies have shown that hybrid power systems are more reliable and cost-effective than single-source energy systems based on viability, dependability, and economic assessments (Nfah and Ngundam, 2008; Ajao et al., 2011; Adaramola et al., 2012a; Tijani et al., 2014; Oladigbolu et al., 2019; Yimen et al., 2020; Al-Rawashdeh et al., 2023a; White et al., 2024). Moreover, integrating diverse energy sources might lead to long-term cost reductions compared to depending only on a single form of renewable energy system (Oladigbolu et al., 2019; Yimen et al., 2020). Several studies on hybrid energy systems show an enormous potential to reduce reliance on fossil fuels and GHG emissions. These systems also provide a reliable and continuous electricity supply, offering significant economic advantages (Nema et al., 2010; Ajlan et al., 2017; Goel and Sharma, 2017; Abdelhady, 2023; Al-Rawashdeh et al., 2023b).
The growing popularity of these technologies highlights their potential as sustainable energy solutions in Nigeria. Several hybrid energy systems studies have been conducted in the West African region to show their viability for energy generation in the region. Adaramola (Adaramola, 2014) investigated the feasibility of an 80 kW on-grid solar PV system for electricity generation in Jos, Nigeria, using the HOMER software. The results indicate that a grid-connected PV system can generate 40% of the required electricity based on a daily solar radiation of 6.0 kW/h/m2, an anticipated annual electricity production of 331,538 kWh, with a levelized cost of electricity (LCOE) of $0.103 USD/kWh.
Tijani et al. (Tijani et al., 2014) conducted a techno-economic analysis of a hybrid PV-diesel-battery off-grid system for an international college in Northern Nigeria, utilizing the HOMER software for their analysis. The study revealed that a system with a 120 kW PV peak output required 972 m2 of solar panels and two batteries (1156 Ah, 12 V), resulting in an LCOE of $0.566 USD/kWh and carbon emissions of 14,410 kg CO2/year. When compared to a standalone diesel generator system (which emits about 141,354 kg of CO2/year and incurs an LCOE of $0.542 USD/kWh), the hybrid system showed an 89.8% reduction in carbon emissions. The researchers highlighted that the hybrid system could provide reliable and sustainable electricity to facilitate innovative research (Muzan et al., 2012), markedly diminishing the college’s reliance on diesel fuel and providing environmental benefits.
Ukoima et al. (2023) conducted a techno-economic analysis of a solar-wind electricity generation system to meet the load requirement of a rural community in Rivers State, Nigeria, using RETScreen software. They proposed a model comprising a 600 kW PV system and a 50-kW wind system. The system generates 902 MWh annually with a net present cost (NPC) of $870,000 USD and an NPV of $3,409,532 when the LCOE is $0.2 USD/kWh.
Adaramola et al., 2017 assessed the efficacy of a hybrid system, including PV and biodiesel generators, to address the electrical and household water demands of a remote village in Ghana. Their findings indicated the LCOE was $0.76 USD/kWh under standard financing conditions (without grants). Nevertheless, the LCOE was reduced to $0.20 USD/kWh when the project received financial support through an initial grant. The substantial decrease in the LCOE underscores the crucial role of financial assistance in enhancing the affordability and accessibility of renewable energy initiatives for isolated populations. Governments and organizations may have a significant impact on fostering sustainable development and enhancing the quality of life in neglected communities by offering funds in advance.
Odou et al., 2020 study on the electrification of villages in the Benin Republic recommends a hybrid PV-diesel generator-battery storage system as the best choice for electrification. The system has the lowest net present cost, provides a reliable power supply, and reduces battery storage costs. It has the shortest payback period of 3.5 years, a 33.3% internal rate of return, and an LCOE of $0.207 USD/kWh. The study suggests that a hybrid system is a reliable solution for Benin Republic’s rural electrification master plan, aiming to provide sustainable and affordable electricity to all villages, thereby bridging the urban-rural electrification gap and enhancing the quality of life for underserved residents.
Eze et al., 2022 assessed the viability of hybrid energy systems in institutional buildings in Kenya by also employing HOMER. The optimal architecture was found to be a PV-grid-diesel system with an LCOE of $0.05 USD/kWh, an NPC of $452,700 and an initial investment of $197,096. Researchers reported a 77% reduction in grid power purchases because of the hybrid system and an 84% reduction in electricity expenses. The decrease in electricity cost and grid power purchases underscores the potential financial benefits associated with the adoption of hybrid energy systems.
Modu et al., 2018 performed a techno-economic study of four systems in Katsina, Northern Nigeria, using the HOMER. The systems studied were 1: off-grid PV, 2: standalone diesel generating plants, 3: PV-diesel, and 4: PV-diesel-batteries. The PV-diesel-battery system exhibited the lowest LCOE among the three systems. This finding suggests that integrating batteries with the PV-diesel system significantly reduces the overall cost of electricity generation in off-grid areas. The PV-diesel-battery system exhibited the lowest LCOE among the three systems. This finding suggests that integrating batteries with the PV-diesel system significantly reduces the overall cost of electricity generation in off-grid areas.
Olatomiwa et al., 2018 proposes an off-grid hybrid renewable energy system for medical clinics in remote Nigeria. The researchers utilized HOMER software to analyze wind and solar sources to develop an ideal system. They determined that the PV-wind-diesel-battery hybrid system, which had an NPC of $102,949 and an LCOE of $0.311 USD/kWh, was optimal for a health center in a rural location.
The literature review highlights the limited studies in the South-South region of Nigeria, specifically PH City, focusing on applying hybrid energy systems in commercial centers (supermarkets). Following the Nigerian government’s termination of fuel subsidies (Amnesty International, 2023), diesel prices have exhibited instability and a persistent upward trend. In response, we developed a hybrid energy system considering Nigeria’s current diesel price of $0.673 USD/L. Then, we conducted a comprehensive sensitivity analysis to examine how fluctuations in diesel prices affect the performance and economic viability of hybrid energy configurations.
The main goal of this analysis is to identify an optimal energy solution that provides consistent reliability, reduces environmental impacts, and maintains cost-effectiveness for supermarkets in Nigeria, considering the rising fuel prices. The study also evaluated the environmental benefits of implementing hybrid energy systems and addressed the following objectives:
• Analyze the technical viability of hybrid energy systems. This study used data from various supermarkets in PH City and designed systems to meet their specific load demands by analyzing combinations of solar PV/battery, solar PV/diesel generator, and solar PV/battery/diesel generator. These systems were assessed to identify an optimal solution for these supermarkets.
• Conduct economic analysis of the hybrid energy systems and assess the most cost-effective solutions to meet the supermarket load demand based on various financial parameters.
• Evaluate the environmental impact of the hybrid energy system under different impact categories such global warming potential, acidification potential, smog formation, etc., and analyze the potential implications of these impact categories on human health and the ecosystem.
• Conduct a sensitivity analysis on the real implications of the change in fuel price and discount rate on the optimal energy system. Additionally, consider the potential long-term benefits of investing in renewable energy sources for supermarkets.
The findings of this analysis can provide valuable insights for decision-makers in the energy sector looking to balance reliability, cost-effectiveness, and environmental sustainability in a volatile market.
This section covers the components used in the HOMER Pro system design and simulation. The study focuses on the supermarkets in Port Harcourt (PH) City in the south-south area of Nigeria, building on a previous study by Ijeoma et al., 2023. A Solar PV/Generator/Battery storage system was simulated.
Port Harcourt (PH) City, located in the south-south region of Nigeria at 4.78° N latitude and 7.01° E longitude, has an estimated population of 3.6 million, ranking it as the fifth most populous metropolis within the nation (Port Harcourt Population, 2024). The city’s electrical supply is notably unreliable, commercial centers (known as supermarkets in this study) rely solely on diesel generators for their electricity. In this study, we examine the financial implications of electricity generation for supermarkets in PH City, considering the rising diesel price and exploring viable alternatives to the prevailing reliance on diesel generators across Nigeria.
Figure 1 shows the schematic design of the procedure, including the system input and output parameters used in the HOMER Pro software simulation and analysis.
Figure 1. System analysis procedure used by HOMER Pro for the system optimization analysis performed in this study.
In a solar PV-battery-diesel generator hybrid energy system, the sun’s energy strikes the PV solar cells, producing electricity. This electricity is then regulated by a maximum power point tracking (MPPT) charge controller, which controls the current and voltage that exits the PV array (Kumar et al., 2021). When the PV system fails to generate electricity or energy demand rises, the battery system serves as a backup energy storage system, ensuring consistent performance (IRENA, 2023). An inverter converts direct current (DC) from the PV array and batteries into alternating current (AC) to fulfill load requirements (HIES Renewable Energy, 2023). In a microgrid with a diesel generator, the generator also acts as a backup system when the PV system is not producing any energy/electricity. The diesel generator can provide power during periods of low solar radiation and supplement power during high energy demand or when the battery system is depleted (Ndukwe and Iqbal, 2019). This hybrid system offers reliable electricity supply in various conditions, ensuring uninterrupted power for critical loads.
HOMER assesses the hybrid system’s feasibility using technical and economic parameters like initial cost, life cycle cost, levelized electricity cost, net present value, and simple payback periods. This comprehensive evaluation aids in decision-making regarding system implementation based on its overall feasibility and cost-effectiveness, ensuring informed decision-making. The technical and economic parameters are explained in detail below.
The load demand of several supermarkets was studied by Ijeoma et al., 2023. They conducted an energy audit for different supermarkets in the city to analyze their power consumption. For this analysis and simulation, the energy audit results were adopted, and the supermarket with the highest energy demand was used as a case study. The load assessment encompassed several vital activities: collecting data through a survey of the electrical appliances utilized within the supermarket, generating a power consumption breakdown for each appliance with details such as function, wattage, and daily operation time, and using statistical analysis to ascertain the aggregate load originating from each supermarket.
The building’s energy consumption was impacted by its operating hours and the installed appliances, with specific consideration given to the building envelopes and HVAC system. According to their study, Market Square (MS) supermarket (located in Woji, New GRA PH city) had the highest power rating in the city. It had a daily peak load of 59.8 kW. For more information on MS power consumption and daily peak load, see Supplementary Material Section S1.
The hourly load distribution in Figure 2 shows the variation of load demand throughout the day. The load demand is low in the morning because the supermarket is just opening, and the load demand increases gradually by the hour and gradually decreases towards the evening.
The solar irradiance and temperature data for PH city were obtained from the database of the National Aeronautics and Space Administration (NASA) (Quansah et al., 2022; NASA, 2023), specifically from the Prediction of Worldwide Energy Resources (POWER) database. This database contains collected meteorological data from July 1983 to June 2005. The average daily horizontal solar irradiation (Hc) on a plane of array (POA) was recorded at 4.21 kWh/m2/day, whereas the average ambient temperature was 25.3°C. For further information, see Supplementary Material Section S2.
A PV module comprises multiple PV cells that are connected in two ways: parallel to increase the electrical current and in series to boost the voltage. When these cells are exposed to sunlight, they generate direct current (DC) electricity (Solar PV Modules, 2024). PV panels are built with one or more PV modules, forming a pre-wired unit ready for installation in the field. The modular design of PV panels allows the systems to be adjusted in size according to changing needs. On the other hand, a PV array comprises several PV modules or panels linked together in series and parallel to provide the necessary voltage and amperage for a specific application. Arrays can vary significantly in size, ranging from just a couple of modules to large expanses covering acres, depending on the energy demands of the system they are powering (Photovoltaic module Enel Green Power, 2024).
The performance of PV modules and arrays is typically assessed based on their maximum DC power output (measured in watts) under Standard Test Conditions (STC). These conditions involve the module operating at 25°C and being exposed to 1000 W per square meter of sunlight with a spectral distribution known as Air Mass 1.5. However, performance usually falls between 85 and 90 percent of the STC ratings in real-world conditions. This variation is due to changes in temperature, shading, and dirt buildup, all of which can affect the performance of PV systems (Solar PV Modules, 2024; Photovoltaic module Enel Green Power, 2024; Solar Photovoltaic Technology Basics | Department of Energy, 2024). The power output of a PV module is usually affected by the operating temperature of the installed location. The temperature of the PV cells is pivotal in ascertaining the module’s efficiency. This is the efficiency at which a PV array converts sunlight into electrical energy at its peak power point, referred to as standard test condition (STC) (Dubey et al., 2013; Understanding PV Module Performance Characteristics - Technical Articles, 2024). HOMER evaluates the temperature of the PV cell. PV nominal operating cell temperature (at STC) is the surface temperature that the PV array reaches if it is exposed to 0.8 kW/m2 of solar radiation, an ambient temperature of 20°C, and a wind speed of 1 m/s.
The capacity of a PV array is determined by analyzing the average solar radiation at the design location, considering daily energy demand and environmental factors. In a battery-based system, the charge controller attached to the PV array must be large enough to manage all the power produced by the array (Harrington and Dunlop, 1992; Ijeoma et al., 2023), which is the maximum power point tracking (MPPT) charge controller capacity.
Solar panels are connected in parallel to increase the number without exceeding the voltage limit of the inverter. Solar panels are connected in series to boost voltage and satisfy the inverter’s minimal operating requirements. Solar systems with shading issues can benefit from the parallel wiring of solar panels, which increases the panels’ current output. Additionally, connecting solar panels in series can enhance energy production in places without enough area for more panels (Series Vs Parallel Solar Panels Connections Explained – Helius Hub, 2024).
A generic flat plate solar module was selected for this study because of its application variety, availability, and affordability in the Nigerian market. The flat plate module had a rated capacity of 380 kW, 25 years lifetime, and an 80% derating factor. The rated capacity will enable HOMER to size the system to meet the supermarket’s load demand and the module’s lifetime will ensure that it lasts throughout the 25-year system lifetime, reducing replacement cost.
For more discussion on how HOMER calculates the power output of a PV module, see Supplementary Material Section S3.
The inverter aids in converting the direct current (DC) from the PV cells to provide the load with alternating current (AC): most electrical devices and appliances function on AC, which necessitates this conversion. Furthermore, to guarantee that the AC generated is safe and effective for the load, the inverter verifies that the current’s voltage and frequency are accurate.
A large generic inverter was used for this analysis. The inverter was selected because it allowed for dynamic sizing of the battery system without having to size the converter. Additionally, it accounted for efficiency losses from DC to AC bus (inverter efficiency). It was intended to be as large as necessary to avoid bottlenecks converting between the AC and DC bus.
A battery is a portable and convenient power source that transforms chemical bond energy into electrical energy through oxidation and reduction reactions, producing power for various devices, including electric cars and small electronics (Storage in PV Systems PVEducation, 2024). Batteries store extra energy from a PV system, which can be used at night or when no other energy input is available, providing a reliable source of electricity even when the sun is not visible. Utilizing batteries in conjunction with a PV system enhances energy efficiency and reduces grid reliance, as batteries can discharge rapidly and generate more current than the charging source (PV System Batteries, 2024).
A Hoppecke 2-V and 3000 Ah, vented, lead-acid deep-cycle battery with a nominal capacity of 7.15 kWh and an 86% roundtrip efficiency was selected for this study. The lead-acid battery is a leading choice of battery for solar systems in Nigeria and sub-Saharan Africa because of its cost compared to lithium-ion batteries (Anuphappharadorn et al., 2014). When the PV system is unavailable, the battery storage is a fallback for meeting electrical demand, allowing the system to continue operating. In photovoltaic (PV) systems, battery storage is a vital component that guarantees a continuous power supply, even during periods of minimal sunlight or at night. The battery can supply load demand by storing extra PV energy even while the PV system is off. This attribute enhances the dependability and adaptability of solar energy applications.
The lifetime of the storage bank in HOMER may be constrained by two distinct factors: the lifetime throughput and the storage float life. Alternatively stated, batteries have the potential to deplete due to age or usage. For more information on how HOMER calculates the battery storage bank, see Supplementary Material Section S3.
A generator is a fuel-consuming apparatus in HOMER that generates electrical energy (and occasionally thermal energy). The capability to dispatch generators enables the system to activate them for electricity production only when required. An auto-size diesel generator system was selected for this study. The auto-size feature allows the generator to automatically size itself to meet the load within the simulation analysis. The generator’s capacity is sized by HOMER to be the smallest rating which will produce no capacity shortage in all sensitivity cases.
The average total efficiency of a generator is calculated by dividing the electrical and thermal energy output by the fuel energy input over 1 year. An autosize diesel generator with a fuel curve intercept of 3.51 L/h, a lower heating value of 43.2 MJ/kg, and density of 820 kg/m3 was used for this analysis. For more information on how the generator efficiency is calculated, and the selected components used in modeling the hybrid PV/battery/generator energy system in HOMER, see Supplementary Material Section S3.
The economic parameters comprise of the net present cost (NPC) and levelized cost of electricity (LCOE). The discount and inflation rate in Nigeria is very irregular, and as such, we assume the discount rate for this analysis was stabilized at 8%. However, the impact of the change in inflation rate was assessed through sensitivity analysis of the economic assessment.
The NPC is determined using the life cycle cost (LCC) approach. LCC involves the overall cost across the project’s life cycle, including startup capital, maintenance and operation costs, replacement costs, diesel costs, emission penalties, and the asset’s resale value at the end of its life (Lee, 2002; Settanni and Tassielli, 2012). By considering these factors, organizations can make informed decisions regarding the financial viability of investing in a particular asset. HOMER determines the NPC by adding all the discounted cash flows for each year during the project’s duration. HOMER ranks all system configurations in the optimization results according to the total NPC, which is also the foundation upon which the total annualized cost and levelized cost of energy are computed (Net Present CostBack ButtonSearch IconFilter Icon, 2023).
The LCOE is a metric used to calculate the total energy cost throughout a project’s lifespan. The function is determined by the LCC of the project and the energy produced over its duration. For more information, see Supplementary Material Section S4 on how LCOE is calculated in HOMER.
Net present value (NPV) is a financial tool used to assess the profitability of an investment or project, considering the time value of money. It helps investors make informed decisions by calculating the expected return on investment and accounting for all costs and benefits. A positive NPV indicates the investment is expected to generate more returns than the initial cost. However, a negative NPV suggests the investment may not be profitable (NPV, 2024). For more information on how HOMER calculates the NPV, see Supplementary Material Section S5.
It should be noted that HOMER assumes linear depreciation of components, meaning that the salvage value of a component is directly proportional to its remaining life. It also assumes that the salvage value depends on the replacement cost rather than the initial capital cost.
The payback period is the time it takes to repay an investment’s cost, with shorter periods indicating more attractive investments and more extended periods indicating less desirable, typically expressed in years (Ijeoma et al., 2023).
Pollution to the environment occurs due to the combustion of fuel within the diesel generator. HOMER reports the emissions for the following pollutants: carbon dioxide (CO2), carbon monoxide (CO), unburned hydrocarbon (UHC), particulate matter (PM), sulfur dioxide (SO2), and nitrogen oxides (NO2). The broader implications of these chemicals to the environment (Author Anonymous, 2024) are explained in Supplementary Material Section S5.
The potential environmental impacts of these compounds in all the systems were quantified using the U.S. Environmental Protection Agency (EPA) life cycle impact assessment (LCIA) model TRACI 2.1 (the Tool for Reduction and Assessment of Chemicals and Other Environmental Impacts) (Bare, 2011). TRACI 2.1 has been developed for sustainability metrics, life cycle impact assessment, industrial ecology, and process design impact assessment for developing increasingly sustainable products, processes, facilities, companies, and communities. The TRACI methodology employs the amount of chemical emission or resource used, along with the estimated potency of the stressor, in all impact categories, regardless of whether the analysis is being conducted within an LCA, process design, or sustainability metrics basis.
The impact assessment categories from TRACI considered in the analysis were global warming potential, acidification potential, eutrophication potential (air and water), photochemical smog potential, and human health (HH) particulate potential (United states environmental protection agency, 2012). TRACI 2.1 characterization factors were applied to the direct emission of different pollutants from the diesel generator used to determine their potential midpoint impacts on the environment. For a detailed description of these impact categories and the TRACI 2.1 characterization factors of each of the pollutants applied to the impact categories, see Supplementary Material Section S5.
HOMER Pro software was utilized to execute the simulation. The software was provided with the following input parameters: solar irradiance and temperature (which were obtained from a NASA database and are incorporated into the software), load demand (power), selection of solar modules, battery storage unit, generator unit, and cost of system components. Various sensitivity scenarios, including changes in generator diesel costs and the derating factor of the solar module, were incorporated into the simulation. The simulation’s output optimizes the most effective system to satisfy the load requirement.
The best system was chosen based on the size of the system component, NPC, initial capital cost, and LCOE. The size of the system component pertains to the overall capacity of the solar modules, battery storage unit, and generator unit. The NPC considers the original capital and operational expenses over the system’s lifespan. The LCOE is a metric that quantifies the average cost of generating each unit of electricity during the system’s whole lifespan. Through careful evaluation of these variables, the simulation can determine the optimal and most economical system configuration to satisfy the load requirement.
This study schematic diagram designed in HOMER Pro for simulation is shown in Figure 3. The HOMER system comprises solar PV, a storage battery, an inverter, and a diesel generator. The system is optimized to meet load demand and has the lowest NPC and LCOE. Factors like solar radiation, battery efficiency, and system reliability are considered. The optimal system is chosen based on the current diesel price in Nigeria, which may fluctuate over time. Regular performance reassessments and adjustments are crucial for continued cost-effectiveness and efficiency.
Figure 3. HOMER general system schematic for the microgrid architectures investigated in this study.
This section covers the technical, economic, and environmental system simulation results and the sensitivity analysis.
Table 1 presents the HOMER simulation results for a hybrid energy system, with diesel prices as a determining factor. These results indicate that the optimal system (with the lowest LCOE) configuration varies depending on diesel prices. This flexibility underscores the system’s ability to adjust to changing economic conditions, highlighting the resilience of hybrid energy systems. The adaptability of these systems is crucial for maintaining sustainability and efficiency in supermarket operations. Being able to modify the system in response to fluctuating diesel prices contributes to overall sustainability and efficiency.
After simulation, the optimal system was selected based on the current diesel price of $0.673 USD/L. The optimal system architecture was a PV/generator/battery system with an inverter. The optimal architecture will be used as our baseline (BL) for sensitivity analysis. For detailed information on the components design characteristics of the BL system see Supplementary Material Section S6.
The BL system has a PV capacity of 313 kW, generating an average of 1,059 kWh/day and 386,574 kWh/year, a capacity factor of 14.1%, with a 117-kW inverter. The generator set has a power capacity of 91 kW and operates at a capacity factor of 1.9%. It has a lifespan of 83.3 years. The generator’s lifespan is prolonged within this architecture as it will only operate for a total of 180 h each year. A battery system of 120 units, each having a voltage of 2V and a capacity of 3000Ah, is connected in a configuration of 4 parallel banks, each with 30 batteries in series, resulting in a 60 V system.
The battery storage system has an anticipated lifespan of 14.5 years and a total energy throughput (the amount of electricity delivered from the battery storage) of 1,214,196 kWh. The results showed that the BL system yielded a total electric energy output of 401,599 kWh per year, with solar PV contributing 386,574 kWh per year (of which 83,894 kWh per year are delivered from battery storage), representing 96.3% (21.7% from the battery) of the whole energy production. The generator’s annual output was 15,025 kWh, accounting for 3.74% of the total energy production. The average monthly energy output of the BL system is depicted in Figure 4; it presents the proportion of the load that is being met using solar PV and the generator set.
This study is an improvement in the analysis conducted by Ijeoma et al., 2023 at the exact location. Their study used a 232 kW PV and a 34,021 Ah battery capacity system to meet the load of the supermarket. However, their system had a 2,299 kWh/year unmet load, requiring an additional energy system to meet the unmet energy, unlike this system, which had zero.
Figure 5 illustrates the annual output of the PV and generator system over the year (a time series based on when they occurred). The time series of PV output (upper graph) reveals the system is highest during daylight hours (between 9 a.m. and 5 p.m.), with an average range of 140 kW–250 kW. On average, it produces between 140 and 250 kW, with variations depending on the specific day of the year. The PV system operates for approximately 4,447 h (about 6 months) per year. The PV system produced a low output around day 85; this can be attributed to the beginning of the rainy season in the region. However, between the period of the PV low generation, the generator kicks in to cover the difference as shown in Figure 6. A great indication of the reliability of a hybrid system.
Figure 5. Time Series Annual Output of PV and generator systems. Upper: PV output–Time Series over the year. Lower: Generator Output- Time Series over the year.
Figure 6. A breakdown of the monthly average excess electrical energy production for the Baseline System (BL) architecture.
The diesel generator supplies a small fraction of the required electricity as shown in Figure 5 (lower graph). The generator exhibited an average power output of 83.5 kW. It was in operation for only 180 h per year from 28 starts/year. Furthermore, it consumed only 4,177 L (equivalent to approximately 1,103 gallons) of diesel fuel annually. The generator is utilized efficiently and effectively, considering its restricted operating hours, starts, and fuel usage. The findings indicate that the generator was used throughout September, corresponding to the rainy season’s zenith when solar PV systems generate less electricity. The generator’s operating life was prolonged to 83.3 years due to minimal operation. Nevertheless, the generator operated at just 1.88% capacity factor. This low-capacity factor could suggest that the generator was not fully utilized, however, this meets the goal of reducing the use time of the diesel generator. (reference a paper on generator fraction of their study).
Our study is an improvement from a similar study conducted by Modu et al., 2018, where the generator system in their PV-diesel-battery system was operated for 4,299 h (about 6 months) per year and consumed 26,662 L/year of diesel with 1,142 starts. The difference in the generator operation behavior is because our system was designed to be more environmentally friendly.
These findings indicate that solar PV systems could satisfy most of the energy requirements, serving as viable alternatives to traditional energy sources. Additionally, further analysis should be conducted to determine if alternative energy sources could supplement or replace the generator during low solar PV generation periods. For a discussion of the overall electrical load and the proportion of the load satisfied by various sources, see Supplementary Material Section S6.
A breakdown of the monthly average excess electrical energy production for the BL system architecture is presented in Figure 6. The BL system generated an excess of 173,195 kWh/year of electric energy, which accounted for 43.1% of the overall energy production. The graph illustrates that the highest excess energy was generated from January to February, coinciding with the months exhibiting the most substantial number of sunlight hours in PH City. Similarly, June, July, August, and September yielded the lowest excess energy. These are the year’s wettest months and are called the “rainy season.” The solar radiation evaluation results offer valuable information about the system’s excess energy production. The data indicates that PH City experienced the greatest level of solar radiation in January and February, corresponding to the graph illustrating the most significant excess energy generation during these months. In contrast, June, July, August, and September see reduced solar radiation due to higher precipitation, leading to decreased excess energy generation during this time.
The functional behavior of our system is identical to the study conducted by Odou et al., 2020 in the Benin Republic for rural electrification; the diesel generator in their hybrid system was operated during July, August, and September. However, their system yielded only 7.7% excess energy. The difference in excess energy production was because our system’s capacity was significantly larger. The larger capacity enabled more efficient peak energy production, resulting in a higher excess energy percentage, emphasizing the significance of system capacity in optimizing energy production.
Notably, the BL system can sell any excess energy it generates to the power grid in exchange for additional income. The revenue generated from selling the excess energy can mitigate the expenses associated with the implementation and upkeep of the system, rendering it a financially feasible choice for energy generation. The significant renewable energy share of 92.7% demonstrates that the BL system effectively diminishes dependence on non-renewable energy sources, reducing carbon emissions and environmental footprint.
Figure 7 visually displays the status of charge in the battery system, showing the amount of energy stored in the batteries. In most cases, the batteries are almost fully charged by midday and are then used in the evening from around 7 p.m. onward. Just like in Figure 6, you can see that the battery has an extremely low charge before day 90 because of the low PV power output during that period. Furthermore, it demonstrates that the battery never reached a full charge of 100% at any point. The charge level suggests that the battery system efficiently harnesses the solar energy produced during the most productive hours. However, enhancing the charging procedure to get a complete charge might be required. To ensure that the battery system operates as efficiently and effectively as possible in supplying a continuous power source, operators must keep an eye on any issues that might be preventing the system from charging to its full potential. By examining the level of charge, operators can ascertain the appropriate timing for recharging or replacing the batteries to maintain a continuous power supply.
Figure 7. A breakdown of the state of charge profile over a model year of the BL system architecture.
Also, this visual representation of the result in Figure 7 makes spotting anomalies or variations in the battery system’s operation simple, facilitating prompt maintenance and troubleshooting. This information is vital for monitoring and managing the overall performance and effectiveness of the battery system. For more discussion on the daily discharge profile of the battery and comprehensive functioning of the entire system is shown in Supplementary Material Section S7.
The economic results of the optimal baseline (BL) system are displayed in Table 2. The PV/Battery/Generator system had an initial cost of $147,904. It possessed an NPC of $232,533, an Operations and Maintenance (O&M) cost of $7,928, and an LCOE of $0.106 USD/kWh. The result shown in Figure 9 indicates that the generator system has no replacement value, and this is because the generator was only used for 180 h (below the 15,000 h of expected life) throughout the system’s lifespan.
The LCOE value of this study is similar to the one reported by Ijeoma et al., 2023, where they achieved an LCOE of $0.14 USD/kWh for the same supermarket using only a standalone solar PV system. The price difference comes from using a diesel generator and needing fewer storage batteries. By running the generator for 18 h, we could save around 630,000 Ah of battery capacity. However, our system needs a 313-kW solar power capacity, whereas theirs only requires 232 kW. The solar panel’s value loss was considered in the economic study. For more information on the economic analysis results of the BL system, see Supplementary Material Section S6.
The outcome of this study is also comparable to other hybrid-energy systems studies in the region, as shown in Table 3. The findings of Ukoima et al., 2023, where they examined a solar PV-wind hybrid energy generation system for a rural community in the same State as PH city. Their analysis yielded an LCOE of $0.2 USD/kWh. The difference could be attributed to the high upfront cost of a wind power plant. The findings of this study indicate that the PV/battery/generator combination is economically efficient and on par with other comparable systems examined in prior research. Table 3 shows that our study yielded a reduction in LCOE, which could be attributed to a decrease in the cost of solar panels and the design of our system to minimize the use of diesel generators.
The BL system produced an extra 173,195 kWh of energy yearly, making up 43.1% of the total production. We could sell this excess energy to the power grid to make more money. The Nigerian Bulk Electricity Trading (NBET) manages transactions from generators to distributors (Ijeoma et al., 2023) excess electricity through negotiated power purchase agreements (PPAs). These agreements ensure we get fair payment for the extra energy we provide to the grid. By participating in these PPAs, our BL system can increase its profits by selling extra energy at an agreed-upon price. A study by BlombergNEF in 2018 found that using diesel generators in Nigeria costs households around $0.23 USD/kWh (BlombergNEF, 2022). This research suggests that on-site solar energy can be more economically beneficial than diesel generators.
BlombergNEF and Sustainable Energy for All 2020 market study on global mini-grids shows that solar hybrid mini-grids in remote places catering to commercial and industrial customers have a lower LCOE compared to residential hybrid mini-grids. In Nigeria, the LCOE for diesel/PV/storage systems in residential locations varied from $0.51 to $1.46 USD/kWh due to the underdeveloped state of the green energy sector (BlombergNEF, 2022), this is because the green energy market is not matured in the region. Based on the BlombergNEF report, if the excess energy produced by the BL system were sold to the grid at the proposed minimum rate of $0.51 USD/kWh, the supermarket could achieve an NPV of $710,364, an SPBP of 2 years, and a 38% IRR. In addition, it decreases the levelized cost of electricity (LCOE) of the BL system by $0.0342, resulting in a new LCOE of $0.0718 USD/kWh. This cost is lower than the current electricity price from the national grid, sold at 120 naira/kWh (NERC approves tariff hike and affirms N1.6trn power subsidy for 2024, 2024) (equal to $0.092 USD/kWh). The IRR of 38% demonstrates the attractiveness of the proposed project to potential investors, as it indicates a profitable return on their investment. The LCOE cost reduction of the BL system enhances its appeal and affordability for users transitioning to renewable energy sources. Additionally, the SPBP of 2 years highlights the efficiency of the optimal BL system in recovering its initial costs, contributing to a potentially lower overall LCOE, and offering a more reliable power supply than the national grid.
Based on historical data from the Central Bank of Nigeria (CBN) on monetary rates (Central Bank of Nigeria Money and Credit Statistics, 2024), the median rate for the monetary policy rate (MPR) for the past 18 years has been 12%. The MPR represents the government’s lending rate to its trusted customers. Simultaneously, the MPR has fallen to 6% and increased to 22.8%. If the current rate persists, the BL system’s net present value (NPV) can reach a maximum of $1,129,147 and a minimum of $166,180. The stability of Nigeria’s monetary rates significantly impacts its economy, making it crucial for investors and policymakers to monitor these fluctuations closely for informed investment decisions.
The result of the economic assessment is crucial for decision-making as it provides a clear indication of the project’s financial viability. Moreover, a shorter payback period suggests investors may recoup their initial investment quickly, making the project even more appealing. The analysis of the potential benefit of selling the excess energy to the grid is shown in Supplementary Material Section S8. Note that at the time of this analysis, 1 USD was equivalent to 1300 naira (1 USD to NGN - US Dollars to Nigerian Nairas Exchange Rate, 2024).
The environmental pollutant emissions from the optimal BL system are detailed in Table 4. This shows the direct emissions resulting from the use of the diesel generator.
The result indicated that the hybrid energy system emitted elevated levels of carbon dioxide annually compared to other pollutants, contributing to climate change. Utilizing the TRACI 2.1 impact characterization factor, we measured the environmental impact of these pollutants on the environment across various impact categories. The potential implications of the environmental impacts of these contaminants are described in Supplementary Material Section S5.
Table 5 provides a comprehensive overview of the environmental consequences caused by these pollutants, allowing us to assess their impact on various aspects such as human health, ecosystem quality, and resource depletion.
Table 5. Potential environmental impacts based on different impact categories: unnormalized results.
The results indicate that the BL system dominantly contributes to global warming potential (GWP), followed by smog potential (SF), acidification potential (AP), eutrophication potential (EP) to water and air and particulate matter (PM2.5) emissions to the environment annually. These factors can potentially cause harmful consequences for both human and aquatic life. By using the TRACI 2.1 characterization factors, we can more clearly understand the extent of harm these contaminants are causing, allowing us to make informed decisions to lessen their effects. These emissions to the environment are a clear indication of the negative impact of using diesel generators for electricity generation.
The result of our analysis was comparable to a similar study conducted by Tijani et al. (Harrington and Dunlop, 1992), where a diesel generator in their hybrid energy system emitted 14,410 kg/yr. Similarly, using a generator in a hybrid energy system analysis examined by Modu et al. (Dubey et al., 2013) produced 70,211 kg/yr. Our system was designed to minimize the release of these harmful substances, which resulted in the emission of 10,935 kg/yr. Lowering emissions from these pollutants contributes to mitigating climate change, improving air quality, helping preserve biodiversity and ecosystems, and improving human health. Replacing the diesel generator with a cleaner energy source like wind power can further reduce these emissions and improve air quality.
To assess the impact of diesel price on the overall system, a sensitivity analysis was conducted to determine the most suitable architectural system if the diesel price increases or decreases by 10%. The baseline system price of diesel ($0.673 USD/L) was used as a reference. Comparative analysis of the performance and costs of different systems under different diesel price scenarios can provide valuable insights into their long-term economic viability.
The sensitivity analysis indicates that as the diesel price decreases relative to the BL system diesel price, the PV/battery/generator system remains the optimal configuration. However, as the BL system price of diesel rises by 70% to $1.14 USD/L, the optimal system changes into a solar PV/battery system with an LCOE of $0.112 USD/kWh.
The sensitivity analysis shown in Figure 8 suggests that when the fuel cost rises, the solar PV/battery system becomes increasingly financially feasible in the long term compared to the PV/battery/generator system. The research findings suggest that the most suitable system choice is contingent upon diesel prices, and there is a potential for additional revenue through excess energy generation. This result aligns with the findings of a hybrid techno-economic evaluation carried out in southwest Nigeria by Adaramola et al., 2012b. They stated four distinct hybrid configurations are feasible at $0.6 USD/L of diesel. These systems are wind/generator/battery, wind/PV/generator/battery, generator/battery, and PV/generator/battery. Nevertheless, as diesel price rises, the viability of the systems without solar PV diminishes. This outcome suggests that integrating solar PV technology into hybrid systems may enhance their financial feasibility, particularly considering the escalating diesel prices.
Figure 8. The agglomerated HOMER simulation sensitivity analysis results for electrical energy production.
The sensitivity analysis underscores the significance of factoring in long-term economic fluctuations in diesel prices. These findings imply that solar PV/battery systems contribute to reduced environmental impact and offer economic advantages. The study aids stakeholders in making well-informed decisions regarding architectural systems, considering financial considerations and sustainability while also evaluating resilience against the variability of diesel prices.
The impact of fuel prices on the LCOE and CO2 is illustrated in Figure 9. The graph below demonstrates a positive correlation between the diesel price increase and the LCOE increase. This correlation is anticipated, as diesel prices significantly impact operational costs in power generation, leading to a rise in the LCOE as they serve as a significant expense factor. The graph above shows an inverse relationship between changes in optimal winning system architecture and carbon dioxide emissions. It implies that higher diesel prices lead to a decrease in CO2 emissions, suggesting that the relationship is not linear. The result indicates that the optimal system architecture, in these cases, opts for a system that consumes a smaller amount of diesel and a system that eliminates the need for a diesel generator. The link is vital for identifying system configurations that reduce CO2 emissions and promote sustainability in power generation, enabling informed decisions for environmental impact reduction.
To evaluate the effects of Nigeria’s inconsistent discount rate, a sensitivity analysis was performed on the economic analysis of selling the excess energy to the grid. The analysis’s goal was to ascertain how changes to the discount rate would impact the system’s overall economic viability. By varying the discount rate within a reasonable range, the study could provide insights into the potential risks and uncertainties associated with this decision to invest in energy projects in Nigeria’s unique economic context.
The impact of the discount rate on the NPV of the BL system is illustrated in Figure 10, focusing just on the surplus energy sold to the grid. The sensitivity analysis demonstrated that variations in the discount rate have a significant impact on the net present value of the BL system. As the discount rate rises, the NPV declines, indicating reduced profitability in selling excess electricity to the grid. These findings emphasize the significance of precisely measuring and considering the discount rate in economic evaluations for energy projects in Nigeria. The detailed sensitivity analysis of the BL system is shown in Supplementary Material Section S8.
In this study, a hybrid energy generation system tailored to the specific demands of Port Harcourt, Nigeria, was simulated using HOMER software based on current diesel fuel costs in Nigeria ($0.673 USD/L). The analysis evaluated the technical, economic, and environmental feasibility of the system for supplying electricity to a commercial center. The finding revealed that the solar PV/Battery/Generator is the optimal system to meet the load demand at a diesel price of $0.673 USD/L. This optimal system generated a total annual electricity output of 401,599 kWh/year, significantly surpassing the required load demand of 204,765 kWh/year. Notably, the LCOE was $0.106 USD/kWh, consistent with other studies in this region. Additionally, the system’s excess energy production (173,195 kWh/year) could yield $710,364 USD/year if sold to the grid, suggesting a 38% IRR, a 2-year SPBP, and an NPV of $942,879. Moreover, environmental analysis of the BL system identified that GWP had the most pronounced environmental impact, followed by SF and AP.
A sensitivity analysis on diesel price fluctuations highlighted the robustness of the solar PV/battery/generator system, maintaining its feasibility with diesel price variations up to 60%. Beyond a 70% increase in diesel prices, a transition to a solar PV/battery configuration becomes optimal. Moreover, diesel consumption significantly influences the LCOE and environmental emissions. Similarly, the discount rate affects the LCOE. All results underscore the potential for energy cost reduction and environmental benefits in Nigeria’s commercial sector, highlighting the importance of implementing sustainable practices and technologies in businesses nationwide. Integrating a hybrid energy system could lead to significant long-term cost savings and a positive environmental impact. Future studies should explore an all-renewable hybrid energy system, integrating solar, wind, and hydroelectric power sources for efficiency and sustainability, and investigate energy storage solutions like pumped hydro for reliable supply.
The original contributions presented in the study are included in the article/Supplementary Material, further inquiries can be directed to the corresponding author.
MI: Conceptualization, Data curation, Formal Analysis, Investigation, Methodology, Project administration, Software, Visualization, Writing–original draft, Writing–review and editing. CL: Visualization, Writing–review and editing. HC: Visualization, Writing–review and editing. BC: Writing–review and editing. MC-D: Conceptualization, Resources, Software, Supervision, Writing–review and editing, Methodology.
The author(s) declare that no financial support was received for the research, authorship, and/or publication of this article.
I would like to acknowledge Patcares Global Services and KEEP project for their support in acquiring research data and its verification.
The authors declare that the research was conducted in the absence of any commercial or financial relationships that could be construed as a potential conflict of interest.
The author(s) declared that they were an editorial board member of Frontiers, at the time of submission. This had no impact on the peer review process and the final decision.
All claims expressed in this article are solely those of the authors and do not necessarily represent those of their affiliated organizations, or those of the publisher, the editors and the reviewers. Any product that may be evaluated in this article, or claim that may be made by its manufacturer, is not guaranteed or endorsed by the publisher.
The Supplementary Material for this article can be found online at: https://www.frontiersin.org/articles/10.3389/fenrg.2024.1397037/full#supplementary-material
1 USD to NGN - US Dollars to Nigerian Nairas Exchange Rate, 2024 1 USD to NGN - US Dollars to Nigerian Nairas Exchange Rate (2024). Xe. Available at: https://www.xe.com/currencyconverter/convert/?Amount=1&From=USD&To=NGN (Accessed March 31, 2024).
Abdelhady, S. (2023). Techno-economic study and the optimal hybrid renewable energy system design for a hotel building with net zero energy and net zero carbon emissions. Energy Convers. Manag. 289, 117195. doi:10.1016/j.enconman.2023.117195
Accelerating access to energy in Africa with solar microgrids: A study - Schneider Electric Blog (2024). Blogs. Available at: https://blog.se.com/sustainability/2023/12/05/accelerating-access-to-energy-in-africa-with-solar-microgrids-a-study/(Accessed April 3, 2024).
Access to electricity SDG7 Data and Projections Analysis (2024). IEA. Available at: https://www.iea.org/reports/sdg7-data-and-projections/access-to-electricity (Accessed January 30, 2024).
Adaramola, M. S. (2014). Viability of grid-connected solar PV energy system in Jos, Nigeria. Int. J. Electr. Power Energy Syst. 61, 64–69. doi:10.1016/j.ijepes.2014.03.015
Adaramola, M. S., Agelin-Chaab, M., and Paul, S. S. (2014). Analysis of hybrid energy systems for application in southern Ghana. Energy Convers. Manag. 88, 284–295. doi:10.1016/j.enconman.2014.08.029
Adaramola, M. S., Oyewola, O. M., and Paul, S. S. (2012a). Technical and economic assessment of hybrid energy systems in South-West Nigeria. ENERGY Explor. EXPLOITATION 30, 533–551. doi:10.1260/0144-5987.30.4.533
Adaramola, M. S., Oyewola, O. M., and Paul, S. S. (2012b). Technical and economic assessment of hybrid energy systems in South-West Nigeria. ENERGY Explor. EXPLOITATION 30, 533–551. doi:10.1260/0144-5987.30.4.533
Adaramola, M. S., Quansah, D. A., Agelin-Chaab, M., and Paul, S. S. (2017). Multipurpose renewable energy resources based hybrid energy system for remote community in northern Ghana. Sustain. Energy Technol. Assessments 22, 161–170. doi:10.1016/j.seta.2017.02.011
Ajao, K. R., Oladosu, O. A., and Popoola, O. T. (2011). USING HOMER POWER OPTIMIZATION SOFTWARE FOR COST BENEFIT ANALYSIS OF HYBRID-SOLAR POWER GENERATION RELATIVE TO UTILITY COST IN NIGERIA. IJRRAS 7. doi:10.1017/CBO9781107415324.004
Ajlan, A., Tan, C. W., and Abdilahi, A. M. (2017). Assessment of environmental and economic perspectives for renewable-based hybrid power system in Yemen. Renew. Sustain. Energy Rev. 75, 559–570. Elsevier Ltd. doi:10.1016/j.rser.2016.11.024
Al-Rawashdeh, H., Al-Khashman, O. A., Al Bdour, J. T., Gomaa, M. R., Rezk, H., Marashli, A., et al. (2023a). Performance analysis of a hybrid renewable-energy system for green buildings to improve efficiency and reduce GHG emissions with multiple scenarios. Sustain. Switz. 15 (9), 7529. doi:10.3390/su15097529
Al-Rawashdeh, H., Al-Khashman, O. A., Al Bdour, J. T., Gomaa, M. R., Rezk, H., Marashli, A., et al. (2023b). Performance analysis of a hybrid renewable-energy system for green buildings to improve efficiency and reduce GHG emissions with multiple scenarios. Sustain. Switz. 15 (9), 7529. doi:10.3390/su15097529
Amnesty International (2023). Nigeria: removal of fuel subsidy must not exacerbate poverty - amnesty International. Available at: https://www.amnesty.org/en/latest/news/2023/06/nigeria-remove-fuel-subsidy-exacerbate-po/(Accessed July 18, 2023).
Anuphappharadorn, S., Sukchai, S., Sirisamphanwong, C., and Ketjoy, N. (2014). “Comparison the economic analysis of the battery between lithium-ion and lead-acid in PV stand-alone application,” in Energy procedia (Elsevier Ltd), 352–358.
Bare, J. (2011). TRACI 2.0: the tool for the reduction and assessment of chemical and other environmental impacts 2.0. Clean. Technol. Environ. Policy 13 (5), 687–696. doi:10.1007/s10098-010-0338-9
Basic energy access lags amid renewable opportunities (2024). New report shows - news - IEA. Available at: https://www.iea.org/news/basic-energy-access-lags-amid-renewable-opportunities-new-report-shows (Accessed January 30, 2024).
BlombergNEF (2022). Climatescope 2022 | Nigeria. Available at: https://www.global-climatescope.org/markets/ng/(Accessed July 16, 2023).
Bukari, D., Kemausuor, F., Quansah, D. A., and Adaramola, M. S. (2021). Towards accelerating the deployment of decentralised renewable energy mini-grids in Ghana: review and analysis of barriers. Renew. Sustain. Energy Rev., 135. doi:10.1016/j.rser.2020.110408
Can diesel power plants be integrated with renewable energy sources? by Brenda Rose Medium (2024). Medium. Available at: https://medium.com/@Breadarose/can-diesel-power-plants-be-integrated-with-renewable-energy-sources-03d23b5d2c6d (Accessed March 25, 2024).
Central Bank of Nigeria Money and Credit Statistics (2024). Cbn. Available at: https://www.cbn.gov.ng/rates/mnymktind.asp?year=2024&month=4 (Accessed March 31, 2024).
Come, Z. E., van der Windt, H. J., Nhumaio, G., and Faaij, A. P. C. (2021). A review of hybrid renewable energy systems in mini-grids for off-grid electrification in developing countries. Renew. Sustain. Energy Rev. 144, 111036. Elsevier Ltd. doi:10.1016/j.rser.2021.111036
Doris Dokua Sasu (2024). Nigeria: share of access to electricity | Statista. Available at: https://www.statista.com/statistics/1307416/total-population-access-to-electricity-in-nigeria/(Accessed March 24, 2024).
Dubey, S., Sarvaiya, J. N., and Seshadri, B. (2013). “Temperature dependent photovoltaic (PV) efficiency and its effect on PV production in the world - a review,” in Energy procedia (Elsevier Ltd), 311–321.
Energy and Environment Partnership (EEP) NDF (2018). OPPORTUNITIES AND CHALLENGES IN THE MINI-GRID SECTOR IN AFRICA. Available at: https://eepafrica.org/publications/#studies (Accessed April 3, 2024).
Energy consumption in Nigeria (2023). Worlddata. Available at: https://www.worlddata.info/africa/nigeria/energy-consumption.php (Accessed October 1, 2023).
Eze, F., Ogola, J., Kivindu, R., Egbo, M., and Obi, C. (2022). Technical and economic feasibility assessment of hybrid renewable energy system at Kenyan institutional building: a case study. Sustain. Energy Technol. Assessments 51, 101939. doi:10.1016/j.seta.2021.101939
For the first time in decades, the number of people without access to electricity is set to increase in 2022 – Analysis (2023). IEA. Available at: https://www.iea.org/commentaries/for-the-first-time-in-decades-the-number-of-people-without-access-to-electricity-is-set-to-increase-in-2022 (Accessed August 28, 2023).
Goel, S., and Sharma, R. (2017). Performance evaluation of stand alone, grid connected and hybrid renewable energy systems for rural application: a comparative review. Renew. Sustain. Energy Rev. 78, 1378–1389. Elsevier Ltd. doi:10.1016/j.rser.2017.05.200
Harrington, S., and Dunlop, J. (1992). Battery charge controller characteristics in photovoltaic systems. IEEE Aerosp. Electron. Syst. Mag. 7 (8), 15–21. doi:10.1109/62.151141
Heavy use of generators puts Nigeria’s climate plans in jeopardy - Punch Newspapers (2023). Punchng. Available at: https://punchng.com/heavy-use-of-generators-puts-nigerias-climate-plans-in-jeopardy/(Accessed September 18, 2023).
HIES Renewable Energy (2023). Solar Inverter - what it is and how to choose the right one. Available at: https://www.hiesscheme.org.uk/renewable-energy/solar-inverters/(Accessed May 7, 2023).
How microgrids can electrify rural Africa | Africa at LSE (2024). Blogs. Available at: https://blogs.lse.ac.uk/africaatlse/2023/12/14/how-microgrids-can-electrify-rural-africa/(Accessed April 1, 2024).
Ijeoma, M. W., Chen, H., Carbajales-Dale, M., and Yakubu, R. O. (2023). Techno-economic assessment of the viability of commercial solar PV system in Port Harcourt, Rivers state, Nigeria. Energies (Basel) 16 (19), 6803. doi:10.3390/en16196803
IRENA (2023). Battery storage paves way for a renewable-powered future. Available at: https://www.irena.org/news/articles/2020/Mar/Battery-storage-paves-way-for-a-renewable-powered-future (Accessed May 7, 2023).
Kumar, B. A., Kumar, A., Kumar Rajak, D., Pruncu, C. I., and Lamberti, L. (2021). Towards better performances for a novel rooftop solar PV system. Sol. Energy 216, 518–529. doi:10.1016/j.solener.2021.01.045
Lee, D. B. (2002). Fundamentals of life-cycle cost analysis. Transp. Res. Rec. 1812, 203–210. doi:10.3141/1812-25
Llera Sastresa, E., Usón, A. A., Bribián, I. Z., and Scarpellini, S. (2010). Local impact of renewables on employment: assessment methodology and case study. Renew. Sustain. Energy Rev. 14, 679–690. doi:10.1016/j.rser.2009.10.017
Mobilizing Clean Energy to Address Energy Poverty in West Africa | The Climate Reality Project (2023). Climaterealityproject. Available at: https://www.climaterealityproject.org/blog/mobilizing-clean-energy-address-energy-poverty-west-africa (Accessed August 28, 2023).
Modu, B., Aliyu, A. K., Bukar, A. L., Abdulkadir, M., Gwoma, Z. M., and Mustapha, M. (2018). Techno-economic analysis of off-grid hybrid PV-diesel-battery system in Katsina State, Nigeria. AZOJETE 14 (2), 317–324. Available at: www.azojete.com.ng.
Muzan, I. W., Faisal, T., Al-Assadi, HMAA, and Iwan, M. (2012). Implementation of industrial robot for painting applications. Procedia Eng. 41, 1329–1335. doi:10.1016/j.proeng.2012.07.318
NASA (2023). NASA POWER | prediction of worldwide energy resources. Available at: https://power.larc.nasa.gov/(Accessed May 7, 2023).
Ndukwe, C., and Iqbal, T. (2019). Sizing and dynamic modelling and simulation of a standalone PV based DC microgrid with battery storage system for a remote community in Nigeria. J. Energy Syst. 3 (2), 67–85. doi:10.30521/jes.544710
Nema, P., Nema, R. K., and Rangnekar, S. (2010). Minimization of green house gases emission by using hybrid energy system for telephony base station site application. Renew. Sustain. Energy Rev. 14, 1635–1639. doi:10.1016/j.rser.2010.02.012
NERC approves tariff hike, affirms N1.6trn power subsidy for 2024 (2024). Icirnigeria. Available at: https://www.icirnigeria.org/nerc-approves-electricity-tariff-hike-affirms-n1-6trn-power-subsidy-for-2024/(Accessed April 1, 2024).
Net Present CostBack ButtonSearch IconFilter Icon (2023). Homerenergy. Available at: https://www.homerenergy.com/products/pro/docs/3.11/net_present_cost.html (Accessed December 19, 2023).
NPV (2024). Net Present Value NPV: What It Means and Steps to Calculate It. Available at: https://www.investopedia.com/terms/n/npv.asp (Accessed March 26, 2024).
Nfah, E. M., and Ngundam, J. M. (2008). Modelling of wind/Diesel/battery hybrid power systems for far North Cameroon. Energy Convers. Manag. 49 (6), 1295–1301. doi:10.1016/j.enconman.2008.01.007
Nigeria Demographics (2023). (Population, age, sex, trends) - worldometer. Available at: https://www.worldometers.info/demographics/nigeria-demographics/(Accessed February 21, 2024).
Nigeria leads Africa as the highest generator importer - Nairametrics (2023). Nairametrics. (Accessed 2023 September 18). Available at: https://nairametrics.com/2023/01/18/nigeria-leads-africa-as-the-highest-generator-importer/
Nigeria Population (2023). Worldometer. Available at: https://www.worldometers.info/world-population/nigeria-population/(Accessed August 28, 2023).
Nigeria relies on generators for 75% electricity – Report (2023). Punchng. Available at: https://punchng.com/nigeria-relies-on-generators-for-75-electricity-report/?utm_source=auto-read-also&utm_medium=web (Accessed 2023 September 18).
Odou, O. D. T., Bhandari, R., and Adamou, R. (2020). Hybrid off-grid renewable power system for sustainable rural electrification in Benin. Renew. Energy 145, 1266–1279. doi:10.1016/j.renene.2019.06.032
Okoro, U. K., and Chineke, T. C. (2021). Whistleblowing on photovoltaic operations in Nigeria: panacea for sustainable development. Bull. Natl. Res. Cent. 45 (1), 140. doi:10.1186/s42269-021-00598-8
Oladigbolu, J. O., Ramli, M. A. M., and Al-Turki, Y. A. (2019). Techno-economic and sensitivity analyses for an optimal hybrid power system which is adaptable and effective for rural electrification: a case study of Nigeria. Sustain. Switz. 11 (18), 4959. doi:10.3390/su11184959
Olatomiwa, L., Blanchard, R., Mekhilef, S., and Akinyele, D. (2018). Hybrid renewable energy supply for rural healthcare facilities: an approach to quality healthcare delivery. Sustain. Energy Technol. Assessments 30, 121–138. doi:10.1016/j.seta.2018.09.007
Ozoegwu, C. G., and Akpan, P. U. (2021). A review and appraisal of Nigeria’s solar energy policy objectives and strategies against the backdrop of the renewable energy policy of the Economic Community of West African States. Renew. Sustain. Energy Rev. 143, 110887. Elsevier Ltd. doi:10.1016/j.rser.2021.110887
Page, J. (2012). The role of solar-radiation climatology in the design of photovoltaic systems. Pract. Handb. Photovoltaics, 573–643. doi:10.1016/b978-0-12-385934-1.00017-9
Photovoltaic module Enel Green Power (2024). Enelgreenpower. Available at: https://www.enelgreenpower.com/learning-hub/renewable-energies/solar-energy/photovoltaic-module (Accessed March 25, 2024).
Port Harcourt Population (2024). Worldpopulationreview. Available at: https://worldpopulationreview.com/world-cities/port-harcourt-population (Accessed January 18, 2024).
Power Plant Generator Woodstock Power Company (2024). Woodstockpower. Available at: https://woodstockpower.com/blog/power-plant-generators/(Accessed March 25, 2024).
PV System Batteries (2024). Solardirect. Available at: https://www.solardirect.com/archives/pv/batteries/batteries.htm (Accessed March 25, 2024).
Quansah, A., Dogbey, F., Asilevi, P. J., Boakye, P., Darkwah, L., Oduro-Kwarteng, S., et al. (2022). Assessment of solar radiation resource from the NASA-POWER reanalysis products for tropical climates in Ghana towards clean energy application. Sci. Rep. 12, 10684. doi:10.1038/s41598-022-14126-9
Series Vs Parallel Solar Panels Connections Explained – Helius Hub (2024). Heliushub. Available at: https://heliushub.com/solar-panels-series-vs-parallel-connections-explained/(Accessed April 3, 2024).
Settanni, E., and Tassielli, G. (2012). LifeCycleCosting(LCC)_Tassielli.indd. Available at: https://www.researchgate.net/publication/275275189.
Solar Photovoltaic Technology Basics | Department of Energy (2024). Energy. Available at: https://www.energy.gov/eere/solar/solar-photovoltaic-technology-basics (Accessed March 25, 2024).
Solar PV Modules (2024). Solardirect. Available at: https://www.solardirect.com/archives/pv/pvlist/pvlist.htm (Accessed March 25, 2024).
Storage in PV Systems PVEducation (2024). Pveducation. Available at: https://www.pveducation.org/pvcdrom/batteries/storage-in-pv-systems (Accessed March 25, 2024).
The Reliability Debate: Renewables Sustainable Review (2024). Sustainablereview. Available at: https://sustainablereview.com/the-reliability-debate-renewables/(Accessed March 25, 2024).
Tijani, H. O., Wei Tan, C., and Bashir, N. (2014). Techno-economic analysis of hybrid photovoltaic/diesel/battery off-grid system in northern Nigeria. J. Renew. Sustain. Energy 6 (3), 033103. doi:10.1063/1.4873122
Ukoima, K. N., Owolabi, A. B., Yakub, A. O., Same, N. N., Suh, D., and Huh, J. S. (2023). Analysis of a solar hybrid electricity generation system for a rural community in river state, Nigeria. Energies (Basel) 16 (8), 3431. doi:10.3390/en16083431
Understanding PV Module Performance Characteristics - Technical Articles (2024). Eepower. Available at: https://eepower.com/technical-articles/pv-module-performance-characteristics/# (Accessed March 2, 2024).
UNITED STATES ENVIRONMENTAL PROTECTION AGENCY (2012) ORD/NRMRL/Sustainable technology division systems analysis branch tool for the reduction and assessment of chemical and other environmental impacts (TRACI) software name and version number: TRACI version 2.1 USER’S MANUAL.
White, J. G., Samikannu, R., Oladiran, M. T., Yahya, A., Makepe, P., Gamariel, G., et al. (2024). Techno-economic feasibility assessment and performance analysis of standalone solar photo voltaic-biomass hybrid system with optimized storage: a case study—grand Bassa, Liberia. Front. Energy Res. 12. doi:10.3389/fenrg.2024.1326558
Wind and solar are not intermittent they are unreliable, unpredictable, uncontrollable and worthless Atomic Insights (2024). Atomicinsights. Available at: https://atomicinsights.com/wind-they-are-unreliable-unpredictable-and-uncontrollable/(Accessed March 25, 2024).
Yimen, N., Tchotang, T., Kanmogne, A., Idriss, I. A., Musa, B., Aliyu, A., et al. (2020). Optimal sizing and techno-economic analysis of hybrid renewable energy systems—a case study of a photovoltaic/wind/battery/diesel system in Fanisau, Northern Nigeria. Processes 8 (11), 1381–1425. doi:10.3390/pr8111381
Keywords: hybrid energy systems, feasibility analysis, environmental assessment, economic assessment, life cycle cost, levelized cost of energy, energy systems decarbonization
Citation: Ijeoma MW, Lewis CG, Chen H, Chukwu BN and Carbajales-Dale M (2024) Technical, economic, and environmental feasibility assessment of solar-battery-generator hybrid energy systems: a case study in Nigeria. Front. Energy Res. 12:1397037. doi: 10.3389/fenrg.2024.1397037
Received: 06 March 2024; Accepted: 25 April 2024;
Published: 14 May 2024.
Edited by:
Siamak Hoseinzadeh, Sapienza University of Rome, ItalyReviewed by:
Saman Faramarzi, Islamic Azad University, West Tehran, IranCopyright © 2024 Ijeoma, Lewis, Chen, Chukwu and Carbajales-Dale. This is an open-access article distributed under the terms of the Creative Commons Attribution License (CC BY). The use, distribution or reproduction in other forums is permitted, provided the original author(s) and the copyright owner(s) are credited and that the original publication in this journal is cited, in accordance with accepted academic practice. No use, distribution or reproduction is permitted which does not comply with these terms.
*Correspondence: Muzan Williams Ijeoma, bWlqZW9tYUBjbGVtc29uLmVkdQ==
Disclaimer: All claims expressed in this article are solely those of the authors and do not necessarily represent those of their affiliated organizations, or those of the publisher, the editors and the reviewers. Any product that may be evaluated in this article or claim that may be made by its manufacturer is not guaranteed or endorsed by the publisher.
Research integrity at Frontiers
Learn more about the work of our research integrity team to safeguard the quality of each article we publish.